- 1METfilter S.L., Seville, Spain
- 2IMDEA Water Institute, Parque Científico Tecnológico, Madrid, Spain
- 3Foundation Centre for New Water Technologies (CENTA), Seville, Spain
- 4Department of Analytical Chemistry and Chemical Engineering, University of Alcalá, Madrid, Spain
Conventional wastewater treatment technologies are costly and energy demanding; such issues are especially remarkable when small communities have to clean up their pollutants. In response to these requirements, a new variety of nature-based solution, so-called METland®, has been recently develop by using concepts from Microbial Electrochemical Technologies (MET) to outperform classical constructed wetland regarding wastewater treatment. Thus, the current study evaluates two operation modes (aerobic and aerobic–anoxic) of a full-scale METland®, including a Life Cycle Assessment (LCA) conducted under a Net Environmental Balance perspective. Moreover, a combined technical and environmental analysis using a Net Eutrophication Balance (NEuB) focus concluded that the downflow (aerobic) mode achieved the highest removal rates for both organic pollutant and nitrogen, and it was revealed as the most environmentally friendly design. Actually, aerobic configuration outperformed anaero/aero-mixed mode in a fold-range from 9 to 30%. LCA was indeed recalculated under diverse Functional Units (FU) to determine the influence of each FU in the impacts. Furthermore, in comparison with constructed wetland, METland® showed a remarkable increase in wastewater treatment capacity per surface area (0.6 m2/pe) without using external energy. Specifically, these results suggest that aerobic–anoxic configuration could be more environmentally friendly under specific situations where high N removal is required. The removal rates achieved demonstrated a robust adaptation to influent variations, revealing a removal average of 92% of Biology Oxygen Demand (BOD), 90% of Total Suspended Solids (TSS), 40% of total nitrogen (TN), and 30% of total phosphorus (TP). Moreover, regarding the global warming category, the overall impact was 75% lower compared to other conventional treatments like activated sludge. In conclusion, the LCA revealed that METland® appears as ideal solution for rural areas, considering the low energy requirements and high efficiency to remove organic pollutants, nitrogen, and phosphates from urban wastewater.
Introduction
Nowadays, one of the main environmental problems is water scarcity and ecological degradation of the water body status (UNESCO, 2018). Indeed, it was estimated that by 2015, still 2.3 billion people in the world would not have access to basic sanitation facilities (UNICEF and WHO, 2017). Although Wastewater Treatment (WWT) systems have improved in recent years, the optimization and implementation in all the populations remain as a high priority. However, small communities and isolated houses have no access to well-functioning sanitation infrastructure due to limited technical and economic resources. It is therefore proposed that WWT should be low-cost technologies with simple operation and maintenance (Mahmood et al., 2013). In order to protect the environment in these situations, a number of decentralized technologies, so-called Nature Based Solutions (NBS), have been developed based on eco-efficiency designs with low operational expenses (constructed wetlands, stabilization ponds, and sand filters, etc.) (Massoud et al., 2009).
In this eco-design context, METland® is an innovative nature-based solution that merges Microbial Electrochemical Technologies (MET) with Constructed Wetlands (CW). The main constructive difference resides in replacing the classical biofiltering material (gravel, sand) of CWs by electroconductive (EC) granular material. This EC material allows the electrons to circulate through the material, avoiding the classical electron acceptor limitation from anoxic environments, so METland® operates maximizing the electron transfer between the EC material and the electroactive bacteria (Rotaru et al., 2021). Thus, METland® is a term to denominate such general concept and it does not imply any specific operation mode. So, such systems can be operated either under flooded and anoxic mode (Aguirre-Sierra et al., 2016) or under downflow aerobic one (Aguirre-Sierra et al., 2020). Interestingly, bacteria from Geobacter genus were abundant as part of the electroactive biofilm regardless of the operation, anaerobic or aerobic (Aguirre-Sierra et al., 2016, 2020). Probably the most relevant consequence of stimulating the electroactive microbial communities from METland® was a vast enhancement of biodegradation rates and consequently a feasible reduction of the footprint requirements (Wang et al., 2020). Moreover, this technology is a suitable on-site solution for the treatment of WW, including micropollutants (Pun et al., 2019), with the clear advantage of no energy consumption or sludge generation (Ramírez-Vargas et al., 2019). A deep exploration of materials and design was carried out in the frame of the iMETland project1, which aimed to implement such innovative solution for cleaning up WW from two small communities (200 p.e.) at Spain and Denmark with a ratio of 0.4 m2/pe. The availability of materials for constructing such MET-based solutions can be a drawback for reaching a global implementation; however, recent studies have explored such issues through a circular economy approach to reveal how the physicochemical properties of carbonaceous material (e.g., EC coke and EC biochar) correlates with the biodegradation of pollutant (Prado et al., 2019). Regarding innovative designs, the use of the so-called e-sinks devices was proved to effectively control the electron flow inside the EC bed for enhancing removal rates of pollutants (Prado et al., 2020). Prediction tools for finding suitable locations to implement METland® have been recently developed through a methodology based on Multi-Criteria Evaluation (MCE) techniques and Geographical Information Systems (GIS) (Peñacoba-Antona et al., 2021). The interest and potential of the solution seems clear but, in the context of a Circular Economy transition, the potential environmental impact of a new technology like METland® should be evaluated to prevent impacts from the design (EEA, 2019).
The Life Cycle Assessment (LCA) methodology is a standardized methodology to quantify the environmental impact associated with a system or product (ISO 14040, 2006; ISO 14044, 2006). LCA allows the introduction of different life-cycle stages from the building to the demolition phase, and enables better decision making due to the inclusion of the quantification of the effects of the entire system under study (Lundin et al., 2000). Therefore, LCA fits as a decision-making tool for technological environmental assessment under the Circular Economy framework (Zhao et al., 2019).
Among all review publications regarding LCA applied to WWT technologies, Corominas et al. (2013) is probably one of the most extensive and complete study covering such topic. This review analyzed the variability and the lack of consensus in the methodological choices within the LCA studies, including phases, scope and goal definition, as well as the boundary selection. Highlighting the necessity to develop standardized guidelines for WWT more detailed than ISO 14040 and ISO 14044 (2006) and Corominas et al. (2013) pointed out the selection of the Functional Unit (FU) as one of the most critical points. Furthermore, this fact has been also mentioned in other reviews, indicating the difficulties to compare the LCA studies because of the discordance in the FU (Zang et al., 2015) or the vague definition (Gallego-Schmid and Tarpani, 2019). Other criticisms associated with the selection of the FU were made in relation to the lack of representativeness, for example, 1 m3 of wastewater (it does not include the effectiveness) (Godin et al., 2012; Corominas et al., 2013; Lorenzo-Toja et al., 2018), population equivalent (country-based differences), grams of phosphorus (organic material not included), or COD equivalent (non-C-based pollutants not considered) (Zhu et al., 2013; Niero et al., 2014). Aside from that, there is a main tendency in WWTs to consider a volumetric FU. Indeed, there is a general consensus to consider the treatment of 1 m3 of WW as FU (Corominas et al., 2013; Gallego-Schmid and Tarpani, 2019).
Focusing on the environmental impact of decentralized systems, some LCAs were performed comparing alternative WWT processes for small and rural communities (Machado et al., 2007; De Feo and Ferrara, 2017; Arashiro et al., 2018). These studies concluded that nature-based solutions like CWs had a lower impact compared with conventional systems like aeration activated sludge due to the high energy consumption of the last (Garfí et al., 2017). Otherwise, previous LCA studies on CWs pointed out the capacity of the system to couple with different flow mode and loadings rates with low energy and no chemical requirements (Lutterbeck et al., 2017; Flores et al., 2019). Additionally, some alternatives to the conventional CW had included artificial aeration (Resende et al., 2019). An LCA of a variety of CW integrating single electrodes for harvesting energy was studied by Corbella et al. (2017), revealing lower environmental impact than a conventional CW with a notably reduction of volume but a higher construction cost.
The objective of this study was the techno-environmental comparison of two different conceptual designs of METland operating at full scale with real urban wastewater, including a multifunctional unit (MFU) study performed to increase the accuracy of future decisions.
Materials and Methods
Designs and Operational Description
A METland® unit was built in the facilities of Foundation Centre for New Water Technologies (CENTA) for testing the technology with real WW (Figure 1). This METland® unit was operated in two different configurations. Firstly, design 1 (D1) was constructed with the following vessel dimensions: 6.5 m length, 3.7 m width, and 1.2 m depth within a surface area of 24 m2. The bed material was divided into three layers with different thicknesses and materials (Figure 2).
i. A bottom layer (0.3 m of river gravel) to create a volume of rounded material for conducting the water to the drainage system as well as incorporate the pipes.
ii. An intermediate layer (0.5 m of EC material) to generate a favorable environment for the growth of bacterial communities, specially EC species from Geobacter genus, previously reported (Aguirre-Sierra et al., 2016).
iii. A top layer (0.1 m of gravel) to isolate the system from direct sun radiation. In addition, it attenuates the temperature variations inside the system.
Secondly, the previous design was modified into a second design (D2). Constructed differences were strictly based on the increasing of thickness of the intermediate layer (from 0.5 m to 0.8 m) of the conductive material. The characteristics of METland® designs (D1 and D2) are shown in Figure 2.
On top of the differences in design, each configuration kept its own operational mode. In D1, the water level reached 60 cm by flooding completely the bottom layer and partially the intermediate-conductive layer. Thus, D1 was partly operated under aerobic and anaerobic conditions. Contrarily, D2 operated strictly under aerobic conditions by percolating WW pulses through the layers till finally water was collected through the drainage system.
Both designs were operated under different COD loading rates and flow rates according to Table 1. D1 was assayed in two independent and consecutive periods with different organic loading rate (Biochemical Oxygen Demand, g BOD5 m–3): first, a medium loading rate period (P1), followed by a high loading rate one (P2). Moreover, D2 was assayed in three periods with increasing loading rate (P3, P4, and P5, respectively).
Evaluation of METland® Performance
The system was fed under discontinuous flow mode with urban WW (post-primary treatment) from the municipality of Carrión de los Céspedes (2,500 inhabitants in 2018; Seville, Spain). METland performance was characterized by weekly sampling from both influent and effluent of the system. The analysis was performed following the standard methods for Total Suspended Solids (TSS), Biochemical Oxygen Demand (BOD5), Chemical Oxygen Demand (COD), Total Nitrogen (TN), and Total Phosphorus (TP) (American Public Health Association, 2005). The loading rates and the removal efficiency were obtained using the weighted average for the calculation of the influent and removal rates through the different periods. The loading rates were calculated for BOD5, TSS, N, and P fed in the METland® with respect to a volumetric unit of bed and day. Such parameter considers the variations in flow mode and bed volume during the different periods, allowing to normalize results. Thus, a two-way analysis of variance was conducted to test the data statistical significance, integrating the effect of influent fluctuations within removals in each design (two-way ANOVA). The comparison among means was tested using R (R Core Team, 2018) with a significance level of p-value < 0.05 (95% confidence).
In relation to the effluent concentration of pollutants, the European Union establishes a limit for being able to discharge the water into the environment (EEC, 1991). Particularly, for small agglomerations, the Council Directive 91/271/EEC imposes a limit for the main parameters of the quality of water (BOD5, COD, TN, TP, and TSS). Furthermore, a correlation was performed between those parameters (influent concentration (I), effluent concentration (O), and effectiveness (E-in percentage of removal)) using R (R Core Team, 2018).
Life Cycle Assessment (LCA)
The full analysis for the selection of the best design should include the environmental impacts associated with the technology. An LCA was performed as an environmental management technique developed to address these impacts. The LCA methodology was applied following the four phases described in ISO 14040 and ISO 14044 (2006): (i) the goal and scope definition, (ii) the inventory analysis, (iii) the impact assessment, and (iv) the interpretation. Additionally, the study was developed in accordance to the International Reference Life Cycle Data System (ILCD) technical guidance (JCR, 2010).
Goal and Scope Definition
The goal of the present study was to select the most environmentally friendly design among two independent METland® configurations. An attributional LCA study was performed along the construction and operation phases, including the monitoring. Coherently with the effectiveness analysis, the scenarios in the LCA compared two designs: D1 and D2, as well as the differences or particularities within several operation periods: P1, P2, P3, P4, and P5 (described in section “Designs and Operational Description”).
To this aim, 1 m3 of treated WW was defined as FU. However, such FU does not include information about chemical nature of WW (e.g., BOD5). These considerations are important for testing systems with real WW because this medium is variable so systems will never be tested with WW of identical composition. Therefore, a MFU approach was also conducted (fully described in section “Multi-Funtional Unit Assessment”).
The system boundaries include the processes related to the construction and operation over a 25-year horizon period (Figure 3). The dismantling phase was considered non-significant in relation to the complete analysis and excluded. Additionally, all stages were systematically studied regarding both input and output flows of materials, energy, and intermediate processes. The final effluent direct emissions were also considered. Emissions to air such as direct Green House Gases (GHGs) were not accounted in this study due to the lack of such on-site data for our METland® systems.
Inventory Analysis
The results of the inventory of construction phase are summarized in Table 2, divided in the two generic designs and five periods. The lifespan of the construction for the inventory was assumed to be ca. 25 years. This assumption (ca. 15–30 years) is within the range previously reported by CW literature (Corominas et al., 2013; Lopsik, 2013; Garfí et al., 2017). Furthermore, this METland® unit was performed reusing the construction of a previous peat filter. Thus, in a new construction, the concrete will be replaced by a geotextile, reducing the overall environmental impact.
The operation inventory accounts the water quality related just to the performance of the METland unit (without the primary treatment) and monitoring (summarized in Supplementary Table A1). The energy needed for monitoring was obtained from solar radiation using photovoltaic panels. In CENTA, the radiation rate in the period analyzed was 18.54 MJ/m2, according to the Spanish Agency of Meteorology (AEMET, 2020).
Life Cycle Impact Assessment Method
The potential environmental impact of METland® designs was calculated using the software OpenLCAv1.8 (OpenLCA.org., 2006). Background processes were obtained from Ecoinvent3.4 database (Wernet et al., 2016), summarized in Supplementary Table A2. The impact method selected was Hierarchical ReCiPe Midpoint (Goedkoop et al., 2013). Table 3 summarizes the 10 impact categories and their abbreviators. The impact categories have been chosen following the tendency observed in the literature (Godin et al., 2012; Garfí et al., 2017; Flores et al., 2019).
Net Eutrophication Balance
Net Environmental Balance (NEB) perspective proposed by Godin et al. (2012) and Igos et al. (2012) allows to take into account the difference between discharging the WW directly into the environment (null option) or treating the WW (WWT scenario). However, this perspective inspired the development of indicators such as Eutrophication Net Environmental Impact (ENEI) proposed by Lorenzo-Toja et al. (2016). Centering in the eutrophication category relativized the NEB by the distance to target goals of legislation in terms of eutrophication. These perspective and indicators allow the consideration into the analysis and results of the inlet qualities and treatment effectiveness and also represent better the environmental trades-offs of the technology. An important advantage fitted to the experimental conditions is described in section “Designs and Operational Description.”
Based on the precedent studies, in this analysis, we proposed an indicator focused on the water quality before and after the treatment. The Net Eutrophication Balance (NEuB) defined by Eq. 1 represents the impact avoided due to the removal of pollutants achieved in the WWT.
EuPi, eutrophication potential of the direct discharge of WW.
EuPe, eutrophication potential caused by the discharge of the treated effluent.
EuPp, indirect eutrophication potential produced by the WWT processes.
NEuB is similar to NEB performed by Godin et al. (2012) but, in our case, it was focused in the selected eutrophication categories of the ReCiPe-Midpoint (H): ME and FE. This method difference represents separately the eutrophication impact associated with the Nitrogen (N) or Phosphorus (P) emissions, respectively.
Results and Discussion
METland® are solutions for effectively removing pollutants from WW. Interrogating the technology through a Life Cycle Assessment would provide info about its sustainability. Thus, in the current section, we will present and discuss the results from a techno-environmental analysis and a MFU study regarding different conceptual designs like mixed (aerobic/anaerobic) from D1 to fully aerobic D2.
Effectiveness Analysis
Our METland® units were treating real urban WW after a primary sedimentation of solids, and fulfilling the WWT discharge limits (EEC, 1991) for all conditions tested and regarding the population and the vulnerability of the implementation area. Removal rates of pollutants must be normalized per unit of biofiltering material (m3) in order to validate the efficiency of the technology under different scenarios (Figure 4).
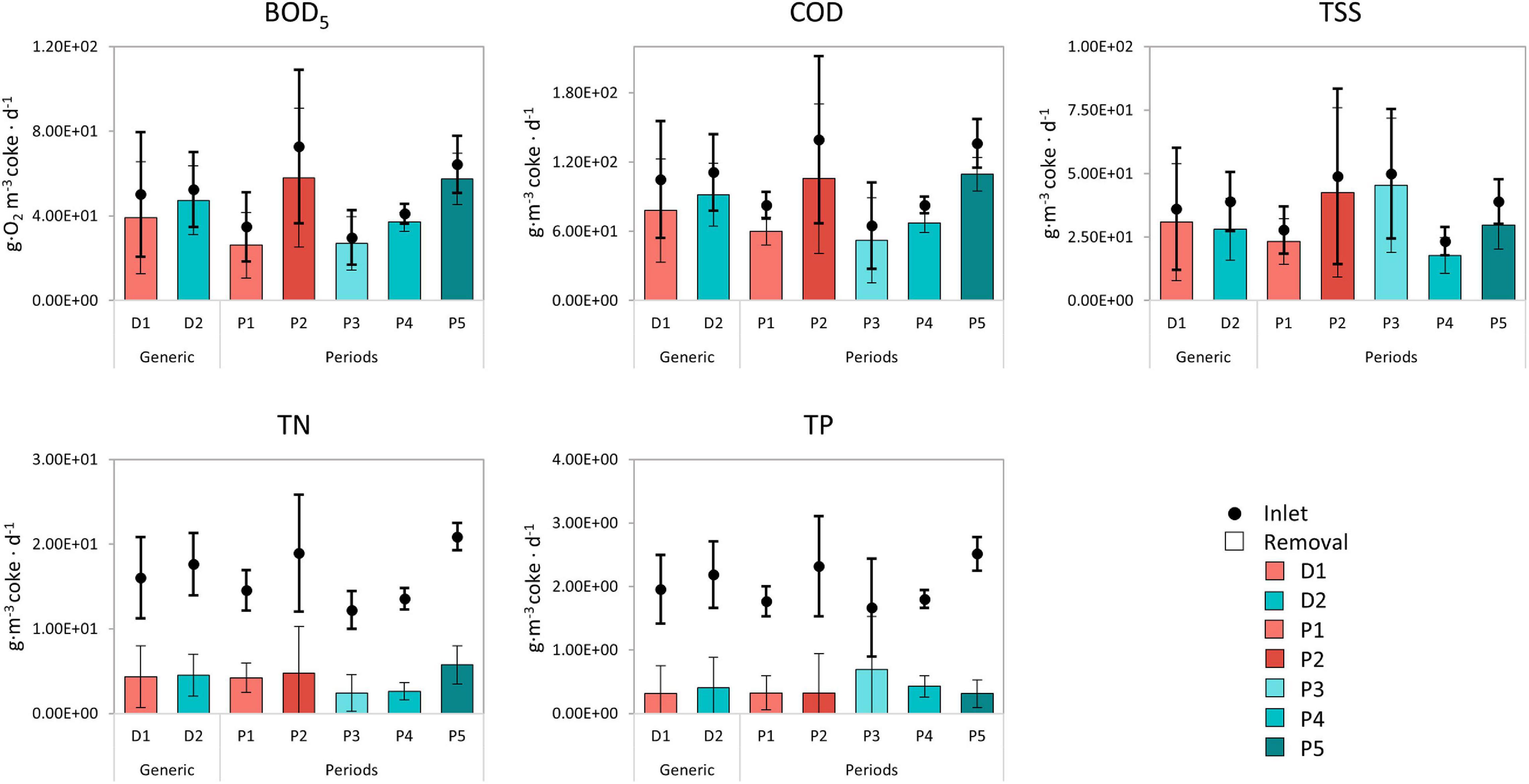
Figure 4. Pollutant removal rates normalized per cubic meter of bed material for both designs (D1 and D2) and five periods (P1–P5). The data correspond to the METland® unit itself (without considering the primary treatment). Columns so-called generic were calculated as the average of different periods. Error bars represent the standard deviation.
Regarding organic pollutants present in WW, the most important indicators are COD and BOD5, and indeed, both parameters revealed a higher removal in D2 than D1. There were significant statistical differences (p < 0.05) between D1 and D2 with a p-value of 4.4⋅10–4 for COD and 1.3⋅10–6 for BOD5. Precisely, the mixed aerobic–anaerobic nature of D1 removed 90% BOD5, while the purely aerobic D2 unit removed 94% of BOD5 regarding the raw WW. The results were consistent with data obtained in previous studies of METland® configurations (Prado et al., 2019; Ramírez-Vargas et al., 2019), including those reported by Aguirre-Sierra et al. (2020) with COD removal ranging from 82 to 99% in vertical down-flow configuration. The differences were possibly related with the higher oxygen availability due to the passive aeration of the downflow (D2) configuration. This situation is similar to the one found in constructed wetland operated under vertical flow. The removal rate per cubic meter of bed material was ca. 80 g COD per m3 of bed per day for D1 and ca. 90 g COD per m3 of bed⋅d–1 for D2 under the tested conditions. The periods analyzed correspond to first stages of operation; however, other studies using mature systems showed removal rates in the range of 150–200 g COD per m3 of bed per day (Aguirre-Sierra et al., 2017). These results suggest a similar removal rate than the ones achieved in additional experiences with one step EC bed where unsaturated and saturated zones co-exist in the same system (Cabred et al., 2019).
The biofiltering nature of METland® also exhibits a vast removal of those fine solids not properly removed by primary treatments. No significant differences were identified between models regarding TSS removal (p < 0.05). Thus, probably due to the higher hydraulic retention time of anoxic step in D1, such mixed aerobic–anaerobic design slightly outperformed D2 by 5% in terms of TSS removal efficiency, achieving 90% of average removal within the WWT. Similar results were reported by the Ramírez-Vargas et al. (2019) analysis with MET-CW-based anaerobic columns, 85–90% of TSS removal. Furthermore, the low growth yield typically detected in electroactive microorganism like Geobacter counteracts any clogging issue inside the bed (Mahadevan et al., 2006). In contrast, nitrogen removal on METland® is performed in two phases: nitrification that occurs under aerobic conditions (oxidation of ammonia to nitrate) and denitrification, reduction from nitrate to nitrogen gas, typically enhanced under anaerobic conditions (Aguirre-Sierra et al., 2017, 2020). Under aerobic–anoxic configuration (D1), both processes were feasible due to the anaerobic environments present in the inner part of the biofilm where Geobacter genus was detected (Aguirre-Sierra et al., 2020). Actually, D1 achieved stable removal rates in response to a variable loading, with an average reduction of 26% (4.53 g TN m–3 ⋅d–1). In contrast, D2 seems more influenced by the oxygen availability and, consequently, the ammonia removal was higher than in D1 via nitrification, revealing significant differences among designs (p-value = 1.1⋅10–9). Interestingly, both configurations show a similar behavior in TN removal (p-value = 0.07), suggesting an unexpected denitrification step even under passive aeration from D2. Such denitrification is supported by the presence of anoxic environments in the inner part of the biofilm where Geobacter was detected through microbial community analysis (Aguirre-Sierra et al., 2020).
In terms of TP, the removal efficiency ranged between 12% (P5) and 41% (P3). Both designs revealed a decrease in the removal of TP correlated with the increase of flow rate, for example, in D1, the removal decreased from 18% in P1 to 14% in P2. Thus, the removal processes were similar to those typically found in constructed wetland, mainly due to de-adsorption by the bed substrate (Bolton et al., 2019). In D1, the volume of EC material was 60% less in comparison to D2, but D2 periods present two times higher flow rate. D1 exhibited 16% average removal of TP compared to 18% in D2. Furthermore, if the primary treatment is included, the removal rate of TP increased to 36% in D1 and 20% in D2; this difference among designs could be due to the retention time. Nevertheless, new EC materials based on biochar with capacity for removing nutrients are currently under investigation (Schievano et al., 2019) and, eventually, will lead to a new generation of METland® where bed material may be fully recyclable at its end-of-life as a soil amendment.
Our studies revealed how the removal efficiency of pollutants can be correlated with flow rate (Figure 5). In accordance to the literature, BOD5 and COD are indirect indicators of organic matter in urban WW (Hur et al., 2010). Indeed, a strong correlation (95–98%) between the COD–BOD5 effectiveness (COD_E and BOD_E, respectively) and the flow rate (m3 per day) was observed, mainly due to the direct relation between the loading rate and the concentration of organic matter per cubic meter. On the other hand, nitrogen (N_E) and phosphorus removal effectiveness (P_E) had an inverse correlation between them (−73%), because the mechanisms of P removal are related mainly to physical processes and the N removal is mainly due to biological ones (Kadlec and Wallace, 2008).
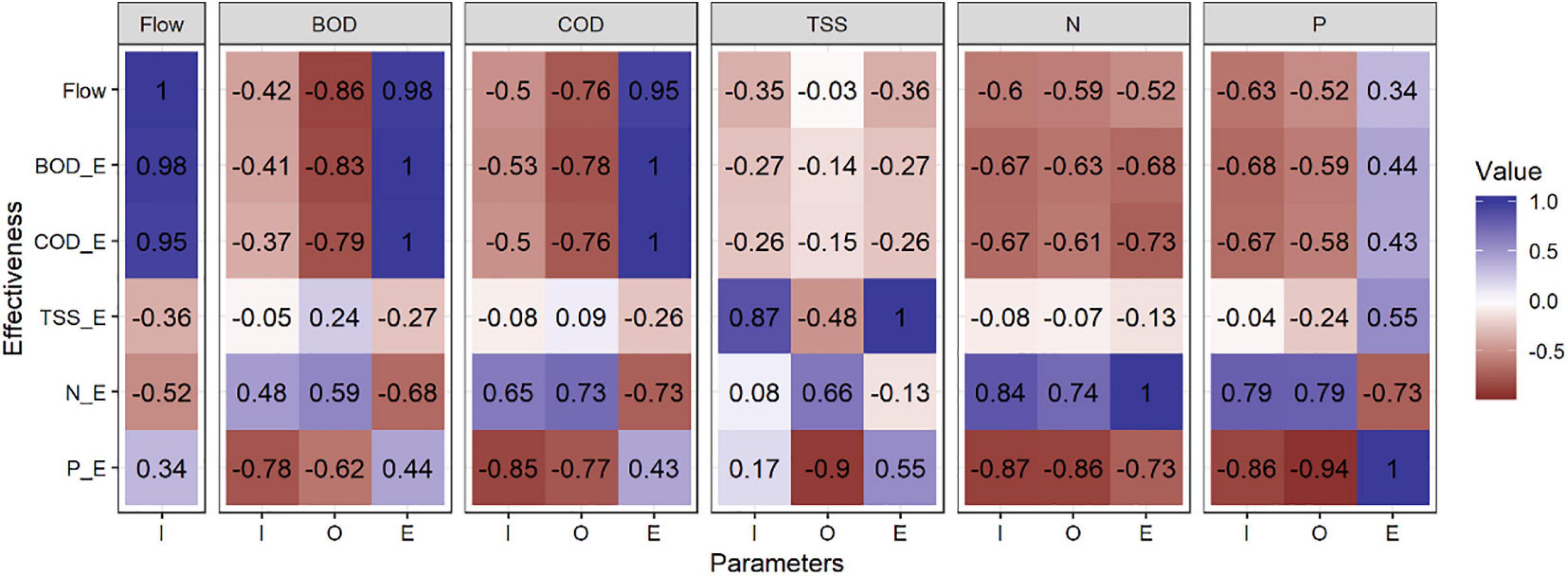
Figure 5. Correlation between the main parameters analyzed in the study and their effectiveness. Codes: Input (I), Output (O), and Effectiveness in % (E).
Life Cycle Assessment Results
The results of the selected impact categories and the process contribution for each design and period are summarized in Figure 6. All the categories except the eutrophication ones (ME and FE) follow the same pattern and similar performances between design and periods. The overall environmental impact of D1 was 33–77% higher than D2. Interestingly, from P1 to P5, a tendency for the total impact to progressively decrease between periods could be noticed, probably due to the increase of the daily flow rate. This mathematical relationship strengthens the need for the MFU analysis. The cause is the distribution of construction impact in a major amount of volume treated during the service life of the WWT. Nonetheless, the NEuB perspective reduces the WWT influence on the eutrophication categories. In those categories, the balance of the impacts achieves a negative value for eutrophication, which represents the avoided impact associated with the N and P removal.
Additionally, the contribution of each process to the impact categories is presented in Figure 6. Construction phase presents a higher contribution in all the categories except for ME and FE, in which the operation phase was the most important one due to the avoided impact by the reduction of N and P emissions to water, mentioned before. A similar feature was found in the literature related to non-conventional technologies in which the environmental impacts are mainly influenced by the construction phase (Fuchs et al., 2011; DiMuro et al., 2014; Corbella et al., 2017; Lopes et al., 2020). This effect could be reasonably explained by the low energy and material flows associated with operation; indeed, METland® does not require energy or chemical consumption and does not produce sludge since electroactive biofilm is tightly associated with the bed material. The unique energy flow included in the assessment was produced by those solar panels feeding the monitoring system (e.g., bioelectrochemical sensors). The energy savings of the system were mainly related to the absence of artificial aeration typically found in standard intensive WWTs (Dixon et al., 2003). On the other hand, assuming the total construction environmental impact in both conventional constructed wetland and METland®, the last one was able to treat higher volume of wastewater per footprint (Aguirre-Sierra et al., 2016, 2020).
The contribution profiles are similar between most of the categories (CC, FD, FET, HT, ME, PMF, and POF): coke (95–74%), concrete (3–18%), and plastic pipes (1–4%). Concretely, in the CC category, coke use represents 74% of D1 and 82% of D2 overall impact. Coke upstream processes accumulate most of the impact, that is, mainly due to the high temperatures and the energy required for this transformation from coal to coke within the pyrolysis process (Liu and Yuan, 2016). It must be mentioned that the life span of coke is longer than other CW materials; therefore, the construction impact will be lower distributed in time. Further works in METland® should focus on the use of low impact and ultra-conductive materials such as EC biochar (charcoal) (Prado et al., 2019; Schievano et al., 2019). Even the usage of valorized agricultural wastes pyrolysed could be an environmentally friendly alternative to consider.
Otherwise, OD category presents a different impact profile in which the plastic pipes accumulate most of the impact (58–68%), followed by coke (28–38%) and the concrete almost insignificant (2%). This dissimilarity was well described in the study of Corbella et al. (2017). In addition, from the OD, it can be clearly deduced that D1 produces a 77% higher impact than D2, presenting the largest difference in total impact between designs. Furthermore, by contrast, categories of ME and FE present a negative value of N and P removal, respectively. In this case, the NEuB perspective provides the inclusion of the effectiveness of METland® treatment. For FE, the greater avoided impact is associated with D2 and for the ME with D1. During P3, the most important P removal (42%) is achieved, resulting in the lowest impact (−2.17⋅10–3 kg P eq. m–3) (Figure 4). The trend observed is a lower impact with higher removal rate in N and P.
The herein studied METland® generated 0.29 kg CO2 eq.⋅m–3. Other authors who previously modeled the impact of the theoretical integration of bioelectrochemical elements predicted a similar impact (0.34 kg CO2 eq.⋅m–3) if a hypothetical anode (59 m3) and a cathode (245 m3) made of graphite were integrated in a constructed wetland (Corbella et al., 2017). So, METland® impact seems to be in the same range than other nature-based solutions (DiMuro et al., 2014; Lutterbeck et al., 2017) and significantly lower than activated sludge systems 1.2 kg CO2 eq.⋅m–3 (Machado et al., 2007; Garfí et al., 2017).
From an environmental point of view, the aerobic configuration (D2) appeared as the best alternative (cubic meter of treated wastewater, FU). Particularly, the low impact of the third period (P5) pointed out the high capacity of the treatment for high flow rates and, consequently, a reduction of the impact. Although the EC material used in the D2 construction was increased 60% in respect of D1, its higher effectiveness resulted in a lower impact.
Multi-Functional Unit Assessment
As mentioned before, volume units as a FU do not necessarily reflect the removal efficiency for all parameters monitored in WW (Comas Matas and Morera Carbonell, 2012; Corominas et al., 2013; Gallego-Schmid and Tarpani, 2019). Therefore, the present study incorporates a MFU analysis. Results were aggregated around a total of six FUs to represent the eco-effectiveness of different parameters: (i) the treatment of 1 m3, used for the central analysis, (ii) the removal of 1 g of TSS, (ii) the removal of 1 g of BOD, (iv) the removal of 1 g of COD, (v) the removal of 1 g of TN, and (vi) the removal of 1 g of TP. The methodological proposal aims to improve the robustness of the decision by solving the uncertainties associated with the influent loads and the associated effectivities. Furthermore, for a deeper understanding, LCA results and operational conditions were analyzed together with Principal Component Analyses (PCA) assessed with R (R Core Team, 2018).
Comparative Results
The comparative results between designs and periods for different FU showed a wide variability (Figure 7). Our designed FU clearly evidenced that D2 was the foremost option. Nonetheless, the effectiveness of the treatments measured under different parameters obtained no direct correlation with the flow (characterized by our FU, m3). Therefore, the analysis of FU associated with the removal of pollutants (N, P, BOD, COD, and TSS) obtains a different trend among the periods. There is no remarkable difference between periods for every specific design. On the contrary, LCA results showed how the lower impact can shift among designs (e.g., BOD and COD removal from P5 at D2 and P2 at D1). Regarding N removal, P5 appeared as the most environmentally friendly option. In contrast, D1 (for both periods) showed similar results. ME and FE categories present a beneficial impact for all the FU due to the Net Environmental Balance perspective. Specifically, these results suggest that D1 could be more environmentally friendly under specific situations where high N removal is required. Nonetheless, a deeper analysis was required so a PCA was further conducted.
Principal Component Analysis
In order to delve into the impact of FUs on the different categories, a PCA was performed. Plotting all periods and designs (individuals) over the two most contributing dimensions revealed a variance of 77% (first dimension: 51.25% and second dimension: 27.52%) (Figure 8). Along such dimensions, the similarities between FUs, impact categories, and technical parameters could be easily compared. D1 periods were marked by Dim 2 and D2 periods by Dim 1. Furthermore, P2 results were similar to the P3–P5 periods.
For a full interpretation of the PCA, the interaction among impact categories, evaluated FUs, and WW parameters (N, P, TSS, COD, BOD, and flow) were analyzed (Figure 9). Firstly, most of the impacts for each category (arrows) followed the same trend when 1 m3 was used as FU; indeed, they were opposite to the flow rate (input supplementary variable). Furthermore, our analysis revealed that the higher the volume treated, the lower the impact. However, both eutrophication categories, ME and FE, were dominated by the effectiveness of their key parameter (N and P, respectively). Such trends were maintained in the remaining FUs including all categories regarding N and P removal. Moreover, the most important trends were associated with the flow rate and N removal. In this sense, the aerobic configuration (D2) was correlated with the environmental foremost option within high flow rates of BOD and COD effectiveness. Nonetheless, aerobic–anoxic configuration (D1) could be more associated with adaptation to high N inlet. However, differences of the flow rates among periods were remarkable. Indeed, aerobic–anoxic configuration (D1) could be an option to consider for high N-content effluents with low flow rate or high N removal needs (e.g., manure effluents and small community effluents discharging to sensitive areas). Nonetheless, the limitations of the experimentation should be analyzed in further studies with a larger number of environmental factors such as meteorological conditions and climates to include dynamic LCA and perform a multi-scenario analysis to define precisely the frontiers between designs.
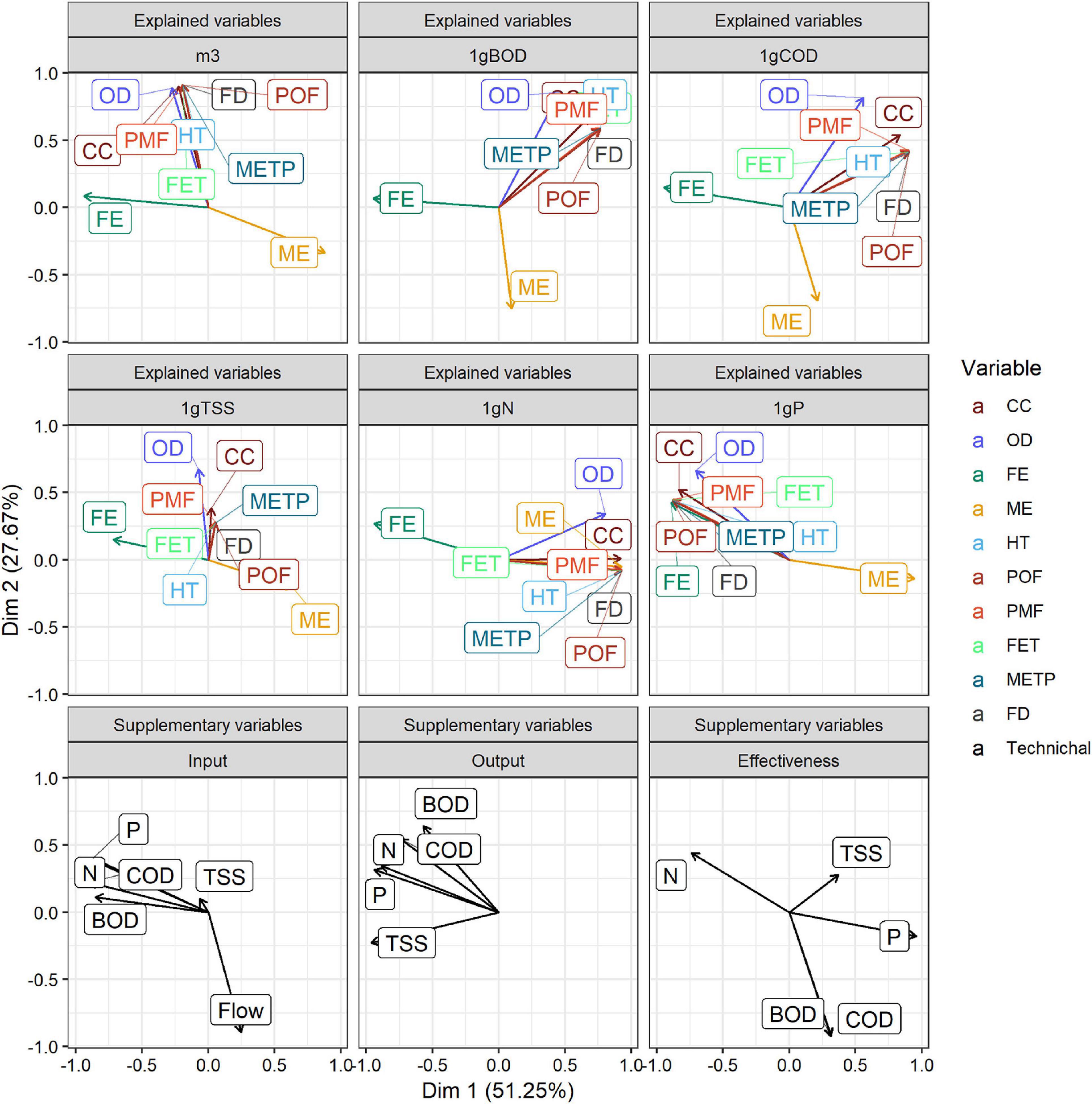
Figure 9. Results of PCA analysis for the results depending on the selected FU, category, and related to the technical parameters.
Conclusion
Our study revealed that the new technology, so-called METland®, achieved environmental impacts as low as other nature-based solutions and significantly lower than those from conventional treatments like activated sludge. Furthermore, the solution is ideal for rural areas, considering the low energy requirements and high efficiencies to remove organic pollutants, nitrogen, and phosphates from urban wastewater.
Moreover, a combined technical and environmental analysis using a NEuB focus concluded that the aerobic downflow mode (D2) was the most environmentally friendly design, achieving the highest removal rates for carbon-based and nitrogen pollutants. The lower impact for such aerobic downflow configuration was also confirmed through a multi-functional analysis with different FU.
METland® technology is being currently upgraded through innovation actions including the use of new materials and operation modes not included in the current study, so we can anticipate further that LCA analysis should be performed in the near future.
Data Availability Statement
The raw data supporting the conclusions of this article will be made available by the authors, without undue reservation.
Author Contributions
AE-N, JS-S, LP-A, and PL: conceptualization. AA-S and LP-A: data curation. JS-S and LP-A: formal analysis, methodology, investigation, software, and visualization. AE-N, EG-C, and JS: funding acquisition and supervision. AE-N, JS-S, and LP-A: writing—original draft. All authors contributed to the article and approved the submitted version.
Conflict of Interest
The authors declare that the research was conducted in the absence of any commercial or financial relationships that could be construed as a potential conflict of interest.
Funding
This investigation received funding from the EuropeanUnion’s Horizon 2020 Research and Innovation Programme under the grant agreement No. 826244 (Project “ELECTRA”; http://www.electra.site). Lorena Peñacoba Antona was funded by the Industrial Ph.D. fellowship program from the Regional Government of Madrid: IND2017/AMB-7648.
Supplementary Material
The Supplementary Material for this article can be found online at: https://www.frontiersin.org/articles/10.3389/fmicb.2021.652173/full#supplementary-material
Abbreviations
AEMET, Spanish Meteorological Agency; BOD5,, biochemical oxygen demand; CC, global warming potential; CENTA, Foundation Centre for New Water Technologies; COD, chemical oxygen demand; CW, constructed wetland; D1, first design; D2, second design; E, effectiveness; EC, electroconductive; ENEI, eutrophication net environmental impact; FD, fossil depletion potential; FE, freshwater eutrophication potential; FET, freshwater ecotoxicity potential; FU, functional unit; GHGs, green house gases; HT, human toxicity potential; I, inflow, influent; LCA, life cycle assessment; ME, marine eutrophication potential; MET, microbial electrochemical technologies; METP, marine ecotoxicity potential; MFC, microbial fuel cell; N, nitrogen; NEB, Net Environmental Balance; NEuB, net eutrophication balance; O, outflow, effluent; OD, ozone depletion potential; P, phosphorus; P1, first design, medium loading rate; P2, first design, high loading rate; P3, second design, low loading rate; P4, second design, medium loading rate; P5, second design, high loading rate; PCA, principal component analysis; PE, polyethylene; PMF, particulate matter formation potential; POF, photochemical oxidant formation potential; PVC, polyvinyl chloride; TN, total nitrogen; TP, total phosphorus; TSS, total suspended solids; WW, wastewater; WWT, wastewater treatment.
Footnotes
References
AEMET (2020). State Meteorological Agency - AEMET - Spanish Government. Available online at: http://www.aemet.es/en/lineas_de_interes/centro_de_documentacion (accessed January 28, 2020).
Aguirre-Sierra, A., Bacchetti-De Gregoris, T., Berná, A., Salas, J. J., Aragón, C., and Esteve-Núñez, A. (2016). Microbial electrochemical systems outperform fixed-bed biofilters in cleaning up urban wastewater. Environ. Sci. Water Res. Technol. 2, 984–993. doi: 10.1039/C6EW00172F
Aguirre-Sierra, A., Bacchetti-De Gregoris, T., Salas, J. J., de Deus, A., and Esteve-Núñez, A. (2020). A new concept in constructed wetlands: assessment of aerobic electroconductive biofilters. Environ. Sci. Water Res. Technol. 6, 1312–1323. doi: 10.1039/C9EW00696F
Aguirre-Sierra, M. A., Esteve-Núñez, A., and Salas-Rodriguez, J. J. (2017). Integrating microbial electrochemical systems in constructed wetlands, a new paradigm for treating wastewater in small communities. Alcalá de Henares: University of Alcalá.
American Public Health Association. (2005). Standard methods for the examination of water and wastewater, 21st Edn. Washington DC: American Public Health Association.
Arashiro, L. T., Montero, N., Ferrer, I., Acién, F. G., Gómez, C., and Garfí, M. (2018). Life cycle assessment of high rate algal ponds for wastewater treatment and resource recovery. Sci. Total Environ. 62, 1118–1130. doi: 10.1016/j.scitotenv.2017.12.051
Bolton, L., Joseph, S., Greenway, M., Donne, S., Munroe, P., and Marjo, C. E. (2019). Phosphorus adsorption onto an enriched biochar substrate in constructed wetlands treating wastewater. Ecol. Eng. X 1, 100005. doi: 10.1016/J.ECOENA.2019.100005
Cabred, S., Giunta Ramos, V., Busalmen, J. E., Busalmen, J. P., and Bonanni, S. (2019). Reduced depth stacked constructed wetlands for enhanced urban wastewater treatment. Chem. Eng. J. 372, 708–714. doi: 10.1016/J.CEJ.2019.04.180
Comas Matas, J., and Morera Carbonell, S. (2012). Life cycle assessment and water management-related issues. Girona: Documenta Universitaria.
Corbella, C., Puigagut, J., and Garfí, M. (2017). Life cycle assessment of constructed wetland systems for wastewater treatment coupled with microbial fuel cells. Sci. Total Environ. 58, 355–362. doi: 10.1016/J.SCITOTENV.2016.12.186
Corominas, L., Foley, J., Guest, J. S., Hospido, A., Larsen, H. F., Morera, S., et al. (2013). Life cycle assessment applied to wastewater treatment: State of the art. Water Res. 47, 5480–5492. doi: 10.1016/J.WATRES.2013.06.049
EEC (1991). Council Directive 91/271/EEC of 21 May 1991 Concerning Urban Waste-Water Treatment. Brussels:EEC.
De Feo, G., and Ferrara, C. (2017). A procedure for evaluating the most environmentally sound alternative between two on-site small-scale wastewater treatment systems. J. Clean. Prod. 164, 124–136. doi: 10.1016/j.jclepro.2017.06.205
DiMuro, J. L., Guertin, F. M., Helling, R. K., Perkins, J. L., and Romer, S. (2014). A Financial and Environmental Analysis of Constructed Wetlands for Industrial Wastewater Treatment. J. Ind. Ecol. 18, 631–640. doi: 10.1111/jiec.12129
Dixon, A., Simon, M., and Burkitt, T. (2003). Assessing the environmental impact of two options for small-scale wastewater treatment: Comparing a reedbed and an aerated biological filter using a life cycle approach. Ecol. Eng. 20, 297–308. doi: 10.1016/S0925-8574(03)00007-7
EEA (2019). Urban waste water treatment for 21st century challenges — European Environment Agency. Available online at: https://www.eea.europa.eu/publications/urban-waste-water-treatment-for (accessed December 21, 2020).
Flores, L., García, J., Pena, R., and Garfí, M. (2019). Constructed wetlands for winery wastewater treatment: A comparative Life Cycle Assessment. Sci. Total Environ. 659, 1567–1576. doi: 10.1016/J.SCITOTENV.2018.12.348
Fuchs, V. J., Mihelcic, J. R., and Gierke, J. S. (2011). Life cycle assessment of vertical and horizontal flow constructed wetlands for wastewater treatment considering nitrogen and carbon greenhouse gas emissions. Water Res. 45, 2073–2081. doi: 10.1016/J.WATRES.2010.12.021
Gallego-Schmid, A., and Tarpani, R. R. Z. (2019). Life cycle assessment of wastewater treatment in developing countries: A review. Water Res. 153, 63–79. doi: 10.1016/J.WATRES.2019.01.010
Garfí, M., Flores, L., and Ferrer, I. (2017). Life Cycle Assessment of wastewater treatment systems for small communities: Activated sludge, constructed wetlands and high rate algal ponds. J. Clean. Prod. 161, 211–219. doi: 10.1016/J.JCLEPRO.2017.05.116
Godin, D., Bouchard, C., and Vanrolleghem, P. A. (2012). Net environmental benefit: introducing a new LCA approach on wastewater treatment systems. Water Sci. Technol. 65, 1624–1631. doi: 10.2166/wst.2012.056
Goedkoop, M., Heijungs, R., Huijbregts, M., De Schryver, A., Struijs, J., and van Zelm, R. (2013). ReCiPe 2008 A life cycle impact assessment method which comprises harmonised category indicators at the midpoint and the endpoint level. Available online at: https://www.rivm.nl/sites/default/files/2018-11/ReCiPe 2008_A lcia method which comprises harmonised category indicators at the midpoint and the endpoint level_First edition Characterisation.pdf (accessed October 1, 2019).
Hur, J., Lee, B.-M., Lee, T.-H., and Park, D.-H. (2010). Estimation of Biological Oxygen Demand and Chemical Oxygen Demand for Combined Sewer Systems Using Synchronous Fluorescence Spectra. Sensors (Basel). 10, 2460. doi: 10.3390/S100402460
Igos, E., Benetto, E., Venditti, S., Kohler, C., Cornelissen, A., Moeller, R., et al. (2012). Is it better to remove pharmaceuticals in decentralized or conventional wastewater treatment plants? A life cycle assessment comparison. Sci. Total Environ. 438, 533–540. doi: 10.1016/J.SCITOTENV.2012.08.096
ISO 14040 (2006). Environmental management - Life cycle assessment - Principles and framework. Geneva: ISO 14040.
ISO 14044 (2006). Environmental management - Life cycle assessment - Requirements and guidelines. Geneva: ISO 14044.
JCR (2010). International Reference Life Cycle Data System (ILCD) Handbook, General guide for Life Cycle Assessment - Detailed guidance. Luxembourg: Publications Office of the European Union, doi: 10.2788/38479 European Commission - Joint Research Centre - Institute for Environment and Sustainability.
Liu, X., and Yuan, Z. (2016). Life cycle environmental performance of by-product coke production in China. J. Clean. Prod. 112, 1292–1301. doi: 10.1016/J.JCLEPRO.2014.12.102
Lopes, T. A. S., Queiroz, L. M., Torres, E. A., and Kiperstok, A. (2020). Low complexity wastewater treatment process in developing countries: A LCA approach to evaluate environmental gains. Sci. Total Environ. 720, 137593. doi: 10.1016/j.scitotenv.2020.137593
Lopsik, K. (2013). Life cycle assessment of small-scale constructed wetland and extended aeration activated sludge wastewater treatment system. Int. J. Environ. Sci. Technol. 10, 1295–1308. doi: 10.1007/s13762-012-0159-y
Lorenzo-Toja, Y., Vázquez-Rowe, I., Amores, M. J., Termes-Rifé, M., Marín-Navarro, D., Moreira, M. T., et al. (2016). Benchmarking wastewater treatment plants under an eco-efficiency perspective. Sci. Total Environ. 56, 468–479. doi: 10.1016/J.SCITOTENV.2016.05.110
Lorenzo-Toja, Y., Vázquez-Rowe, I., Marín-Navarro, D., Crujeiras, R. M., Moreira, M. T., and Feijoo, G. (2018). Dynamic environmental efficiency assessment for wastewater treatment plants. Int. J. Life Cycle Assess. 23, 357–367. doi: 10.1007/s11367-017-1316-9
Lundin, M., Bengtsson, M., and Molander, S. (2000). Life Cycle Assessment of Wastewater Systems: Influence of System Boundaries and Scale on Calculated Environmental Loads. Environ. Sci. Technol. 34, 180–186. doi: 10.1021/es990003f
Lutterbeck, C. A., Kist, L. T., Lopez, D. R., Zerwes, F. V., and Machado, E. L. (2017). Life cycle assessment of integrated wastewater treatment systems with constructed wetlands in rural areas. J. Clean. Prod. 148, 527–536. doi: 10.1016/j.jclepro.2017.02.024
Machado, A. P. P., Urbano, L., Brito, A. G. G., Janknecht, P., Salas, J. J. J., and Nogueira, R. (2007). Life cycle assessment of wastewater treatment options for small and decentralized communities. Water Sci. Technol. 56, 15–22. doi: 10.2166/wst.2007.497
Mahadevan, R., Bond, D. R., Butler, J. E., Esteve-Nuñez, A., Coppi, M. V., Palsson, B. O., et al. (2006). Characterization of metabolism in the Fe(III)-reducing organism Geobacter sulfurreducens by constraint-based modeling. Appl. Environ. Microbiol. 72, 1558–1568. doi: 10.1128/AEM.72.2.1558-1568.2006
Mahmood, Q., Pervez, A., Zeb, B. S., Zaffar, H., Yaqoob, H., Waseem, M., et al. (2013). Natural treatment systems as sustainable ecotechnologies for the developing countries. Biomed Res. Int. 2013, 796373. doi: 10.1155/2013/796373
Massoud, M. A., Tarhini, A., and Nasr, J. A. (2009). Decentralized approaches to wastewater treatment and management: Applicability in developing countries. J. Environ. Manage. 90, 652–659. doi: 10.1016/j.jenvman.2008.07.001
Niero, M., Pizzol, M., Bruun, H. G., and Thomsen, M. (2014). Comparative life cycle assessment of wastewater treatment in Denmark including sensitivity and uncertainty analysis. J. Clean. Prod. 68, 25–35.
OpenLCA.org. (2006). GreenDelta openLCA.org. Available online at: https://www.openlca.org/ (accessed April 15, 2019).
Peñacoba-Antona, L., Gómez-Delgado, M., and Esteve-Núñez, A. (2021). Multi-criteria evaluation and sensitivity analysis for the optimal location of constructed wetlands (METland) at oceanic and Mediterranean Areas. Int. J. Environ. Res. Public Health 18:5415. doi: 10.3390/ijerph18105415
Prado, A., Berenguer, R., and Esteve-Núñez, A. (2019). Electroactive biochar outperforms highly conductive carbon materials for biodegrading pollutants by enhancing microbial extracellular electron transfer. Carbon N. Y. 146, 597–609. doi: 10.1016/j.carbon.2019.02.038
Prado, A., Ramírez-Vargas, C. A., Arias, C. A., and Esteve-Núñez, A. (2020). Novel bioelectrochemical strategies for domesticating the electron flow in constructed wetlands. Sci. Total Environ. 735, 139522. doi: 10.1016/j.scitotenv.2020.139522
Pun, Á, Boltes, K., Letón, P., and Esteve-Nuñez, A. (2019). Detoxification of wastewater containing pharmaceuticals using horizontal flow bioelectrochemical filter. Bioresour. Technol. Reports 7, 100296. doi: 10.1016/j.biteb.2019.100296
R Core Team. (2018). R: A language and environment for statistical computing. Available online at: https://www.r-project.org/ (accessed May 9, 2019).
Ramírez-Vargas, C. A., Arias, C. A., Carvalho, P., Zhang, L., Esteve-Núñez, A., and Brix, H. (2019). Electroactive biofilm-based constructed wetland (EABB-CW): A mesocosm-scale test of an innovative setup for wastewater treatment. Sci. Total Environ. 659, 796–806. doi: 10.1016/J.SCITOTENV.2018.12.432
Resende, J. D., Nolasco, M. A., and Pacca, S. A. (2019). Life cycle assessment and costing of wastewater treatment systems coupled to constructed wetlands. Resour. Conserv. Recycl. 148, 170–177. doi: 10.1016/j.resconrec.2019.04.034
Rotaru, A. E., Yee, M. O., and Musat, F. (2021). Microbes trading electricity in consortia of environmental and biotechnological significance. Curr. Opin. Biotechnol. 67, 119–129. doi: 10.1016/j.copbio.2021.01.014
Schievano, A., Berenguer, R., Goglio, A., Bocchi, S., Marzorati, S., Rago, L., et al. (2019). Electroactive Biochar for Large-Scale Environmental Applications of Microbial Electrochemistry. ACS Sustain. Chem. Eng. 7, 18198–18212. doi: 10.1021/acssuschemeng.9b04229
UNESCO (2018). The United Nations world water development report 2018: nature-based solutions for water. Available online at: https://unesdoc.unesco.org/ark:/48223/pf0000261424 (accessed September 13, 2019).
UNICEF and WHO (2017). Progress on Drinking Water, Sanitation and Hygiene: 2017 Update and SDG Baselines. Available online at: https://www.unicef.org/publications/index_96611.html (accessed March 23, 2019).
Wang, X., Aulenta, F., Puig, S., Esteve-Núñez, A., He, Y., Mu, Y., et al. (2020). Microbial electrochemistry for bioremediation. Environ. Sci. Ecotechnology 1, 100013. doi: 10.1016/j.ese.2020.100013
Wernet, G., Bauer, C., Steubing, B., Reinhard, J., Moreno-Ruiz, E., and Weidema, B. (2016). The ecoinvent database version 3 (part I): overview and methodology. Int. J. Life Cycle Assess. 21, 1218–1230. doi: 10.1007/s11367-016-1087-8
Zang, Y., Li, Y., Wang, C., Zhang, W., and Xiong, W. (2015). Towards more accurate life cycle assessment of biological wastewater treatment plants: a review. J. Clean. Prod. 107, 676–692. doi: 10.1016/J.JCLEPRO.2015.05.060
Zhao, X., Bai, S., and Zhang, X. (2019). Establishing a decision-support system for eco-design of biological wastewater treatment: A case study of bioaugmented constructed wetland. Bioresour. Technol. 274, 425–429. doi: 10.1016/J.BIORTECH.2018.12.016
Keywords: life cycle assessment, METland, Net Environmental Balance, Funtional Unit, wastewater treatment, treatment wetlands, constructed wetland, principal component analysis
Citation: Peñacoba-Antona L, Senán-Salinas J, Aguirre-Sierra A, Letón P, Salas JJ, García-Calvo E and Esteve-Núñez A (2021) Assessing METland® Design and Performance Through LCA: Techno-Environmental Study With Multifunctional Unit Perspective. Front. Microbiol. 12:652173. doi: 10.3389/fmicb.2021.652173
Received: 11 January 2021; Accepted: 03 May 2021;
Published: 11 June 2021.
Edited by:
Jochen A. Mueller, Karlsruhe Institute of Technology (KIT), GermanyReviewed by:
Fabio Masi, IRIDRA Srl, ItalyZhongbing Chen, Czech University of Life Sciences Prague, Czechia
Copyright © 2021 Peñacoba-Antona, Senán-Salinas, Aguirre-Sierra, Letón, Salas, García-Calvo and Esteve-Núñez. This is an open-access article distributed under the terms of the Creative Commons Attribution License (CC BY). The use, distribution or reproduction in other forums is permitted, provided the original author(s) and the copyright owner(s) are credited and that the original publication in this journal is cited, in accordance with accepted academic practice. No use, distribution or reproduction is permitted which does not comply with these terms.
*Correspondence: Abraham Esteve-Núñez, YWJyYWhhbS5lc3RldmVAdWFoLmVz