- 1Laboratorio Interacción Ambiente-Microorganismo, Centro de Biotecnología Genómica, Instituto Politécnico Nacional, Tamaulipas, Mexico
- 2Laboratorio de Investigación Clínica y Ambiental, Departamento de Microbiología, Escuela Nacional de Ciencias Biológicas, Instituto Politécnico Nacional, Mexico City, Mexico
- 3Centro Interdisciplinario de Investigación Para el Desarrollo Integral Regional, Unidad Michoacán, Instituto Politécnico Nacional, Jiquilpan, Mexico
- 4Facultad de Medicina Veterinaria, Universidad Autónoma de Nuevo León, San Nicolás de los Garza, Mexico
- 5Research Department, Universidad del Valle de México, Campus Reynosa, Mexico City, Mexico
The CRISPR-Cas [clustered regularly interspaced short palindromic repeats and the CRISPR-associated genes (Cas)] system provides defense mechanisms in bacteria and archaea vs. mobile genetic elements (MGEs), such as plasmids and bacteriophages, which can either be harmful or add sequences that can provide virulence or antibiotic resistance. Staphylococcus aureus is a Gram-positive bacterium that could be the etiological agent of important soft tissue infections that can lead to bacteremia and sepsis. The role of the CRISPR-Cas system in S. aureus is not completely understood since there is a lack of knowledge about it. We analyzed 716 genomes and 1 genomic island from GENOMES-NCBI and ENA-EMBL searching for the CRISPR-Cas systems and their spacer sequences (SSs). Our bioinformatic analysis shows that only 0.83% (6/716) of the analyzed genomes harbored the CRISPR-Cas system, all of them were subtype III-A, which is characterized by the presence of the cas10/csm1 gene. Analysis of SSs showed that 91% (40/44) had no match to annotated MGEs and 9% of SSs corresponded to plasmids and bacteriophages, indicating that those phages had infected those S. aureus strains. Some of those phages have been proposed as an alternative therapy in biofilm-forming or infection with S. aureus strains, but these findings indicate that such antibiotic phage strategy would be ineffective. More research about the CRISPR/Cas system is necessary for a bigger number of S. aureus strains from different sources, so additional features can be studied.
Introduction
The bacteria and archaea have developed defense mechanisms against bacteriophages (Labrie et al., 2010), in the form of restriction and modification system (R-M system; Huff et al., 2017) and as the CRISPR-Cas [clustered regularly interspaced short palindromic repeats and the CRISPR-associated genes (Cas)] system, both of which degrade the foreign invader genetic material. The CRISPR-Cas is a natural, memory, and hereditary mechanism that protects bacteria against bacteriophages (Faruque et al., 2005; Box et al., 2015; Hille et al., 2018). It is composed of (1) a group of genes cas, (2) a locus or loci, CRISPR formed by spacer sequences (SSs) separated into repeated sequences (SRs), and (3) the leader sequence placed upstream from locus CRISPR (Westra et al., 2014); the set of cas genes is divided into the module of adaptation formed by cas1 and cas2 genes and the effector complex where the rest of the cas genes are placed (Koonin et al., 2017).
The system CRISPR-Cas current classification includes 2 classes, 6 types, and 33 subtypes. Class 1 systems use multi-unit protein complexes (Koonin and Makarova, 2017; Koonin et al., 2017) and Class 2 systems use only one multidomain protein (Shmakov et al., 2017) for the degradation of the genetic material. This DNA degradation occurs in three stages (Hsu et al., 2014): (1) adaptation stage during a primo-infection (Nuñez et al., 2014), (2) expression stage during reinfection, and (3) interference stage for the digestion mobile genetic element (MGE) through endonucleases Cas, which are guided by crRNA (chimera of SE and SR; Hille et al., 2018). In a MGE, there are short sequences (approximately 30 nucleotides) marked by protospacer adjacent motifs (Jiang and Doudna, 2015, 2017), known as protospacers, which are inserted like a SS.
The CRISPR-Cas system has been detected in Gram-positive bacteria, such as Lactobacillus spp. (Wang et al., 2020; Yang et al., 2020) and pathogenic bacteria, such as Enterococcus spp. (Sanderson et al., 2020). However, in Staphylococcus aureus, it has only been detected in few strains. S. aureus is a Gram-positive bacterium that colonizes 30% of the population in an asymptomatic way, and also it is the etiological agent of several important infections (Craft et al., 2019). In 1960, the methicillin-resistant S. aureus (MRSA) strains were detected (Chambers and Deleo, 2009), and those are still a current cause of soft tissue infections. New effective antibiotic therapies are a current demand (Yang et al., 2018; Labruère et al., 2019). The application of bacteriophages as a therapy to treat S. aureus infections (Kaźmierczak et al., 2014) is a promising alternative. The memory capacity of the CRISPR-Cas system allows us to understand the dynamic between an MGE and its hosts (bacteria and archaea). The sequenced bacterial genomes are the current data source for searching CRISPR-Cas system in important medical bacteria such as S. aureus. Despite studies searching CRISPR-Cas system in S. aureus (Cao et al., 2016; Zhao et al., 2018; Rossi et al., 2019), further research is needed to study this system in more S. aureus strains to understand the effects and its association to pathogenicity. Thus, the aim of this study was to search CRISPR-Cas in S. aureus genomes and its characterization via bioinformatic tools, as well as the association of the SS with MGEs.
Materials And Methods
Genomes Collection
The complete S. aureus genomes were downloaded from GENOME-NCBI [n = 864 (484 chromosomes and 380 plasmids)] and ENA-EMBL [n = 521 (232 chromosomes, 288 plasmids, and 1 pathogenicity island)]. In total, there were 716 strains used.
CRISPR-Cas System Determination
The genomes were analyzed using CRISPRCasFinder 4.2.2 (Grissa et al., 2007; Abby et al., 2014; Couvin et al., 2018). The server was used with default parameters: minimal repeat length 23 bp, maximal repeat length 55 bp, repeat mismatch, minimal spacer size in function of repeat size 0.6, maximal spacer size in function of repeat size 2.5, maximally allowed percentage of similarity between spacers 60, percentage mismatches allowed between repeats 20, percentage mismatches allowed for truncated repeat 33. Also, a default 100 bp size of flanking regions in all potential CRISPR arrays was included. A CRISPR-Cas system that presents a group of genes cas and the locus CRISPR with a score of 3 and 4 were considered for the next analysis.
Cas1, Cas2, Cas6, and Cas10 Phylogenetic Analysis Proteins
The files containing the coding sequences of each CRISPR-Cas system-bearing genome were downloaded from GENOME-NCBI. The cas genes were obtained from those files and translated into MEGA-X by using the standard genetic code. Later, the Cas proteins were aligned to the program Clustal O of Unipro UGENE. The scores “pair sum” were calculated in GeneDoc. The best alignments showed lower scores. Subsequently, the phylogenetic trees were built by the UPGMA method using default parameters with 1,000 bootstrap in the program MEGA-X (Kumar et al., 2018).
Cas Protein Analysis
The Cas sequences annotated images were generated in the program EasyFig 2.2.5 (Sullivan et al., 2011).
Phylogenetic Analysis of the Repeated Sequences
The analysis was the same process as the Cas proteins, except that we used the neighbor-joining method with default parameters to build the phylogenetic tree, using MEGAX (Rose et al., 2019).
Secondary Structures of Repeat Sequence Consensus
The secondary structures of repeat sequence consensus (SRc) and the minimum free energy (MFE) were obtained from the RNAfold web server (http://rna.tbi.univie.ac.at//cgi-bin/RNAWebSuite/RNAfold.cgi; Lorenz et al., 2011). The logo of SRc was obtained from WebLogo (Schneider and Stephens, 1990; Crooks et al., 2004).
Spacer Sequence Analysis
The FASTA files were downloaded from CRISPR-CasFinder. Spacer sequence (SE) were submitted to BLAST, and the results associated with the MGE were the ones considered with expected values (e-values) minor or equal to 0.0001 and scores above 40 (Ostria-Hernández et al., 2015). Next, a 0 and 1 matrix was developed, 1 being the cell where the MGE and S. aureus strain intercept. That matrix was analyzed in the ClustVis server using default parameters. Then, a heat map was elaborated with the webserver ClustVis (Metsalu and Vilo, 2015), where the MGE known as the aforementioned infected strain of S. aureus was appreciated.
Results
The CRISPR-Cas system was searched in 1,385 sequences of S. aureus, including chromosomes, plasmids, and 1 pathogenicity island, collected from 2 databases (Supplementary Table A). The search showed that 0.83% (6/716) of S. aureus strains harbored the CRISPR-Cas system. The strains harboring the CRISPR-Cas system were S. aureus 08BA02176 (NC_018608), S. aureus KUH140087 (NZ_AP020315), S. aureus JS395 (NZ_CP012756), S. aureus AR_0470 (NZ_CP029653), S. aureus AR_0472 (NZ_CP029649), and S. aureus AR_0473 (NZ_CP029681). All these strains have different geographical origin: S. aureus 08BA02176 was isolated from a surgery infection in 2008 from a Canadian patient (Golding et al., 2012); S. aureus KUH140087 was isolated in 2014 from a septicemia patient in Kyoto, Japan (Hikichi et al., 2019); S. aureus JS395 was isolated in 2008 from a patient in Switzerland (Larsen et al., 2017), and the S. aureus strains AR_0472, AR_0470, and AR_0473 were sent by the Center for Disease Control and Prevention. While the source of S. aureus AR_0472, AR_0470, and AR_0473 is uncertain, the rest of the strains come from clinic sources. All the detected CRISPR-Cas systems were found in chromosome structures, and none were detected in the pathogenicity island; nevertheless, other islands had it (Chakraborty et al., 2009; Carpenter et al., 2017). The detected systems found belong to the III-A subtype, which is characterized by the gen cas10/csm1 and cas genes ordered as shown in Figure 1 (Koonin et al., 2017). The detected CRISPR-Cas system structure was as follows: (1) cas genes nearby the locus CRISPR and (2) scores of 3 and 4 in the CRISRPRCasFinder scale (Pourcel et al., 2020; Supplementary Table B). The strain contains a group of cas genes (Figure 1) near the locus CRISPR-Cas; the CRISPR locus and the cluster cas are separated by 73 nt (strains JS395 and AR_0470), 74 nt (strains 08BA02176, AR_0472, and AR_0473), and 133 nt (strain KUH140087). Each strain with CRISPR-Cas has a unique locus CRISPR with a different number of SS.

Figure 1. The cluster of genes cas of the CRISPR-Cas subtype III-A of S. aureus system. The annotation genes cas was done in EasyFig 2.2.5.
The SRc was the same in two strains (i.e., AR_0472 and AR_0473) and different in four strains. The SRc length was 36 and 37 nt; the SRc formed by 37 nt is shown in the strains JS395 and AR_0479. The SRc remains nucleotide motifs (Figure 2) that stand out among the conservative nucleotides: four consecutive nucleotides of cysteine (-CCCC-) and four consecutive nucleotides of guanine (-GGGG-). Among the conserved motifs, there is a constant region of eight nucleotides.
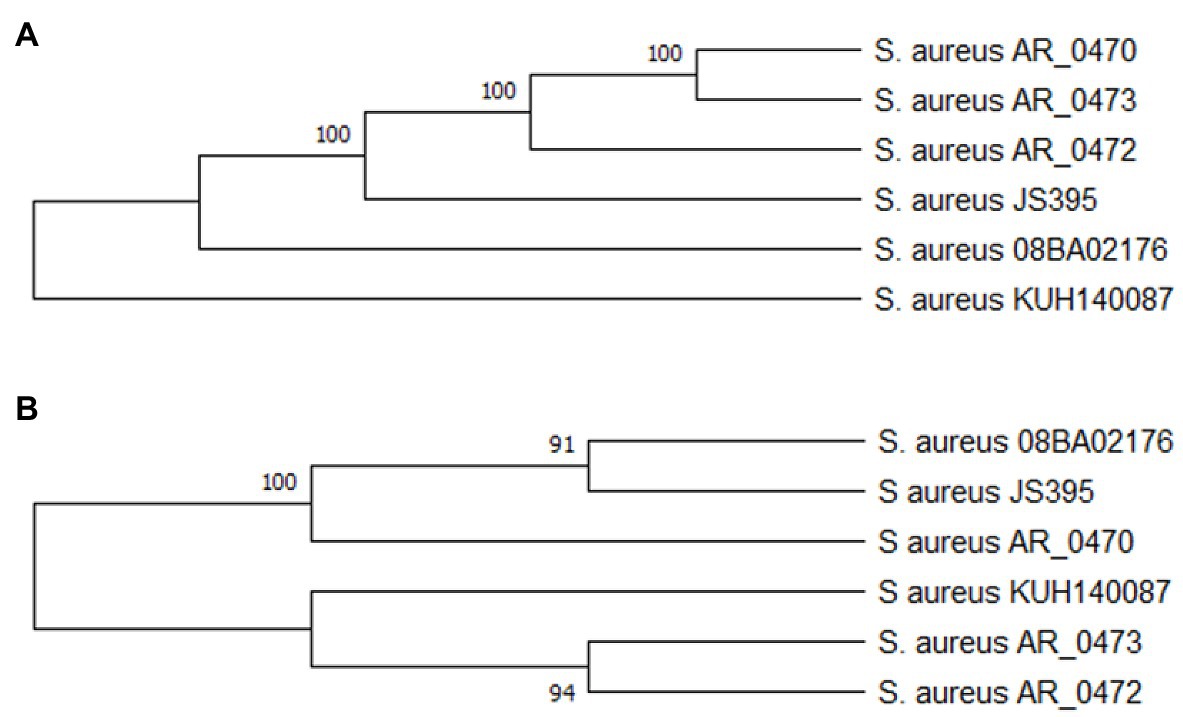
Figure 2. Protein Cas phylogenetic relation (A) and SRc (B). The alignment of the amino acid sequences of the proteins Cas and SRc was done with ClustalO, and the phylogenetic trees of SRc and the Cas proteins were built with the neighbor-joining and UPGMA method. There is a tree for the protein Cas (Cas1, Cas2, Cas10/Csm1, and Cas6) because they presented the same phylogenetic relation. The trees are the consensus of 1,000 bootstrap, and they were done with MEGA X.
The Cas proteins and SR keep a function–structure relationship (stem-loop structure); the coevolution of both structures is necessary for the correct function of the system CRISPR-Cas. Figures 3A,B show the phylogenetic relations of the Cas proteins and SRc, respectively. The Cas proteins and SRc present in S. aureus KUH140087 are phylogenetically away from the ones present in the phylogroup formed by the rest of the strains.
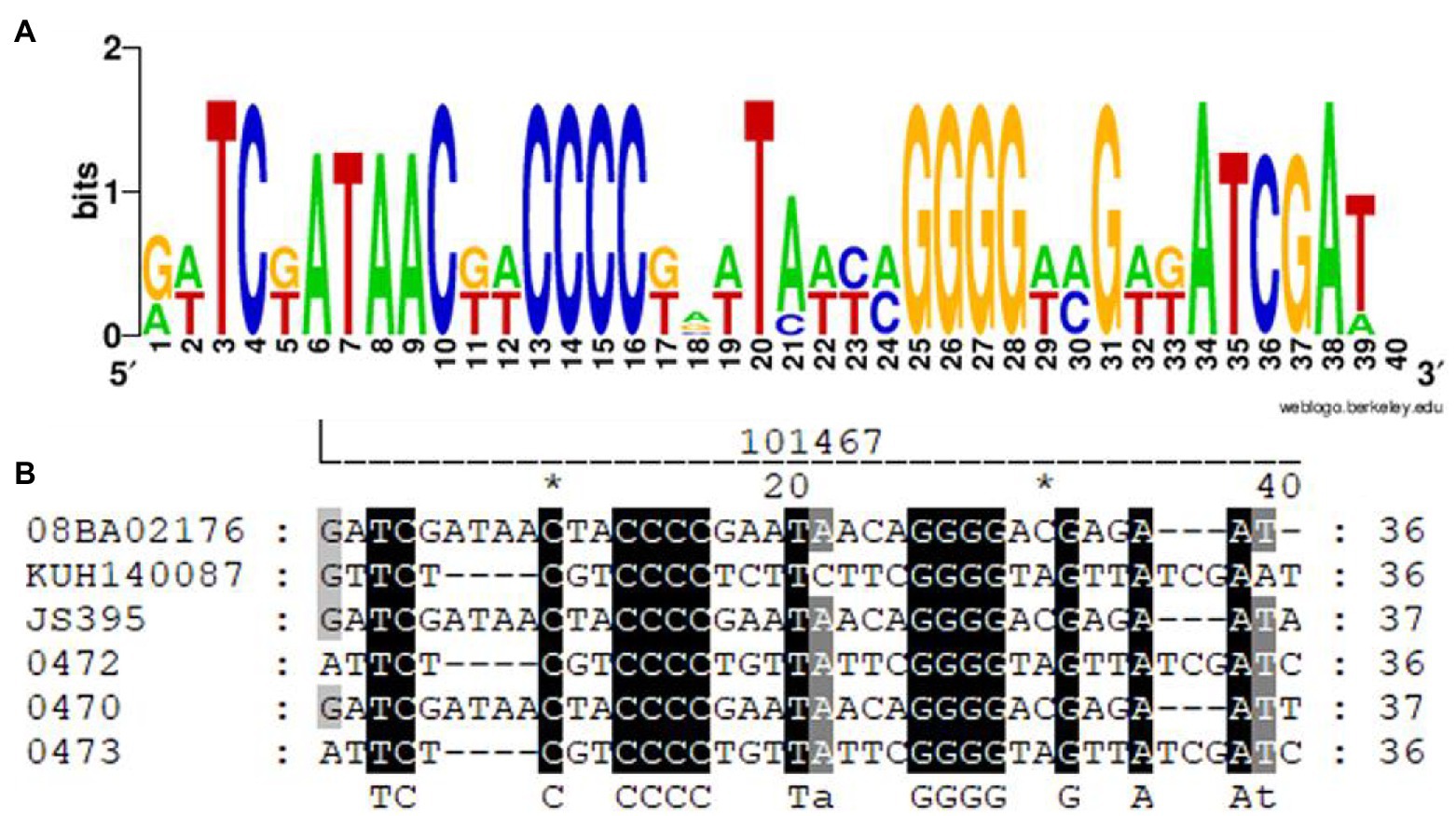
Figure 3. SRc alignment of the CRISPR-Cas system present in S. aureus. Visualization (A) and alignment of nucleotides (B). The motive nucleotides are under the alignment (capital letters). The alignment was done with MUSCLE (UGENE), and the image was obtained from WebLogo (A) and GeneDoc (B).
The SR keeps the nucleotides that form the stem-loop structure, which gives the signals of the location where the cuts must be done on pre-crRNA. Figure 4 shows the SRc secondary structures of the CRISPR-Cas systems found; the stem formed by interactions G:C (guanine:cysteine) can be seen, and the loop also indicates the MFE of each structure.
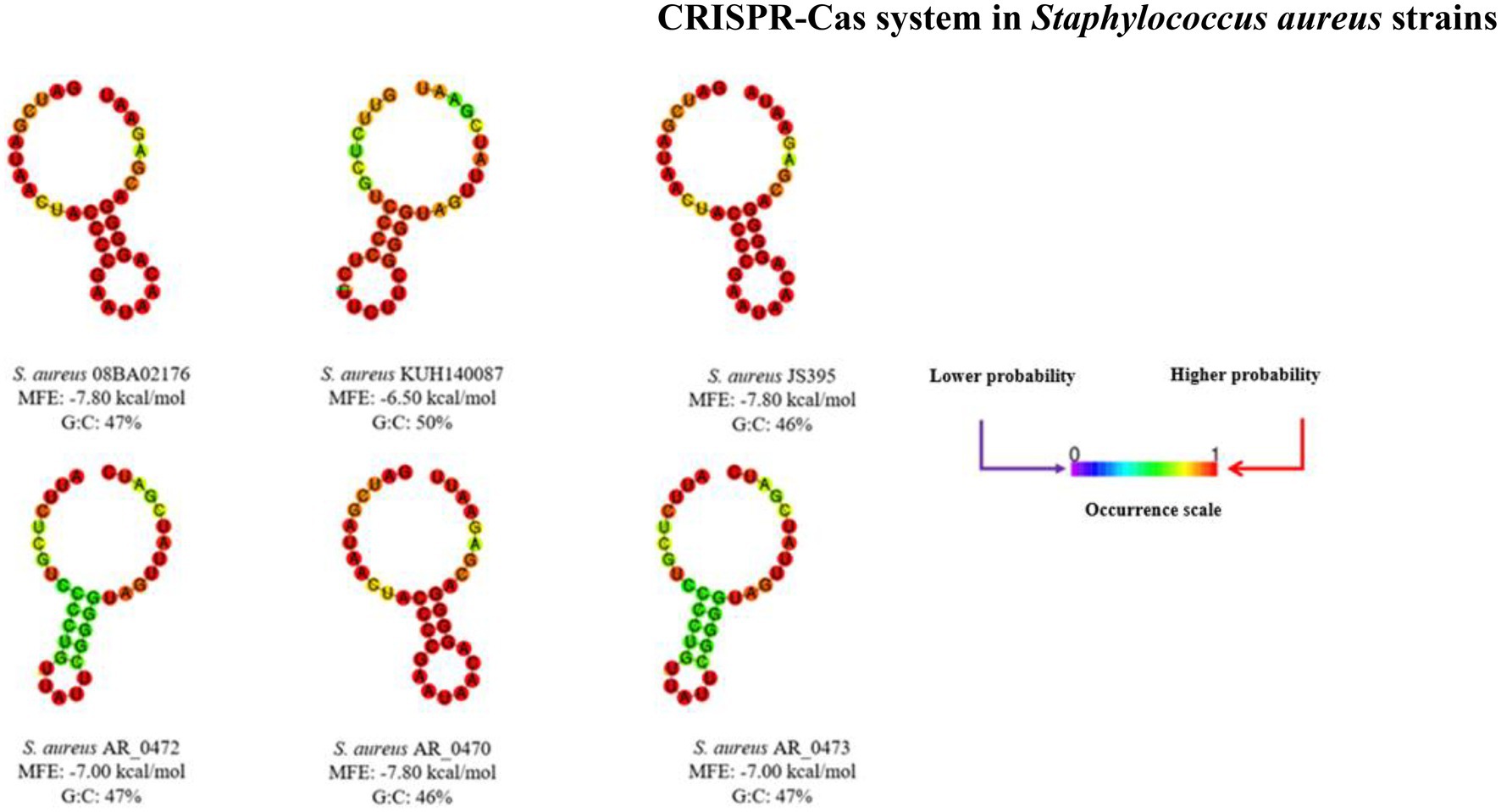
Figure 4. Secondary structures of repeated sequences. Each secondary structure is the result of the interactions of the nucleotides; these structures and the minimum free energy were obtained in the RNAfold server. It shows the scale of occurrence for each nucleotide interaction.
The memory and adaptation characteristics of the CRISPR-Cas system allow the bacteria to identify which MGE infected it. The subtraction of the protospacer from the MGE and its incorporation as SS in the locus CRISPR during the adaptation phase (McGinn and Marraffini, 2019) becomes an advantage in the genomic analysis. The total of SS [6 SS (strain JS395), 12 SS (strain AR_0470, AR_0472, and AR_0473), and 15 SS (strain 08BA02176)] is 62, where 26 (41.93%) are unique SS and 18 are SS duplicated and built 58.07%. Interestingly, the duplicated SSs are in the loci of strains AR_0472, AR_0473, JS395, and AR_0470. The SSs are preserved between the loci CRISPR: the repeated SSs of the strain AR_0472 (n = 12) match in order and sequence with the SS of the strain AR_0473 (n = 12), and the loci CRISPR of the strain JS395 (n = 6) also matches in order and sequence with a final 50% of loci CRISPR of strain AR_0470 (n = 12). Hence, only 44 SSs were considered for BLAST analysis.
The BLAST analysis showed that 9% (4/44) of SSs match with known MGE (Supplementary Table C). In Figure 5, MGEs that infected the S. aureus 08BA02176 (SS6) and S. aureus KUH140087 (SS1, SS2, and SS3) are presented. However, if a SS is associated with more than one MGE, it means that the protospacers are conserved between MGEs. Besides, according to our analysis, a specific protospacer can be found in plasmids or bacteriophages, but a plasmid protospacer is not found in a bacteriophage and vice versa. The SS1 of S. aureus KUH140087 is the only one that interferes with the two plasmids named in Figure 5, and the rest of SS interferes with the bacteriophage.
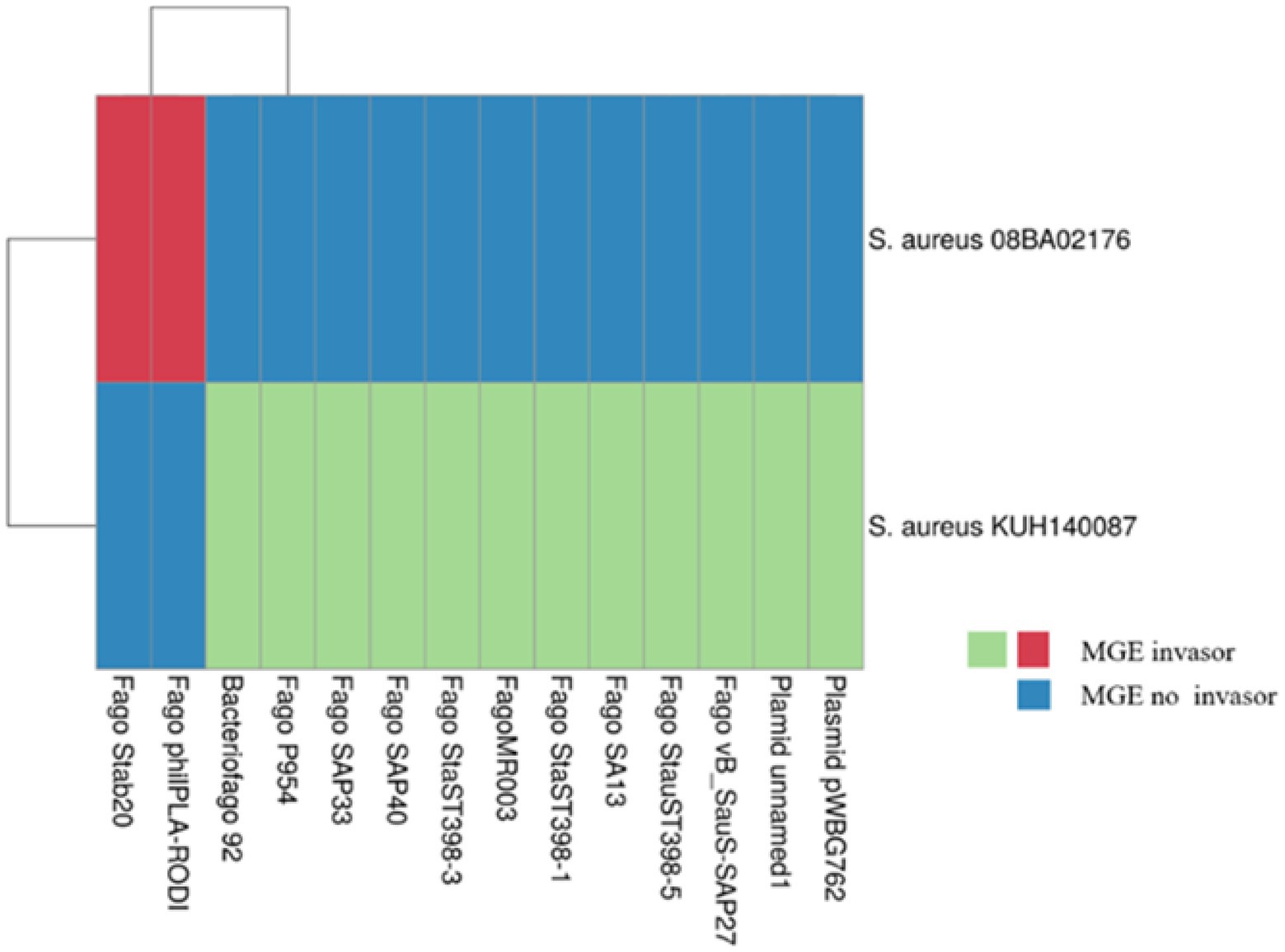
Figure 5. CRISPR-Cas ported strains that exhibited information of known mobile genetic elements (MGE). The annotations of the heat map were appended. The strains shown are unique, in which some of the spacer sequences were associated with an MGE through BLAST. The map was obtained in ClusVis.
Discussion
The CRISPR-Cas system is a heritable mechanism of immunity in bacteria and archaea, which protects them from foreign plasmids and bacteriophages; it is an endonuclease mechanism guided by crRNA (Makarova et al., 2013). Few studies have searched for the CRISPR-Cas system in Staphylococcus spp., where the CRISPR-Cas system was found in 0.94% (6/616) of isolated clinics (Cao et al., 2016) and in 7.89% (3/39) of the S. aureus strains analyzed for Zhao et al. (2018); moreover, the CRISPR-Cas system was searched in 129 isolated from Staphylococcus spp. (S. aureus n = 53, Staphylococcus pseudintermedius n = 74, Staphylococcus haemolyticus n = 1, and Staphylococcus cohnii n = 1) from 9 countries, and the 8% (10/129) are CRISPR-Cas system-bearing strains, but it was detected only in S. pseudintermedius strains (Rossi et al., 2019). Few studies have searched the CRISPR-Cas system in MGE, such as plasmids (Kamruzzaman and Iredell, 2020) or bacteriophages (Naser et al., 2017). The existence of the CRISPR-Cas system in a minimalist form, inactive, partially active, or active in MGE is the result of the constant coevolution between microorganisms and MGE (Faure et al., 2019), or due to competency between plasmids as a plasmid incompatibility mechanism (Kamruzzaman and Iredell, 2020).
In this study, the CRISPR-Cas system was found in six S. aureus strains. Interestingly, the strains were isolated from different countries: S. aureus 08BA02176 in Canada (Golding et al., 2012), S. aureus KUH140087 in Kyoto, Japan (Hikichi et al., 2019), S. aureus JS395 in Switzerland (Larsen et al., 2017), and the S. aureus AR_0472, AR_0470, and AR_0473 strains, whose geographical origin is unknown. The few CRISPR-Cas-bearing strains and their different geographical origin led us to think that the CRISPR-Cas system in S. aureus might be a spontaneous biological phenomenon, which means that the CRISPR-Cas system found in this study might be part of a bacterium that lives together with S. aureus. It has been demonstrated that in S. aureus 08BA02176, S. schleiferi TSCC54, and S. capitis CR01, the CRISPR-Cas system is inside the staphylococcal chromosomic cassette (SSC) SSCmec. The SSCmec is flanked by insertion sequences (IS), in S. aureus 08BA02176 by IS6 and ISL3, in S. schleiferi TSCC54 by IS6 and IS1182, and in S. capitis CR01 by an IS6, and the presence of the MGE mentioned indicates that the CRISPR-Cas system has been transferred horizontally to other strains and species of Staphylococcus (Rossi et al., 2017). The results of this study support the proposal of Rossi et al. (2017) and allow us to postulate that the CRISPR-Cas in S. aureus might be a spontaneous event consequence of a horizontal transfer of the SCCmec because of the low number of strains harboring the CRISPR-Cas system and their different geographical regions. Further evidence of horizontal transfer of the CRISPR-Cas system through SCCmec requires additional bioinformatic analysis and its in vitro demonstration.
The CRISPR-Cas systems in the S. aureus strains analyzed in this study are classified as subtype III-A, since the gen cas10/csm1 is found (Koonin et al., 2017). Studies have been demonstrated that the HD dominion of the protein Cas10/Csm1 is responsible for the activity ssDNasa and the protein Csm3 of the activity endoribonucleases (Tamulaitis et al., 2017). The crRNA is essential for the operation of the CRISPR-Cas system (Behler and Hess, 2020). Figures 3A,B show that the Cas protein and the SRc coevolution comply with the correct functioning of the CRISPR-Cas system and that the stem-loop structure is conserved; in the alignment (Figure 2B) of the SRc, it has been demonstrated that the presence of conserved motifs is formed by four cysteines and four guanines that flanked an inner region of eight nucleotides. Motifs rich C and G can interact to form a pair of C:G, which has also been observed in Proteus spp. (Qu et al., 2019). The alignments of SRc evidence the conserved motifs (Yang et al., 2020) that interact to generate secondary structures (Figure 4), which are characterized by the stem-loop structure (Bhaya et al., 2011) that serves as a point to process the pre-crRNA through the endonuclease Cas6 (Wakefield et al., 2015). The secondary structure stability is bigger as far as there are more G:C interactions and less MFE (Trotta, 2014); nevertheless, the nucleotides bound in different forms to G:C, so there are also stable structures (Yang et al., 2015; Negahdaripour et al., 2017).
Multidrug-resistant (MDR) strains arise because exposition to antimicrobial compound (AMC) in the environment selects them (Vuotto et al., 2018; Sanderson et al., 2020), as well as horizontal AMC gene transfer (Zarei-Baygi et al., 2019) through MGE (Baker et al., 2018). This relation between ARG and MGE is difficult for the therapy of MDR bacterial infections (Vuotto et al., 2018). S. aureus strains that contain the CRISPR-Cas system are detected in this study, three are from a clinical origin (08BA02176, JS395, and KUH140087), and the origin of the rest (AR_0470, AR_0472, and AR_0473) is unknown. The presence of antimicrobial resistance (AMR) in the environment may be the result of its incorrect use, for instance, the livestock industry and the pig industry, where they are used for animal breeding (Zhu et al., 2013) as well as their indiscriminate use to treat infection diseases (Saha et al., 2019) or their long-lasting use in severe or chronic treatments (Karaiskos et al., 2019). The cross pollution favors the outcome of MDR to different places far from its origin (Uhlemann et al., 2017; Aeksiri et al., 2019; Cohen et al., 2019). The effort and the economic consumption to the development of antimicrobial products (Chung and Khanum, 2017; Hashemi et al., 2018), mainly those are effective against MDR strains with metal in the form of a nanoparticle (Alavi and Rai, 2019; Heidary et al., 2019; Kumar et al., 2019), have promoted the search of new treatments, particularly the treatment of bacteriophages (Wernicki et al., 2017). The bacteriophages are being considered as an alternative to therapy in S. aureus MDR strains (MRSA), S. haemolyticus (MRSH), and Staphylococcus epidermidis (MRSE) infections (Oduor et al., 2020). However, in this study, we found that the CRISPR-Cas system may be a factor that could compromise the efficacy of bacteriophage therapy. The BLAST analysis of SE6 has shown that S. aureus 08BA02176 is capable of counteracting the Stab20 bacteriophages infection. Oduor et al. (2019) isolated the Stab20, Stab21, Stab22, and Stab23 bacteriophages, and later it was determined that Stab20 and Stab21 infected 41 and 40, among them, 100 Staphylococcus spp. (MRSA, MSSA, Staphylococcus intermedius, S. epidermidis, Staphylococcus saprophyticus, and S. haemolyticus) strains; moreover, it was found that Stab20 and Stab21 are better spread in some S. aureus strains. The Stab21 bacteriophage is capable to infect an isolated S. aureus from a patient with chronic sinusitis (Oduor et al., 2020). The presence of one SE that matches with Stab20 in the loci CRISPR of the 08BA02176 strain implies that infection with this strain would be difficult, or impossible to treat with a Stab20 bacteriophage therapy. Likewise, S. aureus 08BA02176 strain demonstrated its capability to destroy the ɸIPLA-RODI phage; this phage, when used against S. aureus forming a biofilm, caused a reduction of the population of S. aureus after 18 h (González et al., 2017); nevertheless, the presence of S. aureus 08BA02176 as part of the biofilm makes the use of the ɸIPLA-RODI phage difficult as a treatment. In contrast, it was demonstrated that the ɸMR003 phage infected 97% of the MRSA strains in the study of Peng et al. (2019); however, the CRISPR-Cas system of S. aureus KUH140087 prevents attack by the ɸMR003 phage.
Despite the ongoing protocols using the bacteriophages to treat infections caused by S. aureus (Kaźmierczak et al., 2014; Cui et al., 2017) in an animal model and human studies, it is necessary to generate more knowledge about the CRISPR-Cas system in more S. aureus strains to develop reliable bacteriophage therapies. Nowadays, only 12 S. aureus strains contain the reliable CRISPR-Cas system: AH1, AH2, AH3, SH1, SH2, and SH3 strains from isolated clinics (Cao et al., 2016), and the 08BA02176, KUH140087, JS395, AR_0470, AR_0472, and AR_0473 strains found in this investigation, as well as the ones previously found in the study by Cao et al. (2016) are 08BA02176 and JS395 strains as the CRISPR-Cas system carrier.
In conclusion, we determined that the CRISPR-Cas system found has an origin from other bacteria before getting into the different S. aureus strains detected in this study, due to its rare presence in clinical infections and its wide geographical countries where the CRISPR-Cas system was detected; moreover, the CRISPR-Cas system-bearing bacteria can destroy the bacteriophages becoming the limiting factor that could avoid the therapeutic use of the bacteriophages. Our results can be complemented with the CRISPR-Cas system detection in more S. aureus strains; thus, the enrichment of the database is to associate the memory of the CRISPR-Cas system with the bacteriophages and to discriminate among the best candidates for the curative therapies.
Data Availability Statement
The datasets presented in this study can be found in online repositories. The names of the repository/repositories and accession number(s) can be found in the article/Supplementary Material.
Author Contributions
EC-L and VB-G: design of the work. EC-L, MC-H, AM-V, and GC-E: acquisition and analysis of the data. EC-L, GR, WC-P: writing and revision of the content. EC-L, RF-M, and KV: writing of the content and contribution to figures. VB-G: approval of the last version. All authors contributed to the article and approved the submitted version.
Conflict of Interest
The authors declare that the research was conducted in the absence of any commercial or financial relationships that could be construed as a potential conflict of interest.
Supplementary Material
The Supplementary Material for this article can be found online at: https://www.frontiersin.org/articles/10.3389/fmicb.2021.656996/full#supplementary-material
References
Abby, S. S., Néron, B., Ménager, H., Touchon, M., and Rocha, E. P. (2014). MacSyFinder: a program to mine genomes for molecular systems with an application to CRISPR-Cas systems. PLoS One 9:e110726. doi: 10.1371/journal.pone.0110726
Aeksiri, N., Toanan, W., Sawikan, S., Suwannarit, R., Pungpomin, P., Khieokhajonkhet, A., et al. (2019). First detection and genomic insight into mcr-1 encoding plasmid-mediated colistin-resistance gene in Escherichia coli ST101 isolated from the migratory bird species Hirundo rustica in Thailand. Microb. Drug Resist. 25, 1437–1442. doi: 10.1089/mdr.2019.0020
Alavi, M., and Rai, M. (2019). Recent advances in antibacterial applications of metal nanoparticles (MNPs) and metal nanocomposites (MNCs) against multidrug-resistant (MDR) bacteria. Expert Rev. Anti-Infect. Ther. 17, 419–428. doi: 10.1080/14787210.2019.1614914
Baker, S., Thomson, N., Weill, F. X., and Holt, K. E. (2018). Genomic insights into the emergence and spread of antimicrobial-resistant bacterial pathogens. Science 360, 733–738. doi: 10.1126/science.aar3777
Behler, J., and Hess, W. R. (2020). Approaches to study CRISPR RNA biogenesis and the key players involved. Methods 172, 12–26. doi: 10.1016/j.ymeth.2019.07.015
Bhaya, D., Davison, M., and Barrangou, R. (2011). CRISPR-Cas systems in bacteria and archaea: versatile small RNAs for adaptive defense and regulation. Annu. Rev. Genet. 45, 273–297. doi: 10.1146/annurev-genet-110410-132430
Box, A. M., McGuffie, M. J., O’Hara, B. J., and Seed, K. D. (2015). Functional analysis of bacteriophage immunity through a type I-E CRISPR-Cas system in Vibrio cholerae and its application in bacteriophage genome engineering. J. Bacteriol. 198, 578–590. doi: 10.1128/JB.00747-15
Cao, L., Gao, C. H., Zhu, J., Zhao, L., Wu, Q., Li, M., et al. (2016). Identification and functional study of type III-A CRISPR-Cas systems in clinical isolates of Staphylococcus aureus. Int. J. Med. Microbiol. 306, 686–696. doi: 10.1016/j.ijmm.2016.08.005
Carpenter, M. R., Kalburge, S. S., Borowski, J. D., Peters, M. C., Colwell, R. R., and Boyd, E. F. (2017). CRISPR-Cas and contact-dependent secretion systems present on excisable pathogenicity islands with conserved recombination modules. J. Bacteriol. 199, e00842–e00816. doi: 10.1128/JB.00842-16
Chakraborty, S., Waise, T. M., Hassan, F., Kabir, Y., Smith, M. A., and Arif, M. (2009). Assessment of the evolutionary origin and possibility of CRISPR-Cas (CASS) mediated RNA interference pathway in Vibrio cholerae O395. In Silico Biol. 9, 245–254. doi: 10.3233/ISB-2009-0402
Chambers, H. F., and Deleo, F. R. (2009). Waves of resistance: Staphylococcus aureus in the antibiotic era. Nat. Rev. Microbiol. 7, 629–641. doi: 10.1038/nrmicro2200
Chung, P. Y., and Khanum, R. (2017). Antimicrobial peptides as potential anti-biofilm agents against multidrug-resistant bacteria. J. Microbiol. Immunol. Infect. 50, 405–410. doi: 10.1016/j.jmii.2016.12.005
Cohen, K. A., Manson, A. L., Abeel, T., Desjardins, C. A., Chapman, S. B., Hoffner, S., et al. (2019). Extensive global movement of multidrug-resistant M. tuberculosis strains revealed by whole-genome analysis. Thorax 74, 882–889. doi: 10.1136/thoraxjnl-2018-211616
Couvin, D., Bernheim, A., Toffano-Nioche, C., Touchon, M., Michalik, J., Néron, B., et al. (2018). CRISPRCasFinder, an update of CRISRFinder, includes a portable version, enhanced performance and integrates search for Cas proteins. Nucleic Acids Res. 46, W246–W251. doi: 10.1093/nar/gky425
Craft, K. M., Nguyen, J. M., Berg, L. J., and Townsend, S. D. (2019). Methicillin-resistant Staphylococcus aureus (MRSA): antibiotic-resistance and the biofilm phenotype. Med. Chem. Commun. 10, 1231–1241. doi: 10.1039/C9MD00044E
Crooks, G. E., Hon, G., Chandonia, J. M., and Brenner, S. E. (2004). WebLogo: a sequence logo generator. Genome Res. 14, 1188–1190. doi: 10.1101/gr.849004
Cui, Z., Guo, X., Dong, K., Zhang, Y., Li, Q., Zhu, Y., et al. (2017). Safety assessment of Staphylococcus phages of the family Myoviridae based on complete genome sequences. Sci. Rep. 7:41259. doi: 10.1038/srep41259
Faruque, S. M., Naser, I. B., Islam, M. J., Faruque, A. S., Ghosh, A. N., Nair, G. B., et al. (2005). Seasonal epidemics of cholera inversely correlate with the prevalence of environmental cholera phages. Proc. Natl. Acad. Sci. U. S. A. 102, 1702–1707. doi: 10.1073/pnas.0408992102
Faure, G., Shmakov, S. A., Yan, W. X., Cheng, D. R., Scott, D. A., Peters, J. E., et al. (2019). CRISPR-Cas in mobile genetic elements: counter-defence and beyond. Nat. Rev. Microbiol. 17, 513–525. doi: 10.1038/s41579-019-0204-7
Golding, G. R., Bryden, L., Levett, P. N., McDonald, R. R., Wong, A., Graham, M. R., et al. (2012). Whole-genome sequence of livestock-associated st398 methicillin-resistant Staphylococcus aureus isolated from humans in Canada. J. Bacteriol. 194, 6627–6628. doi: 10.1128/JB.01680-12
González, S., Fernández, L., Campelo, A. B., Gutiérrez, D., Martínez, B., Rodríguez, A., et al. (2017). The behavior of Staphylococcus aureus dual-species biofilms treated with bacteriophage phiIPLA-RODI depends on the accompanying microorganism. Appl. Environ. Microbiol. 83, e02821–e02816. doi: 10.1128/AEM.02821-16
Grissa, I., Vergnaud, G., and Pourcel, C. (2007). CRISPRFinder: a web tool to identify clustered regularly interspaced short palindromic repeats. Nucleic Acids Res. 35, W52–W57. doi: 10.1093/nar/gkm360
Hashemi, M. M., Mmuoegbulam, A. O., Holden, B. S., Coburn, J., Wilson, J., Taylor, M. F., et al. (2018). Susceptibility of multidrug-resistant bacteria, isolated from water and plants in Nigeria, to Ceragenins. Int. J. Environ. Res. Public Health 15:2758. doi: 10.3390/ijerph15122758
Heidary, M., Zaker Bostanabad, S., Amini, S. M., Jafari, A., Ghalami Nobar, M., Ghodousi, A., et al. (2019). The anti-mycobacterial activity of Ag, ZnO, And Ag-ZnO nanoparticles against MDR‐ and XDR-Mycobacterium tuberculosis. Infect. Drug Resist. 12, 3425–3435. doi: 10.2147/IDR.S221408
Hikichi, M., Nagao, M., Murase, K., Aikawa, C., Nozawa, T., Yoshida, A., et al. (2019). Complete genome sequences of eight methicillin-resistant Staphylococcus aureus strains isolated from patients in Japan. Microbiol. Resour. Announc. 8, e01212–e01219. doi: 10.1128/MRA.01212-19
Hille, F., Richter, H., Wong, S. P., Bratovič, M., Ressel, S., and Charpentier, E. (2018). The biology of CRISPR-Cas: backward and forward. Cell 172, 1239–1259. doi: 10.1016/j.cell.2017.11.032
Hsu, P. D., Lander, E. S., and Zhang, F. (2014). Development and applications of CRISPR-Cas9 for genome engineering. Cell 157, 1262–1278. doi: 10.1016/j.cell.2014.05.010
Huff, F., Muth, C., Naumer, C., and Meinhardt, F. (2017). The restriction modification system of Bacillus licheniformis MS1 and generation of a readily transformable deletion mutant. Appl. Microbiol. Biotechnol. 101, 7933–7944. doi: 10.1007/s00253-017-8532-x
Jiang, F., and Doudna, J. A. (2015). The structural biology of CRISPR-Cas systems. Curr. Opin. Struct. Biol. 30, 100–111. doi: 10.1016/j.sbi.2015.02.002
Jiang, F., and Doudna, J. A. (2017). CRISPR-Cas9 structures and mechanisms. Annu. Rev. Biophys. 46, 505–529. doi: 10.1146/annurev-biophys-062215-010822
Kamruzzaman, M., and Iredell, J. R. (2020). CRISPR-Cas system in antibiotic resistance plasmids in Klebsiella pneumoniae. Front. Microbiol. 10:2934. doi: 10.3389/fmicb.2019.02934
Karaiskos, I., Galani, L., Sakka, V., Gkoufa, A., Sopilidis, O., Chalikopoulos, D., et al. (2019). Oral fosfomycin for the treatment of chronic bacterial prostatitis. J. Antimicrob. Chemother. 74, 1430–1437. doi: 10.1093/jac/dkz015
Kaźmierczak, Z., Górski, A., and Dąbrowska, K. (2014). Facing antibiotic resistance: Staphylococcus aureus phages as a medical tool. Viruses 6, 2551–2570. doi: 10.3390/v6072551
Koonin, E. V., and Makarova, K. S. (2017). Mobile genetic elements and evolution of CRISPR-Cas systems: all the way there and back. Genome Biol. Evol. 9, 2812–2825. doi: 10.1093/gbe/evx192
Koonin, E. V., Makarova, K. S., and Zhang, F. (2017). Diversity, classification and evolution of CRISPR-Cas systems. Curr. Opin. Microbiol. 37, 67–78. doi: 10.1016/j.mib.2017.05.008
Kumar, S., Majhi, R. K., Singh, A., Mishra, M., Tiwari, A., Chawla, S., et al. (2019). Carbohydrate-coated gold-silver nanoparticles for efficient elimination of multidrug resistant bacteria and in vivo wound healing. ACS Appl. Mater. Interfaces 11, 42998–43017. doi: 10.1021/acsami.9b17086
Kumar, S., Stecher, G., Li, M., Knyaz, C., and Tamura, K. (2018). MEGA X: molecular evolutionary genetics analysis across computing platforms. Mol. Biol. Evol. 35, 1547–1549. doi: 10.1093/molbev/msy096
Labrie, S. J., Samson, J. E., and Moineau, S. (2010). Bacteriophage resistance mechanisms. Nat. Rev. Microbiol. 8, 317–327. doi: 10.1038/nrmicro2315
Labruère, R., Sona, A. J., and Turos, E. (2019). Anti-methicillin-resistant Staphylococcus aureus nanoantibiotics. Front. Pharmacol. 10:1121. doi: 10.3389/fphar.2019.01121
Larsen, J., Andersen, P. S., Winstel, V., and Peschel, A. (2017). Staphylococcus aureus CC395 harbours a novel composite staphylococcal cassette chromosome mec element. J. Antimicrob. Chemother. 72, 1002–1005. doi: 10.1093/jac/dkw544
Lorenz, R., Bernhart, S. H., Höner Zu Siederdissen, C., Tafer, H., Flamm, C., Stadler, P. F., et al. (2011). ViennaRNA Package 2.0. Algorithms Mol. Biol. 6:26. doi: 10.1186/1748-7188-6-26
Makarova, K. S., Wolf, Y. I., and Koonin, E. V. (2013). Comparative genomics of defense systems in archaea and bacteria. Nucleic Acids Res. 41, 4360–4377. doi: 10.1093/nar/gkt157
McGinn, J., and Marraffini, L. A. (2019). Molecular mechanisms of CRISPR-Cas spacer acquisition. Nat. Rev. Microbiol. 17, 7–12. doi: 10.1038/s41579-018-0071-7
Metsalu, T., and Vilo, J. (2015). ClustVis: a web tool for visualizing clustering of multivariate data using principal component analysis and heatmap. Nucleic Acids Res. 43, W566–W570. doi: 10.1093/nar/gkv468
Naser, I. B., Hoque, M. M., Nahid, M. A., Tareq, T. M., Rocky, M. K., and Faruque, S. M. (2017). Analysis of the CRISPR-Cas system in bacteriophages active on epidemic strains of Vibrio cholerae in Bangladesh. Sci. Rep. 7:14880. doi: 10.1038/s41598-017-14839-2
Negahdaripour, M., Nezafat, N., Hajighahramani, N., Rahmatabadi, S. S., and Ghasemi, Y. (2017). Investigating CRISPR-Cas systems in Clostridium botulinum via bioinformatics tools. Infect. Genet. Evol. 54, 355–373. doi: 10.1016/j.meegid.2017.06.027
Nuñez, J. K., Kranzusch, P. J., Noeske, J., Wright, A. V., Davies, C. W., and Doudna, J. A. (2014). Cas1-Cas2 complex formation mediates spacer acquisition during CRISPR-Cas adaptive immunity. Nat. Struct. Mol. Biol. 21, 528–534. doi: 10.1038/nsmb.2820
Oduor, J., Kadija, E., Nyachieo, A., Mureithi, M. W., and Skurnik, M. (2020). Bioprospecting Staphylococcus phages with therapeutic and bio-control potential. Viruses 12:133. doi: 10.3390/v12020133
Oduor, J., Kiljunen, S., Kadija, E., Mureithi, M. W., Nyachieo, A., and Skurnik, M. (2019). Genomic characterization of four novel Staphylococcus myoviruses. Arch. Virol. 164, 2171–2173. doi: 10.1007/s00705-019-04267-0
Ostria-Hernández, M. L., Sánchez-Vallejo, C. J., Ibarra-García, J. A., and Castro-Escarpulli, G. (2015). Survey of clustered regularly interspaced short palindromic repeats and their associated Cas proteins (CRISPR/Cas) systems in multiple sequenced strains of Klebsiella pneumoniae. BMC Res. Notes 8:332. doi: 10.1186/s13104-015-1285-7
Peng, C., Hanawa, T., Azam, A. H., LeBlanc, C., Ung, P., Matsuda, T., et al. (2019). Silviavirus phage ɸMR003 displays a broad host range against methicillin-resistant Staphylococcus aureus of human origin. Appl. Microbiol. Biotechnol. 103, 7751–7765. doi: 10.1007/s00253-019-10039-2
Pourcel, C., Touchon, M., Villeriot, N., Vernadet, J. P., Couvin, D., Toffano-Nioche, C., et al. (2020). CRISPRCasdb a successor of CRISPRdb containing CRISPR arrays and cas genes from complete genome sequences, and tools to download and query lists of repeats and spacers. Nucleic Acids Res. 48, D535–D544. doi: 10.1093/nar/gkz915
Qu, D., Lu, S., Wang, P., Jiang, M., Yi, S., and Han, J. (2019). Analysis of CRISPR/Cas system of Proteus and the factors affected the functional mechanism. Life Sci. 231:116531. doi: 10.1016/j.lfs.2019.06.006
Rose, R., Golosova, O., Sukhomlinov, D., Tiunov, A., and Prosperi, M. (2019). Flexible design of multiple metagenomics classification pipelines with UGENE. Bioinformatics 35, 1963–1965. doi: 10.1093/bioinformatics/bty901
Rossi, C. C., Andrade-Oliveira, A. L., and Giambiagi-deMarval, M. (2019). CRISPR tracking reveals global spreading of antimicrobial resistance genes by Staphylococcus of canine origin. Vet. Microbiol. 232, 65–69. doi: 10.1016/j.vetmic.2019.04.009
Rossi, C. C., Souza-Silva, T., Araújo-Alves, A. V., and Giambiagi-deMarval, M. (2017). CRISPR-Cas systems features and the gene-reservoir role of coagulase-negative staphylococci. Front. Microbiol. 8:1545. doi: 10.3389/fmicb.2017.01545
Saha, S., Tariq, R., Tosh, P. K., Pardi, D. S., and Khanna, S. (2019). Faecal microbiota transplantation for eradicating carriage of multidrug-resistant organisms: a systematic review. Clin. Microbiol. Infect. 25, 958–963. doi: 10.1016/j.cmi.2019.04.006
Sanderson, H., Ortega-Polo, R., Zaheer, R., Goji, N., Amoako, K. K., Brown, R. S., et al. (2020). Comparative genomics of multidrug-resistant Enterococcus spp. isolated from wastewater treatment plants. BMC Microbiol. 20:20. doi: 10.1186/s12866-019-1683-4
Schneider, T. D., and Stephens, R. M. (1990). Sequence logos: a new way to display consensus sequences. Nucleic Acids Res. 18, 6097–6100. doi: 10.1093/nar/18.20.6097
Shmakov, S., Smargon, A., Scott, D., Cox, D., Pyzocha, N., Yan, W., et al. (2017). Diversity and evolution of class 2 CRISPR-Cas systems. Nat. Rev. Microbiol. 15, 169–182. doi: 10.1038/nrmicro.2016.184
Sullivan, M. J., Petty, N. K., and Beatson, S. A. (2011). Easyfig: a genome comparison visualizer. Bioinformatics 27, 1009–1010. doi: 10.1093/bioinformatics/btr039
Tamulaitis, G., Venclovas, Č., and Siksnys, V. (2017). Type III CRISPR-Cas immunity: major differences brushed aside. Trends Microbiol. 25, 49–61. doi: 10.1016/j.tim.2016.09.012
Trotta, E. (2014). On the normalization of the minimum free energy of RNAs by sequence length. PLoS One 9:e113380. doi: 10.1371/journal.pone.0113380
Uhlemann, A. C., McAdam, P. R., Sullivan, S. B., Knox, J. R., Khiabanian, H., Rabadan, R., et al. (2017). Evolutionary dynamics of pandemic methicillin-sensitive Staphylococcus aureus ST398 and its international spread via routes of human migration. mBio 8, e01375–e01316. doi: 10.1128/mBio.01375-16
Vuotto, C., Donelli, G., Buckley, A., and Chilton, C. (2018). Clostridium difficile Biofilm. Adv. Exp. Med. Biol. 1050, 97–115. doi: 10.1007/978-3-319-72799-8_7
Wakefield, N., Rajan, R., and Sontheimer, E. J. (2015). Primary processing of CRISPR RNA by the endonuclease Cas6 in Staphylococcus epidermidis. FEBS Lett. 589, 3197–3204. doi: 10.1016/j.febslet.2015.09.005
Wang, S., Yang, B., Ross, R. P., Stanton, C., Zhao, J., Zhang, H., et al. (2020). Comparative genomics analysis of Lactobacillus ruminis from different niches. Genes 11:70. doi: 10.3390/genes11010070
Wernicki, A., Nowaczek, A., and Urban-Chmiel, R. (2017). Bacteriophage therapy to combat bacterial infections in poultry. Virol. J. 14:179. doi: 10.1186/s12985-017-0849-7
Westra, E. R., Buckling, A., and Fineran, P. C. (2014). CRISPR-Cas systems: beyond adaptive immunity. Nat. Rev. Microbiol. 12, 317–326. doi: 10.1038/nrmicro3241
Yang, L., Li, W., Ujiroghene, O. J., Yang, Y., Lu, J., Zhang, S., et al. (2020). Occurrence and diversity of CRISPR loci in Lactobacillus casei group. Front. Microbiol. 11:624. doi: 10.3389/fmicb.2020.00624
Yang, C., Lin, F., Li, Q., Li, T., and Zhao, J. (2015). Comparative genomics reveals diversified CRISPR-Cas systems of globally distributed Microcystis aeruginosa, a freshwater bloom-forming cyanobacterium. Front. Microbiol. 6:394. doi: 10.3389/fmicb.2015.00394
Yang, H., Xu, J., Li, W., Wang, S., Li, J., Yu, J., et al. (2018). Staphylococcus aureus virulence attenuation and immune clearance mediated by a phage lysin-derived protein. EMBO J. 37:e98045. doi: 10.15252/embj.201798045
Zarei-Baygi, A., Harb, M., Wang, P., Stadler, L. B., and Smith, A. L. (2019). Evaluating antibiotic resistance gene correlations with antibiotic exposure conditions in anaerobic membrane bioreactors. Environ. Sci. Technol. 53, 3599–3609. doi: 10.1021/acs.est.9b00798
Zhao, X., Yu, Z., and Xu, Z. (2018). Study the features of 57 confirmed CRISPR loci in 38 strains of Staphylococcus aureus. Front. Microbiol. 9:1591. doi: 10.3389/fmicb.2018.01591
Keywords: Staphylococcus aureus, methicillin-resistant Staphylococcus aureus, multidrug resistant, CRISPR-Cas system, phage therapy
Citation: Cruz-López EA, Rivera G, Cruz-Hernández MA, Martínez-Vázquez AV, Castro-Escarpulli G, Flores-Magallón R, Vázquez K, Cruz-Pulido WL and Bocanegra-García V (2021) Identification and Characterization of the CRISPR/Cas System in Staphylococcus aureus Strains From Diverse Sources. Front. Microbiol. 12:656996. doi: 10.3389/fmicb.2021.656996
Edited by:
Friedrich Götz, University of Tübingen, GermanyReviewed by:
Débora Coraça-Huber, Innsbruck Medical University, AustriaAnanda Shankar Bhattacharjee, University of Illinois at Urbana-Champaign, United States
Copyright © 2021 Cruz-López, Rivera, Cruz-Hernández, Martínez-Vázquez, Castro-Escarpulli, Flores-Magallón, Vázquez, Cruz-Pulido and Bocanegra-García. This is an open-access article distributed under the terms of the Creative Commons Attribution License (CC BY). The use, distribution or reproduction in other forums is permitted, provided the original author(s) and the copyright owner(s) are credited and that the original publication in this journal is cited, in accordance with accepted academic practice. No use, distribution or reproduction is permitted which does not comply with these terms.
*Correspondence: Virgilio Bocanegra-García, dmJvY2FuZWdnQGhvdG1haWwuY29t; dmJvY2FuZWdyYUBpcG4ubXg=