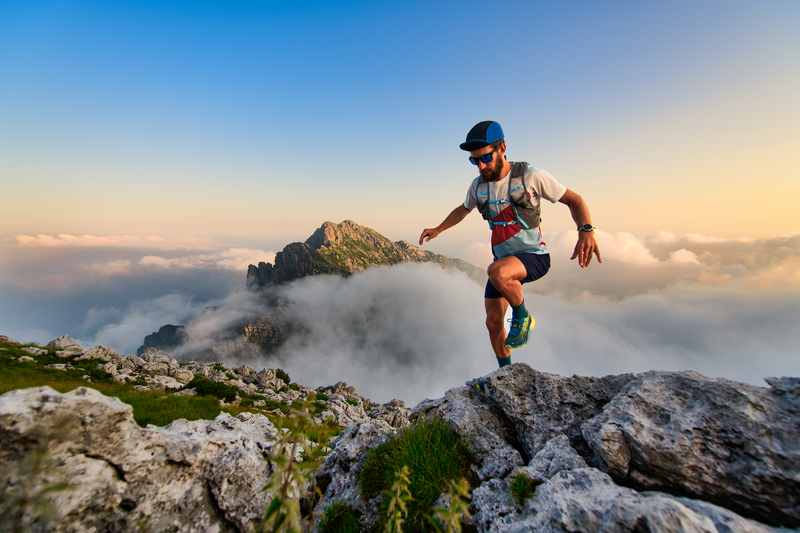
95% of researchers rate our articles as excellent or good
Learn more about the work of our research integrity team to safeguard the quality of each article we publish.
Find out more
ORIGINAL RESEARCH article
Front. Microbiol. , 09 April 2021
Sec. Antimicrobials, Resistance and Chemotherapy
Volume 12 - 2021 | https://doi.org/10.3389/fmicb.2021.656223
This article is part of the Research Topic Antimicrobials in Wildlife and the Environment View all 12 articles
Antimicrobial resistance (AMR) is one of the most important global health concerns; therefore, the identification of AMR reservoirs and vectors is essential. Attention should be paid to the recognition of potential hazards associated with wildlife as this field still seems to be incompletely explored. In this context, the role of free-living birds as AMR carriers is noteworthy. Therefore, we applied methods used in AMR monitoring, supplemented by colistin resistance screening, to investigate the AMR status of Escherichia coli from free-living birds coming from natural habitats and rescue centers. Whole-genome sequencing (WGS) of strains enabled to determine resistance mechanisms and investigate their epidemiological relationships and virulence potential. As far as we know, this study is one of the few that applied WGS of that number (n = 71) of strains coming from a wild avian reservoir. The primary concerns arising from our study relate to resistance and its determinants toward antimicrobial classes of the highest priority for the treatment of critical infections in people, e.g., cephalosporins, quinolones, polymyxins, and aminoglycosides, as well as fosfomycin. Among the numerous determinants, blaCTX–M–15, blaCMY–2, blaSHV–12, blaTEM–1B, qnrS1, qnrB19, mcr-1, fosA7, aac(3)-IIa, ant(3”)-Ia, and aph(6)-Id and chromosomal gyrA, parC, and parE mutations were identified. Fifty-two sequence types (STs) noted among 71 E. coli included the global lineages ST131, ST10, and ST224 as well as the three novel STs 11104, 11105, and 11194. Numerous virulence factors were noted with the prevailing terC, gad, ompT, iss, traT, lpfA, and sitA. Single E. coli was Shiga toxin-producing. Our study shows that the clonal spread of E. coli lineages of public and animal health relevance is a serious avian-associated hazard.
The scale of bacterial resistance to antimicrobials is one of the most important global health concerns. Although antimicrobial resistance (AMR) is an ancient and natural phenomenon, the widespread use of antimicrobials in human and veterinary medicine and also in agriculture contributes to its pandemic dissemination (D’Costa et al., 2011; Martínez, 2012; World Health Organization [WHO], 2014). The enormous consequences of AMR cover most of all treatment failures and increased mortality. However, economic losses as a consequence of AMR increase are also significant and they generate extra healthcare costs and a decrease of productivity (European Centre for Disease Prevention and Control, 2009). Nevertheless, losses in agriculture and animal production sector should also be listed here.
The use of antimicrobials (AMU) is considered one of the main drivers of AMR emergence; therefore, the impact of drug residues in municipal waste waters and of organic fertilizers in agriculture is indisputable (Swift et al., 2019). Several other environmental factors may affect AMR dissemination. Chemicals like disinfectants, fungicides, and pesticides widely used in agriculture may effectively co-select AMR (Hansen et al., 2007; European Commission, 2009; Hobman and Crossman, 2015). Similarly, heavy metals (e.g., copper) used in antifungal plant protection agents should also be deliberated (Borkow and Gabbay, 2005; Hobman and Crossman, 2015). All those pollutants contribute to the selection and spread of AMR in the environment affecting wildlife.
Consequently, free-living animals may serve as a reservoir of AMR determinants. The scale of AMR in wildlife, despite several reports on this subject, continually seems to be incompletely known and underestimated (Guenther et al., 2010a; Arnold et al., 2016; Mo et al., 2018; Wasyl et al., 2018). However, in the assessment of wildlife input to the AMR spread, different animal species with diverse habitats and feeding behaviors need to be considered. It should be emphasized here that migratory species seem to pose a significant threat to AMR dissemination. Seasonal migration, often over long distances and between continents, contributes to greater exposure of animals and their intestinal microbiome to various AMR drivers (Arnold et al., 2016). In this context, the role of free-living birds as AMR carriers is noteworthy and has already drawn the attention of several researchers (Pinto et al., 2010; Literak et al., 2012; Poirel et al., 2012; Veldman et al., 2013; Vredenburg et al., 2014; Oh et al., 2016; Ahlstrom et al., 2018; Zurfluh et al., 2019). To illustrate a ubiquitous and diverse avian community, it covers species that avoid human proximity, such as the golden oriole (Oriolus oriolus), as well as species living close to human settlements, like the house martin (Delichon urbicum). Other examples of the puzzle are herring gulls (Larus argentatus) known to prey on landfills and around sewage treatment plants, areas polluted with a variety of AMR determinants and its affecting factors (Bonnedahl and Järhult, 2014), and white stork (Ciconia ciconia) popular in Polish spring and summer landscape and wintering far away in Africa.
Recent developments in sequencing techniques provide an invaluable tool to elucidate the background and pathways of AMR transmission. Accurate genotypic characterization of bacteria enables to examine the genotypes circulating among environments and to identify their possible links to clinically relevant resistant pathogens and, thus, human and animal infections. Besides a somewhat unrealistic direct contact with free-living birds, there is a serious possibility of livestock or human contact with, for example, bird droppings (Dolejska and Literak, 2019). A flash point for the current study was multidrug-resistant (MDR) Escherichia coli derived from an individual of green woodpecker (Picus viridis) found in nature. The strain was isolated in 2013 from fresh feces collected during the delivery of birds to a veterinary clinic due to clinical symptoms.
In this study, we applied microbiological culture methods commonly used in the official monitoring of slaughter animals (European Food Safety Authority, 2019) to investigate the AMR status of E. coli isolated from free-living birds. The samples were derived from birds coming from natural habitats and rescue centers. The application of whole-genome sequencing (WGS) aimed to characterize AMR determinants and plasmids associated with AMR horizontal transfer and also to investigate the epidemiological relationships and virulence potential of E. coli isolated from free-living birds.
A total of 69 samples (20 intestines, 44 feces, 4 goiter swabs, and 1 stomach sample) from 68 free-living birds were collected between 2017 and 2020 within a convenience sampling in the National Reference Laboratory for Antimicrobial Resistance (NRL) at the National Veterinary Research Institute (NVRI) in Puławy, Poland. The most prevalent tested group constituted birds of prey that belonged to Accipitriformes (n = 23) and Strigiformes (n = 3). The other group consisted of migratory species of Pelecaniformes (n = 19) and ubiquitous Passeriformes (n = 15) representing the most abundant avian order. The dataset was completed with orders represented by Anseriformes (n = 5), Gruiformes (n = 1), Charadriiformes (n = 1), and Columbiformes (n = 1).
Twenty-two samples were derived from deceased birds, mostly birds of prey (n = 9) and waterbirds (n = 6), and sent to the NVRI for diagnostics purposes (e.g., defining the cause of death). The second subset of samples was collected from 15 white storks residing in the Center for Rehabilitation of Free-living Birds in Bukwałd due to mechanical injuries. Antimicrobial treatment status of this group remained unknown. Eight fresh feces samples (six from birds of prey and two from white storks) were collected from animals at the Bird of Prey Rehabilitation Center in Da̧brówka on the day of release. The birds were not treated with antimicrobials during their stay in the rescue center. The remaining samples (n = 24) were taken from animals in their natural environment during the ringing activity or nest inspection carried out in 2019 in Lublin region (S-E Poland) by ornithologists from the Department of Zoology and Nature Protection, UMCS. The study fulfilled the current Polish law and was permitted by the Ministry of the Environment (approval number: DL-III.6713.11.2018.ABR) and the General Directorate for Environmental Protection (approval number: DZP-WG.6401.03.2.2018.jro). The Regional Directorate for Environmental Protection (RDOŚ) in Lublin allowed for the research project through a letter (approval number: WPN. 6401.6.2018.MPR). Ten samples of birds sampled in nature came from juvenile birds of prey—marsh harrier (Circus aeruginosus). All tested bird species, their origin, and included sample types are presented in Supplementary Table 1.
Usually, on the day following their collection, the samples were cultured on buffered peptone water for 18 ± 3 h at 37°C and then streaked on MacConkey agar (Oxoid, Hampshire, United Kingdom), MacConkey agar supplemented with cefotaxime (1 mg/L, Oxoid, Hampshire, United Kingdom), chromIDTM CARBA, chromIDTM OXA-48 agar (bioMérieux, Marcy l’Etoile, France), and MacConkey supplemented with colistin (2 mg/L, Oxoid, Hampshire, United Kingdom) for isolation of commensal, cephalosporin-, carbapenem-, and colistin-resistant E. coli, respectively. Suspected colonies were identified with matrix-assisted laser desorption/ionization time-of-flight mass spectrometry (MALDI-TOF MS, Microflex LT MALDI Biotyper; Bruker Biosciences, Billerica, MA, United States).
All E. coli were tested for antimicrobial susceptibility with the microbroth dilution method (Sensititre; TREK Diagnostic Systems, Thermo Fisher Scientific, Waltham, MA, United States). Resistance tests to nine antimicrobial classes—beta-lactams, quinolones, phenicols, aminoglycosides, folate path inhibitors, tetracyclines, polymyxins, macrolides, and glycylcyclines—were performed with EUVSEC plates (as described in Table 1 of the Annex to 2013/652/EC). For all isolates resistant to cephalosporins, the second panel (EUVSEC2 according to Table 4 of the Annex to 2013/652/EC) was applied. The European Committee on Antimicrobial Susceptibility Testing (EUCAST) epidemiological cutoff values (ECOFFs) for minimum inhibitory concentration (MICs) were used as interpretation criteria. E. coli strain was regarded as resistant (non-wild type, NWT) when MIC values above the cutoff were obtained. Among the NWT category, strains resistant to at least three antimicrobial classes were referred as multidrug-resistant (MDR) (Magiorakos et al., 2012). E. coli with all MIC values below the ECOFF were recognized as susceptible (wild type, WT). The procedure mirrored the official AMR monitoring implemented in the EU according to Directive No. 2013/652/EC.
Seventy-one E. coli strains, including an archival strain from a woodpecker, have been subjected to whole-genome sequencing. Extraction of DNA was prepared with Maxwell® RSC Cultured Cells DNA Kit—Automated DNA Purification from Mammalian and Bacterial Cultured Cells (AS1620 Promega, Madison, Wisconsin, United States) according to the manufacturer’s instruction with Maxwell® RSC Instrument (Promega, Madison, Wisconsin, United States). For yield and purity check, all samples were measured with NanoDropTM One following extraction (Thermo Scientific, Waltham, MA, United States). DNA libraries prepared with Library Preparation Kit (Illumina, Inc., San Diego, CA, United States) according to the manufacturer’s instructions were sequenced with the MiSeq platform (Illumina, Inc., San Diego, CA, United States). Paired-end sequencing per flow cell (2 × 300) was applied.
FastQC 0.11.5 was used for the raw reads quality check and Trimmomatic 0.36 (Bolger et al., 2014) for the read trimming. Corrected reads were de novo assembled by SPAdes 3.9.0 (Bankevich et al., 2012). Resistance and plasmid identification was conducted using abricate 1.0.1 (Seemann, 2019) against ResFinder (Zankari et al., 2012) and PlasmidFinder (Carattoli et al., 2014) databases (2020-07-25) with identity threshold 95% and selected minimum length 60%. PointFinder software 3.1.0 was applied for the identification of chromosomal point mutations (database: 2019-07-02) (Zankari et al., 2012). For the identification of multilocus sequence type (MLST, ST), we used the MLST 2.0 tool (Larsen et al., 2012) with database version 2.0.0 (2020-05-04). Virulence factors were analyzed with VirulenceFinder 2.0 and its database of 2020-05-29 with% ID threshold 90% and minimum length 60% (Joensen et al., 2014). Submission of E. coli with unknown ST to EnteroBase v1.1.21 allowed to assign new sequence type using the Achtman 7 Gene MLST algorithm (Zhou et al., 2020).
CSI Phylogeny 1.4 (call SNPs and infer phylogeny) CGE with input parameters—minimum depth at single nucleotide polymorphism (SNP) positions: 10, relative depth at SNP positions: 10, minimum distance between SNPs (prune): 10, minimum SNP quality: 30, minimum read mapping quality: 25, minimum Z-score: 1.96—was applied for phylogeny tree preparation (Kaas et al., 2014). As reference genome of E. coli from one of the two most represented ST types was chosen (14P KOL). The online tool iTOL v5 was applied for phylogeny tree visualization (Letunic and Bork, 2019).
The sequences were deposited at the European Nucleotide Archive (ENA)2 under accession number PREJB426693.
The variability of the noted MLST and virulence genes was measured with Simpson’s diversity index (Hunter and Gaston, 1988).
To determine the statistical difference in the occurrence of resistant E. coli between groups of birds, a chi-square test with the appropriate correction was applied (Supplementary Table 2). Resistance in different groups of birds was assessed with a 95% confidence interval.
A total of 73 E. coli were isolated. Sixty were obtained from MacConkey agar, six from MacConkey supplemented with cefotaxime, and seven from MacConkey with colistin. No carbapenem-resistant E. coli were found. To avoid duplicate testing of the same strain, three isolates were excluded, as they were obtained on different culture media from the same sample but showed identical minimum inhibitory concentration (MIC) values. As a result, 70 strains were included in the comparison of resistance in different bird groups. Half of the tested E. coli were found resistant (n = 35, 50.0%) and most of them (n = 27, 38.6%) were MDR (resistant to at least three antimicrobial classes). The analyses showed significant differences between the number of resistant strains isolated from birds sampled in nature and all the other groups (p-values 0.0047–0.0003) (Supplementary Table 2). Susceptible E. coli were derived mostly from birds sampled in nature. Yet, two strains derived from blue tit (Cyanistes caeruleus) sampled in nature exhibited non-wild-type MICs with 8 and 11 antimicrobials each.
Of all the antimicrobials assessed, ampicillin and tetracycline resistance dominated (41.4%, each) followed by quinolones (35.7 and 31.4% for ciprofloxacin and nalidixic acid, respectively), as well as folate path inhibitors (28.6% sulfamethoxazole and almost 22.9% trimethoprim). A lower percentage of AMR was observed for chloramphenicol (12.9%) and third-generation cephalosporins (8.6%). Four strains (5.7%) were resistant to gentamicin. Supplementary Table 3 presents detailed MIC value distribution of the tested E. coli on both applied panels. Overall, 20 different AMR profiles were noted (Figure 1).
Figure 1. Phylogeny of Escherichia coli strains isolated from wild birds. Full and empty squares depict the presence or absence of AMR or AMR determinants. AMR profile in black—phenotypic AMR profiles confirmed by the presence of AMR determinants. Red square with white circle means phenotypical resistance not confirmed by genotypic testing. (A) Beta-lactam, (B) quinolone, (C) aminoglycosides, (D) folate path inhibitors, (E) tetracyclines, (F) phenicols, (G) colistin, (H) fosfomycin, (I) macrolide and lincosamide resistance determinants. Visualization prepared with the online tool iTOL v5.
Cephalosporin resistance was defined as extended-spectrum beta-lactamases (ESBLs) and AmpC-type cephalosporinase production. ESBL determinants were identified as blaSHV–12 (archival woodpecker E. coli) and blaCTX–M–15 [n = 1, mute swan (Cygnus olor)—deceased]. AmpC-type cephalosporinases were determined by blaCMY–2 [n = 5, blue tit—nature, white-tailed eagle (Haliaeetus albicilla), buzzard (Buteo buteo), crane (Grus grus)—deceased, white stork—rescue]. Resistance to ampicillin was linked to blaTEM–1B most often. In one ampicillin-resistant E. coli from buzzard (released), any relevant determinant was noted that could confer resistance for beta-lactams.
Mutations in quinolone resistance determining region (QRDR) dominated ciprofloxacin and nalidixic acid resistance, and gyrA substitution S83L was noted in the majority of 26 resistant strains (n = 23). Three quinolone-susceptible strains carried single parC mutations [n = 1, marsh harrier (Circus aeruginosus)—nature; n = 2, white-tailed eagle —deceased] and one wild-type strain possessed parE mutation [marsh harrier—nature].
Plasmid-mediated quinolone resistance (PMQR) genes were identified as qnrS1 [n = 4; two white storks—rescue, raven (Corvus corax), and buzzard—deceased] and qnrB19 (n = 1; buzzard—deceased). The gene aac(6′)-Ib-cr that determines AMR toward quinolones and aminoglycosides was noted in a strain from deceased mute swan carrying blaCTX–M–15 and blaOXA–1.
The genes aac(3)-IIa (n = 2, mute swan—deceased) and aac(3)-IId (n = 2, blue tit—nature) were associated with gentamicin resistance. Of all determinants noted, genes encoding resistance toward aminoglycosides other than gentamicin (e.g., streptomycin not tested phenotypically) were the most abundant (n = 55), and among them, ant(3″)-Ia (n = 18), aph(3″)-Ib (n = 13), and aph(6)-Id (n = 13) prevailed. Genes sul1, sul2, sul3, and dfrA1 were the most often noted among strains resistant to folate path inhibitors, while genes tet(A) (n = 21) and tet(B) (n = 11) dominated in tetracycline-resistant E. coli. Resistance toward phenicols was determined by cmlA1, catA1, catB3, and floR. Genes cmlA1 and catB3 were also found in five strains that were chloramphenicol susceptible.
The mcr-1 gene conferring colistin resistance was noted in two strains with MIC for this antimicrobial below the cutoff (MIC = 2 mg/L). Both E. coli were found in deceased water birds (herring gull and mute swan). WGS also revealed the presence of fosA7 determining resistance toward fosfomycin (not tested phenotypically) in two E. coli (white stork—rescue and white-tailed eagle—released). Three genes that confer resistance for macrolides [mef(B), mph(A)] and lincosamides [lnu(G)] were noted as well (Figure 1).
All genotype–phenotype discrepancies in case of mcr-1 finding in colistin wild-type strain as well as E. coli susceptible to chloramphenicol that carried cmlA1 and catB3 were confirmed by repeated susceptibility testing. Resistance to ampicillin in E. coli without any relevant AMR gene was also verified.
Multiple plasmid replicons (n = 30) were found dispersed in most of the tested E. coli (n = 60). The most frequent replicon was IncFIB(AP001918) (n = 47). Few other replicons IncI1_1_Alpha, p0111, IncFIC(FII), IncQ1, and IncFII occurred in a similar number of strains (from 8 up to 12 strains) (Supplementary Figure 1). Ten from 11 plasmid replicon-free strains were pan-susceptible and AMR gene-free (Figure 1). The exception was one strain from deceased mute swan carrying five resistance genes including genes blaCTX–M–15, blaOXA–1, and aac(6’)-Ib-cr. Chromosomal location of antimicrobial resistance genes in that strain was confirmed with MinION long-read sequencing (Oxford Nanopore Technologies, data not presented).
Seventeen strains possessed one plasmid replicon and three of them carried resistance genes. Among 34 E. coli with two up to four identified plasmid replicons, 25 strains were recognized as non-wild type (NWT), including 17 MDR. Nine E. coli carried from five up to eight plasmid replicons and all were MDR (Figure 1 and Supplementary Figure 1).
Virulence factors were noted in all tested strains. One E. coli possessed a single virulence gene; the remaining carried at least three virulence genes. The majority of E. coli (n = 47) contained 10 or more virulence factors. In nine strains, 20 up to 26 virulence genes were found simultaneously. A huge diversity (D = 0.959) of virulence factors amounting to 65 different determinants was noted. The most prevalent genes were terC (n = 71), gad (n = 63), ompT (n = 51), iss (n = 49), traT (n = 48), lpfA (n = 46), and sitA (n = 42) (Figure 2). One of the E. coli isolated from deceased collared dove (Streptopelia decaocto) was recognized as Shiga toxin-producing E. coli (STEC). It carried stx2A and stx2B encoding Shiga toxin and nleA–C determining non-LEE encoded effectors.
Figure 2. Virulence of E. coli isolated from wild birds. Virulence factor by sequence type and bird’s species. Full and empty squares mean the presence or absence of virulence factors. The matrix was visualized with iTOL v5.
Multilocus sequence typing (MLST) revealed 52 STs among 71 tested E. coli (Simpson diversity index D = 0.989). The most abundant were ST10 and ST9699 (four strains each), followed by ST602 and ST539 (three E. coli each). Nine ST types (including avian pathogenic ST117) were represented by two strains. One strain belonged to pandemic E. coli lineage ST131. The strain was isolated from deceased mute swan, and it was MDR and harbored the CTX-M-15 enzyme. All strains derived from the same sample but different culture media belonged to different STs. The minimum SNP dissimilarities were observed between strains belonging to ST388 (four SNPs). The highest difference of 46,533 SNPs was noted between strain 55P (ST3100) and 54P (ST130). Three novel STs were noted and assigned by EnteroBase as ST11104, ST11105, and ST11194. Those three STs were characterized by new gyr alleles (1,037, 1,038, and 1,047, respectively). All E. coli belonging to ST9699 were cephalosporin-resistant and carried the blaCMY–2 gene. One ST10 strain from deceased herring gull was MDR and possessed inter alia mcr-1.1 and blaTEM–1B. The remaining three ST10 strains were susceptible (Figure 1).
The impact of resistant bacteria on health and environmental issues is unquestionable. Thus, the identification of potential hazards associated with wildlife becomes critical to recognize environmental reservoirs of AMR and to take appropriate and prompt actions against possible emerging AMR mechanisms (Davies and Davies, 2010). Our study, although based on a convenience sampling of a limited fraction of free-living bird populations, confirms its role in AMR transmission and reveals the zoonotic potential of E. coli coming from the avian reservoir.
The main concerns arising from our study relate to resistance toward antimicrobial classes of the highest priority in human medicine, e.g., cephalosporins, quinolones, polymyxins, and aminoglycosides (World Health Organization [WHO], 2019; European Medicines Agency, 2020), as well as fosfomycin, assigned to substances that should be limited for human prescription in the EU (European Medicines Agency, 2020).
The AMR profiles noted in E. coli of avian origin were dominated by resistance toward classes of antimicrobials often used in human and veterinary medicine (Food and Agriculture Organization of the United Nations, 2016; World Health Organization [WHO], 2018; European Medicines Agency and European Surveillance of Veterinary Antimicrobial Consumption, 2019). This finding indicates that ubiquitous birds might be exposed to the anthropogenic impact and acquisition of resistant bacteria through contact with the waste of human or livestock origin. Resistance toward antimicrobial classes that we found prevailing (beta-lactams, tetracyclines, quinolones, aminoglycosides, folate path inhibitors) was also reported in bird communities from distinct geographical areas worldwide, from the Americas through Africa, Europe, Asia to Australia (Nascimento et al., 2003; Guenther et al., 2010b; Literak et al., 2010; Poirel et al., 2012; Veldman et al., 2013; Mohsin et al., 2016; Guenther et al., 2017; Ahlstrom et al., 2018; Marcelino et al., 2019; Zurfluh et al., 2019; Cao et al., 2020; Fuentes-Castillo et al., 2020; Nabil et al., 2020). Moreover, AMR toward those substances was commonly found in E. coli from farm animals and reported from other wildlife species (Navarro-Gonzalez et al., 2013; Wasyl et al., 2013, 2018; Ceccarelli et al., 2020). The AMR status of bacteria isolated from birds living in pristine environments of Antarctica—gentoo penguin (Pygoscelis papua)—seems to be contrasting. Some studies showed an almost complete lack of resistance in bacteria from penguins, while other studies revealed resistance but simultaneously indicated that the result might have been influenced by human activity (Bonnedahl et al., 2008; Miller et al., 2009; Rabbia et al., 2016; Marcelino et al., 2019).
The direct anthropogenic impact could have caused resistance noted in E. coli from birds originating from rescue centers. Although the animals were not treated with antimicrobials, they were fed by humans and kept in human proximity. The inclusion of that group of birds might be considered as a weakness of the study. Nevertheless, we believe that the results may highlight the immediate risk for people dealing with birds. Furthermore, it reveals the potential “microbial pollution” of the environment after the release of birds into their natural habitats.
Exposure to environmental pollution and anthropogenic factors, e.g., human waste, sewage treatment plant effluents, and manure, seems to be the significant cause inducing resistance in migratory species and waterbirds, e.g., white stork, crane, mute swan, and mallard (Anas platyrhynchos), that we found. That scenario has also been postulated by other researchers (Cole et al., 2005; Guenther et al., 2012; Bouaziz et al., 2018; Marcelino et al., 2019).
We assume that limited contact with human-related external factors and the environment explains the lowest number of NWT strains observed among birds sampled in nature as a significant part of that avian category constituted predominantly by young birds. Similar conclusions were derived by researchers from Switzerland that also suggested a higher resistance E. coli rate in adult birds and the parental transmission of AMR as the most probable in the case of juveniles (Zurfluh et al., 2019).
Deliberating the possible source of AMR in avian species, the position of birds within the trophic interactions should also be taken into consideration. It cannot be excluded that the multidrug resistance found in our study among E. coli from raptorial birds might be the aftermath of transmission and accumulation of resistance determinants from their potential prey (Marrow et al., 2009).
It should be emphasized that no resistance determinants toward carbapenems were noted among the tested strains, and so far, carbapenem resistance has rarely been reported in Poland in farm animals and other wildlife species (Lalak et al., 2016; Wasyl et al., 2018; Skarżyńska et al., 2020a,b). However, since the first report of carbapenemase-producing Salmonella Corvallis from the migratory bird—black kite (Milvus migrans) in Germany (Fischer et al., 2013), several new studies that presented carbapenem resistance in bacteria from free-living birds were published (Vittecoq et al., 2017; Bouaziz et al., 2018). One of them described a high prevalence of blaIMP-producing enterobacteria in the silver gull (Chroicocephalus novaehollandiae) on Five Islands of Australia (Dolejska et al., 2016). The authors suggested that colonization of birds with resistant bacteria was a result of the feeding habits of the birds at a local waste depot contaminated with clinical material.
Numerous studies reported the occurrence of ESBLs in free-living birds (Guenther et al., 2012, 2017; Mohsin et al., 2017; Zurfluh et al., 2019). It is worth emphasizing that we found CTX-M-15, ESBL of public health concern, in E. coli from a deceased mute swan. Although ESBL genes are frequently described on IncF and IncI plasmids (Rozwandowicz et al., 2018) in case of ESBL-producing strains in our study, the resistance genes were not located on the same contigs as plasmid replicons. The gene blaCTX–M–15, as well as the other AMR genes in this strain, was located in the chromosome. It has been suggested that the incorporation of AMR genes into the chromosome favors the maintenance of resistance in the bacterial population of E. coli (Rodríguez et al., 2014). The occurrence of chromosomally encoded blaCTX–M genes was previously reported in studies on avian E. coli from Pakistan and Mongolia (Guenther et al., 2017; Mohsin et al., 2017). Moreover, chromosomal integration of blaCTX–M–15 was described in clinical E. coli isolates belonging to the clonal group ST131, and our strain represented this lineage (Rodríguez et al., 2014).
The ESBL encoded by blaSHV–12 in archival E. coli from woodpecker was one of the most prevalent ESBLs associated with nosocomial infections before the increase of CTX-M enzymes (Coque et al., 2008). The SHV-12 was previously reported in E. coli from waterbirds in Poland (Literak et al., 2010), but it was found in free-living birds in Spain and The Netherlands as well (Veldman et al., 2013; Oteo et al., 2018). Moreover, spreading of blaSHV–12 was formerly revealed among indicator E. coli isolated from food animals in Poland (Lalak et al., 2016).
It should be emphasized that in our study cephalosporin resistance was mostly linked to AmpC-type cephalosporinase encoded by blaCMY–2. The finding of the gene in E. coli from blue tit, often found in close vicinity to human settlements, is a cause for concern. During winter, tits are often fed with pork fat and this raises a question on the direction of the gene transmission. It was formerly revealed that in Poland, blaCMY–2 disseminated among E. coli from food-producing animals, e.g., pigs, broilers, and turkeys, as well as from wildlife, e.g., wild boars (Lalak et al., 2016; Wasyl et al., 2018). Among free-living birds, the gene was reported in E. coli from species associated with aquatic environments and birds of prey (Poirel et al., 2012; Veldman et al., 2013; Ahlstrom et al., 2018).
AMR mechanisms toward quinolones in tested E. coli were dominated with mutations in quinolone resistance determining region (QRDR), and this result might indicate the presence of quinolone selection pressure in the environment. Naturally, drug residues in the environment are subjected to photo- and biodegradation, but the latter process seems to have lower rates for quinolones as synthetic compounds (Martinez, 2009). It was formerly revealed that even at very low concentrations quinolones might select for resistance (Gullberg et al., 2011; Andersson and Hughes, 2012). Our results show that QRDR mutations seem to spread clonally in certain lineages of E. coli. QRDR mutations were reported previously in E. coli isolated from gulls and birds of prey. Furthermore, it was demonstrated that the same E. coli lineages were present in wastewaters, streams, and gulls (Vredenburg et al., 2014). Former research on E. coli derived from free-living birds with septicemia also indicated the role of QRDR mutations but reported only mutation in gyrase A subunit (gyrA) leading to the Ser-83Leu amino acid substitution (Jimenez Gomez et al., 2004). Single mutations in parC and parE noted here did not affect the quinolone susceptibility of the strains (Heisig, 1996; Vila et al., 1996; Komp Lindgren et al., 2003; Ling et al., 2003).
In our study, only a few qnr genes were noted conversely to a previous report on E. coli from free-living birds sampled on the Polish Baltic sea coast, which revealed qnrS gene presence in quinolone-resistant strains accompanied by gyr and par mutations in some of them (Literak et al., 2010). It should be underlined that the majority of former studies on quinolone resistance in free-living birds from the United States, Europe, and Asia were focused mostly on plasmid-mediated resistance mechanisms and reported the occurrence of qnrS, qnrB, and aac(6′)-Ib-cr (Literak et al., 2010, 2012; Halova et al., 2014; Oh et al., 2016; Mohsin et al., 2017). Interestingly, our study confirmed the presence of aac(6′)-Ib-cr that determines resistance toward quinolones and aminoglycosides along with beta-lactamases encoded by blaCTX–M–15 and blaOXA–1 in E. coli from a deceased mute swan, supporting the theory on the spread of these resistance gene sets among bird communities (Literak et al., 2010; Veldman et al., 2013; Vredenburg et al., 2014).
The cause for concern was finding plasmid-mediated colistin resistance (mcr-1) in deceased mute swan and herring gull. It is worth noting that the first cases of the mcr-1 occurrence in free-living birds were published in 2016 (Mohsin et al., 2016; Ruzauskas and Vaskeviciute, 2016). Similar to our results, these studies reported mcr-1 in species associated with aquatic environments: herring gull and coot (Fulica atra). A recent study from Egypt revealed over 10% prevalence of mcr-1 in bacteria from resident birds (e.g., pigeons, crows) and even 20% prevalence of the gene in migratory waterfowls birds (Ahmed et al., 2019). Moreover, the gene was detected in water samples collected in the area of bird trapping. All the above results indicate that birds might be considered an important vector of colistin resistance. Previous studies from Poland showed the wide spread of mcr-1 among food-producing animals, particularly turkeys (Zaja̧c et al., 2019). Corresponding to our study, the gene was revealed inter alia on IncX4 plasmid and occurred mostly in isolates with colistin MIC close to ECOFF (2 mg/L). The mentioned Egyptian study also reported mcr-2 presence, although less frequently (Ahmed et al., 2019). The gene was found neither in this research nor in previous studies from Poland (Literak et al., 2010; Zaja̧c et al., 2019).
The finding of fosA7 gene encoding fosfomycin resistance in E. coli derived from birds remaining at rescue centers drew our attention. White-tailed eagle colonized with E. coli carrying fosA7 was released into the natural habitat becoming a source of the resistance in the environment. This perfectly illustrates the feasibility of “microbial pollution” by AMR determinants and resistant bacteria. Recently, the fosA7-like gene and other fosfomycin resistance gene, namely fosA3, were found in Andean condors (Vultur gryphus) (Fuentes-Castillo et al., 2020). Earlier, fosA7 was described in Salmonella isolated from broiler chickens in Canada, as well as from retail meat and clinical incidents in the United States (Rehman et al., 2017; Keefer et al., 2019). The gene was also noted in E. coli recovered from soil exposed to anthropogenic activities in North Carolina (Balbin et al., 2020). In Europe, the presence of fosfomycin resistance gene fosA3 in a Salmonella isolated from the migratory bird black kite was reported in Germany (Villa et al., 2015).
Although we noted an infrequent (5.7%) occurrence of resistance to aminoglycosides represented exclusively by gentamicin, we found a spectrum of genes determining resistance toward other compounds of this group, e.g., streptomycin. This result proved that application of phenotypical methods often limited to several antimicrobials, or identification of selected resistance determinants, may lead to underestimation of real AMR status. Similar conclusions were also presented in another study (Rega et al., 2021). That was perfectly illustrated by the E. coli recognized as wild type, isolated from a marsh harrier sampled in nature. The analysis revealed that the strain carried aminoglycoside phosphotransferases encoded by aph(3″)-Ib and aph(6)-Id. On the other hand, identification of the gene (i.e., mcr-1 discussed earlier) does not always mean resistance. Those findings drive attention to the everlasting discussion of phenotype–genotype congruence and the superiority of the methods applied for testing both aspects.
A variety of sequence types was revealed among the tested E. coli including some relevant lineages. Furthermore, a wide range and number of virulence factors were observed.
A single isolate from deceased herring gull belonged to ST10, one of the clinically important clones, and carried mcr-1. Such an E. coli ST10 with mcr-1 was reported in the Sultanate of Oman from human bloodstream infection (Mohsin et al., 2018). A similar strain was noted in a clinical case in Uruguay (Papa-Ezdra et al., 2020). That E. coli variant was also observed in poultry from Poland and China (Yang et al., 2017; Zaja̧c et al., 2019) and in agricultural soil of Algeria (Touati et al., 2020).
Another highly virulent lineage—ST131 often associated with extended-spectrum β-lactamase CTX-M-15 spread, was reported as predominant among extraintestinal pathogenic E. coli (ExPEC) (Coque et al., 2008; Nicolas-Chanoine et al., 2014). In Poland, the ST131 clone was a frequent cause of neonatal infections (Chmielarczyk et al., 2013). Indeed, our ST131 from deceased mute swan strain possessed several virulence determinants specific for ExPEC pathotype such as enabling colonization (pap) and adherence (iha), as well as determining the outer membrane hemin receptor (chuA) and secreted autotransporter toxin (sat) (Sarowska et al., 2019).
The identification of STEC belonging to ST20 in deceased collared dove captured our attention. Although pigeons were pointed out as a STEC reservoir, most of the researches indicated the presence of Stx2f toxin in the tested strains. A recent study concerning Stx2f-carrying E. coli demonstrated that strains responsible for human infections do not directly originate from the pigeon reservoir (van Hoek et al., 2019). However, the E. coli tested here possessed two subtypes of toxin Stx2, including Stx2A, which has previously been described as more potent in causing clinical outcomes (Fuller et al., 2011). Moreover, the study on STEC from Switzerland reported ST20 clone carrying stx2A from human patients (Fierz et al., 2017).
We also noted E. coli ST117 strains from deceased crane and raven harboring papC (outer membrane usher P fimbriae), accompanied with fyuA (yersiniabactin receptor), iucC (aerobactin synthetase), iroN (enterobactin siderophore receptor protein), vat (vacuolating autotransporter toxin), and iss (increased serum survival). It might be perceived as a poultry health risk since the genes were previously reported in avian pathogenic E. coli (APEC) ST117 resulting in increased mortality and colibacillosis in broilers in Nordic countries (Ronco et al., 2017).
As a flash point for the study, the archival MDR E. coli woodpecker isolate was assigned to the global clone ST224. Noteworthy, our strain possessed high pathogenicity potential carrying multiple virulence genes. MDR E. coli belonging to ST224 lineage were previously isolated from patients with urinary tract infections in China (Cao et al., 2014). MDR strains assigned to ST224 were identified among E. coli from retail food (chicken carcasses and ground beef) in Egypt (Ramadan et al., 2020). ST224 E. coli were also noted among ESBL-producing strains from food-producing animals and wastewater samples in Tunisia (Sghaier et al., 2019). Moreover, E. coli ST224 was reported to cause a fatal pneumonia infection in a domestic cat (Felis catus) (Silva et al., 2018).
All of the above findings indicate free-living bird populations represented by our study group might be considered a source or vector of E. coli posing a possible threat to public and animal health. Identification of resistance toward several antimicrobial classes including substances of the highest priority for human medicine, e.g., cephalosporins and quinolones in all tested groups of birds, verified that free-living birds constitute a meaningful AMR reservoir and vector.
Nothing in nature is lost. All pollution of the environment, farmlands, and water might become a possible source of AMR determinants for animals. In consequence, animals affected by resistant bacteria turn into a vector of AMR transmission. Our study shows that the clonal spread of E. coli lineages of public and animal health relevance is a serious avian-associated hazard.
The original contributions presented in the study are publicly available. This data can be found here: the European Nucleotide Archive (ENA), accession number: PRJEB42669, (http://www.ebi.ac.uk/ena/data/view/PRJEB42669).
The animal study was reviewed and approved by the Ministry of the Environment (approval number: DL-III.6713.11.2018.ABR) General Directorate for Environmental Protection (approval number: DZP-WG.6401.03.2.2018.jro) Regional Directorate for Environmental Protection (RDOŚ) in Lublin (approval number: WPN. 6401.6.2018.MPR).
MS and DW designed the experiments. MP, JW, and WK performed the sampling campaign. MS and MZ performed the experiments. MS and AB performed the NGS analyses. ŁB performed the statistical analyses. MS prepared the manuscript. All authors discussed the results, reviewed and edited the manuscript, and read and approved the final version of the manuscript.
The whole-genome sequencing was possible due to the support of the Department of Omics Analyses from the Ministry of Science and Higher Education (Grant No. 4477/E-180/SPUB/2018).
The authors declare that the research was conducted in the absence of any commercial or financial relationships that could be construed as a potential conflict of interest.
We are grateful to Danuta Wnuk, Paulina Pasim, Ewelina Skrzypiec, Emilia Mikos, Dominika Wojdat, Weronika Koza, Aleksandra Giza, and Ewelina Kamińska for their excellent technical assistance. We thank Ewa Rumińska from the Center for Rehabilitation of Free-living Birds in Bukwałd near Olsztyn and Paweł Bednarczyk from the Bird of Prey Rehabilitation Center in Da̧brówka for providing sample birds remaining at rescue centers.
The Supplementary Material for this article can be found online at: https://www.frontiersin.org/articles/10.3389/fmicb.2021.656223/full#supplementary-material
Ahlstrom, C. A., Bonnedahl, J., Woksepp, H., Hernandez, J., Olsen, B., and Ramey, A. M. (2018). Acquisition and dissemination of cephalosporin-resistant E. coli in migratory birds sampled at an Alaska landfill as inferred through genomic analysis. Sci. Rep. 8:7361. doi: 10.1038/s41598-018-25474-w
Ahmed, Z. S., Elshafiee, E. A., Khalefa, H. S., Kadry, M., and Hamza, D. A. (2019). Evidence of colistin resistance genes (mcr-1 and mcr-2) in wild birds and its public health implication in Egypt. Antimicrob. Resist. Infect. Control 8:197. doi: 10.1186/s13756-019-0657-5
Andersson, D. I., and Hughes, D. (2012). Evolution of antibiotic resistance at non-lethal drug concentrations. Drug Resist. Updat. 15, 162–172. doi: 10.1016/j.drup.2012.03.005
Arnold, K. E., Williams, N. J., and Bennett, M. (2016). ‘Disperse abroad in the land’: the role of wildlife in the dissemination of antimicrobial resistance. Biol. Lett. 12:20160137. doi: 10.1098/rsbl.2016.0137
Balbin, M. M., Hull, D., Guest, C., Nichols, L., Dunn, R., and Thakur, S. (2020). Antimicrobial resistance and virulence factors profile of Salmonella spp. and Escherichia coli isolated from different environments exposed to anthropogenic activities. J. Glob. Antimicrob. Resist. 22, 578–583. doi: 10.1016/j.jgar.2020.05.016
Bankevich, A., Nurk, S., Antipov, D., Gurevich, A. A., Dvorkin, M., Kulikov, A. S., et al. (2012). SPAdes: a new genome assembly algorithm and its applications to single-cell sequencing. J. Comput. Biol. 19, 455–477. doi: 10.1089/cmb.2012.0021
Bolger, A. M., Lohse, M., and Usadel, B. (2014). Trimmomatic: a flexible trimmer for Illumina sequence data. Bioinformatics 30, 2114–2120. doi: 10.1093/bioinformatics/btu170
Bonnedahl, J., and Järhult, J. D. (2014). Antibiotic resistance in wild birds. Ups. J. Med. Sci. 119, 113–116. doi: 10.3109/03009734.2014.905663
Bonnedahl, J., Olsen, B., Waldenström, J., Broman, T., Jalava, J., Huovinen, P., et al. (2008). Antibiotic susceptibility of faecal bacteria in Antarctic penguins. Polar Biol. 31, 759–763. doi: 10.1007/s00300-008-0430-3
Borkow, G., and Gabbay, J. (2005). Copper as a biocidal tool. Curr. Med. Chem. 12, 2163–2175. doi: 10.2174/0929867054637617
Bouaziz, A., Loucif, L., Ayachi, A., Guehaz, K., Bendjama, E., and Rolain, J. M. (2018). Migratory white stork (Ciconia ciconia): a potential vector of the OXA-48-producing Escherichia coli ST38 clone in Algeria. Microb. Drug Resist. 24, 461–468. doi: 10.1089/mdr.2017.0174
Cao, J., Hu, Y., Liu, F., Wang, Y., Bi, Y., Lv, N., et al. (2020). Metagenomic analysis reveals the microbiome and resistome in migratory birds. Microbiome 8:26. doi: 10.1186/s40168-019-0781-8
Cao, X., Zhang, Z., Shen, H., Ning, M., Chen, J., Wei, H., et al. (2014). Genotypic characteristics of multidrug-resistant Escherichia coli isolates associated with urinary tract infections. APMIS 122, 1088–1095.
Carattoli, A., Zankari, E., Garcia-Fernandez, A., Voldby Larsen, M., Lund, O., Villa, L., et al. (2014). In silico detection and typing of plasmids using PlasmidFinder and plasmid multilocus sequence typing. Antimicrob. Agents Chemother. 58, 3895–3903. doi: 10.1128/AAC.02412-14
Ceccarelli, D., Hesp, A., van der Goot, J., Joosten, P., Sarrazin, S., Wagenaar, J. A., et al. (2020). Antimicrobial resistance prevalence in commensal Escherichia coli from broilers, fattening turkeys, fattening pigs and veal calves in European countries and association with antimicrobial usage at country level. J. Med. Microbiol. 69, 537–547. doi: 10.1099/jmm.0.001176
Chmielarczyk, A., Pobiega, M., Wojkowska-Mach, J., Romaniszyn, D., Adamski, P., Heczko, P. B., et al. (2013). Molecular epidemiology, plasmid analysis, virulence, and resistance of Escherichia coli isolated from neonatal intensive care units in Poland. Diagn. Microbiol. Infect. Dis. 76, 542–545. doi: 10.1016/j.diagmicrobio.2013.04.016
Cole, D., Drum, D. J., Stalknecht, D. E., White, D. G., Lee, M. D., Ayers, S., et al. (2005). Free-living Canada geese and antimicrobial resistance. Emerg. Infect. Dis. 11, 935–938. doi: 10.3201/eid1106.040717
Coque, T. M., Baquero, F., and Canton, R. (2008). Increasing prevalence of ESBL-producing Enterobacteriaceae in Europe. Euro Surveill. 13:19044.
Davies, J., and Davies, D. (2010). Origins and evolution of antibiotic resistance. Microbiol. Mol. Biol. Rev. 74, 417–433. doi: 10.1128/MMBR.00016-10
D’Costa, V. M., King, C. E., Kalan, L., Morar, M., Sung, W. W. L., Schwarz, C., et al. (2011). Antibiotic resistance is ancient. Nature 477, 457–461. doi: 10.1038/nature10388
Dolejska, M., and Literak, I. (2019). Wildlife Is Overlooked in the Epidemiology of Medically Important Antibiotic-Resistant Bacteria. Antimicrob. Agents Chemother. 63, e1167–19. doi: 10.1128/AAC.01167-19
Dolejska, M., Masarikova, M., Dobiasova, H., Jamborova, I., Karpiskova, R., Havlicek, M., et al. (2016). High prevalence of Salmonella and IMP-4-producing Enterobacteriaceae in the silver gull on Five Islands, Australia. J. Antimicrob. Chemother. 71, 63–70. doi: 10.1093/jac/dkv306
European Centre for Disease Prevention and Control (2009). The Bacterial Challenge: Time to React. ECDC/EMEA Joint Technical Report 2009. (Stockholm, September 2009). Solna Municipality: European Centre for Disease Prevention and Control, 1–54. doi: 10.2900/2518.
European Commission (2009). Directorate General for Health and Consumer Protection, and Scientific Committee on Emerging and Newly Identified Health Risks 2009. Assessment of the Antibiotic Resistance Effects of Biocides. Available online at: https://ec.europa.eu/health/ph_risk/committees/04_scenihr/docs/scenihr_o_021.pdf (accessed January 5, 2021).
European Food Safety Authority (2019). The European Union Summary Report on antimicrobial Resistance in Zoonotic and Indicator Bacteria from Humans, Animals and Food in 2017. 1-278. Parma: European Food Safety Authority. doi: 10.2903/j.efsa.2019.5598 (accessed May 1, 2021).
European Medicines Agency (2020). Categorisation of Antibiotics in the European Union. EMA/CVMP/CHMP/682198/2017. Amsterdam: European Medicines Agency, 1–73.
European Medicines Agency and European Surveillance of Veterinary Antimicrobial Consumption (2019). Sales of Veterinary Antimicrobial Agents in 31 European Countries in 2017. EMA/294674/2019. Amsterdam: European Medicines Agency, 1–109.
Fierz, L., Cernela, N., Hauser, E., Nüesch-Inderbinen, M., and Stephan, R. (2017). Characteristics of shigatoxin-producing Escherichia coli strains isolated during 2010-2014 from human infections in Switzerland. Front. Microbiol. 8:1471. doi: 10.3389/fmicb.2017.01471
Fischer, J., Schmoger, S., Jahn, S., Helmuth, R., and Guerra, B. (2013). NDM-1 carbapenemase-producing Salmonella enterica subsp. enterica serovar Corvallis isolated from a wild bird in Germany. J. Antimicrob. Chemother. 68, 2954–2956. doi: 10.1093/jac/dkt260
Food and Agriculture Organization of the United Nations (2016). Drivers, Dynamics and Epidemiology of Antimicrobial Resistance in Animal Production. ISBN: 978-92-5-109441-9. Rome: Food and Agriculture Organization of the United Nations, 1–68.
Fuentes-Castillo, D., Esposito, F., Cardoso, B., Dalazen, G., Moura, Q., Fuga, B., et al. (2020). Genomic data reveal international lineages of critical priority Escherichia coli harbouring wide resistome in Andean condors (Vultur gryphus Linnaeus, 1758). Mol. Ecol. 29, 1919–1935. doi: 10.1111/mec.15455
Fuller, C. A., Pellino, C. A., Flagler, M. J., Strasser, J. E., and Weiss, A. A. (2011). Shiga toxin subtypes display dramatic differences in potency. Infect. Immun. 79, 1329–1337. doi: 10.1128/IAI.01182-10
Guenther, S., Aschenbrenner, K., Stamm, I., Bethe, A., Semmler, T., Stubbe, A., et al. (2012). Comparable high rates of extended-spectrum-beta-lactamase-producing Escherichia coli in birds of prey from Germany and Mongolia. PLoS One 7:e53039. doi: 10.1371/journal.pone.0053039
Guenther, S., Grobbel, M., Heidemanns, K., Schlegel, M., Ulrich, R. G., Ewers, C., et al. (2010a). First insights into antimicrobial resistance among faecal Escherichia coli isolates from small wild mammals in rural areas. Sci. Total Environ. 408, 3519–3522. doi: 10.1016/j.scitotenv.2010.05.005
Guenther, S., Grobbel, M., Lubke-Becker, A., Goedecke, A., Friedrich, N. D., Wieler, L. H., et al. (2010b). Antimicrobial resistance profiles of Escherichia coli from common European wild bird species. Vet. Microbiol. 144, 219–225. doi: 10.1016/j.vetmic.2009.12.016
Guenther, S., Semmler, T., Stubbe, A., Stubbe, M., Wieler, L. H., and Schaufler, K. (2017). Chromosomally encoded ESBL genes in Escherichia coli of ST38 from Mongolian wild birds. J. Antimicrob. Chemother. 72, 1310–1313. doi: 10.1093/jac/dkx006
Gullberg, E., Cao, S., Berg, O. G., Ilbäck, C., Sandegren, L., Hughes, D., et al. (2011). Selection of resistant bacteria at very low antibiotic concentrations. PLoS Pathog. 7:e1002158. doi: 10.1371/journal.ppat.1002158
Halova, D., Papousek, I., Jamborova, I., Masarikova, M., Cizek, A., Janecko, N., et al. (2014). Plasmid-mediated quinolone resistance genes in Enterobacteriaceae from American crows: high prevalence of bacteria with variable qnrB genes. Antimicrob. Agents Chemother. 58, 1257–1258. doi: 10.1128/AAC.01849-13
Hansen, L. H., Jensen, L. B., Sorensen, H. I., and Sorensen, S. J. (2007). Substrate specificity of the OqxAB multidrug resistance pump in Escherichia coli and selected enteric bacteria. J. Antimicrob. Chemother. 60, 145–147. doi: 10.1093/jac/dkm167
Heisig, P. (1996). Genetic evidence for a role of parC mutations in development of high-level fluoroquinolone resistance in Escherichia coli. Antimicrob. Agents Chemother. 40, 879–885.
Hobman, J. L., and Crossman, L. C. (2015). Bacterial antimicrobial metal ion resistance. J. Med. Microbiol. 64(Pt. 5), 471–497. doi: 10.1099/jmm.0.023036-0
Hunter, P. R., and Gaston, M. A. (1988). Numerical index of the discriminatory ability of typing systems: an application of Simpson’s index of diversity. J. Clin. Microbiol. 26, 2465–2466.
Jimenez Gomez, P. A., Garcia de los Rios, J. E., Rojas Mendoza, A., de Pedro Ramonet, P., Garcia Albiach, R., and Reche Sainz, M. P. (2004). Molecular basis of quinolone resistance in Escherichia coli from wild birds. Can. J. Vet. Res. 68, 229–231.
Joensen, K. G., Scheutz, F., Lund, O., Hasman, H., Kaas, R. S., Nielsen, E. M., et al. (2014). Real-Time Whole-Genome Sequencing for Routine Typing, Surveillance, and Outbreak Detection of Verotoxigenic Escherichia coli. J. Clin. Microbiol. 52:1501. doi: 10.1128/JCM.03617-13
Kaas, R. S., Leekitcharoenphon, P., Aarestrup, F. M., and Lund, O. (2014). Solving the problem of comparing whole bacterial genomes across different sequencing platforms. PLoS One 9:e104984. doi: 10.1371/journal.pone.0104984
Keefer, A. B., Xiaoli, L., M’Ikanatha, N. M., Yao, K., Hoffmann, M., and Dudley, E. G. (2019). Retrospective whole-genome sequencing analysis distinguished PFGE and drug-resistance-matched retail meat and clinical Salmonella isolates. Microbiology 165, 270–286. doi: 10.1099/mic.0.000768
Komp Lindgren, P., Karlsson, A., and Hughes, D. (2003). Mutation rate and evolution of fluoroquinolone resistance in Escherichia coli isolates from patients with urinary tract infections. Antimicrob. Agents Chemother. 47, 3222–3232. doi: 10.1128/aac.47.10.3222-3232.2003
Lalak, A., Wasyl, D., Zaja̧c, M., Skarżyńska, M., Hoszowski, A., Samcik, I., et al. (2016). Mechanisms of cephalosporin resistance in indicator Escherichia coli isolated from food animals. Vet. Microbiol. 194, 69–73. doi: 10.1016/j.vetmic.2016.01.023
Larsen, M. V., Cosentino, S., Rasmussen, S., Friis, C., Hasman, H., Marvig, R. L., et al. (2012). Multilocus sequence typing of total-genome-sequenced bacteria. J. Clin. Microbiol. 50, 1355–1361. doi: 10.1128/JCM.06094-11
Letunic, I., and Bork, P. (2019). Interactive Tree Of Life (iTOL) v4: recent updates and new developments. Nucleic Acids Res. 47, W256–W259. doi: 10.1093/nar/gkz239
Ling, J. M., Chan, E. W., Lam, A. W., and Cheng, A. F. (2003). Mutations in topoisomerase genes of fluoroquinolone-resistant Salmonellae in Hong Kong. Antimicrob. Agents Chemother. 47, 3567–3573. doi: 10.1128/aac.47.11.3567-3573.2003
Literak, I., Dolejska, M., Janoszowska, D., Hrusakova, J., Meissner, W., Rzyska, H., et al. (2010). Antibiotic-resistant Escherichia coli bacteria, including strains with genes encoding the extended-spectrum beta-lactamase and QnrS, in waterbirds on the Baltic Sea Coast of Poland. Appl. Environ. Microbiol. 76, 8126–8134. doi: 10.1128/AEM.01446-10
Literak, I., Micudova, M., Tausova, D., Cizek, A., Dolejska, M., Papousek, I., et al. (2012). Plasmid-mediated quinolone resistance genes in fecal bacteria from rooks commonly wintering throughout Europe. Microb. Drug Resist. 18, 567–573. doi: 10.1089/mdr.2012.0075
Magiorakos, A. P., Srinivasan, A., Carey, R. B., Carmeli, Y., Falagas, M. E., Giske, C. G., et al. (2012). Multidrug-resistant, extensively drug-resistant and pandrug-resistant bacteria: an international expert proposal for interim standard definitions for acquired resistance. Clin. Microbiol. Infect. 18, 268–281. doi: 10.1111/j.1469-0691.2011.03570.x
Marcelino, V. R., Wille, M., Hurt, A. C., Gonzalez-Acuna, D., Klaassen, M., Schlub, T. E., et al. (2019). Meta-transcriptomics reveals a diverse antibiotic resistance gene pool in avian microbiomes. BMC Biol. 17:31. doi: 10.1186/s12915-019-0649-1
Marrow, J., Whittington, J. K., Mitchell, M., Hoyer, L. L., and Maddox, C. (2009). Prevalence and antibiotic-resistance characteristics of Enterococcus spp. Isolated from free-living and captive raptors in Central Illinois. J. Wildl. Dis. 45, 302–313. doi: 10.7589/0090-3558-45.2.302
Martinez, J. L. (2009). Environmental pollution by antibiotics and by antibiotic resistance determinants. Environ. Pollut. 157, 2893–2902. doi: 10.1016/j.envpol.2009.05.051
Martínez, J. L. (2012). Natural antibiotic resistance and contamination by antibiotic resistance determinants: the two ages in the evolution of resistance to antimicrobials. Front. Microbiol. 3:1. doi: 10.3389/fmicb.2012.00001
Miller, R. V., Gammon, K., and Day, M. J. (2009). Antibiotic resistance among bacteria isolated from seawater and penguin fecal samples collected near Palmer Station, Antarctica. Can. J. Microbiol. 55, 37–45. doi: 10.1139/w08-119
Mo, S. S., Urdahl, A. M., Madslien, K., Sunde, M., Nesse, L. L., Slettemeås, J. S., et al. (2018). What does the fox say? Monitoring antimicrobial resistance in the environment using wild red foxes as an indicator. PLoS One 13:e0198019. doi: 10.1371/journal.pone.0198019
Mohsin, J., Pal, T., Petersen, J. E., Darwish, D., Ghazawi, A., Ashraf, T., et al. (2018). Plasmid-mediated colistin resistance Gene mcr-1 in an Escherichia coli ST10 bloodstream isolate in the sultanate of Oman. Microb. Drug Resist. 24, 278–282. doi: 10.1089/mdr.2017.0131
Mohsin, M., Raza, S., Roschanski, N., Schaufler, K., and Guenther, S. (2016). First description of plasmid-mediated colistin-resistant extended-spectrum beta-lactamase-producing Escherichia coli in a wild migratory bird from Asia. Int. J. Antimicrob. Agents 48, 463–464. doi: 10.1016/j.ijantimicag.2016.07.001
Mohsin, M., Raza, S., Schaufler, K., Roschanski, N., Sarwar, F., Semmler, T., et al. (2017). High prevalence of CTX-M-15-Type ESBL-producing E. coli from migratory avian species in Pakistan. Front. Microbiol. 8:2476. doi: 10.3389/fmicb.2017.02476
Nabil, N. M., Erfan, A. M., Tawakol, M. M., Haggag, N. M., Naguib, M. M., and Samy, A. (2020). Wild birds in live birds markets: potential reservoirs of enzootic avian influenza viruses and antimicrobial resistant Enterobacteriaceae in Northern Egypt. Pathogens 9:196. doi: 10.3390/pathogens9030196
Nascimento, A. M. A., Cursino, L., Gonçalves-Dornelas, H., Reis, A., Chartone-Souza, E., and Marini, M. Â (2003). Antibiotic-resistant gram-negative bacteria in birds from the Brazilian Atlantic forest. Condor 105, 358–361. doi: 10.1093/condor/105.2.358
Navarro-Gonzalez, N., Porrero, M. C., Mentaberre, G., Serrano, E., Mateos, A., Dominguez, L., et al. (2013). Antimicrobial resistance in indicator Escherichia coli isolates from free-ranging livestock and sympatric wild ungulates in a natural environment (Northeastern Spain). Appl. Environ. Microbiol. 79, 6184–6186. doi: 10.1128/AEM.01745-13
Nicolas-Chanoine, M. H., Bertrand, X., and Madec, J. Y. (2014). Escherichia coli ST131, an intriguing clonal group. Clin. Microbiol. Rev. 27, 543–574. doi: 10.1128/CMR.00125-13
Oh, J. Y., Kwon, Y. K., Tamang, M. D., Jang, H. K., Jeong, O. M., Lee, H. S., et al. (2016). Plasmid-Mediated quinolone resistance in Escherichia coli isolates from wild birds and chickens in South Korea. Microb. Drug Resist. 22, 69–79. doi: 10.1089/mdr.2015.0090
Oteo, J., Mencia, A., Bautista, V., Pastor, N., Lara, N., Gonzalez-Gonzalez, F., et al. (2018). Colonization with Enterobacteriaceae-Producing ESBLs, AmpCs, and OXA-48 in Wild avian species, Spain 2015-2016. Microb. Drug Resist. 24, 932–938. doi: 10.1089/mdr.2018.0004
Papa-Ezdra, R., Grill Diaz, F., Vieytes, M., Garcia-Fulgueiras, V., Caiata, L., Avila, P., et al. (2020). First three Escherichia coli isolates harbouring mcr-1 in Uruguay. J. Glob. Antimicrob. Resist. 20, 187–190. doi: 10.1016/j.jgar.2019.07.016
Pinto, L., Radhouani, H., Coelho, C., Martins da Costa, P., Simoes, R., Brandao, R. M., et al. (2010). Genetic detection of extended-spectrum beta-lactamase-containing Escherichia coli isolates from birds of prey from Serra da Estrela natural reserve in Portugal. Appl. Environ. Microbiol. 76, 4118–4120. doi: 10.1128/AEM.02761-09
Poirel, L., Potron, A., De La Cuesta, C., Cleary, T., Nordmann, P., and Munoz-Price, L. S. (2012). Wild Coastline Birds as Reservoirs of Broad-Spectrum-β-Lactamase-Producing Enterobacteriaceae in Miami Beach, Florida. Antimicrob. Agents Chemother. 56, 2756–2758. doi: 10.1128/aac.05982-11
Rabbia, V., Bello-Toledo, H., Jiménez, S., Quezada, M., Domínguez, M., Vergara, L., et al. (2016). Antibiotic resistance in Escherichia coli strains isolated from Antarctic bird feces, water from inside a wastewater treatment plant, and seawater samples collected in the Antarctic Treaty area. Polar Sci. 10, 123–131. doi: 10.1016/j.polar.2016.04.002
Ramadan, H., Jackson, C. R., Frye, J. G., Hiott, L. M., Samir, M., Awad, A., et al. (2020). Antimicrobial resistance, genetic diversity and multilocus sequence typing of Escherichia coli from Humans, retail chicken and ground beef in Egypt. Pathogens 9:357. doi: 10.3390/pathogens9050357
Rega, M., Carmosino, I., Bonilauri, P., Frascolla, V., Vismarra, A., and Bacci, C. (2021). Prevalence of ESβL, AmpC and Colistin-Resistant E. coli in Meat: a comparison between Pork and Wild Boar. Microorganisms 9:214. doi: 10.3390/microorganisms9020214
Rehman, M. A., Yin, X., Persaud-Lachhman, M. G., and Diarra, M. S. (2017). First Detection of a fosfomycin resistance gene, fosA7, in Salmonella enterica serovar heidelberg isolated from broiler chickens. Antimicrob. Agents Chemother. 61, e410–e417. doi: 10.1128/AAC.00410-17
Rodríguez, I., Thomas, K., Van Essen, A., Schink, A. K., Day, M., Chattaway, M., et al. (2014). Chromosomal location of blaCTX-M genes in clinical isolates of Escherichia coli from Germany, The Netherlands and the UK. Int. J. Antimicrob. Agents 43, 553–557. doi: 10.1016/j.ijantimicag.2014.02.019
Ronco, T., Stegger, M., Olsen, R. H., Sekse, C., Nordstoga, A. B., Pohjanvirta, T., et al. (2017). Spread of avian pathogenic Escherichia coli ST117 O78:H4 in Nordic broiler production. BMC Genomics 18:13. doi: 10.1186/s12864-016-3415-6
Rozwandowicz, M., Brouwer, M. S. M., Fischer, J., Wagenaar, J. A., Gonzalez-Zorn, B., Guerra, B., et al. (2018). Plasmids carrying antimicrobial resistance genes in Enterobacteriaceae. J. Antimicrob. Chemother. 73, 1121–1137. doi: 10.1093/jac/dkx488
Ruzauskas, M., and Vaskeviciute, L. (2016). Detection of the mcr-1 gene in Escherichia coli prevalent in the migratory bird species Larus argentatus. J. Antimicrob. Chemother. 71, 2333–2334. doi: 10.1093/jac/dkw245
Sarowska, J., Futoma-Koloch, B., Jama-Kmiecik, A., Frej-Madrzak, M., Ksiazczyk, M., Bugla-Ploskonska, G., et al. (2019). Virulence factors, prevalence and potential transmission of extraintestinal pathogenic Escherichia coli isolated from different sources: recent reports. Gut. Pathog. 11:10. doi: 10.1186/s13099-019-0290-0
Seemann, T. (2019). Abricate, Github. Available online at: https://github.com/tseemann/abricate (accessed March 1, 2020).
Sghaier, S., Abbassi, M. S., Pascual, A., Serrano, L., Díaz-De-Alba, P., Said, M. B., et al. (2019). Extended-spectrum β-lactamase-producing Enterobacteriaceae from animal origin and wastewater in Tunisia: first detection of O25b-B23-CTX-M-27-ST131 Escherichia coli and CTX-M-15/OXA-204-producing Citrobacter freundii from wastewater. J. Glob. Antimicrob. Resist. 17, 189–194. doi: 10.1016/j.jgar.2019.01.002
Silva, M. M., Sellera, F. P., Fernandes, M. R., Moura, Q., Garino, F., Azevedo, S. S., et al. (2018). Genomic features of a highly virulent, ceftiofur-resistant, CTX-M-8-producing Escherichia coli ST224 causing fatal infection in a domestic cat. J. Glob. Antimicrob. Resist. 15, 252–253. doi: 10.1016/j.jgar.2018.10.023
Skarżyńska, M., Leekitcharoenphon, P., Hendriksen, R. S., Aarestrup, F. M., and Wasyl, D. (2020a). Metagenomic glimpse into the animal gut of wild and domestic animals. PLoS One 15:e024298. doi: 10.1371/journal.pone.0242987
Skarżyńska, M., Zaja̧c, M., Kamińska, E., Bomba, A., Żmudzki, J., Jabłoński, A., et al. (2020b). Salmonella and antimicrobial resistance in wild rodents-true or false threat? Pathogens 9:771. doi: 10.3390/pathogens9090771
Swift, B. M. C., Bennett, M., Waller, K., Dodd, C., Murray, A., Gomes, R. L., et al. (2019). Anthropogenic environmental drivers of antimicrobial resistance in wildlife. Sci. Total Environ. 649, 12–20. doi: 10.1016/j.scitotenv.2018.08.180
Touati, M., Hadjadj, L., Berrazeg, M., Baron, S. A., and Rolain, J. M. (2020). Emergence of Escherichia coli harbouring mcr-1 and mcr-3 genes in North West Algerian farmlands. J. Glob. Antimicrob. Resist. 21, 132–137. doi: 10.1016/j.jgar.2019.10.001
van Hoek, A., van Veldhuizen, J. N. J., Friesema, I., Coipan, C., Rossen, J. W. A., Bergval, I. L., et al. (2019). Comparative genomics reveals a lack of evidence for pigeons as a main source of stx(2f)-carrying Escherichia coli causing disease in humans and the common existence of hybrid Shiga toxin-producing and enteropathogenic E. coli pathotypes. BMC Genomics 20:271. doi: 10.1186/s12864-019-5635-z
Veldman, K. T., Tulden, P. W. V., Kant, A. D., Testerink, J., and Mevius, D. J. (2013). Characteristics of cefotaxime-resistant Escherichia coli from wild birds in the Netherlands. Appl. Environ. Microbiol. 79, 7556–7561.
Vila, J., Ruiz, J., Goni, P., and De Anta, M. T. (1996). Detection of mutations in parC in quinolone-resistant clinical isolates of Escherichia coli. Antimicrob. Agents Chemother. 40, 491–493.
Villa, L., Guerra, B., Schmoger, S., Fischer, J., Helmuth, R., Zong, Z., et al. (2015). IncA/C Plasmid Carrying bla(NDM-1), bla(CMY-16), and fosA3 in a Salmonella enterica Serovar Corvallis strain isolated from a migratory wild bird in Germany. Antimicrob. Agents Chemother. 59, 6597–6600. doi: 10.1128/AAC.00944-15
Vittecoq, M., Laurens, C., Brazier, L., Durand, P., Elguero, E., Arnal, A., et al. (2017). VIM-1 carbapenemase-producing Escherichia coli in gulls from southern France. Ecol. Evol. 7, 1224–1232. doi: 10.1002/ece3.2707
Vredenburg, J., Varela, A. R., Hasan, B., Bertilsson, S., Olsen, B., Narciso-da-Rocha, C., et al. (2014). Quinolone-resistant Escherichia coli isolated from birds of prey in Portugal are genetically distinct from those isolated from water environments and gulls in Portugal, Spain and Sweden. Environ. Microbiol. 16, 995–1004. doi: 10.1111/1462-2920.12231
Wasyl, D., Hoszowski, A., Zaja̧c, M., and Szulowski, K. (2013). Antimicrobial resistance in commensal Escherichia coli isolated from animals at slaughter. Front. Microbiol. 4:221. doi: 10.3389/fmicb.2013.00221
Wasyl, D., Zaja̧c, M., Lalak, A., Skarżyńska, M., Samcik, I., Kwit, R., et al. (2018). Antimicrobial resistance in Escherichia coli isolated from wild animals in Poland. Microb. Drug Resist. 24, 807–815. doi: 10.1089/mdr.2017.0148
World Health Organization [WHO] (2014). Antimicrobial Resistance: Global Report on Surveillance. Geneva: World Health Organization. ISBN 978 92 4 156474 8
World Health Organization [WHO] (2018). WHO Report on Surveillance of Antibiotic Consumption:2016-2018 Early Implementation. Licence: CC BY-NC-SA 3.0 IGO. Geneva: World Health Organization.
World Health Organization [WHO] (2019). Critically Important Antimicrobials for Human Medicine, 6th Revision 2018. Geneva: World Health Organization, 1–45. ISBN: 978-92-4-151552-8
Yang, Y. Q., Li, Y. X., Song, T., Yang, Y. X., Jiang, W., Zhang, A. Y., et al. (2017). Colistin resistance gene mcr-1 and its variant in Escherichia coli isolates from chickens in China. Antimicrob. Agents Chemother. 61, e1204–e1216. doi: 10.1128/aac.01204-16
Zaja̧c, M., Sztromwasser, P., Bortolaia, V., Leekitcharoenphon, P., Cavaco, L. M., Ziȩtek-Barszcz, A., et al. (2019). Occurrence and characterization of mcr-1-Positive Escherichia coli isolated from food-producing animals in Poland, 2011-2016. Front. Microbiol. 10:1753. doi: 10.3389/fmicb.2019.01753
Zankari, E., Hasman, H., Cosentino, S., Vestergaard, M., Rasmussen, S., Lund, O., et al. (2012). Identification of acquired antimicrobial resistance genes. J. Antimicrob. Chemother. 67, 2640–2644. doi: 10.1093/jac/dks261
Zhou, Z., Alikhan, N. F., Mohamed, K., Fan, Y., Agama Study, G., and Achtman, M. (2020). The enterobase user’s guide, with case studies on Salmonella transmissions, Yersinia pestis phylogeny, and Escherichia core genomic diversity. Genome Res. 30, 138–152. doi: 10.1101/gr.251678.119
Keywords: antimicrobial resistance, free-living birds, AMR, wildlife, E. coli
Citation: Skarżyńska M, Zaja̧c M, Bomba A, Bocian Ł, Kozdruń W, Polak M, Wia̧cek J and Wasyl D (2021) Antimicrobial Resistance Glides in the Sky—Free-Living Birds as a Reservoir of Resistant Escherichia coli With Zoonotic Potential. Front. Microbiol. 12:656223. doi: 10.3389/fmicb.2021.656223
Received: 20 January 2021; Accepted: 05 March 2021;
Published: 09 April 2021.
Edited by:
Astrid Louise Wester, Norwegian Institute of Public Health (NIPH), NorwayReviewed by:
Mohamed Salah Abbassi, Tunis El Manar University, TunisiaCopyright © 2021 Skarżyńska, Zaja̧c, Bomba, Bocian, Kozdruń, Polak, Wia̧cek and Wasyl. This is an open-access article distributed under the terms of the Creative Commons Attribution License (CC BY). The use, distribution or reproduction in other forums is permitted, provided the original author(s) and the copyright owner(s) are credited and that the original publication in this journal is cited, in accordance with accepted academic practice. No use, distribution or reproduction is permitted which does not comply with these terms.
*Correspondence: Magdalena Skarżyńska, bWFnZGFsZW5hLnNrYXJ6eW5za2FAcGl3ZXQucHVsYXd5LnBs
Disclaimer: All claims expressed in this article are solely those of the authors and do not necessarily represent those of their affiliated organizations, or those of the publisher, the editors and the reviewers. Any product that may be evaluated in this article or claim that may be made by its manufacturer is not guaranteed or endorsed by the publisher.
Research integrity at Frontiers
Learn more about the work of our research integrity team to safeguard the quality of each article we publish.