- College of Veterinary Medicine, Qingdao Agricultural University, Qingdao, China
A novel virulent bacteriophage vB_EcoM_swi3 (swi3), isolated from swine feces, lyzed 9% (6/65) of Escherichia coli and isolates 54% (39/72) of Salmonella enteritidis isolates, which were all clinically pathogenic multidrug-resistant strains. Morphological observation showed that phage swi3 belonged to the Myoviridae family with an icosahedral head (80 nm in diameter) and a contractile sheathed tail (120 nm in length). At the optimal multiplicity of infection of 1, the one-step growth analysis of swi3 showed a 25-min latent period with a burst size of 25-plaque-forming units (PFU)/infected cell. Phage swi3 remained stable both at pH 6.0–8.0 and at less than 50°C for at least 1 h. Genomic sequencing and bioinformatics analysis based on genomic sequences and the terminase large subunit showed that phage swi3 was a novel member that was most closely related to Salmonella phages and belonged to the Rosemountvirus genus. Phage swi3 harbored a 52-kb double-stranded DNA genome with 46.02% GC content. Seventy-two potential open reading frames were identified and annotated, only 15 of which had been assigned to functional genes. No gene associated with pathogenicity and virulence was identified. The effects of phage swi3 in treating pathologic E. coli infections in vivo were evaluated using a mouse model. The administration of a single intraperitoneal injection of swi3 (106 PFU) at 2 h after challenge with the E. coli strain (serotype K88) (108 colony-forming units) sufficiently protected all mice without toxic side effects. This finding highlighted that phage swi3 might be used as an effective antibacterial agent to prevent E. coli and S. enteritidis infection.
Introduction
Pathogenic Escherichia coli and Salmonella enteritidis are the major opportunistic pathogens in animals and humans and are frequently reported worldwide (Majowicz et al., 2010; Yang et al., 2017; Jajere, 2019). Rearing animals on a large scale can increase pathogen infections. Particularly in young animals in high-density farming models, outbreaks caused by E. coli and S. enteritidis are usually associated with gastroenteritis and diarrhea and lead to serious infections and high mortality rates. To control enteric infections, antibiotics are widely used in breeding farms to treat infections. However, the widespread use of antibiotics had led to a series of problems, such as drug resistance, environmental pollution, and antibiotic residues in animal products (Paitan, 2018; Poirel et al., 2018; Jajere, 2019; Thames and Theradiyil Sukumaran, 2020). The prevention and control of bacterial infection urgently require the development of alternative antibiotic products.
Bacteriophages (phages) are viruses that exclusively infect bacteria and are widely distributed in the environment (Parikka et al., 2017). In the past decade, phages have demonstrated a promising alternative antibiotic role in the control of pathogenic bacteria in animals and humans (Chang et al., 2018; Rehman et al., 2019). Additionally, phages are a resource for many biotechnological applications, such as antimicrobial enzymes, bacteria typing, and phage display libraries (Salmond and Fineran, 2015). However, the host range of reported phages is commonly limited to a single species, and few phages can infect more than one species. Therefore, phage cocktails were developed to control polymicrobial infections in phage therapy. In our opinion, a broader-spectrum phage would presumably lead to fewer failures due to a mismatched host and phage combination. Myoviridae phages usually exhibit a broader host range than Siphoviridae and Podoviridae (Chibani-Chennoufi et al., 2004). Moreover, it is necessary to acquire a clear understanding of biology and genetic information to ensure effectiveness and safety before the use of phages. A suitable phage candidate for effective biocontrol should be a lytic phage with a broad host range against a variety of bacterial strains and should not carry virulence and pathogenicity genes in the genome. To date, the reported phages in the database have only been the tip of the iceberg, and most of them belong to the Myoviridae or the Siphoviridae families of tailed phages (Yu et al., 2006).
In this study, a novel polyvalent bacteriophage vB_EcoM_swi3 was isolated and characterized. Phage swi3 showed a wide host range; it could lyze 9% (6/65) of E. coli strain and 54% (39/72) of S. enteritidis strain, and it showed a good protective effect in a mouse model challenged with pathogenic E. coli. These data provide valuable information to assess the potential of phage swi3 as a biocontrol agent against pathogenic bacteria.
Materials and Methods
Bacterial Strains and Animals
Sixty-five pathogenic E. coli and 72 pathogenic S. enteritidis clinical isolates were used in this study (these strains were identified as different strains from different batches of diseased animals in Hebei, Shandong, and Jilin from 2010 to 2020, and drug sensitivity tests revealed different drug resistances). The bacterial strains were cultured in Luria–Bertani culture (LB) at 37°C and stored in 30% glycerol at −80°C.
Female BALB/c mice, 5 weeks of age (20–25 g in weight), were purchased from the Experimental Animal Center of Shandong, China.
All animal procedures were performed in strict accordance with the Regulations for the Administration of Affairs Concerning Experimental Animals, approved through the State Council of the People’s Republic of China (1988.11.1), and with the approval of the Animal Welfare and Research Ethics Committee at Qingdao Agricultural University.
Propagation and Morphology of Phage Swi3
A pathogenic clinical strain of E. coli (serotype K88), hereafter named E. coli K88, was used in this study for phage isolation. Feces and seawater samples were collected from a pig farm in Shandong, China. Phage isolation was performed using the standard enrichment method as described before but with slight modifications (Lu et al., 2017). In brief, an overnight culture of 50 ml of E. coli K88 was cocultured with collected samples at 37°C for 24 h and then centrifuged at 12,000 rpm for 30 min. The supernatant was filtered with a sterile disposable membrane filter (0.22 μm). Then, 100 μl of the filtered supernatant was added to 100 μl of log-phase E. coli K88 and mixed with 3 ml of prewarmed NA top agar (0.7% agar), spread on an NA plate, and cultured for 4 h at 37°C. A single plaque was selected and picked from the plate. Then, the plaque was leached overnight with 0.9% normal saline. This procedure was repeated three times to obtain purified phages. Electron micrographs of purified phage particles were obtained according to a standard method (Kreienbaum et al., 2020). A 10-μl phage sample was dropped onto carbon-coated grids, negatively stained with 2% (w/v) aqueous uranyl acetate (pH 4.0) for 5 min, and air-dried. The samples were observed with a transmission electron microscope (HT7700, Hitachi, Japan) at 80-kV accelerating voltage.
Host Range Determination of Phage Swi3
Sixty-five E. coli and 72 S. enteritidis clinical isolates were used to test the host range of phage swi3 by the spot method. Briefly, 10 μl of diluted phage suspension (1 × 105 PFU/ml) was spotted on each bacterial lawn on agar plates and incubated at 37°C for 4 h. The presence of plaques was examined. Additionally, the efficiency of plating was tested as described before (Ding et al., 2020).
Optimal Multiplicity of Infection of Phage Swi3
To determine the optimal multiplicity of infection (MOI) of phage swi3, E. coli K88 was cultured in LB broth at 37°C with shaking at 250 rpm until the early exponential phase (5 × 107 CFU/ml). Phage swi3 was cocultured with E. coli K88 in 10 ml of LB broth at different MOIs (0.0001, 0.001, 0.01, 0.1, 1, and 10) (Abedon, 2016). A culture of bacteria without phage swi3 (0 MOI) was used as a control. After 2.5 h of incubation, the culture was centrifuged at 10,000 rpm for 10 min, and then the supernatant was used to determine the phage titers of phage swi3 by the double-layered agar method. The MOI resulting in the highest phage titer was considered an optimal MOI and used in subsequent large-scale phage production.
One-Step Growth of Phage Swi3
The burst size and the latent period of phage swi3 were determined by one-step growth analysis as previously described (Sun et al., 2012). In brief, 200 μl of phage suspension (106 PFU) was mixed with 200 ml of bacterial culture (5 × 106 CFU/ml), incubated at 37°C for 5 min, and then centrifuged, and the cell pellet was resuspended in 500 μl of LB broth. Aliquots were taken, and the phage titers were immediately determined by the double-layered agar method. This assay was performed in triplicate.
Thermal/pH Stability of Phage Swi3
The stability of phage swi3 was tested as previously described (Akhwale et al., 2019). Briefly, thermal stability was assessed by incubating the phage swi3 (4 × 108 PFU/ml) at 40, 50, 60, 70, and 80°C, and aliquots (100 μl) were taken at 20, 40, and 60 min. To evaluate the stability of phage swi3 at various pH values (2, 3, 4, 5, 6, 7, 8, 9, 10, 11, 12, and 13), phage suspensions were incubated in LB broth with different pH values at 37°C for 1, 2, and 3 h and then assayed by the double-layered agar method. All assays were performed in triplicate.
Sequencing and Genome Analysis of Phage Swi3
Phage genomic DNA was extracted using a Virus Genome Extract DNA/RNA Kit (Tiangen, Inc., Beijing, China) according to the manufacturer’s instructions. Then, the extracted genomic DNA was sent to Biozeron Company (Shanghai, China) for high-throughput sequencing on an Illumina HiSeq platform and assembled with ABySS, v2.0.21. Contigs were assembled using the de novo assembly algorithm Newbler, version 3.0, with default parameters (Bao et al., 2014). Potential open reading frames were predicted and annotated using RAST2 and GeneMark3 (Besemer and Borodovsky, 2005; Aziz et al., 2008). Additionally, the genome sequence, the terminase large subunit, and the tail-associated protein amino acid sequence of phage swi3 were compared separately in the GenBank database of NCBI, and all homologous phages were selected to construct the phylogenetic tree of phage swi3 using the neighbor-joining method with default parameters in MEGAX software. The genomes of phage swi3, Salmonella phage BP63 (KM366099.1), Salmonella phage LSE7621 (MK568062.1), Salmonella phage UPF_BP2 (NC_048649.1), and Salmonella phage vB_SenM_PA13076 (MF740800.1) were compared and performed using Mauve software with a default parameter (Darling et al., 2004).
Antibacterial Activity of Phage Swi3 in a Mouse Model Challenged With E. coli K88
To test the antibacterial activity of phage swi3, the 50% lethal dose (LD50) of E. coli K88 was first determined. In brief, 28 5-week-old female BALB/c mice were randomly divided into four groups. Each group was treated orally with different doses of E. coli K88 (106, 107, 108, and 109 CFU). The LD50 was determined based on the mortality of mice and used in subsequent challenge tests. To test the protective effect of phage swi3 on mice, 42 5-week-old female BALB/c mice were randomly divided into six groups (a–f). Groups a–d were inoculated orally with 108 CFU of E. coli K88. Then, groups a–c were inoculated intraperitoneally with a single dose (105, 106, and 107 PFU, respectively) of phage swi3 at 2 h after the bacterial challenge. Control group d was inoculated with an equivalent volume of LB instead of phage swi3, control group e was treated with 107 PFU of phage swi3 without E. coli K88 challenge, and control group f only took an equal volume of LB orally. Then, the mice were observed daily for 5 days to record the clinical signs and cumulative mortality. At the same time, blood and feces were collected every 2 h on the first day and every 24 h after the first day to detect the metabolic dynamics of bacteria and phage swi3 in the mice.
Nucleotide Sequence Accession Number
The sequence data of swi3 were deposited at GenBank under accession number MT768059.
Results
Morphology of Phage Swi3
A novel virulent phage was isolated from E. coli K88 and observed by transmission electron microscopy. Phage swi3 has a regular icosahedral head (80 nm in diameter) and a contractile sheathed tail 120 nm in length (Figure 1). Thus, phage swi3 belonged to the family Myoviridae, order Caudovirales, following the current guidelines of the International Committee on Taxonomy of Viruses (ICTV). According to the novel universal system of bacteriophage naming, the suggested full name of phage swi3 will be vB_EcoM_swi3 (Lavigne et al., 2009).
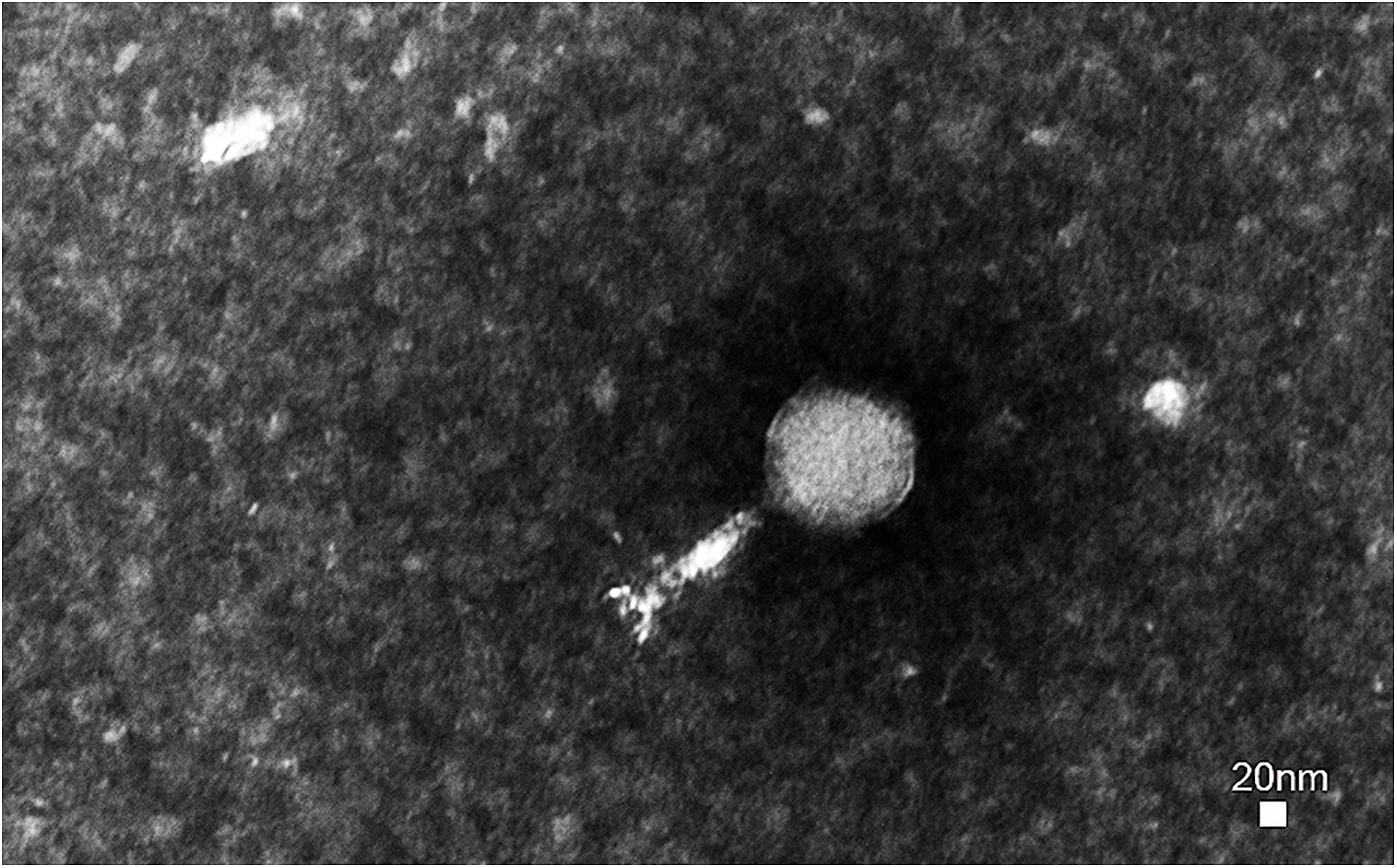
Figure 1. Morphology of phage swi3. Phage swi3 was negatively stained with 2% uranyl acetate and observed by transmission electron microscopy at an accelerating voltage of 80 kV. The scale bars represent 20 nm.
Host Range of Phage Swi3
The swi3 host range was determined using 65 E. coli strains and 72 S. enteritidis strains (Supplementary Table 1). Interestingly, phage swi3 showed characteristics of a wide range across species, not only having a certain lytic ability for six of the 65 E. coli strains but also having a lytic ability for 39 of the 72 S. enteritidis strains.
The Growth of Phage Swi3
The biological characteristics of phage swi3 were measured by the double-layer plate method (Zhang et al., 2013). When the MOI was 1, phage swi3 had the highest titer of 6.4 × 108 PFU/ml after proliferation. The one-step growth analysis showed that the incubation period of swi3 was approximately 25 min, after which there was a rapid increase in the number of released viral particles. It took about 75 min for swi3 to reach the growth plateau phase with a burst size of approximately 25 PFU/infected cells (Figure 2A). In addition, phage swi3 remained stable in the pH range of 6–8 (Figure 2B) and at a temperature less than 50°C for at least 1 h (Figure 2C).
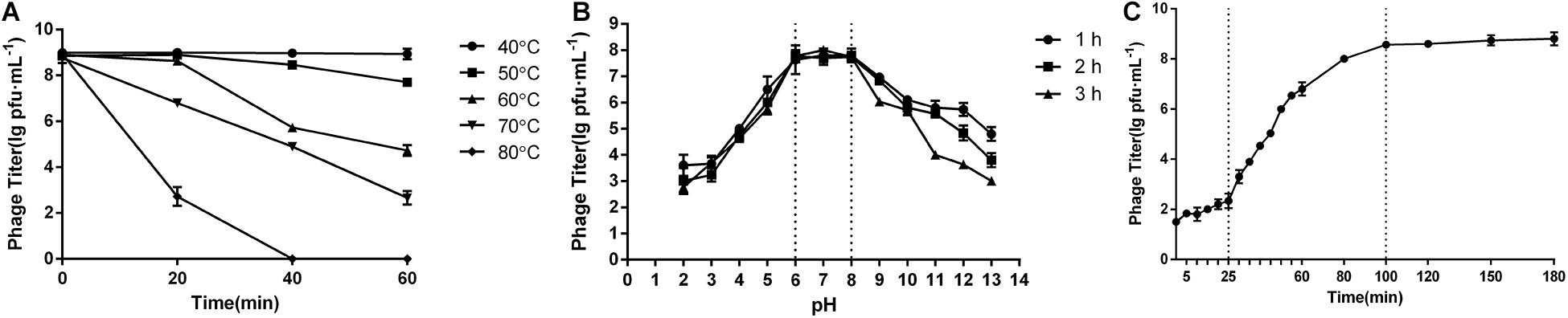
Figure 2. Biological characteristics of phage swi3. (A) One-step growth curve, (B) pH stability, and (C) thermal stability. Data are expressed as means. At the optimal multiplicity of infection of 1, the one-step growth analysis of swi3 showed a 25-min latent period with a burst size of 25-plaque-forming units (PFU)/infected cell. Phage swi3 remained stable both at pH 6.0–8.0 and less than 50°C for at least 1 h.
Genome Analysis of Phage Swi3
The genome of phage swi3 was sequenced and analyzed. The general characteristics of the genome include a total of 52,782 bp with an overall GC content of 46.02%. Seventy-two orfs were predicted, 39 of which were positive-stranded, while the others were negative-stranded. Only 15 of 72 orfs were annotated as functional genes, including six structural-related genes (orf 19, orf 20, orf 21, orf 22, orf 27, and orf 33), seven genes associated with transcription and replication (orf 14, orf 18, orf 52, orf 53, orf 57, orf 59, and orf 72), and two lysis-related genes (orf 44 and orf 45). A detailed phage swi3 genome annotation showed that the orfs related to transcription and replication were mainly concentrated in the downstream part of the whole genome, while the structurally related orfs were mainly concentrated in the upstream part of the whole genome sequence (Figure 3).
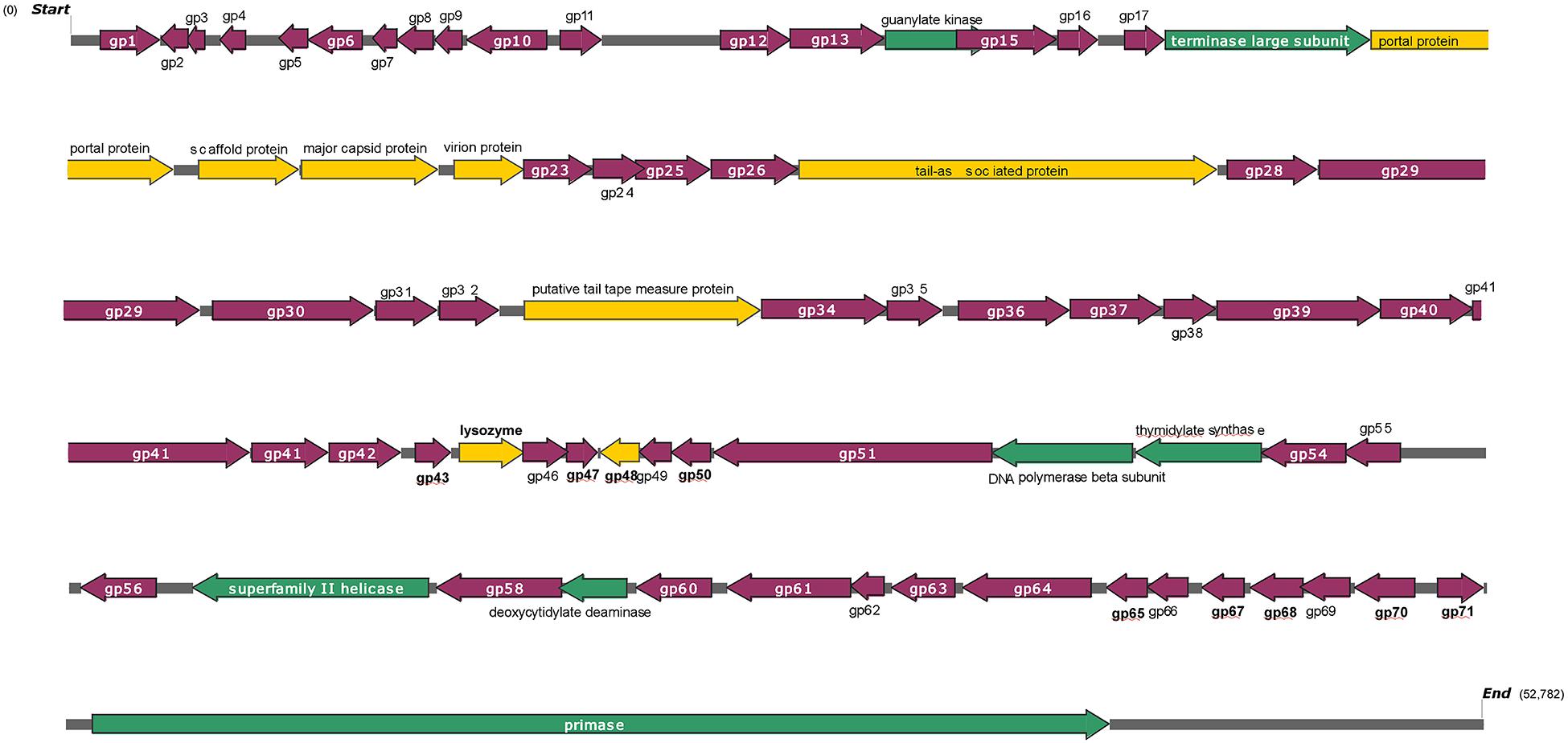
Figure 3. Genome structure of phage swi3. The arrow represents the direction of transcription. Different colors represent open reading frames with different predictive functions. The structural proteins are represented in yellow, blue indicates cleavage-related proteins, the proteins associated with transcriptional transcription are expressed in green, and hypothetical proteins are represented in purple.
Phylogenetic Analysis of Phage Swi3
The phylogenetic tree of phage swi3 was constructed based on the genomic sequence, the terminase large subunit (encoded by orf 19) sequence, the tail-associated protein (encoded by orf 27), and the tail tape measure protein (encoded by orf 33), using the neighbor-joining method with the default parameters in MEGAX software.
Based on the terminase large subunit, most comparable phages had less than 60% homology, a total of 10 phages in NCBI showed high similarities (>94%), and all of them belonged to Salmonella phages. Only Salmonella virus BP63 (KM366099.1) was included in ICTV, with 98% coverage rate and 97.73% identity. The phylogenetic tree showed that 11 phages belonged to two groups, phage swi3 and eight more phages belonged to the Rosemountvirus genus, and the other two phages belonged to the Loughboroughvirus genus (Supplementary Figure 1). Based on the whole-genome sequence, the phylogenetic tree was highly consistent with that of the terminase large subunit; all homologous phages were divided into two groups, and phage swi3 belonged to the Rosemountvirus genus. Additionally, all the homologous phages were Salmonella phages (Supplementary Figure 2). The genomic comparison results showed that no rearrangement or inversion occurred in the phage swi3 genome (Supplementary Figure 3).
Only two tail-related proteins were annotated among the known orfs: the tail-associated protein (encoded by orf 27) and the tail tape measure protein (encoded by orf 33). Their phylogenetic trees were constructed. Based on the tail-associated protein, only four comparable phages were found in the NCBI database with a high homology (>82.7%), and all of them were Salmonella phages. Other phages in the database showed less than 45% homology (Supplementary Figure 4). Based on the tail tape measurement protein, all homologous phages also belonged to Salmonella phages with more than 70% similarity (Supplementary Figure 5).
Protective Effects of Phage Swi3 in a Mouse Model Challenged With E. coli K88
The LD50 of E. coli K88 on mice was determined as 108 CFU, and the protective effects of phage swi3 were tested in a mouse model challenged with E. coli K88. There were obvious protective effects of phage swi3 in the bacteria-challenged mouse model (p < 0.05). After the challenge with E. coli K88, all mice in control group d died within 1 day; their lungs, liver, spleen, and kidneys showed varying degrees of bleeding and swelling, and E. coli K88 was isolated from these diseased organs. All mice in control groups f and e had good health until the end of the experiment, and no organ lesions were found (Supplementary Figure 6). In groups a–c, different doses of phage swi3 showed a good protective effect on E. coli K88-challenged mice. Compared with control group d, except for one mouse in group a that died on the second day, all mice survived, and no organ lesions were found (Figure 4A). The results indicated that a single intraperitoneal injection of swi3 (106 PFU) at 2 h after oral challenge with E. coli K88 could sufficiently protect all mice without toxic side effects.
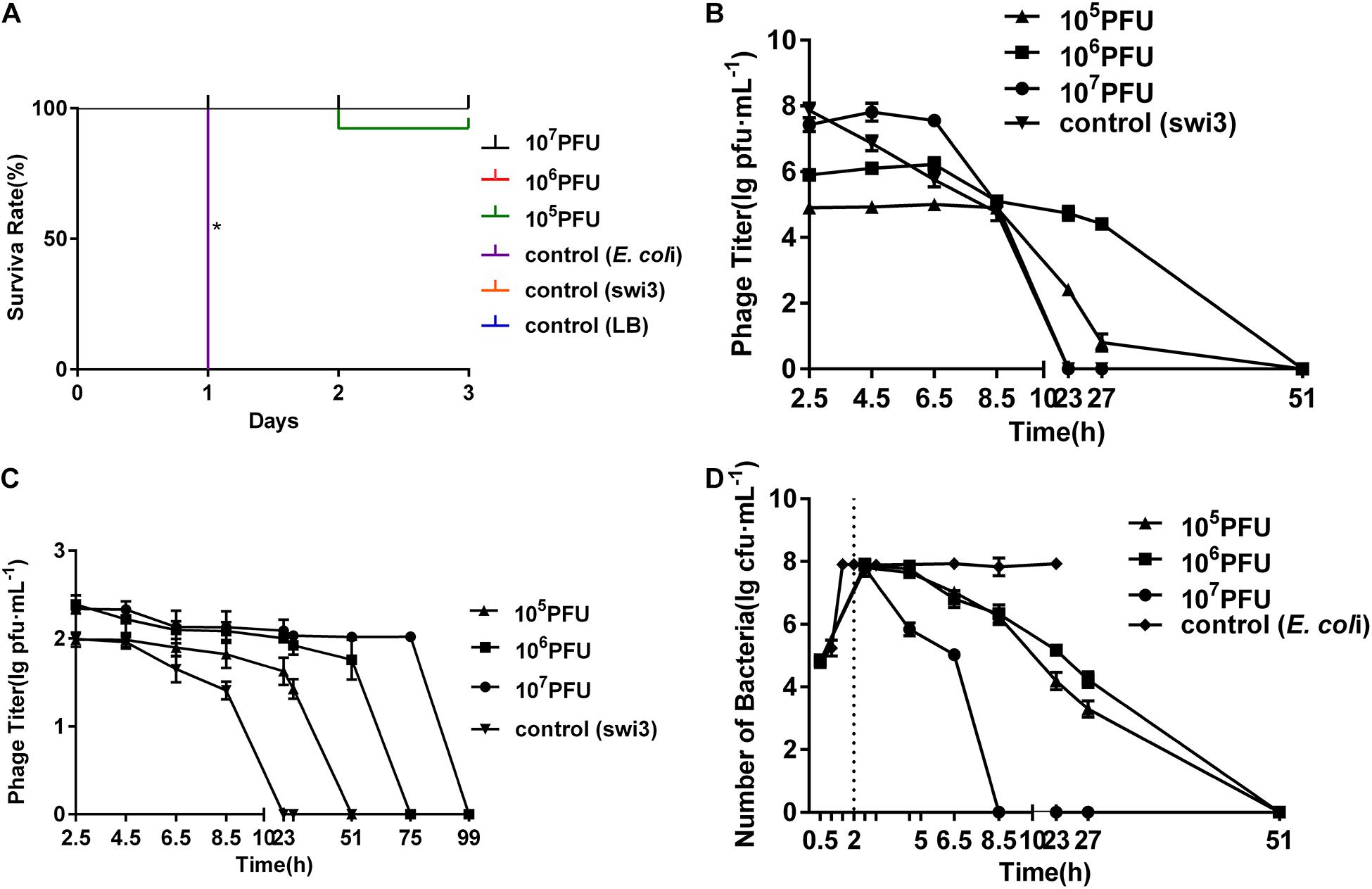
Figure 4. Phage swi3 rescued mice from Escherichia coli K88 infection. (A) Survival rates. The mice were inoculated orally with 108 colony-forming units of the E. coli K88 strain. The data were expressed as means ± SD and statistically analyzed using the one-way ANOVA method at a level of significance *p < 0.05 (GraphPad Software, Inc., San Diego, CA, United States). At 2 h later, different phage doses were introduced intraperitoneally to treat the challenged mice. (B) Dynamic changes of bacteria in blood in different groups. (C) Dynamic changes of phage in blood in different groups. (D) Dynamic changes of phage in feces in different groups.
In addition, the dynamics of bacteria and phages in the blood and feces were measured. E. coli K88 entered the blood and reached its peak (108 CFU/ml) 1.5 h after inoculation. Phage swi3 was intraperitoneally injected into the mice, detected in the blood in a short time, and then cleared E. coli K88 within 8.5–51 h in the blood; no bacteria or phage could be detected after 51 h (Figures 4B,C). Meanwhile, the dynamics of phage swi3 in feces were also measured. With the increase in phage inoculation dose, the phage detection time in feces was prolonged, ranging from 23 to 99 h (Figure 4D).
Discussion
Escherichia coli and Salmonella enteritidis are becoming increasingly important opportunistic pathogens worldwide that endanger animal breeding industries. The difficulties in treating multidrug-resistant strains and the specificity of phage therapy prompted researchers to focus on phage therapy. In this study, we isolated a novel lytic phage swi3 from swine feces, which showed a broad host range against multidrug-resistant E. coli and S. enteritidis.
Generally, bacteriophages show strong host specificity and usually display a species-limited host range. To our knowledge, few phages infecting more than one species of bacteria have been reported (Park et al., 2012; Amarillas et al., 2016; Pham-Khanh et al., 2019). In this study, phage swi3 was isolated from E. coli k88, but the phylogenetic tree analysis results based on the whole-genome sequence and the terminase large subunit showed that all phages with homology in the database were S. enteritidis phages, and the tail-associated protein and the tail tape measure protein were also S. enteritidis phages, which attracted our attention. In general, the host range of phages is related to their genomes. Therefore, two tail-related proteins were selected for BLAST analysis with the sequences in the database. The phylogenetic tree based on the tail-associated protein and the tail tape measure protein also showed a homology with the S. enteritidis phages. Thus, we hypothesized that phage swi3 might be able to lyze S. enteritidis phages. To test our hypothesis, S. enteritidis strains were selected to detect the host range of phage swi3, and 39 of 72 strains could be lyzed. Therefore, the phage swi3 could lyze not only E. coli but also S. enteritidis. The verification test showed that the host bacterial species of the phage could be preliminarily inferred through homology analysis, which was helpful to determine the phage host spectrum. It was speculated that the interspecific recognition mechanism of phage swi3 might be related to its infection process. The phage swi3 belonged to Myoviridae, which first bound to the corresponding receptor on the surface of the host bacteria through the receptor binging protein (RBP) on the tail fiber so that the phage adsorbed to the surface of the host bacteria and then initiated the process of phage infection (Bertozzi Silva et al., 2016). The phage swi3 could lyze Salmonella and E. coli, so its RBP needed to recognize receptors not only on the surface of E. coli but also on the surface of S. enteritidis. In addition, it was possible that the unknown orf-encoded proteins of phage swi3 were involved in the host adsorption process, which requires further analysis and verification. Phage swi3 could infect E. coli and S. enteritidis efficiently and showed a wide host range, which made it suitable for use as a biological control agent.
In the past 10 years, the application of phages in the prevention and control of bacterial diseases in animal reproduction has been widely reported. The results of animal experiments in vivo are different, but they all showed that phage preparations have no toxicity or side effects on the animal body, and due to the characteristics of phage proliferation with the host bacteria, a low-dose phage preparation can have a good bactericidal effect (Schneider et al., 2018; Balasubramanian et al., 2019; Kaabi and Musafer, 2019). In this study, a mouse model challenged with E. coli K88 was used to test the protective effect of phage swi3. The results showed that a low dose of 106 PFU of phage swi3 could protect mice from E. coli attack without any obvious side effects, and the phage was cleared within a short period of time because of the animal immune clearance response, which was consistent with previous reports (Li et al., 2015).
In conclusion, phage swi3 had a broad host spectrum including E. coli and S. enteritidis; it can clear bacteria in animals within a short time without side effects and has a potential value in the treatment of bacterial diseases.
Data Availability Statement
The datasets presented in this study can be found in online repositories. The names of the repository/repositories and accession number(s) can be found below: https://www.ncbi.nlm.nih.gov/genbank/, MT768059.
Ethics Statement
The animal study was reviewed and approved by the Animal Welfare and Research Ethics Committee at Qingdao Agriculture University.
Author Contributions
BS performed the experiments, analyzed the data, and wrote this manuscript. LH, HR, and WL performed the experiments. CZ designed the experiments and revised the manuscript. All authors contributed to the article and approved the submitted version.
Funding
This work was supported by the National Key R&D Program of China (2018YFD0501403) and the Shandong Key Research and Development Program (2019GNC106108).
Conflict of Interest
The authors declare that the research was conducted in the absence of any commercial or financial relationships that could be construed as a potential conflict of interest.
Supplementary Material
The Supplementary Material for this article can be found online at: https://www.frontiersin.org/articles/10.3389/fmicb.2021.649673/full#supplementary-material
Footnotes
- ^ http://www.bcgsc.ca/platform/bioinfo/software/abyss
- ^ http://rast.nmpdr.org
- ^ http://opal.biology.gatech.edu/GeneMark/
References
Abedon, S. T. (2016). Phage therapy dosing: the problem(s) with multiplicity of infection (MOI). Bacteriophage 6:e1220348. doi: 10.1080/21597081.2016.1220348
Akhwale, J. K., Rohde, M., Rohde, C., Bunk, B., Sproer, C., Boga, H. I., et al. (2019). Isolation, characterization and analysis of bacteriophages from the haloalkaline lake Elmenteita, Kenya. PLoS One 14:e0215734. doi: 10.1371/journal.pone.0215734
Amarillas, L., Chaidez, C., Gonzalez-Robles, A., and Leon-Felix, J. (2016). Complete genome sequence of new bacteriophage phiE142, which causes simultaneously lysis of multidrug-resistant Escherichia coli O157:H7 and Salmonella enterica. Stand. Genomic Sci. 11:89. doi: 10.1186/s40793-016-0211-5
Aziz, R. K., Bartels, D., Best, A. A., DeJongh, M., Disz, T., Edwards, R. A., et al. (2008). The RAST Server: rapid Annotations using Subsystems Technology. BMC Genomics 9:75. doi: 10.1186/1471-2164-9-75
Balasubramanian, S., Osburne, M. S., BrinJones, H., Tai, A. K., and Leong, J. M. (2019). Prophage induction, but not production of phage particles, is required for lethal disease in a microbiome-replete murine model of enterohemorrhagic E. coli infection. PLoS Pathog. 15:e1007494. doi: 10.1371/journal.ppat.1007494
Bao, E., Jiang, T., and Girke, T. (2014). AlignGraph: algorithm for secondary de novo genome assembly guided by closely related references. Bioinformatics 30, i319–i328. doi: 10.1093/bioinformatics/btu291
Bertozzi Silva, J., Storms, Z., and Sauvageau, D. (2016). Host receptors for bacteriophage adsorption. FEMS Microbiol. Lett. 363:fnw002. doi: 10.1093/femsle/fnw002
Besemer, J., and Borodovsky, M. (2005). GeneMark: web software for gene finding in prokaryotes, eukaryotes and viruses. Nucleic Acids Res. 33, W451–W454.
Chang, R. Y. K., Wallin, M., Lin, Y., Leung, S. S. Y., Wang, H., Morales, S., et al. (2018). Phage therapy for respiratory infections. Adv. Drug Deliv. Rev. 133, 76–86. doi: 10.1016/j.addr.2018.08.001
Chibani-Chennoufi, S., Bruttin, A., Dillmann, M. L., and Brussow, H. (2004). Phage-host interaction: an ecological perspective. J. Bacteriol. 186, 3677–3686. doi: 10.1128/JB.186.12.3677-3686.2004
Darling, A. C., Mau, B., Blattner, F. R., and Perna, N. T. (2004). Mauve: multiple alignment of conserved genomic sequence with rearrangements. Genome Res. 14, 1394–1403. doi: 10.1101/gr.2289704
Ding, T., Sun, H., Pan, Q., Zhao, F., Zhang, Z., and Ren, H. (2020). Isolation and characterization of Vibrio parahaemolyticus bacteriophage vB_VpaS_PG07. Virus Res. 286:198080. doi: 10.1016/j.virusres.2020.198080
Jajere, S. M. (2019). A review of Salmonella enterica with particular focus on the pathogenicity and virulence factors, host specificity and antimicrobial resistance including multidrug resistance. Vet. World 12, 504–521. doi: 10.14202/vetworld.2019.504-521
Kaabi, S. A. G., and Musafer, H. K. (2019). An experimental mouse model for phage therapy of bacterial pathogens causing bacteremia. Microb. Pathog. 137:103770. doi: 10.1016/j.micpath.2019.103770
Kreienbaum, M., Dorrich, A. K., Brandt, D., Schmid, N. E., Leonhard, T., Hager, F., et al. (2020). Isolation and Characterization of Shewanella Phage Thanatos Infecting and Lysing Shewanella oneidensis and Promoting Nascent Biofilm Formation. Front. Microbiol. 11:573260. doi: 10.3389/fmicb.2020.573260
Lavigne, R., Darius, P., Summer, E. J., Seto, D., Mahadevan, P., Nilsson, A. S., et al. (2009). Classification of Myoviridae bacteriophages using protein sequence similarity. BMC Microbiol. 9:224. doi: 10.1186/1471-2180-9-224
Li, Z., Venegas, V., Nagaoka, Y., Morino, E., Raghavan, P., Audhya, A., et al. (2015). Necrotic Cells Actively Attract Phagocytes through the Collaborative Action of Two Distinct PS-Exposure Mechanisms. PLoS Genet. 11:e1005285. doi: 10.1371/journal.pgen.1005285
Lu, L., Cai, L., Jiao, N., and Zhang, R. (2017). Isolation and characterization of the first phage infecting ecologically important marine bacteria Erythrobacter. Virol. J. 14:104. doi: 10.1186/s12985-017-0773-x
Majowicz, S. E., Musto, J., Scallan, E., Angulo, F. J., Kirk, M., O’Brien, S. J., et al. (2010). The Global Burden of NontyphoidalSalmonellaGastroenteritis. Clin. Infect. Dis. 50, 882–889. doi: 10.1086/650733
Paitan, Y. (2018). Current Trends in Antimicrobial Resistance of Escherichia coli. Curr. Top. Microbiol. Immunol. 416, 181–211. doi: 10.1007/82_2018_110
Parikka, K. J., Le Romancer, M., Wauters, N., and Jacquet, S. (2017). Deciphering the virus-to-prokaryote ratio (VPR): insights into virus-host relationships in a variety of ecosystems. Biol. Rev. Camb. Philos. Soc. 92, 1081–1100. doi: 10.1111/brv.12271
Park, M., Lee, J. H., Shin, H., Kim, M., Choi, J., Kang, D. H., et al. (2012). Characterization and comparative genomic analysis of a novel bacteriophage, SFP10, simultaneously inhibiting both Salmonella enterica and Escherichia coli O157:H7. Appl. Environ. Microbiol. 78, 58–69. doi: 10.1128/AEM.06231-11
Pham-Khanh, N. H., Sunahara, H., Yamadeya, H., Sakai, M., Nakayama, T., Yamamoto, H., et al. (2019). Isolation, Characterisation and Complete Genome Sequence of a Tequatrovirus Phage, Escherichia phage KIT03, Which Simultaneously Infects Escherichia coli O157:H7 and Salmonella enterica. Curr. Microbiol. 76, 1130–1137. doi: 10.1007/s00284-019-01738-0
Poirel, L., Madec, J.-Y., Lupo, A., Schink, A.-K., Kieffer, N., Nordmann, P., et al. (2018). Antimicrobial Resistance in Escherichia coli. Microbiol. Spectr. 6, 289–316. doi: 10.1128/microbiolspec.ARBA-0026-2017
Rehman, S., Ali, Z., Khan, M., Bostan, N., and Naseem, S. (2019). The dawn of phage therapy. Rev. Med. Virol. 29:e2041. doi: 10.1002/rmv.2041
Salmond, G. P. C., and Fineran, P. C. (2015). A century of the phage: past, present and future. Nat. Rev. Microbiol. 13, 777–786. doi: 10.1038/nrmicro3564
Schneider, G., Szentes, N., Horváth, M., Dorn, Á, Cox, A., Nagy, G., et al. (2018). Kinetics of Targeted Phage Rescue in a Mouse Model of Systemic Escherichia coli K1. BioMed Res. Int. 2018:7569645. doi: 10.1155/2018/7569645
Sun, W. J., Liu, C., Yu, L., Cui, F. J., Qiang, Z., Yu, S. L., et al. (2012). A novel bacteriophage KSL-1 of 2-Keto-gluconic acid producer Pseudomonas fluorescens K1005: isolation, characterization and its remedial action. BMC Microbiol. 12:127. doi: 10.1186/1471-2180-12-127
Thames, H. T., and Theradiyil Sukumaran, A. (2020). A Review of Salmonella and Campylobacter in Broiler Meat: emerging Challenges and Food Safety Measures. Foods 9:776. doi: 10.3390/foods9060776
Yang, S. C., Lin, C. H., Aljuffali, I. A., and Fang, J. Y. (2017). Current pathogenic Escherichia coli foodborne outbreak cases and therapy development. Arch. Microbiol. 199, 811–825. doi: 10.1007/s00203-017-1393-y
Yu, M. X., Slater, M. R., and Ackermann, H. W. (2006). Isolation and characterization of Thermus bacteriophages. Arch. Virol. 151, 663–679. doi: 10.1007/s00705-005-0667-x
Keywords: bacteriophage vB_EcoM_swi3, Escherichia coli, Salmonella enteritidis, biological characteristics, genomic analysis, phage therapy
Citation: Sui B, Han L, Ren H, Liu W and Zhang C (2021) A Novel Polyvalent Bacteriophage vB_EcoM_swi3 Infects Pathogenic Escherichia coli and Salmonella enteritidis. Front. Microbiol. 12:649673. doi: 10.3389/fmicb.2021.649673
Received: 13 January 2021; Accepted: 17 May 2021;
Published: 14 July 2021.
Edited by:
Krishna Mohan Poluri, Indian Institute of Technology Roorkee, IndiaReviewed by:
Ahmed Askora, Zagazig University, EgyptHeejoon Myung, Hankuk University of Foreign Studies, South Korea
Copyright © 2021 Sui, Han, Ren, Liu and Zhang. This is an open-access article distributed under the terms of the Creative Commons Attribution License (CC BY). The use, distribution or reproduction in other forums is permitted, provided the original author(s) and the copyright owner(s) are credited and that the original publication in this journal is cited, in accordance with accepted academic practice. No use, distribution or reproduction is permitted which does not comply with these terms.
*Correspondence: Can Zhang, Y2xldmVyZmxhbWVAMTYzLmNvbQ==