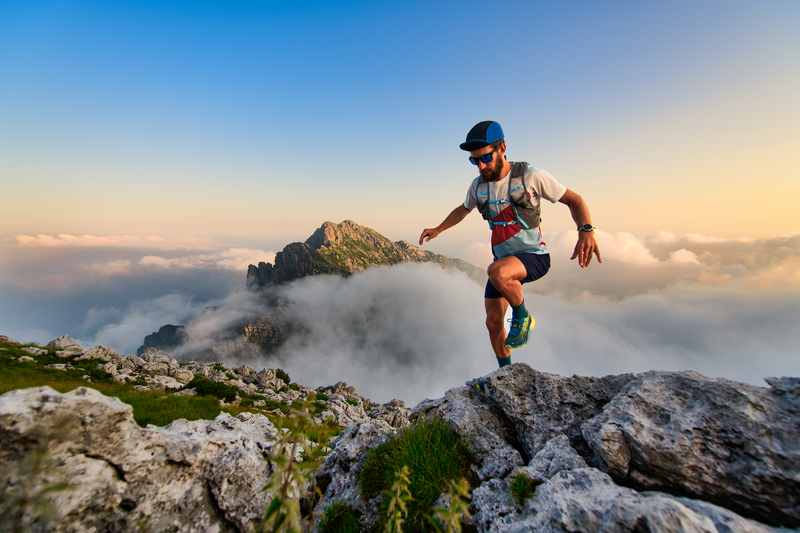
95% of researchers rate our articles as excellent or good
Learn more about the work of our research integrity team to safeguard the quality of each article we publish.
Find out more
ORIGINAL RESEARCH article
Front. Microbiol. , 30 April 2021
Sec. Microbiotechnology
Volume 12 - 2021 | https://doi.org/10.3389/fmicb.2021.647766
This article is part of the Research Topic Microplastics and Microorganisms in the Environment View all 9 articles
Microplastics (MPs) have been attracting wide attention. Biochar (BC) application could improve the soil quality in the contaminated soil. Currently, most studies focused on the effect of MPs or BC on the soil properties and microbial community, while they neglected the combined effects. This study investigated the combined effects of BC or ball-milled BC (BM) and polyethylene plastic fragments (PEPFs) and degradable plastic fragments (DPFs) on the removal of polycyclic aromatic hydrocarbons (PAHs) and phthalate esters (PAEs) from the PAH-contaminated soil and the potential microbial ecological mechanisms. The results showed that BC or BM combined with PEPF could accelerate the removal of PAHs and PAEs. PEPF combined with BM had the most significant effect on the removal of PAHs. Our results indicating two potential possible reasons contribute to increasing the removal of organic pollutants: (1) the high sorption rate on the PEPF and BC and (2) the increased PAH-degrader or PAE-degrader abundance for the removal of organic pollutants.
Plastic is used in a wide range of products (Plastics Europe, 2018/2019) due to its low cost, light weight, and durability (Cozar et al., 2014). The extensive use of plastics resulted in the release of plastic waste into the environment at an alarming rate (Geyer et al., 2017). Larger plastics break into the smaller pieces, resulting in the formation of microplastics (MPs) (Barnes et al., 2009; Rillig, 2012). The concept of MPs (<5 mm) was proposed by Thompson (2004), characterized by small size, large amount, wide distribution (Rillig, 2012), and the adsorption characteristics (Law and Thompson, 2014). MPs are prone to absorb the pollutants on its surface (Besseling et al., 2013; Hodson et al., 2017) or accumulate the specific microbes such as pathogens (Foulon et al., 2016), thus posing potential risks to the ecosystem through the food web and food chain (Browne et al., 2013; Wright et al., 2013).
One of the main MP sources in soil is plastic film. Previous studies showed that plastic could reach 40–60% of the surface of home gardens soil (Huerta Lwanga et al., 2016). The total amount MPs of farmland in North America and the plastic content on the surface soil of the solid waste enrichment region in Germany could reach 44,000–300,000 and 43,454 t⋅a–1 (Ng et al., 2018), respectively. The degradable plastic fragments (DPF) has drawn wide concern recently due to the possibility of replacing traditional plastic film, although its effects on ecosystem in the short- or long-term are still not fully understood (Bandopadhyay et al., 2018). A previous study showed that both polyethylene (PE) MPs and DPF-MPs could affect soil carbon and nitrogen content (Qi et al., 2020). The additives used in the plastic production process such as phthalate esters (PAEs) could also pose a serious problem to the soil ecosystem after being released into the soil (Wang et al., 2013). Kong et al. (2012) showed that vegetable soil had the highest PAE value, indicating that PAEs in plastic film could enter into the soil. In addition, MPs are high-molecular polymers with characteristics such as hydrophobic properties and high octanol–water partition coefficient (Kow), thus making it easy for them to adsorb hydrophobic organic compounds (HOCs) (Rillig, 2012; Horton et al., 2017). MPs could accumulate microbes such as Actinobacteria, Bacteroidetes, and Proteobacteria, which could degrade the polymer (Zhang et al., 2019), polycyclic aromatic hydrocarbons (PAHs), and dioxins (Gatheru Waigi et al., 2017). MPs could also affect some microorganisms involved in the biogeochemical cycle, such as Burkholderiaceae, Xanthobacteraceae, and Pseudomonadaceae (Kielak et al., 2016), and could raise the abundance of Bradyrhizobiaceae and Burkholderiaceae, which associated with nitrogen fixation (Fei et al., 2020) and changed the soil nutrient condition. Therefore, study on the effects of MPs on the structure and function of soil microbial community could help us deeply and comprehensively understand the fate of contaminants in the environment and the potential mechanism.
Biochar (BC) could improve the soil quality and enhance the adsorption and degradation of pollutants to reduce the microbial toxicity of the pollutants, so it is commonly used for the improvement of organic contaminated soil, although the effects of BC on degradation of organic pollutants showed mixed results currently (He et al., 2018). Some studies showed that BC could accelerate the degradation rate of organic pollutants as a result of enhancing the microbial activity or the microbial utilization of the organic pollutants on the BC. BC has direct or indirect effects on microorganisms (Zhu et al., 2017). BC could directly provide protection and nutrient to the soil microorganisms (Jia et al., 2017; Luo et al., 2018) or provide the microbes a more suitable living environment by changing the soil properties. In addition, BC could release dissolved organic carbon (DOC) into soil solution after application and could directly alter the properties of soil DOC (Dittmar et al., 2012). The DOC of BC is labile and more susceptible to biodegradation than BC; therefore, it plays an important role in controlling microbial activities (Wang B. et al., 2017; Bao et al., 2020). A recent study showed that soil DOC could be the main factor influencing the total relative abundances of dominant PAH-degraders (Ding et al., 2020), while the results of the other studies were opposite. During the preparation process of BC, PAHs could also be produced. Lyu et al. (2016) showed that BC produced at 300–400°C had higher PAHs or PCDD/DF toxicity than BC prepared at a temperature higher than 400°C. The mineral elements, volatile organic compounds (VOCs), and free radicals contained in BC can affect microbial activity and change the soil microbial community structure (Abel et al., 2013) and soil enzyme activity, which could further affect soil function. Therefore, the environmental and ecological effects caused by BC have drawn great concern (Wang C. et al., 2017).
Biochar is widely used as soil amendment; however, MP fragments exist in the soil at the same time, which could change the structure and function of the soil and microbial community (Ding et al., 2017; Rillig, 2018). The present study focused on the combined effects of MP fragments and BC on the soil physical and chemical properties, and the degradation of pollutants in PAH-polluted soil. We aimed to illustrate (1) the response of soil DOM and its components to the combined effects of plastic fragments and BC; (2) the influence of plastic fragments and BC on the removal of PAHs and PAEs; and (3) the potential microbial ecological mechanisms.
The PE plastic bags were purchased from a subsidiary agricultural product store in Tianjin, China. The degradable mulching film was obtained from an agricultural product store, composed of polylactic acid (PLA) and polybutylene adipate-co-terephthalate (PBAT). The film was cut into small pieces by using sharp blades and scissors of ∼4.5 mm in length. Before use, the fragments were sterilized under ultraviolet light for 20 min to minimize the potential microbial contamination. The BC used in this study was made from poplar woodchips. Before use, the collected woodchips were washed, dried, and pass through a 100 mesh sieve (Xiao et al., 2020). Then the woodchips were heated at 500°C in a muffle furnace for 3 h under an oxygen-limited condition, cooled to room temperature, washed with distilled water, and dried at 80°C for 24 h. The yield of the BC was about 30.85%. The obtained BC was divided into two parts: one part was stored in the dark before use and labeled as BC, and the other one was used to prepare the ball-milled BC (BM) (Lyu et al., 2018; Xiao et al., 2020). The BC was placed in an agate jar (500 ml). The ratio of BC to agate ball was 1:50 (BC-to-ball mass ratios). The agate jar was sealed and placed into a planetary ball mill machine (F-P2000, Focucy, Hunan, China). The ball milling was performed at a speed of 200 rpm for 24 h at room temperature; and the rotation direction was changed every 6 h. The resulting BC was labeled as BM.
The contents of C, H, and N of the BC were determined by an element analyzer (vario EL CUBE, Germany). The contents of C, H, and N were 75.74, 5.12, and 0.78% for BC and 71.79, 3.88, and 0.84% for BM. The morphologies of BC and BM were characterized by a field-emission scanning electron microscope (SEM; JSM-7800F, Rigaku, Tokyo, Japan). The specific surface areas, pore volume, and pore size of BC and BM were determined by Micromeritics ASAP2460 (Atlanta, United States) based on the N2 adsorption–desorption method. The data were analyzed according to the methods of Brunauer–Emmett–Tuller (BET) and Barrett–Joyner–Halenda (BJH). The Fourier-transform infrared (FT-IR) spectroscopy was used to analyze the surface functional groups on the surfaces of BC and BM by TENSOR 37 (Bruker, Germany) in the region of 400–4,000 cm–1 with a resolution of 4 cm–1.
The PAH-contaminated soil was brought back to the laboratory, air-dried, sieved through 2-mm mesh, and then thoroughly mixed for the subsequent microcosm experiment. The initial concentrations (Supplementary Table 1) and the structural formulas (Supplementary Figure 1) of 16 priority PAHs are listed in the Supplementary Material. Beakers measuring 350 ml were used in this study. Before use, the beakers were washed and sterilized. For each beaker, 300-g soil was mixed with the BC and MPs, resulting in nine different treatments (Supplementary Table 2). BC, BM, D, and P represented BC, BM, degradable plastic fragments (DPFs), and PE plastic fragments (PEPFs). After water was added [equivalent to the 60% of the field capacity (w/w)], the samples were incubated at 22°C (80% relative humidity). Each treatment was carried out in three replicates. Samples from each pot were collected on the 105th day of the experiment. The samples were separated into two parts: one part was stored in the refrigerator for testing soil physical and chemical properties, and the other part was stored at −80°C for molecular microbiological analysis.
Soil DOM solution was extracted by adding deionized water (ratio of soil:water was 1:5; 8-g soil with 40 ml of deionized water) in a 50-ml centrifuge tube according to the method described in the previous studies (Jaffrain et al., 2007; Ren et al., 2020). Soil extracts were centrifuged at 3,500 rpm for 15 min and then filtered by pre-rinsed 0.45-μm cellulose-acetate membranes (Solarbio, Beijing, China). The filtered solutions were analyzed by multi N/C 3100 (Analytik Jena AG, Germany) for DOM analysis. The functional groups of the subsamples were measured by UV-Vis spectrophotometer (LAMBDA-35, PerkinElmer, United States). UV-Vis absorption from 200 to 500 nm (1-nm steps) was measured in a 10-mm quartz cuvette with deionized water as blank. The specific UV absorbance (SUVA) at 210, 250, 254, 260, 265, 272, 280, 285, 300, 340, 350, 365, 400, 436, and 465 nm was measured for all samples. Detailed information as well as the wavelengths used in this study and their corresponding organic functional groups is shown in Supplementary Table 3. The soil NO3––N was determined by UV spectrophotometry (TU-1810DASPC, PERSEE, Beijing, China) according to the method GB/T 32737-2016; the extracts were tested at 220 and 275 nm.
Quantification of 16 PAHs and six priority PAEs [di(2-ethylhexyl)phthalate (DEHP), dibutyl phthalate (DBP), dimethyl phthalate (DMP), diethyl phthalate (DEP), butyl phenyl phthalate (BBP), and di-n-octyl phthalate (DNOP)] in soil was carried out by gas chromatography–mass spectrometry (GC-MS; GC7890B/MS5977B) according to the method of US EPA 8270E-2018. Briefly, a 10-g soil sample was used for each sample and then placed into the extraction pool. After diatomite was added and acetone/hexane (1:1, v/v) was stirred and extracted according to accelerated solvent extraction (ASE) method, the sample was concentrated to 1 ml via rotary evaporation method. Then a 200-μl sample was put into the GC vial, and the internal standard was added and mixed evenly. The internal standard substances and their corresponding contents are listed in the Supplementary Table 4. Then analysis was carried out by GC-MS with DB-5MS capillary column at a flow of 1.5 ml.min–1. The samples were injected at 280°C without splitting injection. The GC oven temperature was held at 45°C for 3 min, then increased by 30°C.min–1 from 45°C to 280°C, and then followed by 10°C .min–1 from 280°C to 300°C; and then the temperature was held at 300°C for 4.5 min. The temperature of the ion source was set as 230°C.
Microbial community genomic DNA was extracted from soil samples using the FastDNA®, Spin Kit (MP Biomedicals, Santa Ana, CA, United States) according to manufacturer’s instructions. The extracted DNA was checked on 1% agarose gel electrophoresis, and the concentration and purity were determined using NanoDrop 2000 UV-Vis spectrophotometer (Thermo Scientific, Wilmington, United States). The primers containing barcode and adaptor used in this study are listed in Supplementary Table 5. The triplicate PCR products were recovered by using the AxyPrep DNA Gel Extraction Kit (Axygen Biosciences, Union City, CA, United States) following the manufacturer’s instructions and quantified using QuantusTM Fluorometer (Promega, United States). Purified amplicons were pooled in equimolar; and paired-end sequencing (2 × 250) was performed on an Illumina MiSeq platform (Illumina, San Diego, CA, United States) according to the standard protocols by Majorbio Bio-Pharm Technology Co. Ltd. (Shanghai, China). The detailed PCR method and bioinformatic analysis are listed in the Supplementary Material (the PCR mixtures and amplification conditions).
Statistical analyses were performed using IBM SPSS Statistics 24.0. One-way ANOVA was used to determine the effect of different treatments on PAHs, PAEs, and soil DOM. The means of significant effects at p < 0.05 were then compared using the Duncan multiple-range test. Each treatment was conducted in three replicates. The error bars in the figures were expressed as the standard deviation (SD); and all of the data in the figures are presented as means ± SD. Operational taxonomic unit (OTU)-level alpha diversity indices, Chao1 (Chao, 1984), abundance-based coverage estimators (ACEs) (Chao and Yang, 1993), and Shannon index (Shannon, 1948) were calculated using the OTU table in Mothur. Figures were visualized by R 3.6.1 (R Core Team, 2019) and RStudio 1.1.463 (RStudio Team, 2018). Package information and detailed data analysis method were listed in our previous study (Ren et al., 2020).
The SEM results of the original BC and BM are shown in Supplementary Figure 2. The surface of BC was rough and porous. The porous size was at the micron scale (Supplementary Figure 2A,C) and common in pyrolytic materials (Zhou et al., 2013). After ball milling, the BC became fine particles (diameter < 1 μm) (Supplementary Figures S2B,D), indicating that ball milling could effectively transform the BC into ultrafine particles.
Supplementary Table 6 shows the BET results. The specific surface area of the BC could be increased from 85.7 to 321.9 m2.g–1 after ball milling. The pore volume and average pore size of the BM were higher than those of BC. Previous studies have shown that ball milling technology could not only increase the external and internal surface area by reducing the size of the BC but could also increase the internal surface area by getting through the inner pore network.
The FT-IR spectra of the BC and BM are shown in Supplementary Figure 3. Before analysis, all samples were dried and kept dry to avoid the interference of moisture. Vibration peaks were observed at 3,443 cm–1 (–OH) and 1,385 cm–1 (O-C–O). After ball milling, the extension of BC shifted from 1,610 to 1,612 cm–1 at C-O. In addition, the peak appeared at 1,171 cm–1, indicating that the C–O functional groups increased after ball milling.
The DOC and total dissolved carbon (TC) had the same changing tendency (Figure 1). In soil without plastic fragments, the content of DOC and TC in CKBC (1% biochar + no adding plastics fragments) was significantly higher than that of CKN (no adding biochar + no adding plastics fragments) (p = 0.001; p = 0.002, LSD, p < 0.05), while in CKBM (1% ball-milled biochar + no adding plastics fragments), it was significantly lower than that of CKN (p = 0.036; p = 0.030, Least-Significant Difference (LSD), p < 0.05). The potential reason might be that BC could increase the soil DOC (Cui, 2021) with the higher adsorption capacity of ball milling BC (Kumar et al., 2020) due to the larger specific surface area after ball milling (Xiang et al., 2020). In soil with plastic fragments, both BC and BM decreased the DOC and TC. DN slightly increased the soil DOC compared with CKN, which might be due to its degradable characteristics, while PN decreased the soil DOC, which might be because the soil environment could change the surface of PEPF and increase the surface functional groups, resulting in the sorption of DOC on PEPF. A recent study showed that the co-existence of PE-MPs and BC could increase the sorption of ion (Li et al., 2021). A previous study showed that the soil DOC with rice straw and PLA-MPs was significantly lower than that in soil with rice straw alone when rice straw was used as the carbon source (Chen et al., 2020). Our result showed that the co-existence of BC and plastic fragments could significantly decrease the soil DOC compared with the soil with BC alone, which was similar to the previous results. As for the dissolved inorganic carbon (DIC), CKBC and DBC decreased its contents. The changing trends of total dissolved nitrogen (TN) and nitrate nitrogen (NO3––N) are shown in Figure 2. DBC (1% biochar + degradable plastic fragments) and DBM (1% ball-milled biochar + degradable plastic fragments) significantly decreased the content of TN (p = 0.024, p = 0.008, LSD, p < 0.05), while they had no significant effect on NO3––N compared with DN; PBM (1% ball-milled biochar + PE plastics fragments) significantly decreased the content of NO3––N compared with PN (p = 0.016, LSD, p < 0.05).
Figure 1. DOC, DIC, and TC concentration of different treatments. Different letters mean significant differences, and the same letters mean no significant difference, Duncan (p < 0.05). DOC, dissolved organic carbon; DIC, dissolved inorganic carbon; TC, total dissolved carbon.
Figure 3 and Supplementary Table 7 show the results of the SUVA at different wavelengths. PN significantly increased the content of amines (SUVA210) and aromatic substances (SUVA254, SUVA260, SUVA272, SUVA280, and SUVA285) compared with CKN, while it had no significant effect on the DOC molecular weight (A250/A365), aromatic ring substituents (A253/A203), the ratio of C-C and C-O (A265/A465), and the soil aggregation degree (A300/A400). DN had no significant influence on characteristic functional groups. DBC could change the components of DOC and significantly raise the contents of amines and aromatic substances as compared with CKBC, which might be because the degradable MPs could change the components of DOC (Chen et al., 2020).
Figure 3. Specific ultraviolet absorbance (SUVA) at different wavelengths: SUVA210, SUVA254, SUVA272, and A253/A203 of different treatments. SUVA210 means amines, SUVA254 and SUVA272 mean aromatic substances, and A253/A203 represent aromatic ring substituents.
Therefore, DBC significantly decreased DOC and increased the amines and aromatic substances, indicating that the combined effects could change the components of DOC. CKBM or DBM significantly increased the content of amines and aromatic substances as compared with CKN or DN, respectively. Compared with CKN, DN had no significant effect, while DBM could significantly decrease the DOC and increase the amines and aromatic substances, indicating that the combined effects could change the components of DOC (Figures 1, 3). PN could decrease the DOC as well as the contents of amines and aromatic substances, illustrating that PN could alter the components of DOC. PBC (1% biochar + PE plastics fragments) or PBM had similar results with PN. Therefore, the combined effect of DPF and BC could change the components of DOC, while the potential reason for PN changing the DOC contents was due to its own characteristics.
The changes of PAHs and PAEs in groups CKN, CKBC, CKBM, DBC, DBM, PBC, and PBM are shown in Tables 1, 2. The degradation rates of PAHs and PAEs are shown in Tables 3, 4, respectively. In this study, PAHs of CKBC and CKBM were higher than those in CKN. The contents of total PAHs of PBC and PBM were lower than those of the other groups. Therefore, PN combined with BC potentially accelerated the removal of total PAHs. As for the degradation rate, CKN had the highest degradation rate of PAHs (2 + 3) (Table 4). For PAHs (4), PBC had the highest degradation rate 61.75 ± 6.65%, followed by PBM 56.03 ± 8.32%, both of which had a significant difference with CKN (p = 0.002, p = 0.007, LSD, p < 0.05). The degradation rate of PAHs (5 + 6) in PBC and PBM could reach 77.10 ± 4.02% and 80.18 ± 3.87%, respectively. The total PAH degradation rates of PBC and PBM were 49.74 ± 8.43% and 45.09 ± 9.98%, which were higher than those of other treatments. Among the six priority controlled PAEs, DEHP and DBP were detected (Table 2). PAEs as the common pollutants in soil have a negative effect on soil enzyme and microbes and are often regarded as the “environmental endocrine-disrupting chemicals” (Xu et al., 2020). Our results showed that the contents of PAEs in CKBC and CKBM were significantly higher than those in CK. The contents of PAEs in CKBM were higher than CKBC. PAEs had a lower content in PBC and PBM than other groups. As for the degradation rate (Table 4), DBP, DEHP, and total PAE degradation rates in group PBC and PBM were significantly higher than those in the other groups.
A previous study suggested that the effects of BC on degradation of pollutants in soil showed mixed results (He et al., 2018): accelerating or reducing the degradation rate. There are several reasons for reducing the natural degradation rate by BC. Firstly, the pyrolysis process could introduce PAHs to BC, and the content of PAHs is correlated with the producing temperature, conditions, and type of raw materials (Keiluweit et al., 2012; Wang C. et al., 2017). Our results showed that the contents of PAHs in CKBC and CKBM were higher than those in CKN, which might be due to the PAHs produced in BC production process entering into the soil with BC (Table 1). Another potential reason was the adsorption characteristics of BC increasing the adsorption and immobilization of organic pollutants in soil resulting in the declined degradation. The strong sorption of organic pollutants on the carbonaceous materials could reduce their microbial degradation leading to high residues of the pollutants in the soil (Ding et al., 2020). Some studies also illustrated that BC showed the potential toxicity to microbes induced by the organic compounds on the BC (Zhu et al., 2017; Yaashikaa et al., 2020). These might be the potential possibilities for the PAHs and PAEs in CKBC and CKBM higher than those in CKN. In addition, the contents of PAHs and PAEs in CKBM were higher than those in CKBC. This might be due to its larger specific surface area resulting in more adsorption sites. The combined effects of PE-MPs and BC on the significant decrease of PAHs and PAEs in soil might be due to the sorption effect of PE-MPs on the organic pollutants (Jiménez-Skrzypek et al., 2021) or the selective effects on the specific microbes.
The microbial α-diversity indices and the Venn diagram of common and specific OTUs are shown in Figure 4 and Supplementary Table 8. The bacterial and fungal OTUs ranged from 200 to 517 and from 148 to 214, respectively. The common OTUs of bacterial and fungal community were 61 and 27, respectively. For bacteria, CKN had the highest specific OTU number (86), and the PBM had the lowest number (7). For fungi, CKN had the lowest number (31), and PBM had the highest number (65) (Figure 4). In soil without plastic fragments, BC decreased the bacterial OTUs (CK < CKBC < CKBM), while it increased the fungal OTUs (CK > CKBC > CKBM). Compared with CKN, the combined effects of plastic fragments and BC could decrease the OTUs of bacteria, while it increased those of fungi. A previous study showed that MPs could decrease the microbial α-diversity (Zhang et al., 2019). In our study, the result of bacterial community was consistent with the existing research results. In soil without BC, DN and PN decreased the Shannon and Chao indices of bacteria compared with CKN. Compared with CKN and CKBM, CKBC had the lowest values of bacterial diversity index, although CKBM had the higher diversity than CKBC, while it had a lower richness index. DBM and PBM increased the bacterial community diversity, while they decreased the richness. As for the fungi, DBM increased the diversity, while it decreased the richness; PBM decreased the diversity, while it increased the richness. The influences of BC on diversity and richness of the microbial community were affected by the types of MPs. CKBC and CKBM increased the richness of fungal community and CKBM increased its diversity index. Both DBC and PBC decreased the diversity and richness of fungi. DBM decreased the diversity and richness, and PBM decreased the diversity, while it increased the richness.
The microbial community structure at the family level is shown in Figure 5. The results showed that Pseudomonadaceae was the dominant bacteria in all the treatments. Compared with CKN, plastic fragments increased the abundance of Pseudomonadaceae, from 85.15% in CKN to 93.28% (DN) and 95.51% (PN), and decreased the abundance of Sphingomonadaceae, from 2.18% in CKN to 0.48% (DN) and 0.92% (PN). In soil with plastic fragments, BC had no significant influence on the abundance of Pseudomonadaceae, while BM decreased the abundance of Pseudomonadaceae and increased the abundance of Methylophilaceae, Bacillaceae, Burkholderiaceae, and Bogoriellaceae. The abundance of Pseudomonadaceae decreased from 93.28% in DN to 82.53% in DBM; the abundance of Methylophilaceae, Bacillaceae, Burkholderiaceae, and Bogoriellaceae increased from 0.34 to 4.84%, 0.29 to 3.35%, 0.60 to 2.02%, and 0.12 to 4.23% in DN and DBM. The abundance of Pseudomonadaceae decreased from 95.51% (PN) to 84.28% (PBM); the abundance of Methylophilaceae, Bacillaceae, Burkholderiaceae, and Bogoriellaceae increased from 0.90 to 4.93%, 0.16 to 5.96%, 0.63 to 1.10%, and 0.01 to 0.38% in PN and PBM. In soil with plastic fragments, BC had no significant influence on the abundance of the microbes. BM decreased the abundance of Pseudomonadaceae, while it increased Methylophilaceae, Bacillaceae, Burkholderiaceae, and Bogoriellaceae. The influence of DPF and PEPF was similar. Methylophilaceae played an important role in the removal of PAHs at the later stage (Lu et al., 2019). Some species in the family Burkholderiaceae have degradable genes (Oyehan and Al-Thukair, 2017).
As for the fungi, previous study showed that Fusarium had the assistant effect on the removal of PAHs (Rafin et al., 2009). Acremonium, Pleurotus ostreatus, Trichoderma, Trametes versicolor, and Pleurotus are associated with the removal of PAHs (Liu et al., 2017). PAHs can be immobilized by the particularity of fungal extracellular enzymes (Baldantoni et al., 2017). A previous study also showed that the content of PAHs decreased after adding fungi (Bellino et al., 2019). Therefore, the study of fungal community structure could help to further understand the mechanism of PAH degradation. The main fungal community at the family level included Cladosporiaceae, Trichocomaceae, Aspergillaceae, Nectriaceae, and Filobasidiaceae. Except for DBC, DBM, and PBM, the dominant family in other treatments was Cladosporiaceae. In addition, several researches showed that Fusarium in the family Hypocreaceae had a degradable effect on DPB (Wang et al., 2016; Cheng et al., 2018).
Our study showed that adding BC or ball milling BC to PAH-contaminated soil with plastic residual could reduce the content of PAHs in soil and accelerate its removal. PBM had the highest removal rate. The reason for the highest removal rate might be due to that combined effects that BM and plastic fragments had on the selective effect on the specific microbes associated with PAHs, thus accelerating the removal of PAHs. BM had a similar influence on the microbial structure in soil with DPF and PEPF, decreased the abundance of Pseudomonadaceae, and increased the abundance of Methylophilaceae, Bacillaceae, Burkholderiaceae, and Bogoriellaceae. These microbes are all related to PAHs and could act as the indicator microbes related to PAH degradation. Some fungal communities are also associated with PAHs (Potin et al., 2004; Aranda et al., 2017; Vasudevan et al., 2018). The change of fungal community might lead to the changes in bacterial degradation capacity (Wu et al., 2020). In PBM, the abundance of Nectriacea increased. A previous study showed that some species in Nectriaceae played a key role in the removal of PAHs (Lladó et al., 2013; Brusetti et al., 2018), which might be one of the reasons for the decrease of PAH content in the treatment of PBM. Therefore, except for the sorption effect of MPs and BC on the organic pollutants potentially accelerating the removal rate, the selective enrichment effect of the plastic debris and BC on the specific bacterial community potentially helps to accelerate the removal of PAHs.
In this study, the combined effects of plastic fragments and BC on the soil properties, the removal of pollutants, and the microbial community were tested. Our results illustrated that the combined effects of PEPF with BC or BM could accelerate the removal of PAHs. The influence of DPF on soil DOC was related to the soil carbon content. BM decreased the soil DOC, which might be due to its strong adsorption while irrelevant with the types of plastics. The combined effect of DPF with BC or BM could change the component of DOC, while the change of DOC caused by PEPF was associated with its own characteristics. Our results indicating two potential possible reasons contribute to increasing the removal of organic pollutants, (1) the high sorption rate on the PEPF and BC and (2) the increased PAH-degrader or PAE-degrader abundance for the removal of organic pollutants.
The original contributions presented in the study are included in the article/Supplementary Material, further inquiries can be directed to the corresponding author/s.
XR did the experiment and prepared the manuscript. JT designed the experiment and prepared the manuscript. LW did the data treatment analysis work. HS prepared the manuscript. All the authors contributed to the article and approved the submitted version.
This work was supported by the National Natural Science Foundation of China (Nos. U1806216 and 41877372), the National Key R&D Program of China (2018YFC1802002), and the 111 program, Ministry of Education, China (No. T2017002).
The authors declare that the research was conducted in the absence of any commercial or financial relationships that could be construed as a potential conflict of interest.
The Supplementary Material for this article can be found online at: https://www.frontiersin.org/articles/10.3389/fmicb.2021.647766/full#supplementary-material
Abel, S., Peters, A., Trinks, S., Schonsky, H., Facklam, M., and Wessolek, G. (2013). Impact of biochar and hydrochar addition on water retention and water repellency of sandy soil. Geoderma 20, 183–191. doi: 10.1016/j.geoderma.2013.03.003
Aranda, E., Godoy, P., Reina, R., Badia-Fabregat, M., Rosell, M., Marco-Urrea, E., et al. (2017). Isolation of Ascomycota fungi with capability to transform PAHs: insights into the biodegradation mechanisms of Penicillium oxalicum. Int. Biodeteriorat. Biodegradat. 122, 141–150. doi: 10.1016/j.ibiod.2017.05.015
Baldantoni, D., Morelli, R., Bellino, A., Prati, M. V., Alfani, A., and De Nicola, F. (2017). Anthracene and benzo(a)pyrene degradation in soil is favoured by compost amendment: perspectives for a bioremediation approach. J. Hazard. Mater. 339, 395–400. doi: 10.1016/j.jhazmat.2017.06.043
Bandopadhyay, S., Martin-Closas, L., Pelacho, A. M., and DeBruyn, J. M. (2018). Biodegradable plastic mulch films: impacts on soil microbial communities and ecosystem functions. Front. Microbiol. 9:819. doi: 10.3389/fmicb.2018.00819
Bao, H., Wang, J., Zhang, H., Li, J., Li, H., and Wu, F. (2020). Effects of biochar and organic substrates on biodegradation of polycyclic aromatic hydrocarbons and microbial community structure in PAHs-contaminated soils. J. Hazard. Mater. 385:121595. doi: 10.1016/j.jhazmat.2019.121595
Barnes, D. K. A., Galgani, F., Thompson, R. C., and Barlaz, M. (2009). Accumulation and fragmentation of plastic debris in global environments. Philos. Trans. R. Soc. B: Biol. Sci. 364, 1985–1998. doi: 10.1098/rstb.2008.0205
Bellino, A., Baldantoni, D., Picariello, E., Morelli, R., Alfani, A., and De Nicola, F. (2019). Role of different microorganisms in remediating PAH-contaminated soils treated with compost or fungi. J. Environ. Manage. 252:109675. doi: 10.1016/j.jenvman.2019.109675
Besseling, E., Wegner, A., Foekema, E. M., van den Heuvel-Greve, M. J., and Koelmans, A. A. (2013). Effects of microplastic on fitness and PCB bioaccumulation by the lugworm Arenicola marina (L.). Environ. Sci. Technol. 47, 593–600. doi: 10.1021/es302763x
Browne, M. A., Niven, S. J., Galloway, T. S., Rowland, S. J., and Thompson, R. C. (2013). Microplastic moves pollutants and additives to worms. reducing functions linked to health and biodiversity. Curr. Biol. 23, 2388–2392. doi: 10.1016/j.cub.2013.10.012
Brusetti, L., Ciccazzo, S., Borruso, L., Bellucci, M., Zaccone, C., and Beneduce, L. (2018). Metataxonomy and functionality of wood-tar degrading microbial consortia. J. Hazard. Mater. 353, 108–117. doi: 10.1016/j.jhazmat.2018.03.041
Chao, A. (1984). Nonparametric estimation of the number of classes in a population. Candinav. J. Statist. 11, 265–270.
Chao, A., and Yang, M. C. K. (1993). Stopping rules and estimation for recapture debugging with unequal failure rates. Biometrika 80, 193–201. doi: 10.1093/biomet/80.1.193
Chen, H., Wang, Y., Sun, X., Peng, Y., and Xiao, L. (2020). Mixing effect of polylactic acid microplastic and straw residue on soil property and ecological function. Chemosphere 243:125271. doi: 10.1016/j.chemosphere.2019.125271
Cheng, J., Liu, Y., Wan, Q., Yuan, L., and Yu, X. (2018). Degradation of dibutyl phthalate in two contrasting agricultural soils and its long-term effects on soil microbial community. Sci. Total Environ. 640–641, 821–829. doi: 10.1016/j.scitotenv.2018.05.336
Cozar, A., Echevarria, F., Gonzalez-Gordillo, J. I., Irigoien, X., Ubeda, B., Hernandez-Leon, S., et al. (2014). Plastic debris in the open ocean. Proc. Natl. Acad. Sci. U S A. 111, 10239–10244. doi: 10.1073/pnas.1314705111
Cui, J. (2021). Long-term effects of biochar application on greenhouse gas production and microbial community in temperate forest soils under increasing temperature. Sci. Total Environ. 767:145021.
Ding, Y., Liu, Y., Liu, S., Huang, X., Li, Z., Tan, X., et al. (2017). Potential benefits of biochar in agricultural soils: a review. Pedosphere 27, 645–661. doi: 10.1016/S1002-0160(17)60375-8
Ding, Z., Zhang, F., Gong, H., Sun, N., Huang, J., and Chi, J. (2020). Responses of phenanthrene degradation to the changes in bioavailability and microbial community structure in soils amended with biochars pyrolyzed at low and high temperatures. J. Hazard. Mater. 410:124584. doi: 10.1016/j.jhazmat.2020.124584
Dittmar, T., de Rezende, C. E., Manecki, M., Niggemann, J., Coelho Ovalle, A. R., Stubbins, A., et al. (2012). Continuous flux of dissolved black carbon from a vanished tropical forest biome. Nat. Geosci. 5, 618–622. doi: 10.1038/ngeo1541
Fei, Y., Huang, S., Zhang, H., Tong, Y., Wen, D., Xia, X., et al. (2020). Response of soil enzyme activities and bacterial communities to the accumulation of microplastics in an acid cropped soil. Sci. Total Environ. 707:135634. doi: 10.1016/j.scitotenv.2019.135634
Foulon, V., Le Roux, F., Lambert, C., Huvet, A., Soudant, P., and Paul-Pont, I. (2016). Colonization of polystyrene microparticles by Vibrio crassostreae: light and electron microscopic investigation. Environ. Sci. Technol. 50, 10988–10996. doi: 10.1021/acs.est.6b02720
Gatheru Waigi, M., Sun, K., and Gao, Y. (2017). Sphingomonads in microbe-assisted phytoremediation: tackling soil pollution. Trends Biotechnol. 35, 883–899. doi: 10.1016/j.tibtech.2017.06.014
Geyer, R., Jambeck, J. R., and Law, K. L. (2017). Production, use, and fate of all plastics ever made. Sci. Adv. 3:e1700782. doi: 10.1126/sciadv.1700782
He, L., Fan, S., Müller, K., Wang, H., Che, L., Xu, S., et al. (2018). Comparative analysis biochar and compost-induced degradation of di-(2-ethylhexyl) phthalate in soils. Sci. Total Environ. 625, 987–993. doi: 10.1016/j.scitotenv.2018.01.002
Hodson, M. E., Duffus-Hodson, C. A., Clark, A., Prendergast-Miller, M. T., and Thorpe, K. L. (2017). Plastic bag derived-microplastics as a vector for metal exposure in terrestrial invertebrates. Environ. Sci. Technol. 51, 4714–4721. doi: 10.1021/acs.est.7b00635
Horton, A. A., Walton, A., Spurgeon, D. J., Lahive, E., and Svendsen, C. (2017). Microplastics in freshwater and terrestrial environments: evaluating the current understanding to identify the knowledge gaps and future research priorities. Sci. Total Environ. 586, 127–141. doi: 10.1016/j.scitotenv.2017.01.190
Huerta Lwanga, E., Gertsen, H., Gooren, H., Peters, P., Salánki, T., van der Ploeg, M., et al. (2016). Microplastics in the terrestrial ecosystem: implications for Lumbricus terrestris (Oligochaeta, Lumbricidae). Environ. Sci. Technol. 50, 2685–2691. doi: 10.1021/acs.est.5b05478
Jaffrain, J., Gérard, F., Meyer, M., and Ranger, J. (2007). Assessing the quality of dissolved organic matter in forest soils using ultraviolet absorption spectrophotometry. Soil Sci. Soc. Am. J. 71:1851. doi: 10.2136/sssaj2006.0202
Jia, R., Qu, Z., You, P., and Qu, D. (2017). Effect of biochar on photosynthetic microorganism growth and iron cycling in paddy soil under different phosphate levels. Sci. Total Environ. 612, 223–230. doi: 10.1016/j.scitotenv.2017.08.126
Jiménez-Skrzypek, G., Hernández-Sánchez, C., Ortega-Zamora, C., González-Sálamo, J., and González-Curbelo, M. Á, and Hernández-Borges, J. (2021). Microplastic-adsorbed organic contaminants: analytical methods and occurrence. TrAC Trends Anal. Chem. 136:116186. doi: 10.1016/j.trac.2021.116186
Keiluweit, M., Kleber, M., Sparrow, M. A., Simoneit, B. R. T., and Prahl, F. G. (2012). Solvent-Extractable polycyclic aromatic hydrocarbons in biochar: influence of pyrolysis temperature and feedstock. Environ. Sci. Technol. 46, 9333–9341. doi: 10.1021/es302125k
Kielak, A. M., Barreto, C. C., Kowalchuk, G. A., van Veen, J. A., and Kuramae, E. E. (2016). The ecology of acidobacteria: moving beyond genes and genomes. Front. Microbiol. 7:744. doi: 10.3389/fmicb.2016.00744
Kong, S., Ji, Y., Liu, L., Chen, L., Zhao, X., Wang, J., et al. (2012). Diversities of phthalate esters in suburban agricultural soils and wasteland soil appeared with urbanization in China. Environ. Pollut. 170, 161–168. doi: 10.1016/j.envpol.2012.06.017
Kumar, M., Xiong, X., Wan, Z., Sun, Y., Tsang, D. C. W., Gupta, J., et al. (2020). Ball milling as a mechanochemical technology for fabrication of novel biochar nanomaterials. Bioresour. Technol. 312:123613. doi: 10.1016/j.biortech.2020.123613
Law, K. L., and Thompson, R. C. (2014). Microplastics in the seas. Science 345, 144–145. doi: 10.1126/science.1256304
Li, X., Jiang, X., Song, Y., and Chang, S. X. (2021). Coexistence of polyethylene microplastics and biochar increases ammonium sorption in an aqueous solution. J. Hazard. Mater. 405:124260. doi: 10.1016/j.jhazmat.2020.124260
Liu, S.-H., Zeng, G.-M., Niu, Q.-Y., Liu, Y., Zhou, L., Jiang, L.-H., et al. (2017). Bioremediation mechanisms of combined pollution of PAHs and heavy metals by bacteria and fungi: a mini review. Bioresour. Technol. 224, 25–33. doi: 10.1016/j.biortech.2016.11.095
Lladó, S., Gràcia, E., Solanas, A. M., and Viñas, M. (2013). Fungal and bacterial microbial community assessment during bioremediation assays in an aged creosote-polluted soil. Soil Biol. Biochem. 67, 114–123. doi: 10.1016/j.soilbio.2013.08.010
Lu, C., Hong, Y., Liu, J., Gao, Y., Ma, Z., Yang, B., et al. (2019). A PAH-degrading bacterial community enriched with contaminated agricultural soil and its utility for microbial bioremediation. Environ. Pollut. 251, 773–782. doi: 10.1016/j.envpol.2019.05.044
Luo, Y., Dungait, J. A. J., Zhao, X., Brookes, P. C., Durenkamp, M., Li, G., et al. (2018). Pyrolysis temperature during biochar production alters its subsequent utilization by microorganisms in an acid arable soil. Land. Degrad. Dev. 29, 2183–2188. doi: 10.1002/ldr.2846
Lyu, H., Gao, B., He, F., Zimmerman, A. R., Ding, C., Tang, J., et al. (2018). Experimental and modeling investigations of ball-milled biochar for the removal of aqueous methylene blue. Chem. Eng. J. 335, 110–119. doi: 10.1016/j.cej.2017.10.130
Lyu, H., He, Y., Tang, J., Hecker, M., Liu, Q., Jones, P. D., et al. (2016). Effect of pyrolysis temperature on potential toxicity of biochar if applied to the environment. Environ. Pollut. 218, 1–7. doi: 10.1016/j.envpol.2016.08.014
Ng, E.-L., Huerta Lwanga, E., Eldridge, S. M., Johnston, P., Hu, H.-W., Geissen, V., et al. (2018). An overview of microplastic and nanoplastic pollution in agroecosystems. Sci. Total Environ. 627, 1377–1388. doi: 10.1016/j.scitotenv.2018.01.341
Oyehan, T. A., and Al-Thukair, A. A. (2017). Isolation and characterization of PAH-degrading bacteria from the Eastern province. Saudi Arabia. Mar. Pollut. Bull. 115, 39–46. doi: 10.1016/j.marpolbul.2016.11.007
Potin, O., Rafin, C., and Veignie, E. (2004). Bioremediation of an aged polycyclic aromatic hydrocarbons (PAHs)-contaminated soil by filamentous fungi isolated from the soil. Int. Biodeterioration Biodegradation 54, 45–52. doi: 10.1016/j.ibiod.2004.01.003
Qi, Y., Ossowicki, A., Yang, X., Huerta Lwanga, E., Dini-Andreote, F., Geissen, V., et al. (2020). Effects of plastic mulch film residues on wheat rhizosphere and soil properties. J. Hazard. Mater. 387:121711. doi: 10.1016/j.jhazmat.2019.121711
Rafin, C., Veignie, E., Fayeulle, A., and Surpateanu, G. (2009). Benzo[a]pyrene degradation using simultaneously combined chemical oxidation, biotreatment with Fusarium solani and cyclodextrins. Bioresour. Technol. 100, 3157–3160. doi: 10.1016/j.biortech.2009.01.012
Ren, X., Tang, J., Liu, X., and Liu, Q. (2020). Effects of microplastics on greenhouse gas emissions and the microbial community in fertilized soil. Environ. Pollut. 256:113347. doi: 10.1016/j.envpol.2019.113347
Rillig, M. C. (2012). Microplastic in terrestrial ecosystems and the soil? Environ. Sci. Technol. 46, 6453–6454. doi: 10.1021/es302011r
Shannon, C. E. (1948). A mathematical theory of communication.pdf. Bell System Tech. J. 27, 623–656.
Thompson, R. C. (2004). Lost at sea: where is all the plastic? Science 304, 838–838. doi: 10.1126/science.1094559
Vasudevan, V., Gayathri, K. V., and Krishnan, M. E. G. (2018). Bioremediation of a pentacyclic PAH, Dibenz(a,h)Anthracene- a long road to trip with bacteria, fungi, autotrophic eukaryotes and surprises. Chemosphere 202, 387–399. doi: 10.1016/j.chemosphere.2018.03.074
Wang, B., Zhang, W., Li, H., Fu, H., Qu, X., and Zhu, D. (2017). Micropore clogging by leachable pyrogenic organic carbon: a new perspective on sorption irreversibility and kinetics of hydrophobic organic contaminants to black carbon. Environ. Pollut. 220, 1349–1358. doi: 10.1016/j.envpol.2016.10.100
Wang, C., Wang, Y., and Herath, H. M. S. K. (2017). Polycyclic aromatic hydrocarbons (PAHs) in biochar – Their formation, occurrence and analysis: a review. Organ. Geochem. 114, 1–11. doi: 10.1016/j.orggeochem.2017.09.001
Wang, J., Luo, Y., Teng, Y., Ma, W., Christie, P., and Li, Z. (2013). Soil contamination by phthalate esters in Chinese intensive vegetable production systems with different modes of use of plastic film. Environ. Pollut. 180, 265–273. doi: 10.1016/j.envpol.2013.05.036
Wang, Z., Liu, S., Xu, W., Hu, Yunlong, Hu, et al. (2016). The microbiome and functions of black soils are altered by dibutyl phthalate contamination. Appl. Soil Ecol. 99, 51–61. doi: 10.1016/j.apsoil.2015.11.024
Wright, S. L., Thompson, R. C., and Galloway, T. S. (2013). The physical impacts of microplastics on marine organisms: a review. Environ. Pollut. 178, 483–492. doi: 10.1016/j.envpol.2013.02.031
Wu, M., Guo, X., Wu, J., and Chen, K. (2020). Effect of compost amendment and bioaugmentation on PAH degradation and microbial community shifting in petroleum-contaminated soil. Chemosphere 256:126998. doi: 10.1016/j.chemosphere.2020.126998
Xiang, W., Zhang, X., Chen, J., Zou, W., He, F., Hu, X., et al. (2020). Biochar technology in wastewater treatment: a critical review. Chemosphere 252:126539. doi: 10.1016/j.chemosphere.2020.126539
Xiao, Y., Lyu, H., Tang, J., Wang, K., and Sun, H. (2020). Effects of ball milling on the photochemistry of biochar: enrofloxacin degradation and possible mechanisms. Chem. Eng. J. 384:123311. doi: 10.1016/j.cej.2019.123311
Xu, C., Zhang, B., Gu, C., Shen, C., Yin, S., Aamir, M., et al. (2020). Are we underestimating the sources of microplastic pollution in terrestrial environment? J. Hazardous Mater. 400:123228. doi: 10.1016/j.jhazmat.2020.123228
Yaashikaa, P. R., Kumar, P. S., Varjani, S., and Saravanan, A. (2020). A critical review on the biochar production techniques, characterization, stability and applications for circular bioeconomy. Biotechnol. Rep. 28:e00570. doi: 10.1016/j.btre.2020.e00570
Zhang, M., Zhao, Y., Qin, X., Jia, W., Chai, L., Huang, M., et al. (2019). Microplastics from mulching film is a distinct habitat for bacteria in farmland soil. Sci. Total Environ. 688, 470–478. doi: 10.1016/j.scitotenv.2019.06.108
Zhou, Y., Gao, B., Zimmerman, A. R., Fang, J., Sun, Y., and Cao, X. (2013). Sorption of heavy metals on chitosan-modified biochars and its biological effects. Chem. Eng. J. 231, 512–518. doi: 10.1016/j.cej.2013.07.036
Keywords: microplastics, biochar, PAHs, PAEs, microbes, removal
Citation: Ren X, Tang J, Wang L and Sun H (2021) Combined Effects of Microplastics and Biochar on the Removal of Polycyclic Aromatic Hydrocarbons and Phthalate Esters and Its Potential Microbial Ecological Mechanism. Front. Microbiol. 12:647766. doi: 10.3389/fmicb.2021.647766
Received: 30 December 2020; Accepted: 19 March 2021;
Published: 30 April 2021.
Edited by:
Xianhua Liu, Tianjin University, ChinaReviewed by:
Xiaochen Chen, Fuzhou University, ChinaCopyright © 2021 Ren, Tang, Wang and Sun. This is an open-access article distributed under the terms of the Creative Commons Attribution License (CC BY). The use, distribution or reproduction in other forums is permitted, provided the original author(s) and the copyright owner(s) are credited and that the original publication in this journal is cited, in accordance with accepted academic practice. No use, distribution or reproduction is permitted which does not comply with these terms.
*Correspondence: Jingchun Tang, dGFuZ2pjaEBuYW5rYWkuZWR1LmNu; Lan Wang, ZW52d2FuZ2xAbmFua2FpLmVkdS5jbg==
Disclaimer: All claims expressed in this article are solely those of the authors and do not necessarily represent those of their affiliated organizations, or those of the publisher, the editors and the reviewers. Any product that may be evaluated in this article or claim that may be made by its manufacturer is not guaranteed or endorsed by the publisher.
Research integrity at Frontiers
Learn more about the work of our research integrity team to safeguard the quality of each article we publish.