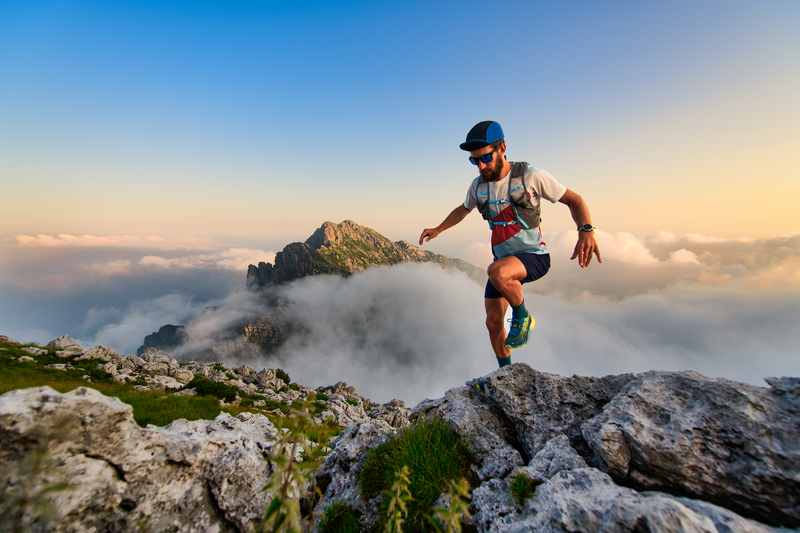
95% of researchers rate our articles as excellent or good
Learn more about the work of our research integrity team to safeguard the quality of each article we publish.
Find out more
ORIGINAL RESEARCH article
Front. Microbiol. , 09 April 2021
Sec. Microbial Physiology and Metabolism
Volume 12 - 2021 | https://doi.org/10.3389/fmicb.2021.645028
Anabaena variabilis is a filamentous cyanobacterium that is capable to differentiate specialized cells, the heterocysts and akinetes, to survive under different stress conditions. Under nitrogen limited condition, heterocysts provide the filament with nitrogen by fixing N2. Akinetes are spore-like dormant cells that allow survival during adverse environmental conditions. Both cell types are characterized by the presence of a thick multilayered envelope, including a glycolipid layer. While in the heterocyst this glycolipid layer is required for the maintenance of a microoxic environment and nitrogen fixation, its function in akinetes is completely unknown. Therefore, we constructed a mutant deficient in glycolipid synthesis and investigated the performance of heterocysts and akinetes in that mutant strain. We chose to delete the gene Ava_2595, which is homolog to the known hglB gene, encoding a putative polyketide synthase previously shown to be involved in heterocyst glycolipid synthesis in Anabaena sp. PCC 7120, a species which does not form akinetes. Under the respective conditions, the Ava_2595 null mutant strain formed aberrant heterocysts and akinete-like cells, in which the specific glycolipid layers were absent. This confirmed firstly that both cell types use a glycolipid of identical chemical composition in their special envelopes and, secondly, that HglB is essential for glycolipid synthesis in both types of differentiated cells. As a consequence, the mutant was not able to fix N2 and to grow under diazotrophic conditions. Furthermore, the akinetes lacking the glycolipids showed a severely reduced tolerance to stress conditions, but could germinate normally under standard conditions. This demonstrates the importance of the glycolipid layer for the ability of akinetes as spore-like dormant cells to withstand freezing, desiccation, oxidative stress and attack by lytic enzymes. Our study established the dual role of the glycolipid layer in fulfilling different functions in the evolutionary-related specialized cells of cyanobacteria. It also indicates the existence of a common pathway involving HglB for the synthesis of glycolipids in heterocysts and akinetes.
To cope with various stress conditions, cyanobacteria require adaptation and survival strategies. Cell differentiation is key for successful survival in harsh and changing environmental conditions (Rippka and Herdman, 1985; Flores and Herrero, 2010). The planktonic freshwater filamentous cyanobacterium Anabaena variabilis ATCC 29413 can undergo a variety of cellular differentiation processes forming motile hormogonia, nitrogen-fixing heterocysts, and spore-like dormant cells called akinetes, and therefore, serves as a model organism to study the cell differentiation process in prokaryotes (Flores and Herrero, 2010; Maldener et al., 2014). The related species Anabaena sp. PCC 7120, which does not form akinetes, is the model organism to study heterocyst differentiation.
In A. variabilis, the nitrogen (N2) fixing heterocysts appear in a semi-regular pattern along the filament in response to insufficient supply of combined nitrogen under aerobic conditions (Fay, 1992; Muro-Pastor and Maldener, 2019). Nitrogen fixation is mediated by the enzyme nitrogenase, which is highly sensitive to oxygen. To protect the nitrogenase, a microoxic environment is maintained in the heterocysts by inactivation of oxygen-evolving photosynthesis, by increased respiration, and by formation of an additional multilayered cell envelope outside the cell wall (Murry et al., 1984; Walsby, 1985; Murry and Wolk, 1989; Valladares et al., 2003, 2007; Magnuson, 2019). The heterocyst envelope is composed of two layers: an inner heterocyst-specific glycolipid (HGL) layer, which acts as a barrier to oxygen and limits its diffusion into the heterocyst, and an outermost heterocyst envelope polysaccharide (HEP) layer, which protects the HGL layer from physical damage (Wolk et al., 1994; Adams, 2000; Maldener et al., 2014; Muro-Pastor and Maldener, 2019).
Akinetes are spore-like non-motile cells that differentiate from the vegetative cells in response to diverse environmental factors including changes in light intensity, temperature, and nutrient deficiency. Akinetes can endure dryness and cold, while being vulnerable to high temperatures unlike spores, which are heat resistant. For this reason, they are just referred to as “spore-like” cells. Akinetes differ from the vegetative cells by their cellular structure, composition, and morphology. Akinetes ensure a longer period of survival to cyanobacteria under harsh and unfavorable conditions due to their resistance to cold and desiccation, and serve a perennation role (Yamamoto, 1976; Kaplan-Levy et al., 2010). Akinete formation is a transient process. When environmental conditions are favorable for growth, akinetes germinate into vegetative cells and start their life cycle over (Maldener et al., 2014). The light, temperature, and nutrient conditions favorable for growth appear to be the major stimulus for akinete germination (Yamamoto, 1976; Van Dok and Hart, 1997; Perez et al., 2016; Sukenik et al., 2018). Compared to akinete differentiation, which takes several days up to weeks, germination was observed to occur much faster in just few hours (Perez et al., 2018).
The process of akinete differentiation is characterized by the transient accumulation of storage compounds (such as glycogen, cyanophycin, lipids and nucleic acids), reduction of metabolic activities and formation of a thick multilayered envelope (Sutherland et al., 1979; Sukenik et al., 2012; Perez et al., 2016, 2018). This specialized envelope is composed of an outermost polysaccharide layer and inner layers of glycolipids, similar in composition to that of the heterocyst envelope (Cardemil and Wolk, 1976, 1981; Reddy, 1983; Soriente et al., 1993; Wolk et al., 1994; Wolk, 1996; Perez et al., 2018; Qiu et al., 2019). Whilst the function of the HGL layer is well understood in heterocysts (Haury and Wolk, 1978; Ernst et al., 1992; Fay, 1992), its role in akinetes and stress survival is unknown. Furthermore, the akinete envelope has some extra laminated layers, the function of which remains unknown (Braune, 1980; Nichols and Adams, 1982; Perez et al., 2018). Due to high similarity between their cell envelopes, akinetes have been supposed to be the evolutionary precursors of heterocysts (Wolk et al., 1994).
Several genes encoding the enzymes responsible for HEP and HGL synthesis in the heterocyst envelope have been identified [summarized in Nicolaisen et al. (2009); Maldener et al. (2014)]. The clustered genes alr5351–alr5357 (also known as hglEA, hglD, hglC, and hglB) in Anabaena sp. PCC 7120 (Black and Wolk, 1994; Bauer et al., 1997; Awai et al., 2009; Saito and Awai, 2020) and the hglE gene in Nostoc punctiforme (Campbell et al., 1997) are associated with glycolipid layer formation. The hglBCDEA gene cluster encodes putative enzymes for the biosynthesis of HGL aglycone (Fan et al., 2005; Awai et al., 2009; Saito and Awai, 2020). The devBCA gene cluster has been shown to be required for HGL export in Anabaena sp. PCC 7120 and A. variabilis (Fiedler et al., 1998a, b; Staron et al., 2011). Similarly, the gene cluster hgdABC is essential for proper HGL layer deposition in the heterocyst envelope in Anabaena sp. PCC 7120 (Fan et al., 2005; Shvarev et al., 2018).
Several heterocyst genes have also been found associated with akinete formation. For instance, the hepA gene, encoding a putative polysaccharide exporter, is essential for correct heterocyst envelope formation and consequently, the A. variabilis hepA-mutant forms an abnormal akinete envelope (Leganés, 1994). The overexpression of the heterocyst regulatory gene devR results in enhanced akinete differentiation in N. punctiforme (Campbell et al., 1996). In Nostoc ellipsosporum, deletion of the regulatory gene hetR inhibited both heterocyst and akinete differentiation (Leganés et al., 1994). However, a hetR mutant of N. punctiforme under phosphate starvation conditions could form large akinete-like cold-resistant cells (Wong and Meeks, 2002). These studies indicate a role for heterocyst genes in akinete formation, suggesting a common pathway regulating their differentiation.
The hglB gene [also known as hetM (Black and Wolk, 1994) or alr5357] encodes a putative polyketide synthase required for the synthesis of the glycolipid aglycones. HglB possesses two functional domains, an N-terminal acyl carrier protein (ACP) domain and a C-terminal thioester reductase (TER) domain (Black and Wolk, 1994; Bauer et al., 1997; Awai et al., 2009). The characterization of a hglB mutant of Anabaena sp. PCC 7120 (Maldener et al., 2003) demonstrated the role of this gene in HGL synthesis during heterocyst differentiation. A. variabilis harbors a gene in its genome with high similarity to hglB. To investigate the role of this hglB homolog in the synthesis of the glycolipid layer of akinetes, we performed a mutational analysis in A. variabilis. As expected, the HGL layer of heterocysts is absent in the hglB mutant of A. variabilis. But also, distinct laminated layers are absent in the mutant akinetes. These aberrant akinetes lose their resistance against various stress conditions implying a different function of the glycolipid layer in heterocysts and akinetes. With this study, we were also able to show that envelope formation requires the same biosynthetic pathway in akinetes and heterocysts indicating an evolutionary relationship between both differentiation processes.
Vegetative cultures of Anabaena variabilis ATCC 29413 strain FD (Currier and Wolk, 1979; Thiel et al., 2014) and derived mutant strains (Supplementary Table 1) were grown photoautotrophically under continuous illumination (17–22 μmol photons m–2 s–1) at 28°C with shaking at 120 rpm in standard medium of Allen and Arnon (1955) diluted 4-fold with water (AA/4) and supplemented with 5 mM KNO3. The solid media remained undiluted with 1.5% (w/v) Difco Agar. For growing the mutant strain, 50 μg ml–1 neomycin was added to the medium. To induce heterocyst differentiation, the exponentially growing cultures (OD750 nm 0.4–0.5) were harvested and washed three times in nitrate-free AA/4 medium, resuspended in same medium equal to the original volume, and cultivated under nitrogen depleted conditions.
Escherichia coli strains were grown in lysogeny broth (LB) medium at 37°C, supplemented with the following antibiotics: 50 μg ml–1 kanamycin (Km), 25 μg ml–1 chloramphenicol (Cm), 25 μg ml–1 streptomycin (Sm) and 100 μg ml–1 spectinomycin (Sp), when required. For growth on solid medium, 1.5% (w/v) agar was added. The E. coli strain Top 10 was used as a host for plasmid constructions. For triparental conjugation, the E. coli strain J53 (bearing the conjugative plasmid RP4), strain HB101 (bearing the helper plasmid pRL528 and the cargo plasmid), and the wild type (WT) A. variabilis culture were used (Maldener et al., 1991; Supplementary Table 1).
To construct the hglB mutant in A. variabilis, the gene Ava_2595 (hglB) was inactivated by insertion of the neomycin-resistance-conferring cassette (C.K3) into the genome by double-crossover homologous recombination (Elhai and Wolk, 1988). For this, the left- and right-flanking regions of 500 bp from hglB were amplified in PCR using primers 1979 and 1980, and 1983 and 1984 (see Supplementary Table 2 for primers) using genomic DNA as template and high fidelity Q5-polymerase (NEB, Ipswich, MA, United States). The C.K3 cassette was amplified from the plasmid pIM74 (Fiedler et al., 1998b) using primers 1981 and 1982. All PCR products were fused into the PstI digested suicide vector pRL271 (Supplementary Table 1) using Gibson assembly (Gibson et al., 2009). The resulting plasmid pIM752 was transferred into WT A. variabilis cells via triparental mating followed by the selection of clones on neomycin and 5% sucrose-containing agar plates (Maldener et al., 1991). Several clones were checked for full segregation of the mutated gene by colony PCR using primers 2534 and 2535. One of these genotypically verified and identical mutant clones was chosen for our study and named as DR752.
For akinete induction, BG11 medium containing NaNO3 (Rippka et al., 1979) was used, as the differentiation of akinetes was better observed in this medium compared to AA/4. The late-exponentially grown cultures (OD750nm 0.8) were induced to differentiate akinetes by transferring them to low light condition (2–3 μmol photons m–2 s–1) with shaking at 50 rpm (Perez et al., 2016).
The germination of mature akinetes, exposed to different stress conditions, was induced by washing and transferring the culture to fresh BG11 media containing NaNO3 and optimal light conditions (Perez et al., 2018). The formation of akinetes and their germination was monitored with a Leica DM 2500 light microscope with an x100/1.3 oil objective, connected to a Leica DFC420C camera (Leica Microsystems GmbH, Wetzlar, Germany).
To observe the heterocyst envelope polysaccharide layer, Alcian blue staining was performed (Mckinney, 1953). Alcian blue solution [1.5% in H2O (w/v)] was added to the cell suspension (in a ratio of 1:100) and incubated at room temperature (RT) for 5–10 min.
The culture containing heterocysts was mixed with the TTC solution [0.05% of TTC (w/v) in the final mixture] and incubated in dark for 15–30 min at RT (Fay and Kulasooriya, 1972).
In order to visualize the glycolipid layer in the heterocyst and akinete envelope, samples with heterocyst and akinetes were stained with boron-dipyrromethene difluoride (BODIPY) 493/503 (Molecular Probes, Thermo Fisher Scientific, Waltham, MA, United States) as described previously (Perez et al., 2016). After all staining procedures, filaments were placed on the slides covered with 1.5% agarose and observed by light microscopy with a Leica DM 2500 microscope connected to Leica DFC420C camera or with a Leica DM 5500B fluorescence microscope connected to Leica DFC420C camera. The green fluorescence signal was monitored with a BP470 40-nm excitation filter and a BP525 50-nm emission filter.
For electron microscopy studies, individual cultures containing akinetes or heterocysts were fixed with 2.5% glutaraldehyde and post-fixed with 2% potassium permanganate followed by immobilization in agarose. After dehydration by successive increment of the ethanol concentration, the samples were embedded in Epon. Ultrathin sections were stained with uranyl acetate and lead citrate (Fiedler et al., 1998a), and examined using a Philips Tecnai 10 electron microscope at 80 kHz.
The total RNA was isolated at different time points after akinete induction (0, 3, 6, 12, and 18 days) or nitrogen step-down (0, 8, and 48 h) from wild-type and mutant DR752 cells, using UPzol reagent (Biotechrabbit, Henningsdorf, Germany) according to the manufacturer’s instructions. Briefly, cells were harvested at the different time points, immediately snap-freezed in liquid nitrogen and stored at −80°C till further use. Upon addition of 1 mL UPzol solution, cells were homogenized using glass beads and total RNA was extracted. Concentration and purity of the extracted RNA were estimated using nanophotometer (Implen) and RNA gel-electrophoresis followed by DNase treatment. The genomic DNA contamination was controlled by PCR followed by agarose gel electrophoresis. The DNase-treated RNA was used to generate cDNA using the RT-reaction kit (Applied Biosystems) and 1 μl of this cDNA was used for semi-q RT PCR followed by visualization on an agarose gel. Primers for semi-q RT PCR reactions are listed in Supplementary Table 2.
Nitrogenase activity was measured with the acetylene reduction method as previously described (Bornikoel et al., 2018; Shvarev et al., 2019). Briefly, after nitrogen step-down and 48 h cultivation under nitrogen-limiting conditions, the cell suspensions (20 μg Chl) were incubated under an atmosphere of 13.3% acetylene in air (oxic conditions) for several hours in flasks sealed with gas-tight caps and shaken in the light at 28°C. To generate anoxic conditions, a solution of 10 μM 3-(3,4-dichlorophenyl)-1, 1-dimethylurea (DCMU) (dissolved in methanol) was added and then, the sealed flasks were degassed, filled with argon and incubated for 1 h followed by incubation with acetylene. From each flask, 1 ml of the gaseous phase was taken, and the amount of ethylene produced was measured using gas chromatography.
Thin-layer chromatography (TLC) was performed to analyze the glycolipids composition of heterocysts and akinetes as described (Winkenbach et al., 1972) with minor modifications. First, the chlorophyll a (Chla) concentration was measured (Mackinney, 1941). Briefly, Chla was extracted from 1 ml of cultures grown with (BG11) and without nitrogen source (BG110), and from the cultures induced to form akinetes by low light for 2–4 months by adding methanol to a final concentration of 90% (v/v). The suspension was vortexed for 1 min and the cells were centrifuged at 13,000 rpm for 2 min at RT after incubation for 5 min in darkness followed by the measurement of adsorption of the supernatant at 665 nm. The final Chla concentration was calculated using the formula:
For TLC, the cells were pelleted at equal Chla concentration and resuspended in a methanol:chloroform mixture (1:1). Afterward, the lipids in the supernatant were concentrated by evaporation in air under a fume hood. The lipids were dissolved in chloroform and applied on an aluminum plate coated with silica gel (Macherey-Nagel, #818033). TLC was run in a mobile phase composed of chloroform:methanol:acetic acid:water in a ratio of 23:4:2.7:1. The lipids were visualized by spraying the TLC plate with 25% sulfuric acid and exposing it to 180°C for 60–120 s.
The viability of akinetes (3–4 months old) was tested after exposing them to different stress conditions using an agar spot assay. For this, Chla content of akinetes culture was determined as described in previous section. For inducing the akinetes to different stress conditions, 1 ml of akinete cultures with a concentration of 5 μg Chla ml–1 were exposed to either freeze (−20°C) and cold (4°C) conditions or desiccated (centrifuged and pellet dried at 28°C) for 10, 20, and 30 days, respectively. Other stress conditions included the treatment with 10 mM H2O2 for 2 h at 28°C, with 300 μg ml–1 lysozyme overnight at RT in dark and the freeze (in liquid nitrogen for 5–7 min) and thaw (at RT for 20–30 min) cycles for three or six times. For each stress conditions and time points, various dilutions of akinete samples (in the range of 5 μg ml–1−0.5 μg ml–1 Chla) were prepared and 10 μl of each dilution were carefully dropped on agar plates and incubated for 1 week at 28°C under continuous light.
By BLAST search, we identified the homologous hglB gene Ava_2595 in the genome of A. variabilis. We created the mutant, which will be denoted DR752 from here onward, by insertion of a neomycin resistance cassette (CK.3) into the Ava_2595 gene by double homologous recombination (Supplementary Figure 1A). The complete segregation of the mutated chromosome version in neomycin resistant clones was confirmed by colony PCR (Supplementary Figure 1B) and one correct clone was used for further studies.
Under standard growth conditions in media supplemented with NO3–, no significant differences in doubling time (Figure 1A), filament length and cell morphology were observed in DR752 mutant compared to the wild type (WT) (not shown). However, the mutant was not able to grow on NO3–-free medium (Figure 1A). In WT filaments, normally distributed heterocysts as well as terminal heterocysts were observed. In contrast, delayed heterocyst differentiation mostly by the terminal cells of the filaments was observed in mutant DR752 (Supplementary Figure 1C). After 2 days of nitrogen starvation, deposition of the outer polysaccharide layer in mutant heterocysts was confirmed by Alcian-blue staining, which stains the polysaccharide envelope in blue (Supplementary Figure 2A). In contrast, the envelope glycolipids could not be detected by staining with the fluorescent dye BODIPY (Supplementary Figure 2B), which stains the HGL layer of heterocysts and akinetes (Perez et al., 2016).
Figure 1. Growth of the mutant strain DR752 and analysis of heterocyst-specific glycolipids in the envelope. (A) The growth of Anabaena variabilis wild type (WT) and DR752 mutant colonies on solid AA/4 medium (NO3–) and medium lacking a combined nitrogen source (N2) after 7 days of incubation. (B) Thin-layer chromatography of lipid extracts from liquid cultures containing 50 μg of chlorophyll before and after nitrogen step-down for 72 h; 1-WT with nitrate, 2-WT without nitrate, 3-DR752 with nitrate, 4-DR752 without nitrate. The arrow indicates the position of one heterocyst-specific glycolipid. (C) Transmission electron micrographs of terminal heterocysts of WT and mutant DR752. The lower panels show the magnified view of the envelope, indicated by squares. Black arrow points toward the laminated layer and star indicates the exopolysaccharide layer. Bars, 0.5 μm.
To investigate whether HGL is being synthesized in the mutant DR752 after nitrogen step-down, we performed thin-layer chromatography (TLC) with the lipids extracted from filaments of the WT and the DR752 strains. In contrast to the WT, the mutant did not show a band with the typical TLC migration property (Figure 1B), confirming that the hglB gene encodes a protein that is involved in HGL biosynthesis. This is in line with the semi-quantitative RT-PCR results showing that the hglB gene is differentially expresses in the WT strain during heterocyst differentiation (Supplementary Figure 3). As expected, a transcript of hglB could not be detected in the RNA of the mutant. Interestingly, the gene hetN, localized downstream of hglB is constitutively expressed in the mutant. In the WT, hetN was up regulated during nitrogen step-down (Supplementary Figure 3). Since the CK.3 cassette contains a strong promoter, but lacks a termination signal, we assume that the hetN gene, encoding a suppressor of heterocysts involved in pattern formation, gets under control of this constitutive promoter (Callahan and Buikema, 2001). Overexpression of hetN suppresses heterocyst differentiation in Anabaena sp. PCC 7120, which could explain the delayed heterocyst formation in the mutant DR752.
Furthermore, we studied the ultrastructure of the mutant heterocysts by transmission electron microscopy (TEM) of ultrathin sections of mutant and WT filaments (Figure 1C). As expected, the mutant did not form the laminated layer, explaining why it could not grow diazotrophically under oxic conditions.
To assess the oxygen status in the heterocysts of the mutant, the filaments were incubated with triphenyl tetrazolium chloride (TTC), which forms dark red-brown precipitates in a microoxic environment (Fay and Kulasooriya, 1972). The WT heterocysts showed such precipitates but the DR752 mutant heterocysts did not (Figure 2A).
Figure 2. Nitrogenase activity and nifHDK operon rearrangement assessment. (A) Bright field images of nitrogen-starved filaments of WT and mutant DR752 after incubation with triphenyl tetrazolium chloride (TTC). Black arrowheads point to the heterocysts. TTC reduces to dark formazan crystals under microoxic conditions. Scale bar, 10 μm. (B) Assessment of the nitrogenase activity of WT and mutant DR752 under oxic and anoxic conditions. Filaments were incubated for 48 h in nitrogen-free medium before measuring the nitrogenase activity using the acetylene reduction assay. Data are representative of two independent experiments. The histogram shows the mean values ± standard deviation of three experimental replicates. Student’s t-test P-value is indicated as asterisks (***P < 0.0001). (C) The nifD rearrangement in WT and mutant DR752 after 72 h of nitrogen step-down was shown by PCR using primers (Ava903 FW and 919 Rv) left and right to the 11-kb insertion element as listed in Supplementary Table 2.
Furthermore, we used the acetylene reduction assay to measure the nitrogenase activity under oxic and anoxic conditions (Figure 2B). The DR752 mutant showed no nitrogenase activity under oxic conditions despite the occurrence of the nifD gene rearrangement required to produce a functional enzyme (Figure 2C; Brusca et al., 1989; Thiel and Pratte, 2014). In contrast, under anoxic conditions, the nitrogenase activity was measurable in the mutant and the WT at a similar level. This indicates that the mutant is not able to provide microoxic conditions suitable for the activity of the nitrogenase, as previously described for the hglB mutant of Anabaena PCC 7120 (Fox–, Fix+ phenotype).
We have previously shown that the laminated layers of A. variabilis akinete mostly consist of the heterocyst-specific glycolipid HG26-diol (Perez et al., 2018). However, neither the function of this layer in akinete nor any enzyme for its synthesis was known so far. Hence, we investigated whether the hglB mutant from A. variabilis was affected in akinete differentiation and function. Stationary phase cultures of the WT and strain DR752 were incubated in low light conditions which trigger akinete formation (Perez et al., 2016). During the first 7 days in low light, WT filaments fragmented, whereas the mutant DR752 retained the long filaments and did not show any early sign of morphological differentiation (Figure 3A). After 15 days, approximately half of the WT filaments had turned into mature akinetes showing the typical morphology with the oval shape, thylakoid degradation evidenced by brownish color and a thick envelope (Perez et al., 2016). In contrast, the mutant strain showed only few immature akinetes with greenish color without a defined envelope (Figure 3A). After 30 days, most of the cells were mature akinetes in the WT culture. However, in the mutant DR752, approximately one-third of the mature akinetes were formed. This delayed akinete differentiation in DR752 indicates a possible role of HglB in akinete formation. However, upon a prolonged exposure of 2–4 months to low light, most of the vegetative cells in the DR752 mutant had finally differentiated into mature looking akinetes (not shown). Then, we investigated whether the akinetes of DR752 mutant were able to germinate similar to the WT akinetes. Three months after incubation of the filaments in low light, nearly all cells had differentiated to akinetes. After transferring these cultures to normal light, formation of filaments by germinating akinetes was observed. Cell division and resuscitation of the photosynthetic pigments occurred at the same speed in WT and mutant (Figure 3B).
Figure 3. Akinete differentiation and germination process in WT and mutant DR752. (A) Bright field micrographs of the WT and mutant DR752 culture after 7, 15, and 30 days of akinetes differentiation in low light. Bars, 10 μm. (B) Germination of 3–4 months old mature akinetes was induced by transferring the cultures to normal light conditions and monitored by light microscopy after 0, 24, 48, and 72 h. Bars, 5 μm.
Heterocysts and akinetes of several species have been suggested to possess a similar envelope structure and identical glycolipids (Nichols and Adams, 1982; Soriente et al., 1993; Leganés, 1994; Adams and Duggan, 1999; Perez et al., 2018). Two laminated layers are visible in electron micrographs of akinetes from A. variabilis (Figure 4C; see also Perez et al., 2016, 2018), and so far, it was not known, whether both consist of the same glycolipids. Therefore, we first compared the akinete envelopes of the WT with the mutant DR752 using the green fluorescent dye BODIPY, which stains the HGLs of akinetes and heterocysts. After cultivation in low light for 2–4 months, a strong well-defined green-fluorescent signal was detected in the outline of mature akinetes of the WT strain. In contrast, BODIPY did not stain the akinetes from the mutant, indicating the absence of lipid layers (Figure 4A).
Figure 4. Analysis of glycolipids and expression of hglB during akinetes formation. (A) Akinete envelope stained with the green fluorescent dye BODIPY. Control, mutant akinetes without BODIPY staining; BF, bright field; GFP, green fluorescent protein filter; AF, autofluorescence red. Bars, 7.5 μm. (B) Thin-layer chromatogram of lipid extracts from liquid cultures induced to develop akinetes in low light for 4 months. The position of the glycolipid band corresponding to the heterocyst-specific glycolipid HG26-diol is indicated by an arrow. (C) Transmission electron micrographs (TEM) of akinetes of WT and mutant DR752. Right panels show the magnified view of akinete envelope, indicated by square. Star indicates the exopolysaccharide layer. White arrowheads indicate the following layers: TL, thin laminated layer; TLL, thick laminated layer; CM, cell membrane; OM, outer membrane. The empty space between outer membrane and polysaccharide layer is a dehydration artifact of the sample preparation procedure. Bars, 0.5 μm. (D) Expression analysis of hglB in the WT culture during akinete induction performed by semi-quantitative RT-PCR. rnpB (RNase P RNA coding gene) was used as a loading control with (rnpB+) and without reverse transcriptase (rnpB-). The primers used for semi-quantitative RT-PCR are RT rnpBFw, RT rnpBRv, RT hglBFw, and RT hglBRv; the sequences of the primers are listed in Supplementary Table 2.
To investigate the presence of glycolipids in the akinete envelopes of WT and mutant DR752, total lipids were extracted from cultures exposed to low light for 2–4 months and separated by TLC. In the WT extract, a lipid was detected at the position where HG26-ol from heterocyst migrates (Figure 4B), consistent with our previous study (Perez et al., 2018). This specific band was not observed in the DR752 mutant akinete culture, confirming that the hglB gene is involved in the synthesis of this glycolipid.
Next, we analyzed ultrathin sections of the akinetes of WT and DR752 mutant by TEM to solve the structure of the envelope at a better resolution. In the WT, two distinct electron dense laminated layers were visible between the outer exopolysaccharide layer and the outer membrane of the gram-negative cell wall [(Perez et al., 2016); Figure 4C]. Akinetes of the mutant DR752 showed the outermost polysaccharide layer, but both darkly stained laminated layers were absent. This confirms that the heterocyst lipid HG26-diol is also a component of these laminated layers, and that the HglB is essential for HGL-synthesis in akinetes. This is in line with the increased transcription of the hglB-gene during akinete differentiation as shown by semi-quantitative RT-PCR (Figure 4D).
Akinetes are highly resistant to various environmental stress factors such as cold, desiccation and nutrient starvation. However, little is known about the molecular basis for such resistance and the role of the special thick envelope (Kaplan-Levy et al., 2010). After having shown that the mutant DR752 has no laminated layers in their akinete envelopes, this mutant offered the opportunity to investigate the importance of the glycolipid layer in resistance and response to different environmental extremes. For this, the akinetes of WT and mutant DR752 along with the WT vegetative cells as control were exposed to various stress conditions for different time points before diluting and plating on non-selective agar plates to determine their survival by colony formation. The WT vegetative cells and the akinetes of WT and mutant DR752 showed similar survival and germination efficiency, respectively being treated (Figure 5, left panels). Surprisingly, the same survival efficiency was observed for all types of cells when exposed to low temperature (cold, 4°C) (Figure 5A). However, the vegetative filaments were highly susceptible to the other tested stress conditions. The DR752 mutant akinetes were significantly less resistant and suffered a decline in survival efficiency when subjected to freeze and desiccation, compared to WT akinetes (Figure 5A). Upon successive freeze-thaw cycles, the mutant DR752 akinetes showed a significant decrease in germination relative to the WT akinetes (Figure 5B). Interestingly, the mutant DR752 akinetes were particularly more susceptible and could not germinate upon exposure to six freeze-thaw cycles as compared to three cycles (Figure 5B). To determine the effect of oxidative stress, the WT and mutant DR752 akinetes were treated with 10 mM H2O2 for 2 h. In these conditions the mutant DR752 akinetes showed decreased resistance and survival compared to the WT akinetes (Figure 5B, right panel). Under these conditions, the mutant akinetes showed cell lysis. Similarly, when treated with 300 μg ml–1 lysozyme overnight, survival efficiency of the DR752 mutant akinetes was severely affected and only few colonies could germinate (Figure 5C). These results demonstrated that the mutant DR752 akinetes were more stable than the vegetative filaments but compared to the WT akinetes they were less resistant against various environmental stress conditions. Altogether, this study indicates that the glycolipid layer of the akinete envelope has an essential function in survival of akinetes.
Figure 5. Viability of WT vegetative filaments and the akinetes of WT and mutant DR752 exposed to different stress conditions. Vegetative cells (Veg. cells) and 3–4 months akinete-induced culture of WT and mutant DR752 were exposed to/treated with (A) cold, freeze and desiccation for 20 days, (B) three and six freeze-thaw cycles or 10 mM H2O2 for 2 h and (C) 300 μg ml–1 lysozyme for overnight. Serial dilution and plating were performed on agar plates before and after treatments and incubated for 7 days to allow germination and pseudo colony formation.
Many genes are known to be involved in HGL formation in heterocysts of the model organism Anabaena sp. PCC 7120. In contrast, nothing is known about formation and role of the laminated layer in the akinete envelope. In this context, we have unraveled a new role of the homologous hglB gene in A. variabilis in glycolipid layer formation of the akinete envelope. As expected from the previously described phenotype of the hglB-mutant of Anabaena sp. PCC 7120, the DR752 mutant was also not able to grow on N2 as a sole nitrogen source and formed aberrant heterocysts lacking the laminated layer (Figures 1A,C). Since the DR752 mutant could not synthesize the HGLs (Figure 1B), we confirmed the previous assumption that HglB is an enzyme involved in HGL synthesis, even though the enzymatic activity of HglB has not been explored yet (Awai et al., 2009).
Consistent with the lack of the HGL layer, the mutant DR752 was not able to fix N2 in presence of oxygen, a phenotype described as Fox– (Ernst et al., 1992; Campbell et al., 1997; Fan et al., 2006), because unable to provide microoxic conditions to protect the nitrogenase (Figure 2A). Consistently, nitrogenase activity was observed only under anoxic conditions. It has been shown that under anoxic conditions a second molybdenum-dependent nitrogenase is expressed also in vegetative cells of A. variabilis (Thiel et al., 1995, 2014), and is likely to contribute to a significant amount of activity under anoxic condition in the mutant as in the WT (Figure 2B). These observations support the non-functionality of the mutant heterocyst owing to the gas-permeability of cell envelope. The genome rearrangement within the nifD-gene (Brusca et al., 1989) is obviously not under control of oxygen, and can take place also in the mutant after withdrawal of a nitrogen source (Figure 2C).
Like heterocyst differentiation, akinete differentiation was delayed in the mutant and mature looking akinetes were detected much later compared to the WT (Figure 3A). Obviously, the state of the envelope formation has an influence on the maturation process of akinetes, but how this is sensed and influences the downstream signaling cascade needs to be further explored. However, an immediate downstream gene hetN, which was shown as a suppressor gene of heterocyst differentiation in Anabaena sp. PCC 7120 (Callahan and Buikema, 2001), was found to be constitutively expressed in DR752 mutant in contrast to the WT, where we observed its upregulation only after nitrogen step-down (Supplementary Figure 3). This may be due to the strong psbA- promoter of the CK.3 cassette (Elhai and Wolk, 1988) in the DR752 mutant, which might cause polar effects on the downstream genes. However, the hetN gene is not involved in glycolipid synthesis and we believe that its possible involvement can only be in delaying the heterocyst differentiation observed in the mutant. Whether hetN overexpression is also responsible for delayed akinete differentiation needs to be investigated in future.
For heterocyst envelope formation, it is well known that the envelope builds up in a highly regulated and ordered process [reviewed in: (Maldener et al., 2014; Flores et al., 2019)]. Electron micrographs and chemical characterization of the akinete envelope in A. variabilis and N. punctiforme showed that the laminated layer is composed of the heterocyst glycolipid HG26-diol (Perez et al., 2018). The HGLs are found in a plethora of heterocyst-forming cyanobacteria (Reddy, 1983; Gambacorta et al., 1998; Wörmer et al., 2012; Bauersachs et al., 2014), but they were also identified in akinetes of Cyanospira rippkae (Soriente et al., 1993), consistent with the perception that HGLs are not exclusive to heterocyst, but also found in akinetes (Wörmer et al., 2012). In addition, the outermost akinete envelope consists of the same polysaccharide material that comprises the HEP layer of the heterocyst envelope (Cardemil and Wolk, 1979; Leganés, 1994; Perez et al., 2018) thus, emphasizing the evolutionary and developmental relationships between these two specialized cells (Wolk et al., 1994).
In addition to the chemical and structural identity of the laminated layer of both cell types, our study indicates that akinetes and heterocysts use an overlapping biosynthetic pathway to produce the glycolipid envelope, involving the putative polyketide synthase HglB. To our knowledge, hglB (Ava_ 2595) from A. variabilis is the first known gene involved in the envelope formation of akinetes. In this respect, it would be interesting to analyze akinete formation of the previously described hglE mutant of N. punctiforme, which is lacking the HGLs in heterocysts and is affected in diazotrophic growth (Campbell et al., 1997).
To illustrate the importance of the glycolipid layers of the akinete cell envelope in stress tolerance and survival, we exposed the akinetes of WT and the hglB-mutant to unfavorable conditions. The significantly reduced resistance to freezing in the mutant, especially when using multiple freeze-thaw cycles, could be the consequence of a reduced cell wall stability (Figures 5A,B). Since, water expands when forming ice, the cell must withstand extreme pressure. In the mutant lacking the glycolipid layers the cell envelope is likely less stable. Indeed, the mutant akinetes and the vegetative cells lysed more easily upon freezing (visible by blue color of the medium; not shown), making them less viable in sub-zero environments. While the WT akinetes survived desiccation easily, the germination efficiency of the mutant was severely reduced (Figure 5A). This emphasizes the importance of the HGLs for resistance to desiccation, suggesting that the glycolipid layers prevent water efflux during dry periods. In bacteria, it is reported that desiccation causes hyperosmotic stress characterized by water loss, membrane disorganization and accumulation of reactive oxygen species and ultimately leads to the cell death (Potts, 1994; Greffe and Michiels, 2020). The reduced resistance to exogenous H2O2 could be explained by a thinner physical protective envelope in the mutant akinetes, which makes them more susceptible to damage by reactive oxygen species. The akinetes of the mutant are found to be less stable against any tested stress conditions, except the exposure to cold. Although the mutant develops defective akinetes, they are nevertheless more resistant to extreme environmental influences than vegetative cells due to the presence of the extracellular polysaccharide layer and intracellular adaptations (Argueta and Summers, 2005; Sukenik et al., 2015; Perez et al., 2016). Expectedly, the vegetative cells, which do not have an extra protecting cell envelope, were less resistant to any of the stress factors applied and only able to withstand cold for 20 days of incubation, conditions, which do not mimic cold winter seasons. In nature, cyanobacteria live in community with eukaryotic predators and other competing bacteria, which secrete proteases and cell wall lysing enzymes. Lysozyme, is a peptidoglycan degrading muramidase, which destroys the cell walls of bacteria (Ragland and Criss, 2017). The thick envelope of akinetes is capable to protect the dormant cells from lysozyme (Jensen and Sicko, 1971; Argueta and Summers, 2005) but the fully developed envelope with the glycolipid layers is a prerequisite for this (Figure 5C). Altogether, this study highlights the role of glycolipids in protecting the akinetes in harsh environments with changing conditions.
In summary, this study has established the diverse role of hglB in akinete and heterocyst envelope formation in A. variabilis. Additionally, we showed that glycolipid layers are necessary to protect the akinetes from various stress factors. The sharing of similar envelope-structures indicates that the developmental processes for akinetes and heterocysts occur in a similar way but fulfill different functions in these two specialized cell types of multicellular cyanobacteria. Further genes known to be involved in heterocyst envelope formation should be studied in the future to confirm the common biosynthetic pathways in heterocyst and akinetes and to learn more about their function in survival of dormant cells. It would also be interesting to understand the upstream divergent signaling pathways and to which point they converge.
The raw data supporting the conclusions of this article will be made available by the authors, without undue reservation.
RG designed and performed the experiments, interpreted the data, wrote most of the manuscript, drafted the work, made manuscript revisions, and gave final approval. IM designed and supervised the research, wrote part of the manuscript, made substantial contributions to the design of the work, and made interpretation of data for the work. Both authors contributed to the article and approved the submitted version.
This work was funded by the grant GRK1708 from the Deutsche Forschungsgemeinschaft (DFG, German Research Foundation).
The authors declare that the research was conducted in the absence of any commercial or financial relationships that could be construed as a potential conflict of interest.
We thank Carolina Nishi for construction of mutant strain DR752, Claudia Menzel and Thomas Härtner for technical assistance, and Prof. Karl Forchhammer for helpful discussions. We also thank Libera Lo Presti for critical reading and editing of the manuscript.
The Supplementary Material for this article can be found online at: https://www.frontiersin.org/articles/10.3389/fmicb.2021.645028/full#supplementary-material
Adams, D. G. (2000). Heterocyst formation in cyanobacteria. Curr. Opin. Microbiol. 3, 618–624. doi: 10.1016/S1369-5274(00)00150-8
Adams, D. G., and Duggan, P. S. (1999). Heterocyst and akinete differentiation in cyanobacteria. New Phytol. 144, 3–33. doi: 10.1046/j.1469-8137.1999.00505.x
Allen, M. B., and Arnon, D. I. (1955). Studies on nitrogen-fixing blue-green algae. I. growth and nitrogen fixation by Anabaena cylindrica Lemm. Plant Physiol. 30, 366–372. doi: 10.1104/pp.30.4.366
Argueta, C., and Summers, M. L. (2005). Characterization of a model system for the study of Nostoc punctiforme akinetes. Arch. Microbiol. 183, 338–346. doi: 10.1007/s00203-005-0778-5
Awai, K., Lechno-Yossef, S., and Wolk, C. P. (2009). “Heterocyst envelope glycolipids,” in Lipids in Photosynthesis Essential and Regulatory Functions, eds H. Wada and N. Murata (Dordrecht: Springer), 179–202. doi: 10.1007/978-90-481-2863-1_9
Bauer, C. C., Ramaswamy, K. S., Endley, S., Scappino, L. A., Golden, J. W., and Haselkorn, R. (1997). Suppression of heterocyst differentiation in Anabaena PCC 7120 by a cosmid carrying wild-type genes encoding enzymes for fatty acid synthesis. FEMS Microbiol. Lett. 151, 23–30. doi: 10.1016/S0378-1097(97)00128-6
Bauersachs, T., Mudimu, O., Schulz, R., and Schwark, L. (2014). Distribution of long chain heterocyst glycolipids in N2-fixing cyanobacteria of the order Stigonematales. Phytochemistry 98, 145–150. doi: 10.1016/j.phytochem.2013.11.007
Black, T. A., and Wolk, C. P. (1994). Analysis of a Het- mutation in Anabaena sp. strain PCC 7120 implicates a secondary metabolite in the regulation of heterocyst spacing. J. Bacteriol. 176, 2282–2292. doi: 10.1128/jb.176.8.2282-2292.1994
Bornikoel, J., Staiger, J., Madlung, J., Forchhammer, K., and Maldener, I. (2018). LytM factor Alr3353 affects filament morphology and cell–cell communication in the multicellular cyanobacterium Anabaena sp. PCC 7120. Mol. Microbiol. 108, 187–203. doi: 10.1111/mmi.13929
Braune, W. (1980). Structural aspects of akinete germination in the cyanobacterium Anabaena variabilis. Arch. Microbiol. 126, 257–261. doi: 10.1007/BF00409929
Brusca, J. S., Hale, M. A., Carrasco, C. D., and Golden, J. W. (1989). Excision of an 11-kilobase-pair DNA element from within the nifD gene in Anabaena variabilis heterocysts. J. Bacteriol. 171, 4138–4145. doi: 10.1128/jb.171.8.4138-4145.1989
Callahan, S. M., and Buikema, W. J. (2001). The role of HetN in maintenance of the heterocyst pattern in Anabaena sp. PCC 7120. Mol. Microbiol. 40, 941–950. doi: 10.1046/j.1365-2958.2001.02437.x
Campbell, E. L., Cohen, M. F., and Meeks, J. C. (1997). A polyketide-synthase-like gene is involved in the synthesis of heterocyst glycolipids in Nostoc punctiforme strain ATCC 29133. Arch. Microbiol. 167, 251–258. doi: 10.1007/s002030050440
Campbell, E. L., Hagen, K. D., Cohen, M. F., Summers, M. L., and Meeks, J. C. (1996). The devR gene product is characteristic of receivers of two-component regulatory systems and is essential for heterocyst development in the filamentous cyanobacterium Nostoc sp. strain ATCC 29133. J. Bacteriol. 178, 2037–2043. doi: 10.1128/jb.178.7.2037-2043.1996
Cardemil, L., and Wolk, C. P. (1976). The polysaccharides from heterocyst and spore envelopes of a blue green alga. methylation analysis and structure of the backbones. J. Biol. Chem. 251, 2967–2975.
Cardemil, L., and Wolk, C. P. (1979). The polysaccharides from heterocyst and spore envelopes of a blue-green alga. structure of the basic repeating unit. J. Biol. Chem. 254, 736–741.
Cardemil, L., and Wolk, C. P. (1981). Polysaccharides from the envelopes of heterocysts and spores of the blue-green algae Anabaena variabilis and Cylindrospermum licheniforme. J. Phycol. 17, 234–240. doi: 10.1111/j.1529-8817.1981.tb00845.x
Currier, T. C., and Wolk, C. P. (1979). Characteristics of Anabaena variabilis influencing plaque formation by cyanophage N-1. J. Bacteriol. 139, 88–92. doi: 10.1128/jb.139.1.88-92.1979
Elhai, J., and Wolk, C. P. (1988). Conjugal transfer of DNA to cyanobacteria. Methods Enzymol. 167, 747–754. doi: 10.1016/0076-6879(88)67086-8
Ernst, A., Black, T., Cai, Y., Panoff, J. M., Tiwari, D. N., and Wolk, C. P. (1992). Synthesis of nitrogenase in mutants of the cyanobacterium Anabaena sp. strain PCC 7120 affected in heterocyst development or metabolism. J. Bacteriol. 174, 6025–6032. doi: 10.1128/jb.174.19.6025-6032.1992
Fan, Q., Huang, G., Lechno-Yossef, S., Wolk, C. P., Kaneko, T., and Tabata, S. (2005). Clustered genes required for synthesis and deposition of envelope glycolipids in Anabaena sp. strain PCC 7120. Mol. Microbiol. 58, 227–243. doi: 10.1111/j.1365-2958.2005.04818.x
Fan, Q., Lechno-Yossef, S., Ehira, S., Kaneko, T., Ohmori, M., Sato, N., et al. (2006). Signal transduction genes required for heterocyst maturation in Anabaena sp. strain PCC 7120. J. Bacteriol. 188, 6688–6693. doi: 10.1128/JB.01669-05
Fay, P. (1992). Oxygen relations of nitrogen fixation in cyanobacteria. Microbiol. Rev. 56, 340–373. doi: 10.1128/mmbr.56.2.340-373.1992
Fay, P., and Kulasooriya, S. A. (1972). Tetrazolium reduction and nitrogenase activity in heterocystous blue-green algae. Arch. Mikrobiol. 87, 341–352. doi: 10.1007/BF00409133
Fiedler, G., Arnold, M., Hannus, S., and Maldener, I. (1998a). The DevBCA exporter is essential for envelope formation in heterocysts of the cyanobacterium Anabaena sp. strain PCC 7120. Mol. Microbiol. 27, 1193–1202. doi: 10.1046/j.1365-2958.1998.00762.x
Fiedler, G., Arnold, M., and Maldener, I. (1998b). Sequence and mutational analysis of the devBCA gene cluster encoding a putative ABC transporter in the cyanobacterium Anabaena variabilis ATCC 29413. Biochim. Biophys. Acta - Biomembr. 1375, 140–143. doi: 10.1016/S0005-2736(98)00147-3
Flores, E., and Herrero, A. (2010). Compartmentalized function through cell differentiation in filamentous cyanobacteria. Nat. Rev. Microbiol. 8, 39–50. doi: 10.1038/nrmicro2242
Flores, E., Picossi, S., Valladares, A., and Herrero, A. (2019). Transcriptional regulation of development in heterocyst-forming cyanobacteria. Biochim. Biophys. Acta - Gene Regul. Mech. 1862, 673–684. doi: 10.1016/j.bbagrm.2018.04.006
Gambacorta, A., Pagnotta, E., Romano, I., Sodano, G., and Trincone, A. (1998). Heterocyst glycolipids from nitrogen-fixing cyanobacteria other than Nostocaceae. Phytochemistry 48, 801–805. doi: 10.1016/S0031-9422(97)00954-0
Gibson, D. G., Young, L., Chuang, R. Y., Venter, J. C., Hutchison, C. A., and Smith, H. O. (2009). Enzymatic assembly of DNA molecules up to several hundred kilobases. Nat. Methods 6, 343–345. doi: 10.1038/nmeth.1318
Greffe, V. R. G., and Michiels, J. (2020). Desiccation-induced cell damage in bacteria and the relevance for inoculant production. Appl. Microbiol. Biotechnol. 104, 3757–3770. doi: 10.1007/s00253-020-10501-6
Haury, J. F., and Wolk, C. P. (1978). Classes of Anabaena variabilis mutants with oxygen-sensitive nitrogenase activity. J. Bacteriol. 136, 688–692. doi: 10.1128/jb.136.2.688-692.1978
Jensen, T. E., and Sicko, L. M. (1971). The effect of lysozyme on cell wall morphology in a blue-green alga, Cylindrospermum sp. J. Gen. Microbiol. 68, 71–75. doi: 10.1099/00221287-68-1-71
Kaplan-Levy, R. N., Hadas, O., Summers, M. L., Rücker, J., and Sukenik, A. (2010). Akinetes: dormant cells of cyanobacteria. Top. Curr. Genet. 21, 5–27. doi: 10.1007/978-3-642-12422-8_2
Leganés, F. (1994). Genetic evidence that hepA gene is involved in the normal deposition of the envelope of both heterocysts and akinetes in Anabaena variabilis ATCC 29413. FEMS Microbiol. Lett. 123, 63–67. doi: 10.1016/0378-1097(94)90275-5
Leganés, F., Fernández-Piñas, F., and Wolk, C. P. (1994). Two mutations that block heterocyst differentiation have different effects on akinete differentiation in Nostoc ellipsosporum. Mol. Microbiol. 12, 679–684. doi: 10.1111/j.1365-2958.1994.tb01055.x
Maldener, I., Hannus, S., and Kammerer, M. (2003). Description of five mutants of the cyanobacterium Anabaena sp. strain PCC 7120 affected in heterocyst differentiation and identification of the transposon-tagged genes. FEMS Microbiol. Lett. 224, 205–213. doi: 10.1016/S0378-1097(03)00444-0
Maldener, I., Lockau, W., Cai, Y., and Wolk, C. P. (1991). Calcium-dependent protease of the cyanobacterium Anabaena: molecular cloning and expression of the gene in Escherichia coli, sequencing and site-directed mutagenesis. Mol. Gen. Genet. 225, 113–120. doi: 10.1007/BF00282649
Maldener, I., Summers, M. L., and Sukenik, A. (2014). “Cellular differentiation in filamentous cyanobacteria,” in The Cell Biology of Cyanobacteria, eds F. Enrique and H. Antonia (Norfolk VA: Caister Academic Press), 263–291.
Mckinney, R. E. (1953). Staining bacterial polysaccharides. J. Bacteriol. 66, 453–454. doi: 10.1128/jb.66.4.453-454.1953
Muro-Pastor, A. M., and Maldener, I. (2019). “Cyanobacterial heterocysts,” in Encyclopedia of Life Sciences (ELS), (Chichester: Wiley), 1–10. doi: 10.1002/9780470015902.a0000306.pub3
Murry, M. A., Horne, A. J., and Benemann, J. R. (1984). Physiological studies of oxygen protection mechanisms in the heterocysts of Anabaena cylindrica. Appl. Environ. Microbiol. 47, 449–454. doi: 10.1128/aem.47.3.449-454.1984
Murry, M. A., and Wolk, C. P. (1989). Evidence that the barrier to the penetration of oxygen into heterocysts depends upon two layers of the cell envelope. Arch. Microbiol. 151, 469–474. doi: 10.1007/BF00454860
Nichols, J. M., and Adams, D. G. (1982). “Akinetes,” in The Biology of Cyanobacteria, eds N. G. Carr and B. A. Whitton (Oxford: Blackwell), 387–412.
Nicolaisen, K., Hahn, A., and Schleiff, E. (2009). The cell wall in heterocyst formation by Anabaena sp. PCC 7120. J. Basic Microbiol. 49, 5–24. doi: 10.1002/jobm.200800300
Perez, R., Forchhammer, K., Salerno, G., and Maldener, I. (2016). Clear differences in metabolic and morphological adaptations of akinetes of two nostocales living in different habitats. Microbiol. (United Kingdom) 162, 214–223. doi: 10.1099/mic.0.000230
Perez, R., Wörmer, L., Sass, P., and Maldener, I. (2018). A highly asynchronous developmental program triggered during germination of dormant akinetes of filamentous diazotrophic cyanobacteria. FEMS Microbiol. Ecol. 94:fix131. doi: 10.1093/femsec/fix131
Potts, M. (1994). Desiccation tolerance of prokaryotes. Microbiol. Rev. 58, 755–805. doi: 10.1128/mmbr.58.4.755-805.1994
Qiu, Y., Tian, S., Gu, L., Hildreth, M., and Zhou, R. (2019). Identification of surface polysaccharides in akinetes, heterocysts and vegetative cells of Anabaena cylindrica using fluorescein-labeled lectins. Arch. Microbiol. 201, 17–25. doi: 10.1007/s00203-018-1565-4
Ragland, S. A., and Criss, A. K. (2017). From bacterial killing to immune modulation: recent insights into the functions of lysozyme. PLoS Pathog. 13:e1006512. doi: 10.1371/journal.ppat.1006512
Reddy, P. M. (1983). Lipid composition of akinetes, heterocysts and vegetative cells of cyanobacteria. Biochem. und Physiol. der Pflanz. 178, 575–578. doi: 10.1016/s0015-3796(83)80019-5
Rippka, R., Deruelles, J., and Waterbury, J. B. (1979). Generic assignments, strain histories and properties of pure cultures of cyanobacteria. J. Gen. Microbiol. 111, 1–61. doi: 10.1099/00221287-111-1-1
Rippka, R., and Herdman, M. (1985). Division patterns and cellular differentiation in cyanobacteria. Ann. l’Institut Pasteur Microbiol. 136, 33–39. doi: 10.1016/S0769-2609(85)80018-1
Saito, T., and Awai, K. (2020). A polyketide synthase HglEa, but not HglE2, synthesizes heterocyst specific glycolipids in Anabaena sp. PCC 7120. J. Gen. Appl. Microbiol. 66, 99–105. doi: 10.2323/jgam.2019.11.004
Shvarev, D., Nishi, C. N., and Maldener, I. (2019). Glycolipid composition of the heterocyst envelope of Anabaena sp. PCC 7120 is crucial for diazotrophic growth and relies on the UDP-galactose 4-epimerase HgdA. Microbiologyopen 8:e00811. doi: 10.1002/mbo3.811
Shvarev, D., Nishi, C. N., Wörmer, L., and Maldener, I. (2018). The ABC transporter components HgdB and HgdC are important for glycolipid layer composition and function of heterocysts in Anabaena sp. PCC 7120. Life 8:26. doi: 10.3390/life8030026
Soriente, A., Gambacorta, A., Trincone, A., Sili, C., Vincenzini, M., and Sodano, G. (1993). Heterocyst glycolipids of the cyanobacterium Cyanospira rippkae. Phytochemistry 33, 393–396. doi: 10.1016/0031-9422(93)85526-W
Staron, P., Forchhammer, K., and Maldener, I. (2011). Novel ATP-driven pathway of glycolipid export involving TolC protein. J. Biol. Chem. 286, 38202–38210. doi: 10.1074/jbc.M111.269332
Sukenik, A., Kaplan-Levy, R. N., Welch, J. M., and Post, A. F. (2012). Massive multiplication of genome and ribosomes in dormant cells (akinetes) of Aphanizomenon ovalisporum (Cyanobacteria). ISME J. 6, 670–679. doi: 10.1038/ismej.2011.128
Sukenik, A., Maldener, I., Delhaye, T., Viner-Mozzini, Y., Sela, D., and Bormans, M. (2015). Carbon assimilation and accumulation of cyanophycin during the development of dormant cells (akinetes) in the cyanobacterium Aphanizomenon ovalisporum. Front. Microbiol. 6:1067. doi: 10.3389/fmicb.2015.01067
Sukenik, A., Rücker, J., and Maldener, I. (2018). “Dormant cells (akinetes) of filamentous cyanobacteria demonstrate a great variability in morphology, physiology, and ecological function,” in Cyanobacteria: From Basic Science to Applications, eds A. K. Mishra, D. N. Tiwari, and A. N. Rai (Amsterdam: Elsevier), 65–77. doi: 10.1016/B978-0-12-814667-5.00004-0
Sutherland, J. M., Herdman, M., and Stewart, W. D. P. (1979). Akinetes of the cyanobacterium Nostoc PCC 7524: macromolecular composition, structure and control of differentiation. J. Gen. Microbiol. 115, 273–287. doi: 10.1099/00221287-115-2-273
Thiel, T., Lyons, E. M., Erker, J. C., and Ernst, A. (1995). A second nitrogenase in vegetative cells of a heterocyst-forming cyanobacterium. Proc. Natl. Acad. Sci. U.S.A. 92, 9358–9362. doi: 10.1073/pnas.92.20.9358
Thiel, T., and Pratte, B. S. (2014). Regulation of three nitrogenase gene clusters in the cyanobacterium Anabaena variabilis ATCC 29413. Life 4, 944–967. doi: 10.3390/life4040944
Thiel, T., Pratte, B. S., Zhong, J., Goodwin, L., Copeland, A., Lucas, S., et al. (2014). Complete genome sequence of Anabaena variabilis ATCC 29413. Stand. Genomic Sci. 9, 562–573. doi: 10.4056/sigs.3899418
Valladares, A., Herrero, A., Pils, D., Schmetterer, G., and Flores, E. (2003). Cytochrome c oxidase genes required for nitrogenase activity and diazotrophic growth in Anabaena sp. PCC 7120. Mol. Microbiol. 47, 1239–1249. doi: 10.1046/j.1365-2958.2003.03372.x
Valladares, A., Maldener, I., Muro-Pastor, A. M., Flores, E., and Herrero, A. (2007). Heterocyst development and diazotrophic metabolism in terminal respiratory oxidase mutants of the cyanobacterium Anabaena sp. strain PCC 7120. J. Bacteriol. 189, 4425–4430. doi: 10.1128/JB.00220-07
Van Dok, W., and Hart, B. T. (1997). Akinete germination in Anabaena circinalis (Cyanophyta). J. Phycol. 33, 12–17. doi: 10.1111/j.0022-3646.1997.00012.x
Walsby, A. E. (1985). The permeability of heterocysts to the gases nitrogen and oxygen. Proc. R. Soc. London - Biol. Sci. 226, 345–366. doi: 10.1098/rspb.1985.0099
Winkenbach, F., Wolk, C. P., and Jost, M. (1972). Lipids of membranes and of the cell envelope in heterocysts of a blue-green alga. Planta 107, 69–80. doi: 10.1007/BF00398015
Wolk, C. P. (1996). Heterocyst formation. Annu. Rev. Genet. 30, 59–78. doi: 10.1146/annurev.genet.30.1.59
Wolk, C. P., Ernst, A., and Elhai, J. (1994). “Heterocyst metabolism and development,” in The Molecular Biology of Cyanobacteria, ed. D. A. Bryant (Dordrecht: Springer), 769–823. doi: 10.1007/978-94-011-0227-8_27
Wong, F. C. Y., and Meeks, J. C. (2002). Establishment of a functional symbiosis between the cyanobacterium Nostoc punctiforme and the bryophyte Anthoceros punctatus requires genes involved in nitrogen control and initiation of heterocyst differentiation. Microbiology 148, 315–323. doi: 10.1099/00221287-148-1-315
Wörmer, L., Cirés, S., Velázquez, D., Quesada, A., and Hinrichs, K. U. (2012). Cyanobacterial heterocyst glycolipids in cultures and environmental samples: diversity and biomarker potential. Limnol. Oceanogr. 57, 1775–1788. doi: 10.4319/lo.2012.57.6.1775
Keywords: cyanobacteria, Anabaena, heterocyst, akinete, HglB, glycolipids, stress tolerance, evolution
Citation: Garg R and Maldener I (2021) The Dual Role of the Glycolipid Envelope in Different Cell Types of the Multicellular Cyanobacterium Anabaena variabilis ATCC 29413. Front. Microbiol. 12:645028. doi: 10.3389/fmicb.2021.645028
Received: 04 January 2021; Accepted: 15 March 2021;
Published: 09 April 2021.
Edited by:
Weiwen Zhang, Tianjin University, ChinaReviewed by:
Patrick Jean-Adrien Videau, Southern Oregon University, United StatesCopyright © 2021 Garg and Maldener. This is an open-access article distributed under the terms of the Creative Commons Attribution License (CC BY). The use, distribution or reproduction in other forums is permitted, provided the original author(s) and the copyright owner(s) are credited and that the original publication in this journal is cited, in accordance with accepted academic practice. No use, distribution or reproduction is permitted which does not comply with these terms.
*Correspondence: Iris Maldener, iris.maldener@uni-tuebingen.de
Disclaimer: All claims expressed in this article are solely those of the authors and do not necessarily represent those of their affiliated organizations, or those of the publisher, the editors and the reviewers. Any product that may be evaluated in this article or claim that may be made by its manufacturer is not guaranteed or endorsed by the publisher.
Research integrity at Frontiers
Learn more about the work of our research integrity team to safeguard the quality of each article we publish.