- Department of Horticulture and Crop Science, Ohio Agricultural Research and Development Center, The Ohio State University, Wooster, OH, United States
Water stress decreases the health and quality of horticulture crops by inhibiting photosynthesis, transpiration, and nutrient uptake. Application of plant growth promoting rhizobacteria (PGPR) can increase the growth, stress tolerance, and overall quality of field and greenhouse grown crops subjected to water stress. Here, we evaluated Serratia plymuthica MBSA-MJ1 for its ability to increase plant growth and quality of Petunia × hybrida (petunia), Impatiens walleriana (impatiens), and Viola × wittrockiana (pansy) plants recovering from severe water stress. Plants were treated weekly with inoculum of MBSA-MJ1, and plant growth and quality were evaluated 2 weeks after recovery from water stress. Application of S. plymuthica MBSA-MJ1 increased the visual quality and shoot biomass of petunia and impatiens and increased the flower number of petunia after recovery from water stress. In addition, in vitro characterizations showed that MBSA-MJ1 is a motile bacterium with moderate levels of antibiotic resistance that can withstand osmotic stress. Further, comprehensive genomic analyses identified genes putatively involved in bacterial osmotic and oxidative stress responses and the synthesis of osmoprotectants and vitamins that could potentially be involved in increasing plant water stress tolerance. This work provides a better understanding of potential mechanisms involved in beneficial plant-microbe interactions under abiotic stress using a novel S. plymuthica strain as a model.
Introduction
Abiotic stresses negatively impact a multitude of biochemical and physiological responses in crops, reducing plant growth, performance, and quality (Anjum et al., 2017; Nordstedt et al., 2020). Water stress, in particular, can permanently damage the photosynthetic health of plants, leading to a reduction in plant size and yield (Armitage et al., 1983; Zargar et al., 2017; Liang et al., 2020; Nordstedt and Jones, 2020). A decrease in available water leads to a reduction in total nutrient uptake and increases in cellular oxidative damage that inhibit plant growth (Farooq et al., 2009). Although the negative impact of water stress on the yield of food crops is well-documented, water stress can also decrease the quality of high-value ornamental crops grown in containers, which are more prone to rapid fluxes in water availability (Nordstedt et al., 2020). Water stress reduces the marketability of ornamental crops by negatively impacting plant size, flowering, and leaf color (Sánchez-Blanco et al., 2009). In addition to reducing the appearance of these high-value crops, damage to photosynthetic health can lead to reduced transplant success and plant vigor in the customer’s garden (Armitage et al., 1983; Sun et al., 2020).
Similar to plants, microbial communities are strongly influenced by water stress, which creates unfavorable osmotic conditions and increases competition for resources (Makhalanyane et al., 2015; Ngumbi and Kloepper, 2016). As water content in the rhizosphere decreases, bacteria must adapt by accumulating solutes, producing exopolysaccharides, and/or producing spores to survive. Fortunately, many of the strategies that rhizospheric bacteria utilize to cope with water stress can also be beneficial in helping their plant host overcome severe water stress. These beneficial bacteria are termed plant growth promoting rhizobacteria (PGPR), and they can increase plant growth and crop quality under a variety of environmental stresses including water stress (Ngumbi and Kloepper, 2016; Vurukonda et al., 2016; Backer et al., 2018).
These plant-microbe interactions are established via root exudates that are secreted into the rhizosphere (Lugtenberg and Kamilova, 2009). Root exudates comprise a variety of compounds that recruit and support microbial growth, such as sugars, amino acids, organic acids, secondary metabolites, carbon dioxide, and water. Plants secrete these exudates into the rhizosphere to recruit and support microbial growth, and in return PGPR can modulate phytohormone levels, influence root architecture, synthesize compounds such as osmoprotectants or vitamins, and increase the availability of nutrients to plants (Ngumbi and Kloepper, 2016). The composition of these root exudates changes during times of water stress, potentially optimizing conditions for PGPR to survive and continue supporting their plant host (Gargallo-Garriga et al., 2018).
In this work, we have identified Serratia plymuthica MBSA-MJ1 as a PGPR that improves plant growth and flowering of ornamental plant species after recovery from severe water stress. Serratia are a group of widespread gram-negative bacteria found in water, soil, and animals. The nature of Serratia species ranges from parasitic to mutualistic, however, many share the ability to survive under diverse environmental conditions and compete with other microorganisms to successfully colonize within their ecological niche (Petersen and Tisa, 2013). In addition to being found in a variety of environments, plant-associated Serratia spp. are also found to colonize a variety of plant hosts in the endosphere, phyllosphere, and rhizosphere (Benhamou et al., 2000; De Vleesschauwer and Höfte, 2007; Müller et al., 2013; Martínez et al., 2018).
Strains of S. plymuthica are most commonly associated with plant roots and have been isolated from a variety of plant hosts, including oilseed rape, poplar trees, wheat, potato, pumpkin, maize, and rice (Åström and Gerhardson, 1988; Gyaneshwar et al., 2001; Berg et al., 2002, 2006; van der Lelie et al., 2009; Garbeva et al., 2012; Müller et al., 2013; Neupane, 2013). Because of their association with plant roots, they have been studied extensively for their use as both biocontrol agents against soil-dwelling phytopathogens and as PGPR. Their efficacy as both a biocontrol agent and a PGPR is commonly attributed to the production of antimicrobial compounds, phytohormones, and secondary metabolites (Lavania et al., 2006; Matilla et al., 2016). Most reports of plant-associated S. plymuthica strains show evidence of biocontrol activity (Berg, 2000; Pang et al., 2009; Czajkowski and van der Wolfa, 2012; Garbeva et al., 2012; Müller et al., 2013; Adam et al., 2016), while some also report the additional ability to stimulate plant growth (Neupane, 2013; Li et al., 2020). Recently, S. plymuthica strains have been reported to colonize Arabidopsis thaliana (Proença et al., 2019) and stimulate the growth of water stressed Ziziphus jujuba (Zhang et al., 2020). The strain Serratia sp. 1–9 stimulates growth of wheat plants subjected to severe water stress (Wang et al., 2014), while other Serratia spp. stimulate plant growth under salt stress (El-Esawi et al., 2018), in heavy metal contaminated soils (Koo and Cho, 2009), and under low temperature stress (Zhang et al., 1997; Kang et al., 2015).
These beneficial plant-microbe interactions are very complex, and the complexity contributes to a lack of clarity as to how some bacteria increase plant growth and stress tolerance. These bacteria must be able to survive under the stressful environmental conditions that are impacting their plant host, while colonizing the rhizosphere or the plant tissue (i.e., endophytes) without causing disease symptoms. The microbial symbiont can then begin positively influencing plant growth and stress tolerance by modulating phytohormone levels, increasing nutrient availability, or producing osmoprotectants and vitamins for plant utilization. However, the mechanisms that are employed can range widely between different strains of PGPR. Whole-genome sequencing data provide insight into the dynamic mechanisms that PGPR might be utilizing in these complex interactions. To date there are over 1,000 Serratia spp. reference genomes publicly available, however, there are a limited number of references available for plant-associated strains. Additionally, there are currently no publicly available genome data for S. plymuthica strains that increase growth of water stressed plants. Therefore, the genomic analysis completed here, coupled with the in vitro and in planta experiments provide support for pathways or biochemical processes that S. plymuthica MBSA-MJ1 might be utilizing to survive, colonize, and ultimately increase plant growth under water stress. Identification of putative genes underlying the plant growth-promoting traits of this novel bacterium will lead to a better understanding of how bacteria can influence plant growth during water stress and contribute to future work in functional gene analysis.
Materials and Methods
Bacterial Strain
Serratia plymuthica MBSA-MJ1 is part of a bacteria collection from the laboratory of Dr. Christopher Taylor (The Ohio State University; Aly et al., 2007), although the exact source of this specific strain is unknown. MBSA-MJ1 was previously evaluated for its ability to reduce the severity of Botrytis cinerea infection in Petunia × hybrida (South et al., 2020).
Water Stress Greenhouse Trial
The ability of S. plymuthica MBSA-MJ1 to increase the quality and growth of plants after recovery from water stress was evaluated using a previously established in planta greenhouse trialing protocol (Nordstedt et al., 2020). Greenhouse conditions were set to maintain canopy temperature at 24/18°C (day/night). Supplemental lighting was provided with metal halide and high-pressure sodium lights (GLX/GLS e-systems GROW lights, PARSource, Petaluma, CA, United States) to provide a 16 h photoperiod and maintain light levels above 250 mmol m−2 s−1. The experiment included three economically important ornamental crops including: Petunia × hybrida “Picobella Blue” (petunia; Syngenta Flowers Gilroy, CA), Impatiens walleriana “Super Elfin Ruby” (impatiens; PanAmerican Seed, West Chicago, IL), and Viola × wittrockiana “Delta Pure Red” (pansy; Syngenta Flowers). Seeds were sown for each of the plant species and transplanted after 3 weeks into 11.4 cm diameter pots containing Pro-Mix PGX potting media (Premier Tech Horticulture, Quakertown, PA, United States). Plants were arranged in a Randomized Complete Block Design (RCBD) with one plant per block with 13, 14, and 18 blocks for petunia, pansy, and impatiens, respectively. Plants were fertilized at each irrigation with a water-soluble fertilizer at a rate of 50 mg L−1 N from 15 N–2.2P–12.5 K–2.9Ca–1.2 Mg (JR Peters Inc., Allentown, PA, United States).
Following transplant, 120 ml inoculum of MBSA-MJ1 was applied weekly to each treatment plant. Inoculum was prepared by diluting an overnight culture grown in LB media (OD595 = 0.8) 1:100 in reverse osmosis (RO) water as described previously (Nordstedt et al., 2020). Uninoculated LB media diluted in water served as the negative control. Plants were subjected to water stress at 5 weeks post-transplant by temporarily ceasing all irrigation and bacterial treatments until all plants showed a loss of turgidity in their leaves (wilted). To assess recovery, plants were then rewatered with clear water (i.e., no fertilizer) and weekly bacterial treatments were resumed. Plant growth and quality after recovery from water stress were evaluated at 8 weeks post-transplant, which was approximately 2 weeks after the plants were rewatered. These parameters were evaluated by counting flowers (open flowers plus buds showing color) on each plant and collecting shoots (flowers, leaves, and stems) to measure total dry weight. Root-adhering potting media were removed from the roots, and root tissue was collected separately from shoot tissue for dry weights. Root and shoot tissue were dried at 49°C for at least 96 h prior to dry weight measurements.
In vitro Characterization of Strain MBSA-MJ1
Growth Under Osmotic Stress
Serratia plymuthica MBSA-MJ1 was evaluated for its ability to grow under polyethylene glycol (PEG)-induced osmotic stress conditions according to Nordstedt et al. (2020). Briefly, overnight cultures of MBSA-MJ1 were used to inoculate Yeast Extract Mannitol (YEM) media amended with 30% PEG8000. After 96 h incubation, OD595 was measured as an indicator of growth under osmotic stress conditions.
ACC Deaminase Activity
Enzyme activity of 1-aminocyclopropane-1-carboxcylic acid (ACC) deaminase by S. plymuthica MBSA-MJ1 was evaluated using a colorimetric assay according to Nordstedt and Jones (2020). Pseudomonas putida UW4 (formerly Enterobacter cloacae UW4) was used as the positive control for ACC deaminase activity (Shah et al., 1998), and an uninoculated sample was used for the negative control. Briefly, cultures of MBSA-MJ1 and UW4 were incubated in tryptic soy broth (TSB) for 48 h, then bacterial cells were collected by centrifugation, washed in Dworkin and Foster (DF) media, resuspended in DF media containing ACC as the sole nitrogen source, and incubated for an additional 24 h. After incubation, cells were again collected by centrifugation, triple washed with Tris-HCl, and resuspended in Tris-HCl supplemented with toluene. The samples were added to wells of a microtiter plate containing 0.5 M ACC and incubated in a 30°C water bath for 15 min. HCl was added to the samples and the cells were again collected by centrifugation. The supernatant was mixed with HCl and 2,4-dinitrophenylhydrazine, and the samples were incubated in the water bath for 30 min. NaOH was added to the samples and the absorbance was measured at 540 nm to quantify levels of α-ketobutyrate, the product of ACC degradation by ACC deaminase.
Antibiotic Resistance
To test antibiotic resistance of S. plymuthica MBSA-MJ1, 0.5X LB agar plates were prepared and supplemented with 1X and 0.5X concentrations of each antibiotic, with 1X concentrations as follows: rifampicin (50 μg/ml), streptomycin (50 μg/ml), kanamycin (50 μg/ml), ampicillin (100 μg/ml), chloramphenicol (25 μg/ml), gentamicin (25 μg/ml), carbenicillin (100 μg/ml), and tetracycline (5 μg/ml). Cells of MBSA-MJ1 from overnight culture were collected by centrifugation, resuspended in phosphate-buffered saline (PBS) buffer, and 5 μl was struck out onto each antibiotic media in triplicate (n = 3). Plates were incubated at 28°C for 96 h and then growth on each antibiotic was recorded as yes or no.
Motility
In vitro motility was evaluated according to Sun et al. (2006). Overnight culture of S. plymuthica MBSA-MJ1 was diluted to OD595 = 0.8 and then stabbed in nutrient yeast glucose agar (NYGA) semi-solid agar media in triplicate (n = 3). The stabbed culture was incubated at 28°C for 96 h, at which point motility was determined by the ability of the culture to grow away from the inoculation stab.
Whole-Genome Sequencing of Strain MBSA-MJ1
The whole-genome sequence of S. plymuthica MBSA-MJ1 was generated to gain insight into the strain’s biology and potential mechanisms to withstand osmotic stress and stimulate the growth of plants during recovery from water stress. Genomic DNA of S. plymuthica MBSA-MJ1 was extracted from an overnight liquid culture using the Quick-DNA Bacterial Miniprep kit (Zymo Research, Irvine, CA, United States) and then submitted to Diversigen (formerly CoreBiome; St. Paul, MN) for whole genome shotgun sequencing using the Illumina NovaSeq platform similar to Nordstedt and Jones (2020). Annotation files generated by Prokka (Seemann, 2014) were searched for genes putatively involved in bacterial stress tolerance and plant growth promotion. Kyoto Encyclopedia of Genes and Genomes (KEGG) pathway annotation and mapping was conducted using BlastKOALA (v2.2; Kanehisa et al., 2016). Secreted proteins were predicted using the SignalP webserver (v5.0; Almagro Armenteros et al., 2019), and PlasmidSPAdes was used for detection of plasmid sequences within the genomic data (Antipov et al., 2016).
Taxonomic Classification and Phylogenetic Comparison
Taxonomy was assigned to S. plymuthica MBSA-MJ1 using the Microbial Genomes Atlas (MiGA) because of the program’s ability to compare a query sequence against all taxonomically classified taxa in the NCBI prokaryotic genome database (Rodriguez-R et al., 2018). MiGA assigns taxonomy based on the average nucleotide identity (ANI) concept, which requires >94% ANI to classify two sequences as the same species (Konstantinidis and Tiedje, 2005).
Following taxonomic classification, phylogenetic relationships were established using reference genome sequences of 22 S. plymuthica, 13 other Serratia spp., and eight closely-related species (according to MiGA ANI results) from the order Enterobacterales. The 13 additional Serratia spp. strains were chosen to represent one strain for each Serratia species in the NCBI genome database. The ANI values were based on BLAST and calculated using the webtool Enveomics (Rodriguez-R and Konstantinidis, 2016). Distance was estimated using the neighbor-joining clustering method. The Newick output files from the Enveomics ANI program were used to construct the phylogenetic tree using TreeDyn (Chevenet et al., 2006). Additionally, ANI matrix values between the Serratia strains were used to determine clades within the phylogenetic tree. Strains that shared the highest level of ANI similarity were grouped, with each clade consisting of strains that each share at least 94% ANI with each other. Strains that do not share at least 94% ANI with any of the strains were not assigned to a clade.
Statistical Analysis
Statistical analyses for the production-scale greenhouse trial were conducted in R Studio version 3.5.2 using an ANOVA with the model: Y = μ + treatment + block. The Tukey’s HSD test was used to determine statistical significance between MBSA-MJ1 treatment and the negative control. Each plant species was analyzed independently of each other.
Data Availability
The bacterial genome sequence data were deposited with NCBI under BioSample number SAMN16442191.
Results
Plant Growth Promotion Under Water Stress
Serratia plymuthica MBSA-MJ1 was initially identified as a bacteria that promoted plant growth in Petunia × hybrida “Picobella Blue” after recovery from water stress in a high throughput greenhouse trial (data not shown). MBSA-MJ1 application increased total shoot dry weight by an average of 14% and increased flower number by an average of three flowers per plant.
In this manuscript, we present experiments evaluating the growth promoting effects of MBSA-MJ1 on petunia, impatiens, and pansy by assessing growth and flowering after plants were subjected to severe water stress. Application of MBSA-MJ1 significantly increased the shoot biomass of petunia and impatiens compared to the uninoculated control. The greatest effect was observed with petunia, which had an average increase of 45% compared to the uninoculated control plants after recovery from severe water stress. Impatiens and pansy had an average increase in shoot biomass of 26 and 13%, respectively, when treated with MBSA-MJ1, although the difference was not statistically significant in pansy (Figure 1A). Bacterial application also had a beneficial effect on flower number, but this increase was only statistically significant in petunia. Petunia and impatiens treated with MBSA-MJ1 had an average increase of seven and six flowers per plant, respectively, and no difference was observed in pansy (Figure 1B). Petunia, impatiens, and pansy plants treated with MBSA-MJ1 had a 41, 15, and 41% reduction in the root:shoot, respectively (Figure 1C). At approximately 2 weeks after the drought stressed plants were rewatered, petunia and impatiens treated with MBSA-MJ1 were visibly healthier than control plants. Treated plants had darker green foliage, while the foliage of untreated plants was lighter green with more chlorotic leaves (Figure 2). Visual differences between the treated and control pansies were not as obvious.
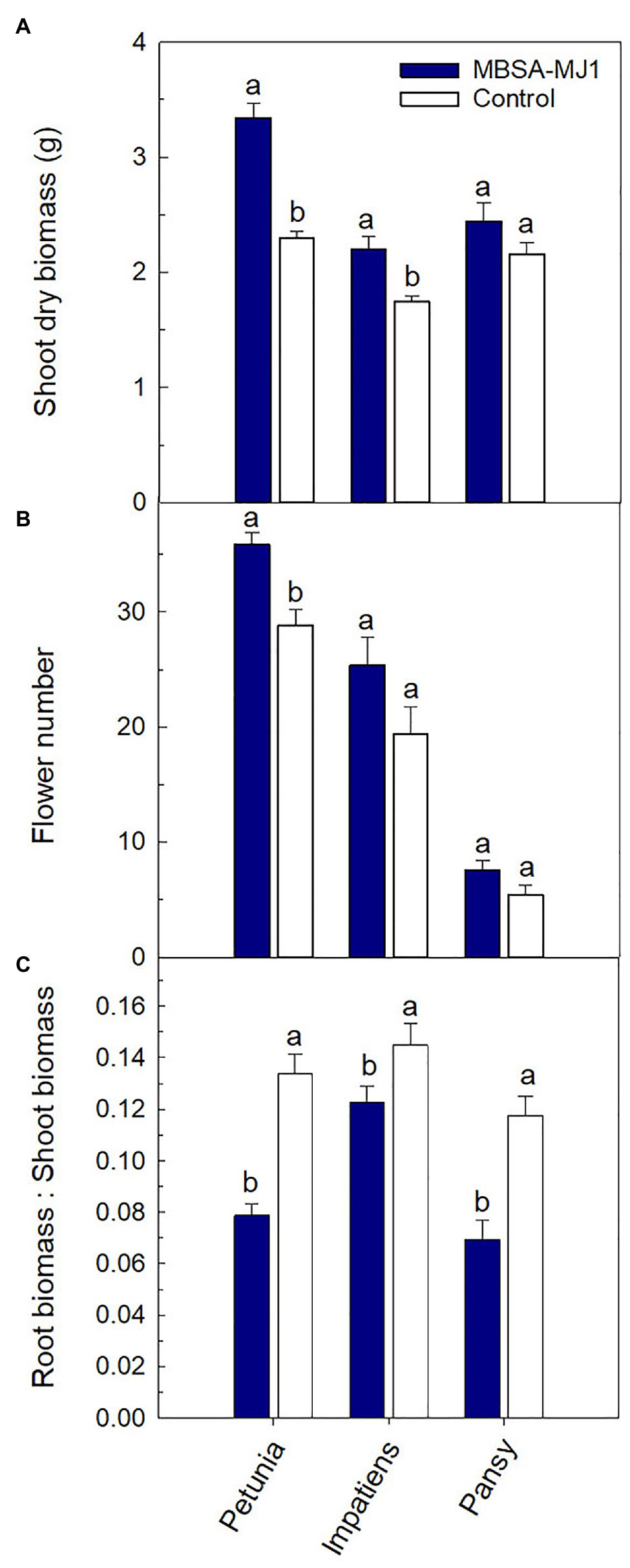
Figure 1. Application of S. plymuthica MBSA-MJ1 improved plant growth performance parameters for Petunia × hybrida (petunia), Impatiens walleriana (impatiens), and Viola × wittrockiana (pansy) plants after recovery from severe water stress. Petunia, impatiens, and pansy plants had 13, 18, and 14 replicates, respectively. Plants were treated weekly with S. plymuthica MBSA-MJ1 (blue) or uninoculated LB (control; white). Inoculum was prepared by diluting bacteria culture (OD595 = 0.8) or LB media in water 1:100, and 120 ml was applied as a media drench. Plants were grown for 5 weeks and then subjected to severe water stress by withholding all irrigation and bacterial treatment until plants were visibly wilted. Total shoot biomass (dry weight; A) and total number of flowers (B) were measured 2 weeks after recovery from water stress (8 weeks after transplant). Root:shoot (C) was calculated with root and shoot dry weights. Bars represent the mean (± SE), and different letters indicate significant differences between the bacteria treatment and control as determined by the Tukey honest significant difference statistical test.
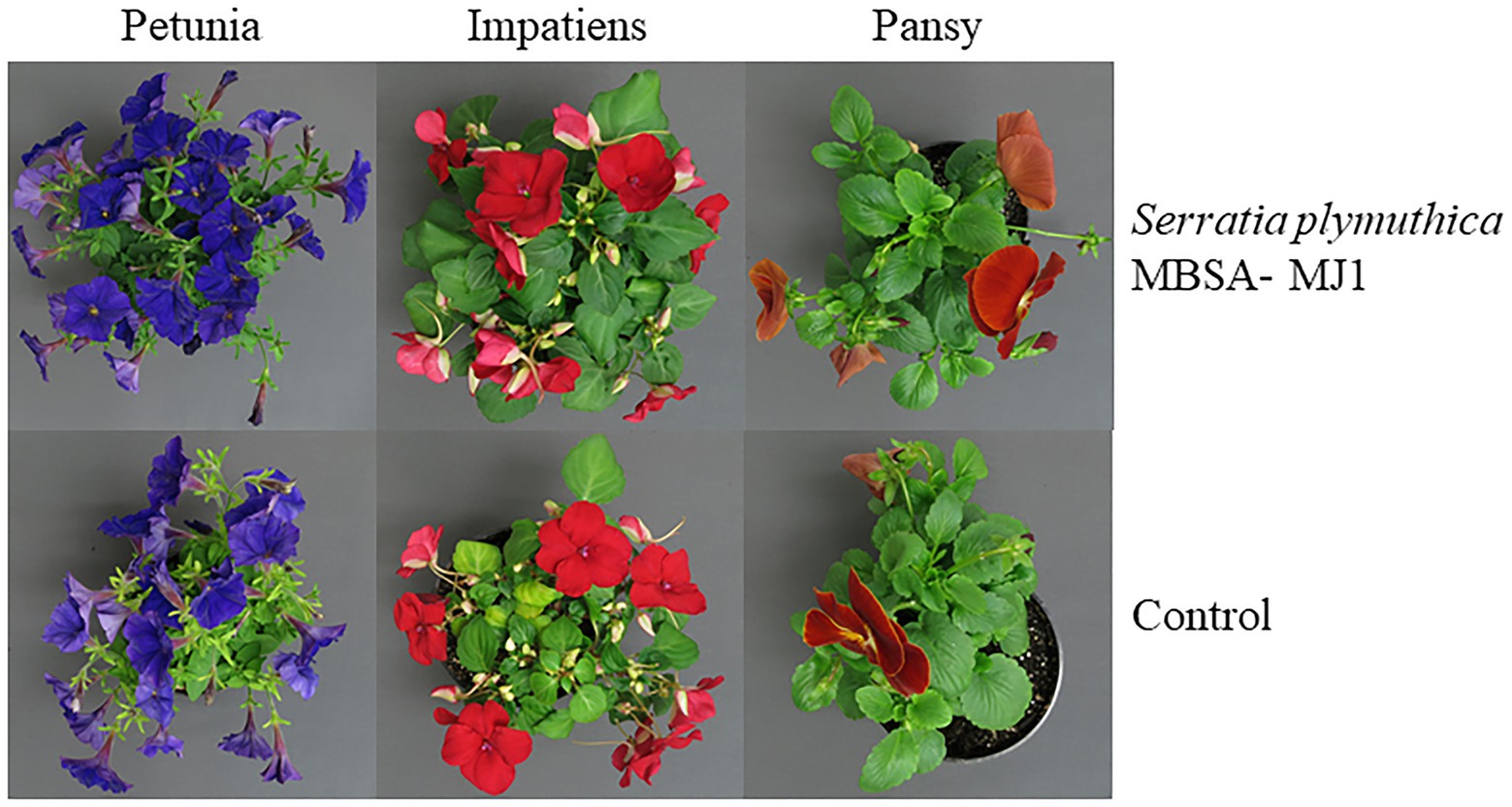
Figure 2. Application of S. plymuthica MBSA-MJ1 increased the visual quality of Petunia × hybrida (petunia) and I. walleriana (impatiens) plants, but not Viola × wittrockiana (pansy) plants, after recovery from water stress. Plants were treated weekly with S. plymuthica MBSA-MJ1 or uninoculated LB (control). Inoculum was prepared by diluting bacteria culture (OD595 = 0.8) or LB media in water 1:100, and 120 ml was applied as a media drench. Plants were grown for 5 weeks and then subjected to severe water stress by withholding all irrigation and bacterial treatment until plants were visibly wilted (approximately 7 days). Irrigation and weekly treatments were then resumed until week 8 when observations of visual quality were evaluated.
In vitro Characterization
Serratia plymuthica MBSA-MJ1 was able to grow under PEG-induced osmotic stress with an average OD595 = 0.45. ACC deaminase activity was not detected in cultures of MBSA-MJ1 (Supplementary Table S1). Antibiotic resistance of MBSA-MJ1 was confirmed by its ability to grow on 1X and 0.5X concentrations of ampicillin, chloramphenicol, and tetracycline, and on 0.5X concentration of carbenicillin. MBSA-MJ1 did not show resistance to rifampicin, streptomycin, kanamycin, or gentamycin. The motile nature of MBSA-MJ1 was demonstrated by its ability to grow away from the inoculation stab in semi-solid agar media.
Genome Assembly and Annotation
De novo assembly and quality filtering of the Illumina reads resulted in 69 contigs with > 1,000 bp length. The draft genome being reported consists of 5,472,087 bases with an average GC content of 56.11%. A total of 5,097 CDSs were identified, with 3,807 (75%) of them being putative protein-coding genes with predicted functions, and 660 identified by SignalP as secreted proteins (Table 1). The KEGG pathway analysis annotated 3,262 genes, 64% of all CDSs, involved in various metabolic pathways. Of the genes assigned to different KEGG functional categories, the largest numbers belonged to the protein families, including genetic information processing (12.7%), signaling and cellular processing (12.1%), and environmental information processing (11.4%). Genes assigned to carbohydrate and amino acid metabolism categories made up 10 and 6.4% of the total gene assignments, respectively (Table 2). No plasmids were identified in the genome sequence data of MBSA-MJ1.
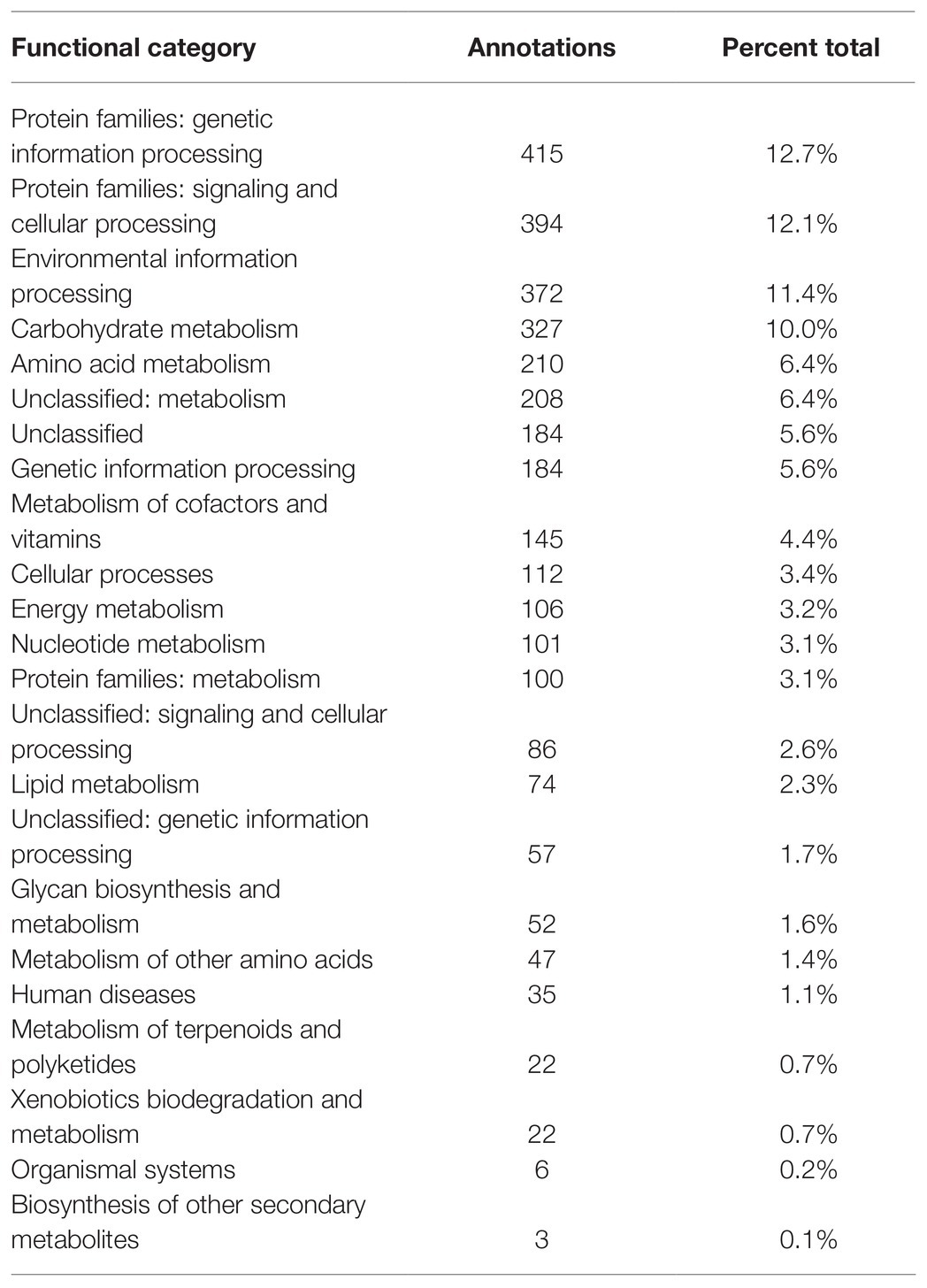
Table 2. Kyoto Encyclopedia of Genes and Genomes (KEGG) functional categories of S. plymuthica MBSA-MJ1.
Taxonomic Classification
Average nucleotide identity results generated by MiGA classify MBSA-MJ1 as S. plymuthica as its sequence shares >94% ANI with 20 different S. plymuthica strains in the NCBI prokaryotic genome database (Supplementary Table S2). In addition, using the ANI matrix results generated by Enveomics, we established five clades within the phylogenetic tree based on distinguishable and high-level ANI similarity between strains (Supplementary Table S3). Serratia plymuthica MBSA-MJ1 is included in clade I, the largest clade in the analysis, with 13 other S. plymuthica strains (Figure 3). Several species were not assigned to clades due to only one strain being included for species outside of S. plymuthica, and a lack of ANI similarity between these other species.
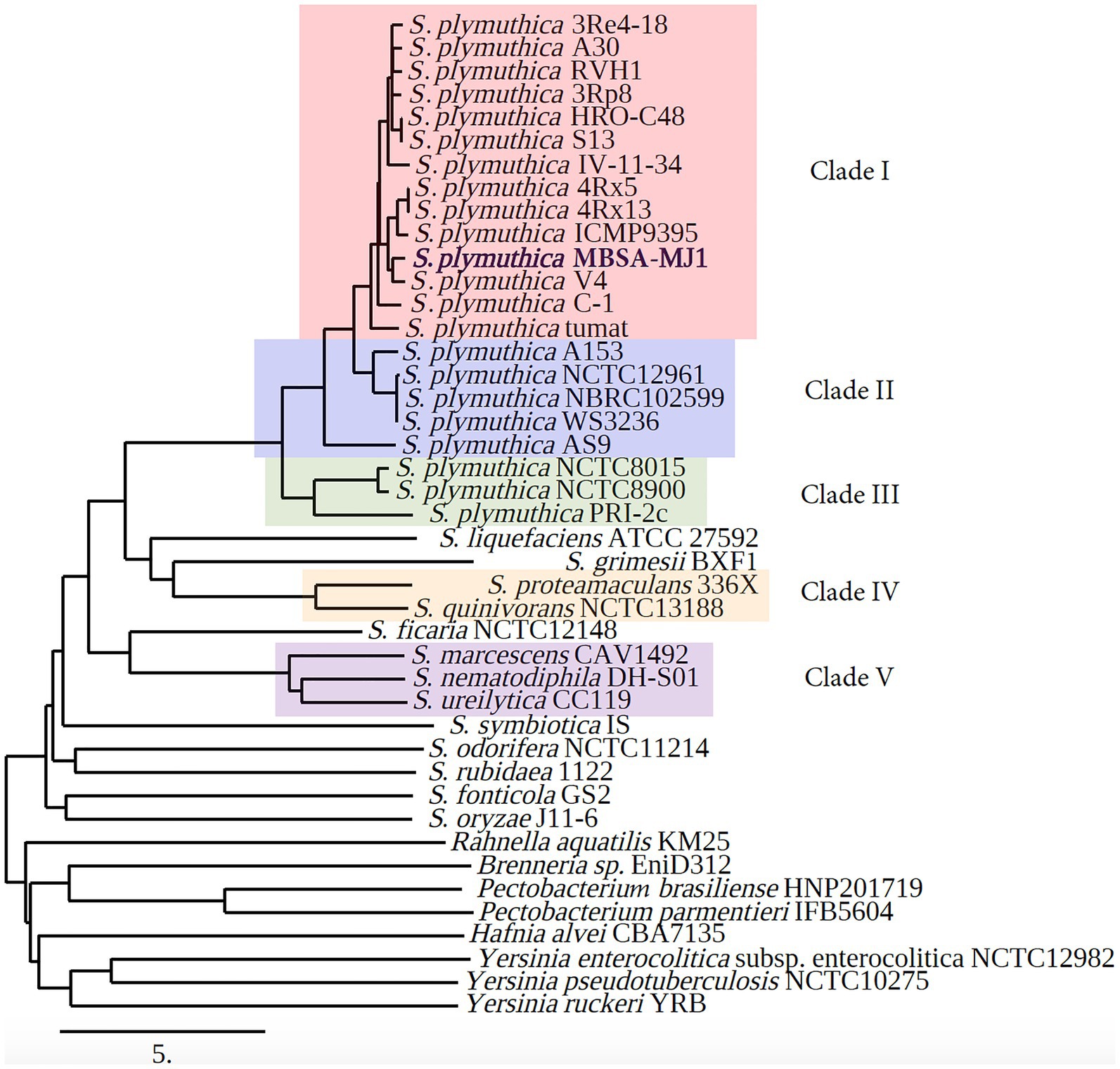
Figure 3. Phylogenetic tree based on average nucleotide identity (ANI) shows that S. plymuthica MBSA-MJ1 groups with other S. plymuthica strains. The ANI was calculated using whole genome sequences downloaded from NCBI and distance was estimated using neighbor-joining as the clustering method with the Enveomics webtool. The ANI data were also used to establish clades within the different phylogenetic clusters. Strains that shared the highest level of ANI similarity were grouped, with each clade consisting of strains that share at least 94% ANI with each other. Strains that do not share at least 94% ANI with any of the strains were not assigned to a clade. The different clades are indicated as Clade I (red), Clade II (blue), Clade III (green), Clade IV (yellow), and Clade V (purple). The NCBI Biosample number for each genome with the ANI statistics is indicated in Supplementary Tables S2 and S3.
Identification of Genes Putatively Involved in Bacterial Osmotic Stress Tolerance
Multiple genes were identified in the genome of S. plymuthica MBSA-MJ1 for the regulation of cellular osmolarity including genes encoding for aquaporin Z (aqpZ), glycerol uptake (glpF), and the biosynthesis of osmoregulated periplasmic glucans (mdoGH and mdoC; Delamarche et al., 1999; Lacroix et al., 1999; Lequette et al., 2004; Liu et al., 2015). The genome of MBSA-MJ1 also encodes for the ABC transporter responsible for internalizing osmoprotectants (proVWX), and six copies of the osmoprotectant uptake protein (proP). Genes comprising the two-component system (TCS) involved in coping with osmotic stress (envZ/ompRC) and its regulator (mzrA), and two cellular envelope stress TCSs (cpxAR and baeSR) were also identified (Supplementary Table S4; Gerken et al., 2009).
The import and accumulation of K+ ions is a common microbial response to osmotic stress (Wood et al., 2001; Wood, 2011). The genome of MBSA-MJ1 encodes for the Kdp potassium uptake system (kdpABCDE), which includes the kdpDE TCS (Kixmüller and Greie, 2012), the K+ uptake proteins (kup and trkH), and the K+ antiporters (chaA and nhaP; Supplementary Table S4; Radchenko et al., 2006; Resch et al., 2011). Na+ extrusion systems also ensure a low sodium content in the cytoplasm while still maintaining osmotic balance during stress (Górecki et al., 2014). Multiple genes involved in sodium transport were identified (nhaA, nhaR, nhaK, nhaB, nhaP, cyrA, and yrbG; Supplementary Table S4; Bremer and Krämer, 2019). In addition to the substrate specific K+ and Na+ transporters, the genome of MBSA-MJ1 also encodes for safety valves for the rapid release of ions and organic solutes in a non-specific manner under sudden osmotic shock (mscL, mscM, and mscS; Supplementary Table S4; Edwards et al., 2012; Bremer and Krämer, 2019).
Identification of Genes Putatively Involved in Oxidative Defense Response
Microbes must be able to overcome plant reactive oxygen species (ROS) defenses to effectively colonize the rhizosphere or endophytically colonize plant tissue. Multiple genes involved in antioxidant production were identified in the genome of S. plymuthica MBSA-MJ1 including: superoxide dismutases (sodA and sodC), ferric uptake regulator (fur), hydrogen peroxide catalases (katA and katG), hydroperoxide reductase (ahpD), thiol peroxidase (tpx), peroxiredoxins (bcp and tsaA), peroxidase (efeB), nitric oxide dioxygenase (hmp), nitric oxide reductases (norV and norW), the transcriptional regulator (norR), nitric oxide sensor (nsrR), and thioredoxins (trxA, trxB, and trxC; Supplementary Table S4; Niederhoffer et al., 1990; Hu et al., 1999; Inaoka et al., 1999; Park et al., 2000; Prieto-Álamo et al., 2000; Zeller and Klug, 2006; Cosgrove et al., 2007; Jangiam et al., 2010; Baptista et al., 2012; Perkins et al., 2015; Zhang et al., 2015; Hong et al., 2019). The genome of MBSA-MJ1 also encodes for multiple genes involved in ROS detoxification systems, including five copies of glutathione S-transferases (gstB), glutathione peroxidases (gpx and btuE), the glutaredoxins operon (grxABCD), and two copies of the glutathione ABC transporter (gsiABCD; Supplementary Table S4; Tamburro et al., 2004; Smirnova and Oktyabrsky, 2005; Arenas et al., 2010; Sánchez-Riego et al., 2013; Johnson and Hug, 2019). Additionally, several key regulators involved in controlling oxidative stresses, such as the stress response sigma factor (rpoS) and its regulator (rssB), hydrogen peroxide sensor (oxyR), and the organic hydroperoxide resistance proteins (ohrR and ohrB) were also identified (Supplementary Table S4; Zuber, 2009; Battesti et al., 2011; Flores-Cruz and Allen, 2011; Fontenelle et al., 2011).
In addition to encoding for genes involved in ROS defense protection, the genome of MBSA-MJ1 also encodes for multiple universal stress proteins that confer resistance to oxidative stress (uspABCEG), multiple heat shock proteins (grpE, htpX, hspQ, and hslR), and chaperone proteins (dnaJ, dnaK, groES/EL, clpB, cbpM, and cbpA; Supplementary Table S4; Kornitzer et al., 1991; Squires et al., 1991; Gragerov et al., 1992; Korber et al., 2000; Chae et al., 2004; Shimuta et al., 2004; Nachin et al., 2005; Pepe et al., 2020).
Identification of Genes Putatively Involved in Rhizosphere Colonization
After overcoming the plant’s ROS defense responses, rhizosphere colonization involves different mechanisms which may include flagellar development, quorum sensing, motility, chemotaxis, and biofilm formation (Cole et al., 2017). Flagellar development requires over 50 genes across multiple operons, of which the genome of S. plymuthica MBSA-MJ1 encodes for the entire suite involved in flagellar transcriptional activation, biosynthesis, and construction (flhCD, flgAMN, flgBCDEFGHIJKL, flhBAE, fliAZY, fliDST, fliE, fliFGHIJK, fliMNOPQR, flgMN, flgKL, fliC, fliDST, motAB, cheAW, tar, tsr, and aer; Supplementary Table S4; Chilcott and Hughes, 2000; Macnab, 2003). In addition to flagellar development, three TCSs involved in the regulation of quorum sensing, motility, and chemotaxis (qseCB, envZ/ompR, and rcsCDB) were identified (Supplementary Table S4; Sperandio et al., 2002; Francez-Charlot et al., 2003). The genome of MBSA-MJ1 also encodes for multiple copies each of the recombinases/integrases involved in surface colonization (xerC and xerD), the seven essential lipopolysaccharide transport proteins (lptABCDEFG), cellulose biosynthesis (bcsABZC), and the clpP protein involved in surface adhesion (Supplementary Table S4; O’Toole and Kolter, 1998; Zogaj et al., 2001; Martínez-Granero et al., 2005; Villa et al., 2013; Eida et al., 2020).
Identification of Genes Putatively Involved in Antibiotic Production and Resistance
Genomic analysis identified the presence of the chloramphenicol acetyltransferase (cat) gene, an enzyme that provides resistance to chloramphenicol (Agersø et al., 2019), and six copies of the efflux pump contributing to tetracycline resistance (stp) within the genome of S. plymuthica MBSA-MJ1 (Supplementary Table S4; Ramón-García et al., 2007), corroborating our in vitro findings that MBSA-MJ1 grew on chloramphenicol and tetracycline. In addition to the antibiotics tested in our in vitro experiment, the genome of MBSA-MJ1 encodes for genes implicated in resistance to bicyclomycin (bcr), fosmidomycin (fsr), polymyxin (arnABCD), and β-lactam multi-drug resistance (ampC, ampR, and ampD; Supplementary Table S4; Lindberg et al., 1985; Bentley et al., 1993; Fujisaki et al., 1996; Miryala and Ramaiah, 2019). Several multidrug resistance and export proteins were identified within the genome (emrA, emrB, emrD, emrE, emrKY, mdfA, acrAB-tolC-acrZ, mdtABC, mdtJI, mdtH, mdtK, mdtL, and mdtN; Supplementary Table S4; Nishino et al., 2009). The genome of MBSA-MJ1 also encodes for the enzyme responsible for 4-hydroxybenzoate biosynthesis (ubiC), an antibiotic against plant pathogenic bacteria (Supplementary Table S4; Siebert et al., 1994; Eida et al., 2020).
Identification of Genes Putatively Involved in Increasing Plant Water Stress Tolerance
Plant growth promoting rhizobacteria can increase plant water stress tolerance using multiple mechanisms, including the synthesis of osmoprotectants and vitamins and by modulating phytohormone levels (Ngumbi and Kloepper, 2016). Genes encoding for the synthesis of the osmoprotectant proline from glutamate (proABC), two proline transporters (proU and proP), the synthesis of the osmoprotectant glycine betaine from choline (betAB), and the glycine betaine transport system (opuCB) were identified in the genome of S. plymuthica MBSA-MJ1 (Supplementary Table S4; Bhargava, 2006; Hilger et al., 2009; Kohler et al., 2015; Zaprasis et al., 2015; Scholz et al., 2016). Genes were also identified for the synthesis of putrescine (speA, speB, and speC), a polyamine involved in osmotic and oxidative stress (Supplementary Table S4; Kurihara et al., 2005).
The genome of MBSA-MJ1 encodes for genes involved in the synthesis of the vitamins thiamin, pyridoxine, biotin, pantothenate, and folic acid. De novo synthesis of thiamin requires 12 genes, including biosynthesis of thiazole (thiFSGH, thiI, and dxs), biosynthesis of pyrimidine (thiC), linking thiazole and pyrimidine (thiE), and thiamin kinases (thiD, thiM, thiL, and pdxK; Begley et al., 1999). There are six genes involved in the biosynthesis of the vitamin pyridoxine (epd, pdxA, pdxB, pdxJ, serC, dxs, and pdxH). Additionally, individual operons encoding for the biosynthesis of biotin (bioCHFADB), pantothenate (panBCDE), and folic acid (folEBKPCA) were identified (Supplementary Table S4; Cronan et al., 1982; Guillén-Navarro et al., 2005; Burgess et al., 2009). The gene enocoding for ACC deaminase synthase (acdS) was not identified in the genome of MBSA-MJ1.
Discussion
The gram-negative bacteria S. plymuthica is a well-known PGPR that has been isolated from numerous important agricultural crops, including oilseed rape, wheat, potato, pumpkin, maize, and rice (Åström and Gerhardson, 1988; Gyaneshwar et al., 2001; Berg et al., 2002, 2006; Garbeva et al., 2012; Müller et al., 2013; Neupane, 2013). Although the plant growth promoting effects of S. plymuthica strains have been primarily attributed to controlling biotic stresses by antagonizing pathogens, our work has shown the utility of this species in stimulating plant growth of containerized horticulture crops after recovery from water stress. Here, we demonstrated the ability of S. plymuthica MBSA-MJ1 to increase the shoot size and flower number of multiple plant species after recovery from severe water stress. In addition, we have investigated the genomic characteristics of strain MBSA-MJ1 and connected them to possible biological functions by identifying mechanisms that this novel bacterium might be utilizing to survive, colonize, and stimulate plant growth under water stress conditions.
In general, petunia and impatiens plants treated with MBSA-MJ1 had an increase in shoot growth parameters after recovering from severe water stress when compared to the uninoculated control plants. Both petunia and impatiens were visibly larger with greener leaves (Figure 2), and petunia had significantly more flowers compared to the uninoculated controls (Figure 1). Improvements in these important characteristics will increase overall crop quality and result in plants that are still marketable even after recovery from severe water stress. Each of the three species had a significant reduction in root:shoot biomass, indicating that plants treated with bacteria did not respond to stress with excessive root growth. This is important for containerized production as the energy for plant growth can then be diverted to shoot growth and flower development. Our findings are similar to previous work that showed plants treated with S. plymuthica DT8 and Pseudomonas spp. significantly increased plant biomass while decreasing the root:shoot of plants recovering from water stress (Nordstedt et al., 2020; Zhang et al., 2020).
The overall treatment effect with MBSA-MJ1 was greatest in petunia, while some positive effects were noted in impatiens and less effect was observed in pansy. Previous work evaluating bacterial application on the same plant species subjected to water stress showed a similar trend in flower number where treatment with Pseudomonas spp. increased the flower number in petunia and impatiens, but not pansy (Nordstedt et al., 2020). However, this previous work did show an increase in pansy shoot biomass after recovery from water stress when treated with Pseudomonas poae 29G9 or Pseudomonas fluorescens 90F12-2. This provides evidence that a single bacterial strain may not be effective for broad application in containerized production systems characterized by the production of many different plant species. Continued work is needed to optimize application parameters such as application timing and rate, and the use of a bacterial consortia with different mechanisms of action to have a broader beneficial effect.
The viability of bacterial cells strongly depends on the water content of their surrounding environment, highlighting a challenge that bacteria face in colonizing plant hosts that are subjected to severe water stress (Kappes and Bremer, 1998; Danhorn and Fuqua, 2007). If bacteria are unable to withstand water stress, they lose the ability to positively influence plant health and stress tolerance during this time. Additionally, Vílchez et al. (2016) suggests a correlation between the level of bacterial desiccation tolerance and the level of water stress tolerance they can confer to plants. The in vitro PEG assay provided evidence of MBSA-MJ1s ability to grow under osmotic stress conditions. Comparatively, MBSA-MJ1 showed a higher level of growth under PEG-induced osmotic stress than many other PGPR previously reported to increase plant growth under water stress (Asghar et al., 2015; Nordstedt et al., 2020; Nordstedt and Jones, 2020). In addition, multiple genes were identified within the genome of MBSA-MJ1 responsible for regulating cellular osmolarity that are potentially involved in increasing its ability to grow under osmotic stress.
Two-component systems in bacteria sense and respond to different environmental cues by altering gene expression (Tipton and Rather, 2017). The envZ/ompR TCS plays a vital role in mediating signal transduction in response to osmotic stress and is involved in the uptake of K+ ions (Gerken and Misra, 2010; Yuan et al., 2011). Additionally, the cpxAR TCS is essential for rhizospheric fitness and growth by helping the bacteria to survive under harsh environments such as severe water stress (Yan et al., 2020). MBSA-MJ1 also encodes for mzrA which not only acts as a regulator of the envZ/ompR TCS without altering its ability to receive and respond to environmental signals, but also links envZ/ompR to cpxAR to further support cellular envelope stress responses (Gerken et al., 2009).
The production of ROS, nitric oxide, and phytoalexins in the rhizosphere by plants is a common defense mechanism during the early stages of plant-microbe interactions (Hammond-Kosack and Jones, 1996; Zeidler et al., 2004). Therefore, PGPR must be able to withstand this highly oxidative environment in order to colonize the rhizosphere and ultimately stimulate plant growth and stress tolerance. Accordingly, we identified a large variety of genes in the genome of MBSA-MJ1 that encode for antioxidants, ROS detoxification, stress response factors that play a key role in controlling oxidative stresses, and universal stress proteins that confer resistance to oxidative stress.
Survival in the rhizosphere also depends on the bacterium’s ability to outcompete other organisms. A common mechanism for this is the production or resistance to antibiotics and other antimicrobial compounds (Andrés-Barrao et al., 2017). Our in vitro work demonstrated MBSA-MJ1’s resistance to different antibiotics, and our genomic analyses identified genes involved in resistance to antibiotics not tested in our in vitro work that could be further contributing to MBSA-MJ1s fitness in the rhizosphere. In addition, the production of secreted proteins has been attributed to many Serratia spp. ability to survive in diverse environmental conditions (Petersen and Tisa, 2013), and a large number of genes encoding for secreted proteins were also identified within the genome of MBSA-MJ1.
The ability to move in a controlled manner confers distinct advantages to rhizospheric bacteria, particularly during colonization. Flagellar development plays a vital role in the motility of bacteria especially during times of environmental stress, and it has been established that nonmotile bacteria are severely impaired in colonizing and surviving within the rhizosphere (Lugtenberg et al., 2001; Capdevila et al., 2004; Guo et al., 2020). However, the bacteria flagellar motor is the most complex structure of the bacterial cell, requiring over 50 genes across multiple operons, of which the MBSA-MJ1 genome contains the entire suite of genes (Chilcott and Hughes, 2000; Armitage and Berry, 2020). These findings corroborate the results from the in vitro motility experiment that confirmed MBSA-MJ1’s ability to move spatially in semi-solid agar media. Chemotaxis is another important trait in competitive colonization as it controls the direction of flagellar rotation and movement (De Weert et al., 2002; Danhorn and Fuqua, 2007; Guo et al., 2020), and the three chemotaxis-related TCS identified in the genome of MBSA-MJ1 likely play a fundamental role in this complex function. PGPR that colonize the rhizosphere have also been known to colonize plant tissue as endophytes (Santoyo et al., 2016). Considering that multiple S. plymuthica strains have been previously identified as endophytes (Benhamou et al., 2000; Liu et al., 2011; Müller et al., 2013), coupled with our genetic evidence in MBSA-MJ1 for flagellar development and chemotaxis, and the positive results from the in vitro motility assay, it is probable that MBSA-MJ1 can also colonize plant tissue as an endophyte. However, this is yet to be experimentally proven.
This work has also identified a multitude of putative mechanisms that this PGPR might be utilizing to stimulate plant growth and increase stress tolerance. Similar to bacteria, plants respond to osmotic stress by synthesizing or accumulating compatible solutes, such as trehalose, mannitol, proline, and glycine-betaine, which increase plant stress tolerance and the ability to grow under water stress conditions (Bhargava, 2006). The proABC operon is responsible for synthesizing the osmoprotectant proline, and the proline transporters putP and proY could provide a mechanism for bacterial-synthesized proline to be made available to the plant host (Bhargava, 2006; Hilger et al., 2009; Kohler et al., 2015; Zaprasis et al., 2015). Additionally, MBSA-MJ1 has the potential to synthesize the osmoprotectant glycine-betaine, which is encoded by betAB, and export the compound into the rhizosphere via the betT high-affinity choline transport system (Scholz et al., 2016). The compatible solute carnitine has been shown to improve plant recovery during times of salt stress and has been implicated in modulating the ABA pathway in plants (Charrier et al., 2012). The six copies of the proP carnitine transport system encoded in the genome of MBSA-MJ1 indicate that carnitine might be another compatible solute involved in increasing the water stress tolerance of plants. Further, the presence of genes involved in synthesizing the polyamine putrescine (speABCE) adds to the list of osmoprotectants that might be involved in this beneficial plant microbe interaction (Kurihara et al., 2005).
Bacterial-produced vitamins also play an important role in plant growth and development and stress responses. Therefore, it is beneficial for plants to form symbiotic relationships with rhizospheric bacteria that can synthesize vitamins for them (Palacios et al., 2014). Our genomic analyses identified multiple genes within the genome of MBSA-MJ1 involved in the synthesis of different B-vitamins, a class of bacterial-produced vitamins that has been attributed to plant growth promotion and increased stress tolerance (Deryło and Skorupska, 1993). Thiamine (vitamin B1) functions as an important enzyme cofactor in carbohydrate and amino acid metabolism and has previously been shown to increase the growth of clover (Deryło and Skorupska, 1993; Schyns et al., 2005). Riboflavin (vitamin B2) is required for the production of cofactors involved in cellular metabolism and is required for normal plant growth and development (Bereswill et al., 1999; Deng et al., 2014). In particular, riboflavin has been shown to enhance plant growth by increasing production of photosynthetic pigments, increasing carbon assimilation, and even increasing tolerance to osmotic stress (Palacios et al., 2014). Riboflavin has also been shown to increase water stress tolerance and antioxidant enzyme activity of tobacco (Deng et al., 2014). Pyridoxine (vitamin B6) is required by all living organisms and acts as a potent antioxidant that effectively quenches ROS (Bilski et al., 2000; Palacios et al., 2014). Pyridoxine has been shown to play a role in plant oxidative stress response (Mooney et al., 2009) and has demonstrated potential function in regulating Na+ homeostasis and thus conferring salt-stress tolerance to plants (Shi et al., 2002). Niacin (vitamin B3) is involved in nearly every metabolic pathway in the cell, with its most important function being as cofactor for diverse cellular oxidation–reduction reactions under stress conditions that might lead to increased stress tolerance in plants (Noctor et al., 2006; Palacios et al., 2014). Biotin and pantothenic acid are also involved in carrying out essential metabolic functions in plants, although they have not yet been evaluated for their influence on plant stress tolerance (White et al., 2001; Guillén-Navarro et al., 2005).
Overall, this work has identified S. plymuthica MBSA-MJ1 as an effective plant growth promoter of containerized horticulture crops subjected to severe water stress. Although Serratia spp. have been extensively studied for control of biotic stresses, there is still much work to be done to understand how they increase abiotic stress tolerance in plants. The comprehensive genome analyses conducted in this study have identified potential genes in the genome of MBSA-MJ1 whose protein products may function in pathways involved in MBSA-MJ1’s ability to survive, colonize, and eventually stimulate plant growth under severe water stress. Future work should focus on functional characterization of the genes identified here in order to begin elucidating the mechanisms most prominently involved in the beneficial responses observed in our greenhouse trials. In addition, the formulation of consortia treatments with other bacterial strains identified as PGPR should be considered as a viable solution to achieving broad beneficial effects across different plant species.
Data Availability Statement
The datasets presented in this study can be found in online repositories. The names of the repository/repositories and accession number(s) can be found at: www.ncbi.nlm.nih.gov/, PRJNA669647.
Author Contributions
NN and MJ conceived the experimental design. NN performed the greenhouse production trial, in vitro assays including collecting and analyzing the data, and all genomic analyses, and led the writing of the manuscript. MJ acquired funding and resources, served as project administrator, and edited the manuscript. Both the authors contributed to the article and approved the submitted version.
Funding
Salaries and research support were provided in part by State and Federal Funds appropriated to the OARDC, The Ohio State University, Journal Article Number HCS 21-03. This work was financially supported by the American Floral Endowment and the OSU D.C. Kiplinger Floriculture Endowment. Support was also provided by The Ohio State University Distinguished Fellowship, the OARDC Director’s Graduate Associateship, and the Altman Family Scholarship.
Conflict of Interest
The authors declare that the research was conducted in the absence of any commercial or financial relationships that could be construed as a potential conflict of interest.
Acknowledgments
We thank Laura Chapin for assistance with the greenhouse experiments, Jonathan Jacobs and Veronica Roman-Reyna for guidance with genomic analyses, and Kaylee South for help with statistical analysis. We also thank Ball Horticultural Company and Syngenta Flowers for providing seeds for the greenhouse experiment.
Supplementary Material
The Supplementary Material for this article can be found online at: https://www.frontiersin.org/articles/10.3389/fmicb.2021.653556/full#supplementary-material
References
Adam, E., Müller, H., Erlacher, A., and Berg, G. (2016). Complete genome sequences of the Serratia plymuthica strains 3Rp8 and 3Re4-18, two rhizosphere bacteria with antagonistic activity towards fungal phytopathogens and plant growth promoting abilities. Stand. Genomic Sci. 11:61. doi: 10.1186/s40793-016-0185-3
Agersø, Y., Bjerre, K., Brockmann, E., Johansen, E., Nielsen, B., Siezen, R., et al. (2019). Putative antibiotic resistance genes present in extant Bacillus licheniformis and Bacillus paralicheniformis strains are probably intrinsic and part of the ancient resistome. PLoS One 14:e0210363. doi: 10.1371/journal.pone.0210363
Almagro Armenteros, J. J., Tsirigos, K. D., Sønderby, C. K., Petersen, T. N., Winther, O., Brunak, S., et al. (2019). SignalP 5.0 improves signal peptide predictions using deep neural networks. Nat. Biotechnol. 37, 420–423. doi: 10.1038/s41587-019-0036-z
Aly, H., Kamalay, J., Walter, N., Okubara, P., and Taylor, C. (2007). “Characterization of the Pseudomonas genus of bacteria for plant-parasitic nematode control,” in ASM Conferences “Pseudomonas 2007”; 26 August 2007; (Seattle, WA, USA), Abstract 166B, 93.
Andrés-Barrao, C., Lafi, F. F., Alam, I., de Zélicourt, A., Eida, A. A., Bokhari, A., et al. (2017). Complete genome sequence analysis of Enterobacter sp. SA187, a plant multi-stress tolerance promoting endophytic bacterium. Front. Microbiol. 8:2023. doi: 10.3389/fmicb.2017.02023
Anjum, S. A., Ashraf, U., Zohaib, A., Tanveer, M., Naeem, M., Ali, I., et al. (2017). Growth and developmental responses of crop plants under drought stress: a review. Zemdirbyste-Agriculture 104, 267–276. doi: 10.13080/z-a.2017.104.034
Antipov, D., Hartwick, N., Shen, M., Raiko, M., Lapidus, A., and Pevzner, P. A. (2016). PlasmidSPAdes: assembling plasmids from whole genome sequencing data. Bioinformatics 32, 3380–3387. doi: 10.1093/bioinformatics/btw493
Arenas, F. A., Díaz, W. A., Leal, C. A., Pérez-Donoso, J. M., Imlay, J. A., and Vásquez, C. C. (2010). The Escherichia coli btuE gene, encodes a glutathione peroxidase that is induced under oxidative stress conditions. Biochem. Biophys. Res. Commun. 398, 690–694. doi: 10.1016/j.bbrc.2010.07.002
Armitage, J., and Berry, R. (2020). Assembly and dynamics of the bacterial flagellum. Annu. Rev. Microbiol. 74, 181–200. doi: 10.1146/annurev-micro-090816-093411
Armitage, A. M., Vines, H. M., Tu, Z.-P., and Black, C. C. (1983). Water relations and net photosynthesis in hybrid geranium. J. Am. Soc. Hortic. Sci. 108, 310–314.
Asghar, H. N., Zahir, Z. A., Akram, M. A., Ahmad, H. T., and Hussain, M. B. (2015). Isolation and screening of beneficial bacteria to ameliorate drought stress in wheat. Soil Environ. 34, 100–110.
Åström, B., and Gerhardson, B. (1988). Differential reactions of wheat and pea genotypes to root inoculation with growth-affecting rhizosphere bacteria. Plant Soil 109, 263–269. doi: 10.1007/BF02202093
Backer, R., Rokem, J. S., Ilangumaran, G., Lamont, J., Praslickova, D., Ricci, E., et al. (2018). Plant growth-promoting rhizobacteria: context, mechanisms of action, and roadmap to commercialization of biostimulants for sustainable agriculture. Front. Plant Sci. 871:1473. doi: 10.3389/fpls.2018.01473
Baptista, J. M., Justino, M. C., Melo, A. M. P., Teixeira, M., and Saraiva, L. M. (2012). Oxidative stress modulates the nitric oxide defense promoted by Escherichia coli flavorubredoxin. J. Bacteriol. 194, 3611–3617. doi: 10.1128/JB.00140-12
Battesti, A., Majdalani, N., and Gottesman, S. (2011). The RpoS-mediated general stress response in Escherichia coli. Annu. Rev. Microbiol. 65, 189–213. doi: 10.1146/annurev-micro-090110-102946
Begley, T. P., Downs, D. M., Ealick, S. E., McLafferty, F. W., Van Loon, A. P. G. M., Taylor, S., et al. (1999). Thiamin biosynthesis in prokaryotes. Arch. Microbiol. 171, 293–300. doi: 10.1007/s002030050713
Benhamou, N., Gagne, S., Le Quere, D., and Dehbi, L. (2000). Bacterial-mediated induced resistance in cucumber: beneficial effect of the endophytic bacterium Serratia plymuthica on the protection against infection by Pythium ultimum. Phytopathology 90, 45–56. doi: 10.1094/PHYTO.2000.90.1.45
Bentley, J., Hyatt, L. S., Ainley, K., Parish, J. H., Herbert, R. B., and White, G. R. (1993). Cloning and sequence analysis of an Escherichia coli gene conferring bicyclomycin resistance. Gene 127, 117–120. doi: 10.1016/0378-1119(93)90625-D
Bereswill, S., Hinkelmann, S., Kist, M., and Sander, A. (1999). Molecular analysis of riboflavin synthesis genes in Bartonella henselae and use of the ribC gene for differentiation of Bartonella species by PCR. J. Clin. Microbiol. 37, 3159–3166. doi: 10.1128/JCM.37.10.3159-3166.1999
Berg, G. (2000). Diversity of antifungal and plant-associated Serratia plymuthica strains. J. Appl. Microbiol. 88, 952–960. doi: 10.1046/j.1365-2672.2000.01064.x
Berg, G., Opelt, K., Zachow, C., Lottmann, J., Götz, M., Costa, R., et al. (2006). The rhizosphere effect on bacteria antagonistic towards the pathogenic fungus Verticillium differs depending on plant species and site. FEMS Microbiol. Ecol. 56, 250–261. doi: 10.1111/j.1574-6941.2005.00025.x
Berg, G., Roskot, N., Steidle, A., Eberl, L., Zock, A., and Smalla, K. (2002). Plant-dependent genotypic and phenotypic diversity of antagonistic rhizobacteria isolated from different Verticillium host plants. Appl. Environ. Microbiol. 68, 3328–3338. doi: 10.1128/AEM.68.7.3328-3338.2002
Bhargava, S. (2006). Genetically modified cyanobacterium Nostoc muscorum overproducing proline in response to salinity and osmotic stresses. J. Biosci. 31, 265–272. doi: 10.1007/BF02703919
Bilski, P., Li, M. Y., Ehrenshaft, M., Daub, M. E., and Chignell, C. F. (2000). Vitamin B6 (pyridoxine) and its derivatives are efficient singlet oxygen quenchers and potential fungal antioxidants. Photochem. Photobiol. 71, 129–134. doi: 10.1562/0031-8655(2000)071<0129:sipvbp>2.0.co;2
Bremer, E., and Krämer, R. (2019). Responses of microorganisms to osmotic stress. Annu. Rev. Microbiol. 73, 313–334. doi: 10.1146/annurev-micro-020518-115504
Burgess, C. M., Smid, E. J., and van Sinderen, D. (2009). Bacterial vitamin B2, B11 and B12 overproduction: an overview. Int. J. Food Microbiol. 133, 1–7. doi: 10.1016/j.ijfoodmicro.2009.04.012
Capdevila, S., Martínez-Granero, F. M., Sánchez-Contreras, M., Rivilla, R., and Martín, M. (2004). Analysis of Pseudomonas fluorescens F113 genes implicated in flagellar filament synthesis and their role in competitive root colonization. Microbiology 150, 3889–3897. doi: 10.1099/mic.0.27362-0
Chae, C., Sharma, S., Hoskins, J. R., and Wickner, S. (2004). CbpA, a DnaJ homolog, is a DnaK co-chaperone, and its activity is modulated by CbpM. J. Biol. Chem. 279, 33147–33153. doi: 10.1074/jbc.M404862200
Charrier, A., Rippa, S., Yu, A., Nguyen, P. J., Renou, J. P., and Perrin, Y. (2012). The effect of carnitine on Arabidopsis development and recovery in salt stress conditions. Planta 235, 123–135. doi: 10.1007/s00425-011-1499-4
Chevenet, F., Brun, C., Bañuls, A. L., Jacq, B., and Christen, R. (2006). TreeDyn: towards dynamic graphics and annotations for analyses of trees. BMC Bioinformatics 7:439. doi: 10.1186/1471-2105-7-439
Chilcott, G. S., and Hughes, K. T. (2000). Coupling of flagellar gene expression to flagellar assembly in Salmonella enterica serovar Typhimurium and Escherichia coli. Microbiol. Mol. Biol. Rev. 64, 694–708. doi: 10.1128/MMBR.64.4.694-708.2000
Cole, B. J., Feltcher, M. E., Waters, R. J., Wetmore, K. M., Mucyn, T. S., Ryan, E. M., et al. (2017). Genome-wide identification of bacterial plant colonization genes. PLoS Biol. 15:e2002860. doi: 10.1371/journal.pbio.2002860
Cosgrove, K., Coutts, G., Jonsson, I. M., Tarkowski, A., Kokai-Kun, J. F., Mond, J. J., et al. (2007). Catalase (KatA) and alkyl hydroperoxide reductase (AhpC) have compensatory roles in peroxide stress resistance and are required for survival, persistence, and nasal colonization in Staphylococcus aureus. J. Bacteriol. 189, 1025–1035. doi: 10.1128/JB.01524-06
Cronan, J. E., Littel, K. J., and Jackowski, S. (1982). Genetic and biochemical analysis of pantothenate biosynthesis in Escherichia coli and Salmonella typhimurium. J. Bacteriol. 149, 916–922. doi: 10.1128/JB.149.3.916-922.1982
Czajkowski, R., and van der Wolfa, J. M. (2012). Draft genome sequence of the biocontrol strain Serratia plymuthica A30, isolated from rotting potato tuber tissue. J. Bacteriol. 194, 6999–7000. doi: 10.1128/JB.01699-12
Danhorn, T., and Fuqua, C. (2007). Biofilm formation by plant-associated bacteria. Annu. Rev. Microbiol. 61, 401–422. doi: 10.1146/annurev.micro.61.080706.093316
De Vleesschauwer, D., and Höfte, M. (2007). Using Serratia plymuthica to control fungal pathogens of plants. CAB Rev. Perspect. Agric. Vet. Sci. Nutr. Nat. Resour. 2:46. doi: 10.1079/PAVSNNR20072046
De Weert, S., Vermeiren, H., Mulders, I. H. M., Kuiper, I., Hendrickx, N., Bloemberg, G. V., et al. (2002). Flagella-driven chemotaxis towards exudate components is an important trait for tomato root colonization by Pseudomonas fluorescens. Mol. Plant-Microbe Interact. 15, 1173–1180. doi: 10.1094/MPMI.2002.15.11.1173
Delamarche, C., Thomas, D., Rolland, J. P., Froger, A., Gouranton, J., Svelto, M., et al. (1999). Visualization of AqpZ-mediated water permeability in Escherichia coli by cryoelectron microscopy. J. Bacteriol. 181, 4193–4197. doi: 10.1128/JB.181.14.4193-4197.1999
Deng, B., Jin, X., Yang, Y., Lin, Z., and Zhang, Y. (2014). The regulatory role of riboflavin in the drought tolerance of tobacco plants depends on ROS production. Plant Growth Regul. 72, 269–277. doi: 10.1007/s10725-013-9858-8
Deryło, M., and Skorupska, A. (1993). Enhancement of symbiotic nitrogen fixation by vitamin-secreting fluorescent Pseudomonas. Plant Soil 154, 211–217. doi: 10.1007/BF00012526
Edwards, M. D., Black, S., Rasmussen, T., Rasmussen, A., Stokes, N. R., Stephen, T. L., et al. (2012). Characterization of three novel mechanosensitive channel activities in Escherichia coli. Channels 6, 272–281. doi: 10.4161/chan.20998
Eida, A. A., Bougouffa, S., L’Haridon, F., Alam, I., Weisskopf, L., Bajic, V. B., et al. (2020). Genome insights of the plant-growth promoting bacterium Cronobacter muytjensii JZ38 with volatile-mediated antagonistic activity against Phytophthora infestans. Front. Microbiol. 11:369. doi: 10.3389/fmicb.2020.00369
El-Esawi, M. A., Alaraidh, I. A., Alsahli, A. A., Alzahrani, S. M., Ali, H. M., Alayafi, A. A., et al. (2018). Serratia liquefaciens KM4 improves salt stress tolerance in maize by regulating redox potential, ion homeostasis, leaf gas exchange and stress-related gene expression. Int. J. Mol. Sci. 19:3310. doi: 10.3390/ijms19113310
Farooq, M., Wahid, A., Kobayashi, N., Fujita, D., and Basra, S. M. A. (2009). Plant drought stress: effects, mechanisms and management. Agron. Sustain. Dev. 29, 185–212. doi: 10.1051/agro:2008021
Flores-Cruz, Z., and Allen, C. (2011). Necessity of OxyR for the hydrogen peroxide stress response and full virulence in Ralstonia solanacearum. Appl. Environ. Microbiol. 77, 6426–6432. doi: 10.1128/AEM.05813-11
Fontenelle, C., Blanco, C., Arrieta, M., Dufour, V., and Trautwetter, A. (2011). Resistance to organic hydroperoxides requires ohr and ohrR genes in Sinorhizobium meliloti. BMC Microbiol. 11:100. doi: 10.1186/1471-2180-11-100
Francez-Charlot, A., Laugel, B., Van Gemert, A., Dubarry, N., Wiorowski, F., Castanié-Cornet, M. P., et al. (2003). RcsCDB his-asp phosphorelay system negatively regulates the flhDC operon in Escherichia coli. Mol. Microbiol. 49, 823–832. doi: 10.1046/j.1365-2958.2003.03601.x
Fujisaki, S., Ohnuma, S., Horiuchi, T., Takahashi, I., Tsukui, S., Nishimura, Y., et al. (1996). Cloning of a gene from Escherichia coli that confers resistance to fosmidomycin as a consequence of amplification. Gene 175, 83–87. doi: 10.1016/0378-1119(96)00128-X
Garbeva, P., van Elsas, J. D., and de Boer, W. (2012). Draft genome sequence of the antagonistic rhizosphere bacterium Serratia plymuthica strain PRI-2C. J. Bacteriol. 194, 4119–4120. doi: 10.1128/JB.00679-12
Gargallo-Garriga, A., Preece, C., Sardans, J., Oravec, M., Urban, O., and Peñuelas, J. (2018). Root exudate metabolomes change under drought and show limited capacity for recovery. Sci. Rep. 8:12696. doi: 10.1038/s41598-018-30150-0
Gerken, H., Charlson, E. S., Cicirelli, E. M., Kenney, L. J., and Misra, R. (2009). MzrA: A novel modulator of the EnvZ/OmpR two-component regulon. Mol. Microbiol. 72, 1408–1422. doi: 10.1111/j.1365-2958.2009.06728.x
Gerken, H., and Misra, R. (2010). MzrA-EnvZ interactions in the periplasm influence the EnvZ/OmpR two-component regulon. J. Bacteriol. 192, 6271–6278. doi: 10.1128/JB.00855-10
Górecki, K., Hägerhäll, C., and Drakenberg, T. (2014). The Na+ transport in gram-positive bacteria defect in the Mrp antiporter complex measured with 23Na nuclear magnetic resonance. Anal. Biochem. 445, 80–86. doi: 10.1016/j.ab.2013.10.003
Gragerov, A., Nudler, E., Komissarova, N., Gaitanaris, G. A., Gottesman, M. E., and Nikiforov, V. (1992). Cooperation of GroEL/GroES and DnaK/DnaJ heat shock proteins in preventing protein misfolding in Escherichia coli. Proc. Natl. Acad. Sci. U. S. A. 89, 10341–10344. doi: 10.1073/pnas.89.21.10341
Guillén-Navarro, K., Encarnación, S., and Dunn, M. F. (2005). Biotin biosynthesis, transport and utilization in rhizobia. FEMS Microbiol. Lett. 246, 159–165. doi: 10.1016/j.femsle.2005.04.020
Guo, Q., Shi, M., Chen, L., Zhou, J., Zhang, L., Li, Y., et al. (2020). The biocontrol agent Streptomyces pactum increases Pseudomonas koreensis populations in the rhizosphere by enhancing chemotaxis and biofilm formation. Soil Biol. Biochem. 144:107755. doi: 10.1016/j.soilbio.2020.107755
Gyaneshwar, P., James, E. K., Mathan, N., Reddy, P. M., Reinhold-Hurek, B., and Ladha, J. K. (2001). Endophytic colonization of rice by a diazotrophic strain of Serratia marcescens. J. Bacteriol. 183, 2634–2645. doi: 10.1128/JB.183.8.2634-2645.2001
Hammond-Kosack, K. E., and Jones, J. D. G. (1996). Resistance gene-dependent plant defense responses. Plant Cell 8, 1773–1791. doi: 10.1105/tpc.8.10.1773
Hilger, D., Polyhach, Y., Jung, H., and Jeschke, G. (2009). Backbone structure of transmembrane domain IX of the Na+/proline transporter PutP of Escherichia coli. Biophys. J. 96, 217–225. doi: 10.1016/j.bpj.2008.09.030
Hong, E. J., Jeong, H., Lee, D. S., Kim, Y., and Lee, H. S. (2019). The ahpD gene of Corynebacterium glutamicum plays an important role in hydrogen peroxide-induced oxidative stress response. J. Biochem. 165, 197–204. doi: 10.1093/jb/mvy097
Hu, Y. M., Butcher, P. D., Mangan, J. A., Rajandream, M. A., and Coates, A. R. M. (1999). Regulation of hmp gene transcription in Mycobacterium tuberculosis: effects of oxygen limitation and nitrosative and oxidative stress. J. Bacteriol. 181, 3486–3493. doi: 10.1128/JB.181.11.3486-3493.1999
Inaoka, T., Matsumura, Y., and Tsuchido, T. (1999). SodA and manganese are essential for resistance to oxidative stress in growing and sporulating cells of Bacillus subtilis. J. Bacteriol. 181, 1939–1943. doi: 10.1128/JB.181.6.1939-1943.1999
Jangiam, W., Loprasert, S., Smith, D. R., and Tungpradabkul, S. (2010). Burkholderia pseudomallei Rpos regulates OxyR and the katG-dpsA operon under conditions of oxidative stress. Microbiol. Immunol. 54, 389–397. doi: 10.1111/j.1348-0421.2010.00230.x
Johnson, L. A., and Hug, L. A. (2019). Distribution of reactive oxygen species defense mechanisms across domain bacteria. Free Radic. Biol. Med. 140, 93–102. doi: 10.1016/j.freeradbiomed.2019.03.032
Kanehisa, M., Sato, Y., and Morishima, K. (2016). BlastKOALA and GhostKOALA: KEGG tools for functional characterization of genome and metagenome sequences. J. Mol. Biol. 428, 726–731. doi: 10.1016/j.jmb.2015.11.006
Kang, S.-M., Khan, A. L., Waqas, M., You, Y.-H., Hamayun, M., Joo, G.-J., et al. (2015). Gibberellin-producing Serratia nematodiphila PEJ1011 ameliorates low temperature stress in Capsicum annuum L. Eur. J. Soil Biol. 68, 85–93. doi: 10.1016/j.ejsobi.2015.02.005
Kappes, R. M., and Bremer, E. (1998). Response of Bacillus subtilis to high osmolarity: uptake of carnitine, crotonobetaine and γ-butyrobetaine via the ABC transport system OpuC. Microbiology 144, 83–90. doi: 10.1099/00221287-144-1-83
Kixmüller, D., and Greie, J. C. (2012). An ATP-driven potassium pump promotes long-term survival of Halobacterium salinarum within salt crystals. Environ. Microbiol. Rep. 4, 234–241. doi: 10.1111/j.1758-2229.2012.00326.x
Kohler, C., Lourenço, R. F., Bernhardt, J., Albrecht, D., Schüler, J., Hecker, M., et al. (2015). A comprehensive genomic, transcriptomic and proteomic analysis of a hyperosmotic stress sensitive α-proteobacterium. BMC Microbiol. 15:71. doi: 10.1186/s12866-015-0404-x
Konstantinidis, K. T., and Tiedje, J. M. (2005). Genomic insights that advance the species definition for prokaryotes. Proc. Natl. Acad. Sci. U. S. A. 102, 2567–2572. doi: 10.1073/pnas.0409727102
Koo, S. Y., and Cho, K.-S. (2009). Isolation and characterization of a plant growth-promoting rhizobacterium, Serratia sp. SY5. J. Microbiol. Biotechnol. 19, 1431–1438.
Korber, P., Stahl, J. M., Nierhaus, K. H., and Bardwell, J. C. A. (2000). Hsp15: a ribosome-associated heat shock protein. EMBO J. 19, 741–748. doi: 10.1093/emboj/19.4.741
Kornitzer, D., Teff, D., Altuvia, S., and Oppenheim, A. B. (1991). Isolation, characterization, and sequence of an Escherichia coli heat shock gene, htpX. J. Bacteriol. 173, 2944–2953. doi: 10.1128/JB.173.9.2944-2953.1991
Kurihara, S., Oda, S., Kato, K., Kim, H. G., Koyanagi, T., Kumagai, H., et al. (2005). A novel putrescine utilization pathway involves γ-glutamylated intermediates of Escherichia coli K-12. J. Biol. Chem. 280, 4602–4608. doi: 10.1074/jbc.M411114200
Lacroix, J. M., Lanfroy, E., Cogez, V., Lequette, Y., Bohin, A., and Bohin, J. P. (1999). The mdoC gene of Escherichia coli encodes a membrane protein that is required for succinylation of osmoregulated periplasmic glucans. J. Bacteriol. 181, 3626–3631. doi: 10.1128/JB.181.12.3626-3631.1999
Lavania, M., Chauhan, P. S., Chauhan, S. V. S., Singh, H. B., and Nautiyal, C. S. (2006). Induction of plant defense enzymes and phenolics by treatment with plant growth-promoting rhizobacteria Serratia marcescens NBRI1213. Curr. Microbiol. 52, 363–368. doi: 10.1007/s00284-005-5578-2
Lequette, Y., Ödberg-Ferragut, C., Bohin, J. P., and Lacroix, J. M. (2004). Identification of mdoD, an mdoG paralog which encodes a twin-arginine-dependent periplasmic protein that controls osmoregulated periplasmic glucan backbone structures. J. Bacteriol. 186, 3695–3702. doi: 10.1128/JB.186.12.3695-3702.2004
Li, H., Qiu, Y., Yao, T., Ma, Y., Zhang, H., and Yang, X. (2020). Effects of PGPR microbial inoculants on the growth and soil properties of Avena sativa, Medicago sativa, and Cucumis sativus seedlings. Soil Tillage Res. 199:104577. doi: 10.1016/j.still.2020.104577
Liang, G., Liu, J., Zhang, J., and Guo, J. (2020). Effects of drought stress on photosynthetic and physiological parameters of tomato. J. Am. Soc. Hortic. Sci. 145, 12–17. doi: 10.21273/JASHS04725-19
Lindberg, F., Westman, L., and Normark, S. (1985). Regulatory components in Citrobacter freundii ampC β-lactamase induction. Proc. Natl. Acad. Sci. U. S. A. 82, 4620–4624. doi: 10.1073/pnas.82.14.4620
Liu, X., Jia, J., Popat, R., Ortori, C. A., Li, J., Diggle, S. P., et al. (2011). Characterisation of two quorum sensing systems in the endophytic Serratia plymuthica strain G3: differential control of motility and biofilm formation according to life-style. BMC Microbiol. 11:26. doi: 10.1186/1471-2180-11-26
Liu, X. D., Wei, Y., Zhou, X. Y., Pei, X., and Zhang, S. H. (2015). Aspergillus glaucus aquaglyceroporin gene glpF confers high osmosis tolerance in heterologous organisms. Appl. Environ. Microbiol. 81, 6926–6937. doi: 10.1128/AEM.02127-15
Lugtenberg, B. J. J., Dekkers, L., and Bloemberg, G. V. (2001). Molecular determinants of rhizosphere colonization by Pseudomonas. Annu. Rev. Phytopathol. 39, 461–490. doi: 10.1146/annurev.phyto.39.1.461
Lugtenberg, B., and Kamilova, F. (2009). Plant-growth-promoting rhizobacteria. Annu. Rev. Microbiol. 63, 541–556. doi: 10.1146/annurev.micro.62.081307.162918
Macnab, R. M. (2003). How bacteria assemble flagella. Annu. Rev. Microbiol. 57, 77–100. doi: 10.1146/annurev.micro.57.030502.090832
Makhalanyane, T. P., Valverde, A., Gunnigle, E., Frossard, A., Ramond, J. B., and Cowan, D. A. (2015). Microbial ecology of hot desert edaphic systems. FEMS Microbiol. Rev. 39, 203–221. doi: 10.1093/femsre/fuu011
Martínez, O. A., Encina, C., Tomckowiack, C., Droppelmann, F., Jara, R., Maldonado, C., et al. (2018). Serratia strains isolated from the rhizosphere of raulí (Nothofagus alpina) in volcanic soils harbour PGPR mechanisms and promote raulí plantlet growth. J. Soil Sci. Plant Nutr. 18, 804–819. doi: 10.4067/S0718-95162018005002302
Martínez-Granero, F., Capdevila, S., Sánchez-Contreras, M., Martín, M., and Rivilla, R. (2005). Two site-specific recombinases are implicated in phenotypic variation and competitive rhizosphere colonization in Pseudomonas flourescens. Microbiology 151, 975–983. doi: 10.1099/mic.0.27583-0
Matilla, M. A., Drew, A., Udaondo, Z., Krell, T., and Salmond, G. P. C. (2016). Genome sequence of Serratia plymuthica A153, a model rhizobacterium for the investigation of the synthesis and regulation of haterumalides, zeamine, and andrimid. Genome Announc. 4, 2013–2014. doi: 10.1128/genomeA.00373-16
Miryala, S. K., and Ramaiah, S. (2019). Exploring the multi-drug resistance in Escherichia coli O157:H7 by gene interaction network: a systems biology approach. Genomics 111, 958–965. doi: 10.1016/j.ygeno.2018.06.002
Mooney, S., Leuendorf, J. E., Hendrickson, C., and Hellmann, H. (2009). Vitamin B6: a long known compound of surprising complexity. Molecules 14, 329–351. doi: 10.3390/molecules14010329
Müller, H., Fürnkranz, M., Grube, M., and Berg, G. (2013). Genome sequence of Serratia plymuthica strain S13, an endophyte with germination- and plant-growth-promoting activity from the flower of Styrian oil pumpkin. Genome Announc. 1, e00594–e00513. doi: 10.1128/genomeA.00594-13
Nachin, L., Nannmark, U., and Nystro, T. (2005). Differential roles of the universal stress proteins of Escherichia coli in oxidative stress resistance, adhesion, and motility. J. Bacteriol. 187, 6265–6272. doi: 10.1128/JB.187.18.6265-6272.2005
Neupane, S. (2013). Genomics and transcriptomics of plant beneficial Serratia spp. Available at: http://urn.kb.se/resolve?urn=urn:nbn:se:slu:epsilon-e-1478 (Accessed January 08, 2021).
Ngumbi, E., and Kloepper, J. (2016). Bacterial-mediated drought tolerance: current and future prospects. Appl. Soil Ecol. 105, 109–125. doi: 10.1016/j.apsoil.2016.04.009
Niederhoffer, E. C., Naranjo, C. M., Bradley, K. L., and Fee, J. A. (1990). Control of Escherichia coli superoxide dismutase (sodA and sodB) genes by the ferric uptake regulation (fur) locus. J. Bacteriol. 172, 1930–1938. doi: 10.1128/JB.172.4.1930-1938.1990
Nishino, K., Nikaido, E., and Yamaguchi, A. (2009). Regulation and physiological function of multidrug efflux pumps in Escherichia coli and Salmonella. Biochim. Biophys. Acta-Proteins Proteomics 1794, 834–843. doi: 10.1016/j.bbapap.2009.02.002
Noctor, G., Queval, G., and Gakière, B. (2006). NAD(P) synthesis and pyridine nucleotide cycling in plants and their potential importance in stress conditions. J. Exp. Bot. 57, 1603–1620. doi: 10.1093/jxb/erj202
Nordstedt, N. P., Chapin, L. J., Taylor, C. G., and Jones, M. L. (2020). Identification of Pseudomonas spp. that increase ornamental crop quality during abiotic stress. Front. Plant Sci. 10:1754. doi: 10.3389/fpls.2019.01754
Nordstedt, N. P., and Jones, M. L. (2020). Isolation of rhizosphere bacteria that improve quality and water stress tolerance in greenhouse ornamentals. Front. Plant Sci. 11:826. doi: 10.3389/fpls.2020.00826
O’Toole, G. A., and Kolter, R. (1998). Initiation of biofilm formation in Pseudomonas fluorescens WCS365 proceeds via multiple, convergent signalling pathways: a genetic analysis. Mol. Microbiol. 28, 449–461. doi: 10.1046/j.1365-2958.1998.00797.x
Palacios, O. A., Bashan, Y., and de-Bashan, L. E. (2014). Proven and potential involvement of vitamins in interactions of plants with plant growth-promoting bacteria—An overview. Biol. Fertil. Soils 50, 415–432. doi: 10.1007/s00374-013-0894-3
Pang, Y., Liu, X., Ma, Y., Chernin, L., Berg, G., and Gao, K. (2009). Induction of systemic resistance, root colonisation and biocontrol activities of the rhizospheric strain of Serratia plymuthica are dependent on N-acyl homoserine lactones. Eur. J. Plant Pathol. 124, 261–268. doi: 10.1007/s10658-008-9411-1
Park, S. G., Cha, M. K., Jeong, W., and Kim, I. H. (2000). Distinct physiological functions of thiol peroxidase isoenzymes in Saccharomyces cerevisiae. J. Biol. Chem. 275, 5723–5732. doi: 10.1074/jbc.275.8.5723
Pepe, S., Scarlato, V., and Roncarati, D. (2020). The Helicobacter pylori HspR-modulator CbpA is a multifunctional heat-shock protein. Microorganisms 8:251. doi: 10.3390/microorganisms8020251
Perkins, A., Nelson, K. J., Parsonage, D., Poole, L. B., and Karplus, P. A. (2015). Peroxiredoxins: guardians against oxidative stress and modulators of peroxide signaling. Trends Biochem. Sci. 40, 435–445. doi: 10.1016/j.tibs.2015.05.001
Petersen, L. M., and Tisa, L. S. (2013). Friend or foe? A review of the mechanisms that drive Serratia towards diverse lifestyles. Can. J. Microbiol. 59, 627–640. doi: 10.1139/cjm-2013-0343
Prieto-Álamo, M. J., Jurado, J., Gallardo-Madueño, R., Monje-Casas, F., Holmgren, A., and Pueyo, C. (2000). Transcriptional regulation of glutaredoxin and thioredoxin pathways and related enzymes in response to oxidative stress. J. Biol. Chem. 275, 13398–13405. doi: 10.1074/jbc.275.18.13398
Proença, D. N., Schwab, S., Vidal, M. S., Baldani, J. I., Xavier, G. R., and Morais, P. V. (2019). The nematicide Serratia plymuthica M24T3 colonizes Arabidopsis thaliana, stimulates plant growth, and presents plant beneficial potential. Braz. J. Microbiol. 50, 777–789. doi: 10.1007/s42770-019-00098-y
Radchenko, M. V., Tanaka, K., Waditee, R., Oshimi, S., Matsuzaki, Y., Fukuhara, M., et al. (2006). Potassium/proton antiport system of Escherichia coli. J. Biol. Chem. 281, 19822–19829. doi: 10.1074/jbc.M600333200
Ramón-García, S., Martín, C., De Rossi, E., and Aínsa, J. A. (2007). Contribution of the Rv2333c efflux pump (the Stp protein) from Mycobacterium tuberculosis to intrinsic antibiotic resistance in Mycobacterium bovis BCG. J. Antimicrob. Chemother. 59, 544–547. doi: 10.1093/jac/dkl510
Resch, C. T., Winogrodzki, J. L., Hase, C. C., and Dibrov, P. (2011). Insights into the biochemistry of the ubiquitous NhaP family of cation/H+ antiporters. Biochem. Cell Biol. 89, 130–137. doi: 10.1139/O10-149
Rodriguez-R, L. M., Gunturu, S., Harvey, W. T., Rosselló-Mora, R., Tiedje, J. M., Cole, J. R., et al. (2018). The microbial genomes atlas (MiGA) webserver: taxonomic and gene diversity analysis of Archaea and Bacteria at the whole genome level. Nucleic Acids Res. 46, W282–W288. doi: 10.1093/nar/gky467
Rodriguez-R, L., and Konstantinidis, K. (2016). The enveomics collection: a toolbox for specialized analyses of microbial genomes and metagenomes. PeerJ [Preprint]. doi: 10.7287/peerj.preprints.1900v1
Sánchez-Blanco, M. J., Álvarez, S., Navarro, A., and Bañón, S. (2009). Changes in leaf water relations, gas exchange, growth and flowering quality in potted geranium plants irrigated with different water regimes. J. Plant Physiol. 166, 467–476. doi: 10.1016/j.jplph.2008.06.015
Sánchez-Riego, A. M., López-Maury, L., and Florencio, F. J. (2013). Glutaredoxins are essential for stress adaptation in the cyanobacterium Synechocystis sp. PCC 6803. Front. Plant Sci. 4:428. doi: 10.3389/fpls.2013.00428
Santoyo, G., Moreno-Hagelsieb, G., del Carmen Orozco-Mosqueda, M., and Glick, B. R. (2016). Plant growth-promoting bacterial endophytes. Microbiol. Res. 183, 92–99. doi: 10.1016/j.micres.2015.11.008
Scholz, A., Stahl, J., de Berardinis, V., Müller, V., and Averhoff, B. (2016). Osmotic stress response in Acinetobacter baylyi: identification of a glycine-betaine biosynthesis pathway and regulation of osmoadaptive choline uptake and glycine-betaine synthesis through a choline-responsive BetI repressor. Environ. Microbiol. Rep. 8, 316–322. doi: 10.1111/1758-2229.12382
Schyns, G., Potot, S., Geng, Y., Barbosa, T. M., Henriques, A., and Perkins, J. B. (2005). Isolation and characterization of new thiamine-deregulated mutants of Bacillus subtilis. J. Bacteriol. 187, 8127–8136. doi: 10.1128/JB.187.23.8127-8136.2005
Seemann, T. (2014). Prokka: rapid prokaryotic genome annotation. Bioinformatics 30, 2068–2069. doi: 10.1093/bioinformatics/btu153
Shah, S., Li, J., Moffatt, B. A., and Glick, B. R. (1998). Isolation and characterization of ACC deaminase genes from two different plant growth-promoting rhizobacteria. Can. J. Microbiol. 44, 833–843. doi: 10.1139/w98-074
Shi, H., Xiong, L., Stevenson, B., Lu, T., and Zhu, J. K. (2002). The Arabidopsis salt overly sensitive 4 mutants uncover a critical role for vitamin B6 in plant salt tolerance. Plant Cell 14, 575–588. doi: 10.1105/tpc.010417
Shimuta, T. R., Nakano, K., Yamaguchi, Y., Ozaki, S., Fujimitsu, K., Matsunaga, C., et al. (2004). Novel heat shock protein HspQ stimulates the degradation of mutant DnaA protein in Escherichia coli. Genes Cells 9, 1151–1166. doi: 10.1111/j.1365-2443.2004.00800.x
Siebert, M., Severin, K., and Heide, L. (1994). Formation of 4-hydroxybenzoate in Escherichia coli: characterization of the ubiC gene and its encoded enzyme chorismate pyruvate-lyase. Microbiology 140, 897–904. doi: 10.1099/00221287-140-4-897
Smirnova, G. V., and Oktyabrsky, O. N. (2005). Glutathione in bacteria. Biokhimiya 70, 1459–1473. doi: 10.1007/s10541-005-0248-3
South, K. A., Hand, F. P., and Jones, M. L. (2020). Beneficial bacteria identified for the control of Botrytis cinerea in petunia greenhouse production. Plant Dis. 104, 1801–1810. doi: 10.1094/PDIS-10-19-2276-RE
Sperandio, V., Torres, A. G., and Kaper, J. B. (2002). Quorum sensing Escherichia coli regulators B and C (QseBC): a novel two-component regulatory system involved in the regulation of flagella and motility by quorum sensing in E. coli. Mol. Microbiol. 43, 809–821. doi: 10.1046/j.1365-2958.2002.02803.x
Squires, C. L., Pedersen, S., Ross, B. M., and Squires, C. (1991). ClpB is the Escherichia coli heat shock protein F84.1. J. Bacteriol. 173, 4254–4262. doi: 10.1128/JB.173.14.4254-4262.1991
Sun, W., Dunning, F. M., Pfund, C., Weingarten, R., and Bent, A. F. (2006). Within-species flagellin polymorphism in Xanthomonas campestris pv campestris and its impact on elicitation of Arabidopsis FLAGELLIN SENSING2-dependent defenses. Plant Cell 18, 764–779. doi: 10.1105/tpc.105.037648
Sun, Y., Wang, C., Chen, H. Y. H., and Ruan, H. (2020). Response of plants to water stress: a meta-analysis. Front. Plant Sci. 11:978. doi: 10.3389/fpls.2020.00978
Tamburro, A., Robuffo, I., Heipieper, H. J., Allocati, N., Rotilio, D., Di Ilio, C., et al. (2004). Expression of glutathione S-transferase and peptide methionine sulphoxide reductase in Ochrobactrum anthropi is correlated to the production of reactive oxygen species caused by aromatic substrates. FEMS Microbiol. Lett. 241, 151–156. doi: 10.1016/j.femsle.2004.10.013
Tipton, K. A., and Rather, P. N. (2017). An ompR-envZ two-component system ortholog regulates phase variation, osmotic tolerance, motility, and virulence in Acinetobacter baumannii strain AB5075. J. Bacteriol. 199, e00705–e00716. doi: 10.1128/JB.00705-16
van der Lelie, D., Taghavi, S., Monchy, S., Schwender, J., Miller, L., Ferrieri, R., et al. (2009). Poplar and its bacterial endophytes: coexistence and harmony. CRC. Crit. Rev. Plant Sci. 28, 346–358. doi: 10.1080/07352680903241204
Vílchez, J. I., García-Fontana, C., Román-Naranjo, D., González-López, J., and Manzanera, M. (2016). Plant drought tolerance enhancement by trehalose production of desiccation-tolerant microorganisms. Front. Microbiol. 7:1577. doi: 10.3389/fmicb.2016.01577
Villa, R., Martorana, A. M., Okuda, S., Gourlay, L. J., Nardini, M., Sperandeo, P., et al. (2013). The Escherichia coli lpt transenvelope protein complex for lipopolysaccharide export is assembled via conserved structurally homologous domains. J. Bacteriol. 195, 1100–1108. doi: 10.1128/JB.02057-12
Vurukonda, S. S. K. P., Vardharajula, S., Shrivastava, M., and SkZ, A. (2016). Enhancement of drought stress tolerance in crops by plant growth promoting rhizobacteria. Microbiol. Res. 184, 13–24. doi: 10.1016/j.micres.2015.12.003
Wang, S., Ouyang, L., Ju, X., Zhang, L., Zhang, Q., and Li, Y. (2014). Survey of plant drought-resistance promoting bacteria from Populus euphratica tree living in arid area. Indian J. Microbiol. 54, 419–426. doi: 10.1007/s12088-014-0479-3
White, W. H., Gunyuzlu, P. L., and Toyn, J. H. (2001). Saccharomyces cerevisiae is capable of de novo pantothenic acid biosynthesis involving a novel pathway of β-alanine production from spermine. J. Biol. Chem. 276, 10794–10800. doi: 10.1074/jbc.M009804200
Wood, J. M. (2011). Bacterial osmoregulation: a paradigm for the study of cellular homeostasis. Annu. Rev. Microbiol. 65, 215–238. doi: 10.1146/annurev-micro-090110-102815
Wood, J. M., Bremer, E., Csonka, L. N., Kraemer, R., Poolman, B., Van der Heide, T., et al. (2001). Osmosensing and osmoregulatory compatible solute accumulation by bacteria. Comp. Biochem. Physiol. A Mol. Integr. Physiol. 130, 437–460. doi: 10.1016/S1095-6433(01)00442-1
Yan, K., Liu, T., Duan, B., Liu, F., Cao, M., Peng, W., et al. (2020). The CpxAR two-component system contributes to growth, stress resistance, and virulence of Actinobacillus pleuropneumoniae by upregulating wecA transcription. Front. Microbiol. 11:1026. doi: 10.3389/fmicb.2020.01026
Yuan, J., Wei, B., Shi, M., and Gao, H. (2011). Functional assessment of EnvZ/OmpR two-component system in Shewanella oneidensis. PLoS One 6:e23701. doi: 10.1371/journal.pone.0023701
Zaprasis, A., Bleisteiner, M., Kerres, A., Hoffmann, T., and Bremer, E. (2015). Uptake of amino acids and their metabolic conversion into the compatible solute proline confers osmoprotection to Bacillus subtilis. Appl. Environ. Microbiol. 81, 250–259. doi: 10.1128/AEM.02797-14
Zargar, S. M., Gupta, N., Nazir, M., Mahajan, R., Malik, F. A., Sofi, N. R., et al. (2017). Impact of drought on photosynthesis: molecular perspective. Plant Gene 11, 154–159. doi: 10.1016/j.plgene.2017.04.003
Zeidler, D., Zähringer, U., Gerber, I., Dubery, I., Hartung, T., Bors, W., et al. (2004). Innate immunity in Arabidopsis thaliana: lipopolysaccharides activate nitric oxide synthase (NOS) and induce defense genes. Proc. Natl. Acad. Sci. U. S. A. 101, 15811–15816. doi: 10.1073/pnas.0404536101
Zeller, T., and Klug, G. (2006). Thioredoxins in bacteria: functions in oxidative stress response and regulation of thioredoxin genes. Naturwissenschaften 93, 259–266. doi: 10.1007/s00114-006-0106-1
Zhang, F., Dashti, N., Hynes, R. K., and Smith, D. L. (1997). Plant growth-promoting rhizobacteria and soybean [Glycine max (L.) Merr.] growth and physiology at suboptimal root zone temperatures. Ann. Bot. 79, 243–249. doi: 10.1006/anbo.1996.0332
Zhang, C., Xin, Y., Wang, Y., Guo, T., Lu, S., and Kong, J. (2015). Identification of a novel dye-decolorizing peroxidase, efeB, translocated by a twin-arginine translocation system in Streptococcus thermophilus CGMCC 7.179. Appl. Environ. Microbiol. 81, 6108–6119. doi: 10.1128/AEM.01300-15
Zhang, M., Yang, L., Hao, R., Bai, X., Wang, Y., and Yu, X. (2020). Drought-tolerant plant growth-promoting rhizobacteria isolated from jujube (Ziziphus jujuba) and their potential to enhance drought tolerance. Plant Soil 452, 423–440. doi: 10.1007/s11104-020-04582-5
Zogaj, X., Nimtz, M., Rohde, M., Bokranz, W., and Römling, U. (2001). The multicellular morphotypes of Salmonella typhimurium and Escherichia coli produce cellulose as the second component of the extracellular matrix. Mol. Microbiol. 39, 1452–1463. doi: 10.1046/j.1365-2958.2001.02337.x
Keywords: drought, floriculture, horticulture, osmoprotectants, plant growth promoting rhizobacteria, plant-microbe interaction, vitamins, whole-genome sequence
Citation: Nordstedt NP and Jones ML (2021) Genomic Analysis of Serratia plymuthica MBSA-MJ1: A Plant Growth Promoting Rhizobacteria That Improves Water Stress Tolerance in Greenhouse Ornamentals. Front. Microbiol. 12:653556. doi: 10.3389/fmicb.2021.653556
Edited by:
Aleksa Obradović, University of Belgrade, SerbiaReviewed by:
Shabir Hussain Wani, Sher-e-Kashmir University of Agricultural Sciences and Technology, IndiaClaudia S. L. Vicente, University of Évora, Portugal
Copyright © 2021 Nordstedt and Jones. This is an open-access article distributed under the terms of the Creative Commons Attribution License (CC BY). The use, distribution or reproduction in other forums is permitted, provided the original author(s) and the copyright owner(s) are credited and that the original publication in this journal is cited, in accordance with accepted academic practice. No use, distribution or reproduction is permitted which does not comply with these terms.
*Correspondence: Michelle L. Jones, am9uZXMuMTk2OEBvc3UuZWR1