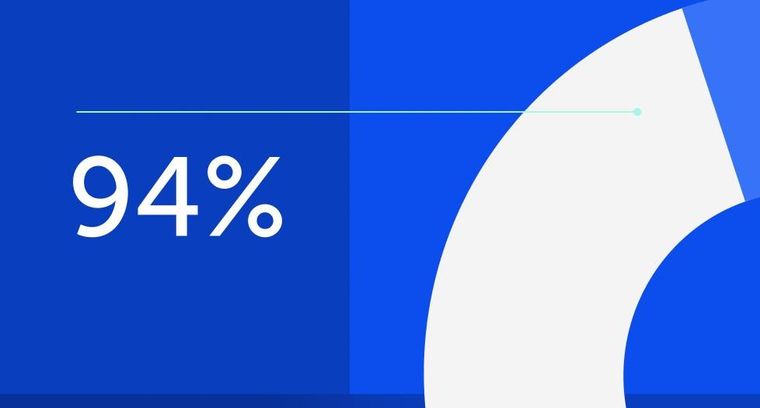
94% of researchers rate our articles as excellent or good
Learn more about the work of our research integrity team to safeguard the quality of each article we publish.
Find out more
ORIGINAL RESEARCH article
Front. Microbiol., 11 March 2021
Sec. Antimicrobials, Resistance and Chemotherapy
Volume 12 - 2021 | https://doi.org/10.3389/fmicb.2021.643498
This article is part of the Research TopicMetabolic Regulation of Drug Resistance and Pathogenicity in Aquatic PathogensView all 13 articles
In this study, a hypothetical protein (ORF02740) secreted by Edwardsiella piscicida was identified. We renamed the ORF02740 protein as EvpQ, which is encoded by a mobile genetic element (MGE) in E. piscicida genome. The evpQ gene is spaced by 513 genes from type VI secretion system (T6SS) gene cluster. Low GC content, three tRNA, and three transposase genes nearby evpQ define this MGE that evpQ localizes as a genomic island. Sequence analysis reveals that EvpQ shares a conserved domain of C70 family cysteine protease and shares 23.91% identity with T3SS effector AvrRpt2 of phytopathogenic Erwinia amylovora. Instead, EvpQ of E. piscicida is proved to be secreted at a T6SS-dependent manner, and it can be translocated into host cells. EvpQ is thereof a novel T6SS effector. Significantly decreased competitive index of ΔevpQ strain in blue gourami fish (0.53 ± 0.27 in head kidney and 0.44 ± 0.19 in spleen) indicates that EvpQ contributes to the pathogenesis of E. piscicida. At 8-, 18-, and 24-h post-subculture into DMEM, the transcription of evpQ was found to be negatively regulated by Fur and positively regulated by EsrC, and the steady-state protein levels of EvpQ are negatively controlled by RpoS. Our study lays a foundation for further understanding the pathogenic role of T6SS in edwardsiellosis.
Edwardsiella piscicida, previously known as Edwardsiella tarda, is a Gram-negative intracellular pathogen (Abayneh et al., 2013; Shao et al., 2015). It causes edwardsiellosis in more than 20 important species of farmed fish, especially flounder (Paralichthys olivaceus) and turbot (Scophthalmus maximus) (Matsuyama et al., 2005; Padrós et al., 2006; Mohanty and Sahoo, 2007). The survival and replication of E. piscicida in its hosts depends on its type VI secretion system (T6SS) and type III secretion system (T3SS) (Tan et al., 2005; Okuda et al., 2006; Zheng and Leung, 2007).
Srinivasa Rao et al. (2004) identified the Edwardsiella virulent proteins (EVPs) from E. piscicida PPD130/91 in a mass spectrometric screen for secreted virulence factors. These EVPs were analogous to the T6SS proteins named first by Pukatzki et al. (2006). E. piscicida T6SS are encoded in a gene cluster which contains 13 conserved core genes encoding proteins necessary to assemble the basic secretion apparatus (Zheng and Leung, 2007). Besides the core apparatus protein, EvpP (T6SS effector protein), EvpC (a homolog of Hcp), and EvpI (a homolog of VgrG) are three secreted proteins encoded inside the T6SS gene cluster (Zheng and Leung, 2007). T6SS is a contractile injection apparatus that translocates a tail tube and a spike loaded with various effectors directly into eukaryotic or prokaryotic target cells (Silverman et al., 2013; Flaugnatti et al., 2016; Hernandez et al., 2020; Wettstadt and Filloux, 2020). The T6SS spike punctures the bacterial cell envelope allowing the transport of effectors, and it consists of a torch-like VgrG trimer (encoded by evpI in E. piscicida) on which sits a PAAR protein sharpening the VgrG tip (encoded by evpJ in E. piscicida), and VgrG itself sits on the Hcp tube (encoded by evpC in E. piscicida). All these elements are packed into a T6SS sheath and are propelled out of the bacterial cell and into target cells. Vibrio cholerae VgrG-1 is the first identified T6SS effector, which is secreted by T6SS and displays a C-terminal actin cross-linking domain upon its translocation into phagocytic target cells (Pukatzki et al., 2007; Ma et al., 2009). EvpP is the first T6SS effector identified in Edwardsiella spp., and it can target intracellular Ca2+ signaling to impair Jnk activation and ASC oligomerization, therefore promoting bacterial colonization (Chen et al., 2017).
Edwardsiella piscicida T6SS plays a pivotal role at the early infection stage in vivo when T3SS expression is repressed by alternative sigma factor RpoS (Yang et al., 2017; Yin et al., 2018). The two-component system sensor PhoQ detects changes of environmental temperature as well as Mg2+ concentration, and the response regulator PhoP regulates the T6SS through direct activation of esrB in E. piscicida (Chakraborty et al., 2010). PhoR-PhoB two-component system senses changes in Pi concentration, and the ferric uptake regulator (Fur) senses changes in iron concentration to regulate the expression of T6SS (Chakraborty et al., 2011). PhoB and EsrC bind simultaneously on DNA upstream of evpA, tightly regulating the transcription of whole T6SS cluster, except the T6SS effector gene evpP. Fur senses high iron concentration and binds directly to the Fur box in the promoter of evpP to inhibit the binding of EsrC to the same region (Chakraborty et al., 2011). The elegantly regulated T6SS contributes to the LD50 of E. piscicida by ∼100 times (Zheng and Leung, 2007).
In this study, the novel T6SS effector, EvpQ, was identified in E. piscicida. EvpQ was revealed to be encoded on a genomic island, and its regulation network and contribution to virulence was characterized.
Edwardsiella tarda PPD130/91 was recognized as Edwardsiella piscicida PPD130/91 ever since 2015 (Ling et al., 2000; Shao et al., 2015). The E. piscicida strains and plasmids used in this study are described in Table 1. E. piscicida strains were grown in TSB medium static. To activate T6SS and T3SS, E. piscicida and its derived strains were grown in Dulbecco’s modified Eagle’s medium (DMEM, Invitrogen) at 25°C under 5% (v/v) CO2 atmosphere. When required, appropriate antibiotics were supplemented at the concentrations of 100 μg/ml ampicillin (Amp; Sigma), 12.5 μg/ml colistin (Col; Sigma), 34 μg/ml chloramphenicol (Cm; Amresco), 15 μg/ml tetracycline (Tet; Amresco), and 50 μg/ml kanamycin (Km; BioFroxx).
Epithelioma papillosum of carp (Cyprinus carpio) (EPC) cells (Wolf and Mann, 1980) were grown in M199 medium (HyClone), supplemented with 10% fetal bovine serum (FBS, Gibco). EPC cells were maintained in a 5% CO2 atmosphere at 25°C.
Using the λ Red recombination system as described previously (Datsenko and Wanner, 2000), the chromosomal copy of evpQ gene was replaced with kanamycin gene, obtaining the strain ΔevpQ::km. The scarless mutant strains ΔevpQ and ΔrpoS were obtained by sacB-based allelic exchange using a method as previously described (Edwards et al., 1998; Zheng et al., 2005). All mutants obtained were verified by DNA sequencing. All primers used are listed in Table 2.
The pACYC-evpQ-HA is derived from pACYC-184 (Amersham), which carries the evpQ-HA gene under the control of tetracyclin resistance gene promoter. To construct, DNA fragment including the ribosome-binding site and complete ORF of evpQ were amplified by PCR from E. piscicida PPD130/91 genomic DNA using primer pair evpQ-com-for and evpQ-com-rev (Table 2). The PCR products, containing EcoRI and ScaI sites, were digested and ligated into pACYC-184 to generate pACYC-evpQ-HA. The evpQ gene together with its ribosome-binding site digested with BamHI and BglII was inserted into pACYC-escE-cyaA (Lu et al., 2016), which was also digested with the same two restriction enzymes, acquiring pACYC-evpQ-cyaA. All the plasmids constructed were verified by DNA sequencing before being introduced into E. piscicida strains by electroporation. The strains obtained were verified by immunoblotting.
To tag the chromosomal copy of evpQ with DNA encoding a double HA epitope, the λ Red recombination system was used as described (Datsenko and Wanner, 2000; Uzzau et al., 2001). Briefly, the forward primer (evpQ-2HA-for) containing the 3’-terminal sequence (without the stop codon) of evpQ followed by a sequence encoding a 2HA epitope and the reverse primer (evpQ-2HA-rev) corresponding to a chromosomal region downstream from evpQ were used to amplify the kanamycin (Km) resistance gene from pSU315. The PCR product was electroporated into competent cells of E. piscicida PPD130/91 wild-type strain, its isogenic mutant strain ΔesrC, ΔrpoS, or Δfur strain, and each of which were transformed with pKD46 (Datsenko and Wanner, 2000). The colonies obtained were screened through probing with anti-HA antibody.
Secreted proteins [extracellular proteins (E)] and bacterial lysates [total bacterial proteins (TBPs)] were prepared as described by Zheng and Leung (2007). ECPs and TBPs were loaded onto a NuPAGE 12% gel for electrophoresis in MES SDS running buffer (Invitrogen). Proteins on sodium dodecyl sulfate polyacrylamide gel electrophoresis (SDS-PAGE) gel were transferred onto polyvinylidene fluoride (PVDF) membrane (Millipore), before being probed with rabbit antibodies against HA (1:2,000) (Sigma), DnaK (1:10,000) (Cusabio Technology LLC), EseG (1:1,000) (Xie et al., 2010), EseE (1:1,000) (Yi et al., 2016), and EvpC (1:5,000) (Zheng and Leung, 2007).
CyaA translocation assay was conducted on EPC cells as previously described (Xie et al., 2015), and cyclic AMP (cAMP) levels were assayed by using an enzyme-linked immunoassay (ELISA) according to the instruction of the manufacturer (ARBOR ASSAYS).
Infected EPC cells were fractionated as described by Coombes et al. (2007) with minor modifications. Briefly, EPC cells were seeded at 6 × 106 cells per 100 mm diameter tissue culture dish 1 day before infection. Before infection, the E. piscicida culture reaching 0.5 at OD540 nm were applied onto EPC cells, at an MOI of 15 in 3 ml M199 medium per dish. Dishes were maintained at 25°C in a 5% CO2 incubator for 30 min before the medium was aspirated, and DMEM supplemented with 16 μg/ml gentamycin was added. Infections were allowed to proceed for another 2 h before the EPC cells were harvested and resuspended in 300 μl homogenization buffer (HB) [250 mM sucrose, 3 mM imidazole, 0.5 mM EDTA (pH 7.4)] supplemented with halt protease inhibitor cocktail (Thermo Fisher Scientific). The cells were homogenized on ice by mechanical lysis using a 1.0-ml syringe with a 22-gauge needle. The lysate was spun for 15 min at 3,000 × g at 4°C. The supernatants were precipitated by methanol and chloroform as described by Wang et al. (2017) and dissolved in 1 × sodium dodecyl sulfate (SDS) loading buffer.
Mixed competitive infection in naïve blue gourami (Trichogaster trichopterus Pallas) (9.54 ± 1.52 g) was performed to determine the contribution of EvpQ to pathogenesis. Overnight-cultured E. piscicida wild-type and ΔevpQ::km strains were subcultured at 1:40, respectively, and cultured at 25°C in TSB for 2.5 h. The bacteria were washed three times in PBS, and the OD540 was adjusted to 0.5. Equal amounts of bacteria were mixed and injected intramuscularly (i.m.) at 1 × 105 CFU per fish. At 48 h post-inoculation, spleen and head kidney were dissected and homogenized, and a series dilution were spread onto TSA plates supplemented with colistin. The colonies obtained were patched onto TSA plate with colistin/kanamysin and TSA plate with colistin to determine the ratio of ΔevpQ::km strain to the wild-type strain. The competitive index (CI) value is the ratio of the ΔevpQ:km strain and wild-type strain within the output divided by their ratio within the input.
The experiments with fish were performed in strict accordance with the recommendations in the Guide for the Care and Use of Laboratory Animals of the Institute of Hydrobiology, Chinese Academy of Sciences.
Probability (P) values were calculated by two-way analysis of variance and least-significant difference (LSD) or Student’s t-test, as stated in the figure legends, and they were considered significantly different if the P-values were less than 0.05.
To understand the strategies that Edwardsiella spp. use to combat host immune system, our previous study searched new proteins secreted by T3SS through comparative proteomics on the extracellular proteins of the ΔesaB and ΔesaBΔesaN strains. This approach is based on the fact that the spiC mutant over-secretes the Salmonella pathogenesis island-2 (SPI-2) effector proteins (Yu et al., 2004). E. piscicida EsaB is homologous with Salmonella SpiC (Liu et al., 2017). EsaN is an ATPase that energizes the transportation of T3SS effectors (Xie et al., 2010). The protein encoded by ORF02740 was found to be slightly hyper-secreted by ΔesaBΔesaN strain than by ΔesaB strain (data not shown). This suggests that ORF02740 is secreted, not depending on T3SS. We named this protein as EvpQ (Edwardsiella virulent protein Q).
To corroborate the secretion of EvpQ from the comparative proteomics, a 2HA tag was introduced at the C-terminal of evpQ on the genome of E. piscicida wild-type 130/91 and its isogenic ΔesaB strain. The secretion of EvpQ was probed with anti-HA antibody. EvpC, a major protein secreted via T6SS was used as a loading control (Zheng and Leung, 2007). As shown in Figure 1, dramatically increased secretion of T3SS effectors EseG and EseJ was detected from the ΔesaB strain as compared with the wild-type strain; however, no secretion difference can be discerned for EvpQ. Chaperone protein DnaK was not detected from the ECPs, indicating that the detection of EvpQ from the ECPs is not due to leakage from bacterial pellets (Figure 1). These results indicate that EvpQ is secreted in a manner different from T3SS effectors.
Figure 1. EvpQ is secreted by Edwardsiella piscicida in a manner different from T3SS effectors. Five percent of bacterial pellets (TBPs) and 10% of culture supernatants (ECPs) from similar amounts of WT evpQ:2HA and ΔesaB evpQ:2HA strains grown in Dulbecco’s modified Eagle’s medium (DMEM) were separated using sodium dodecyl sulfate polyacrylamide gel electrophoresis (SDS-PAGE) and transferred onto polyvinylidene fluoride (PVDF) membranes for probing with anti-HA (EvpQ), anti-EseG, anti-EseJ, anti-DnaK, and anti-EvpC.
The evpQ gene encodes a peptide of 173-amino acid residues with a predicted molecular mass of 20.1 kDa, and a predicated isoelectric (pI) point of 9.3. Homologs of EvpQ were not detected from the other species of Edwardsiella, such as Edwardsiella ictaluri 93–146, Edwardsiella anguillarum ET080813, Edwardsiella hoshinae ATCC 35051, or Edwardsiella tarda KC-Pc-HB1. Using InterPro1 for informative analysis, the EvpQ protein was found to belong to peptidase_C70 super family. SWISS-MODEL analysis reveals that EvpQ shares 23.91% identity with type III effector protein AvrRpt2 from Erwinia amylovora. AvrRpt2 is a T3SS effector that manipulates the host to evade proteolysis (Mudgett and Staskawicz, 1999; Shindo and Van der Hoorn, 2008). The EvpQ protein was thereof speculated to be a novel virulence factor of E. piscicida.
T6SS plays a pivotal role in the pathogenesis of E. piscicida (Zheng and Leung, 2007). To investigate if EvpQ is secreted through T6SS, the pACYC-evpQ-HA was introduced into E. piscicida wild-type PPD130/91, T6SS-deficient ΔevpO strain, and T3SS-deficient ΔesaN strain. The expression of T3SS and T6SS was induced by culturing the aforementioned strains in DMEM. EvpO is an ATPase that energizes the transportation of T6SS substrates (Zheng and Leung, 2007). It was observed that the secretion of EvpQ was dependent totally on T6SS and not through T3SS (Figure 2A). The expression and secretion of T3SS effector EseG and T6SS substrate EvpC were examined as the control. As expected, EseG was not secreted from the ΔesaN strain and EvpC not from the ΔevpO strain, indicating that the strains are correct. EseG was not detected from the ECP of the ΔesaN strain, indicating that the detection of EvpQ from the ΔesaN strain is not due to its leakage from bacterial pellets (Figure 2A). Together, these results demonstrate that EvpQ depends on an active T6SS for its secretion.
Figure 2. EvpQ is secreted at a T6SS-dependent manner and is translocated into eukayotic host. (A) EvpQ is secreted at a T6SS-dependent manner. TBPs and ECPs from each strain grown in DMEM were harvested at stationary phase, and equal amount of TBPs and ECPs were loaded for separating by SDS-PAGE and transferred onto PVDF membranes for immunoblotting. (B) Extracellular and intracellular profiles of E. piscicida strains. ECPs from similar amounts of E. piscicida strains grown in DMEM were separated using SDS-PAGE and stained with Coomassie blue. EvpI, EvpP, and EvpC are T6SS proteins secreted. (C) EvpQ does not depend on T3SS for its translocation. The EPC cells were infected with E. piscicida strains transformed with pACYC-evpQ::cyaA. At 2 h post-infection, the EPC monolayers were processed to examine the intracellular cAMP level as described in section “Materials and Methods.” Means ± SD from one representative experiment was shown. * **P < 0.001; NS, not significant. (D) EvpQ is translocated into host cells. EPC cells were infected, respectively, with WT evpQ::2HA strain and ΔevpQ strain before fractionation by mechanical disruption. Cell lysates of EPC cells and bacteria were analyzed by immunoblotting using anti-HA, anti-EseE, and anti-actin antibodies. Lanes 1–2, E. piscicida cell lysates of WT evpQ::2HA strain and ΔevpQ strain, respectively; lane 3, protein marker; lanes 4–5, cell lysates of EPC cells infected, respectively, with WT evpQ::2HA strain and ΔevpQ strain.
Some regulators can also be secreted through bacterial secretion system, and deletion of regulator gene changes the extracellular protein profile (Rietsch et al., 2005). To learn whether EvpQ could be a secreted regulator, we examined the extracellular and intracellular protein profiles (ECPs and TBPs) of E. piscicida wild-type strain, ΔevpQ strain, and ΔevpQ/pACYC-evpQ-HA strain. As shown in Figure 2B, depletion or overexpression of EvpQ did not change the extracellular or intracellular protein profile of the E. piscicida strains. This result suggests that EvpQ is not a secreted regulator, and it could be a T6SS effector.
Will EvpQ be translocated into host cells? To answer this question, we constructed a reporter plasmid pACYC-evpQ::cyaA, expressing a chimeric protein EvpQ::CyaA, as described by Lu et al. (2016). When the CyaA fusion is translocated into the host cell cytoplasm, CyaA will convert ATP into cAMP in the presence of the eukaryotic-cell cytoplasmic protein calmodulin. The increase of cAMP is taken as the translocation of EvpQ protein. This method has been used in many pathogenic bacteria, including E. piscicida, to successfully monitor the translocation of bacterial T3SS effectors (Sory and Cornelis, 1994; Casper-Lindley et al., 2002; Xie et al., 2015). The pACYC-evpQ::cyaA was introduced into E. piscicida strains to infect EPC cells. At 2 h post-infection (hpi), the cAMP levels inside EPC cells were measured as a readout of translocation of EvpQ::CyaA. As shown in Figure 2C, the cAMP level in WT/pACYC-eseG::cyaA-infected cells was examined as the positive control (423.46 ± 70.12 fmol/μg protein), and in ΔesaN/pACYC-eseG::cyaA-infected cells as the negative control (18.86 ± 8.75 fmol/μg protein). It was found that the cAMP level in WT/pACYC-evpQ::cyaA-infected cells was 7.97 ± 3.59 fmol/μg protein, in ΔesaN/pACYC-evpQ::cyaA-infected cells was 17.65 ± 7.27 fmol/μg protein, and in ΔevpO/pACYC-evpQ::cyaA-infected cells was 4.45 ± 1.89 fmol/μg protein. No significant difference of cAMP levels was detected from the three strains examined. These data demonstrate that EvpQ is not translocated into host cells in a T3SS-dependent manner. However, we could not draw a conclusion that EvpQ cannot be translocated into host cells through T6SS. Considering that the cAMP system was primarily set up for T3SS effector identification (Sory and Cornelis, 1994), moreover, EvpQ is fused at the N-termini of CyaA (501 aa), this results in a large fusion protein, which could have blocked its translocation through T6SS tail tube.
Next, we labeled evpQ on the genome with 2HA tag (18 aa) using the λ Red recombinase method (Datsenko and Wanner, 2000). To localize EvpQ in infected host cells, EPC cells infected with the E. piscicida WT evpQ::2HA and ΔevpQ strains were subjected to mechanic fractionation. Actin was used as marker of host cell component in EPC cells. EseE, a T3SS chaperone of EseC (Yi et al., 2016), was used as a marker of bacterial components. EvpQ-2HA, but not EseE, was detected from the supernatants of the infected EPC cell lysates (cytosol and membrane) (Figure 2D). This indicates that this fraction was not contaminated by the bacteria. This data provide evidence on the translocation of EvpQ into EPC cells. Taken together, we have demonstrated that EvpQ is a T6SS effector that is translocated into eukaryotic host cells.
The role of EvpQ in the pathogenesis of E. piscicida was investigated in blue gourami infection model by using CI assay. The equal number of the ΔevpQ:km strain and wild-type strain were mixed to inject blue gourami fish i.m. at 1 × 105 CFU per fish. At 48 h post-inoculation, spleen and head kidney were dissected to determine the amount of ΔevpQ:km and wild-type strains. The CIs from head kidney were 0.53 ± 0.27, and the CIs from spleen were 0.44 ± 0.19 (Figure 3). As the CIs were significantly less than 1.0, we conclude that the deletion of evpQ significantly attenuates the virulence of E. piscicida PPD130/91.
Figure 3. The ΔevpQ strain is less competitive than wild-type E. piscicida. Six naïve blue gourami fish were injected intramuscularly with a mixture of equal numbers of wild-type and ΔevpQ::km strains and killed 48 h post-injection. CIs from spleen and head kidney were given for individual fish, and means ± SD were shown by the horizontal lines. Student’s t test was used to calculate the P-value with the hypothetical mean of 1.0. ***P < 0.001; **P < 0.01.
With the help of MGE, many bacteria have accessorized their genomes with DNA from bacteria outside of their species or genus. The most common MGEs of horizontal transfer are genomic islands (GIs), plasmids, and bacteriophages. GIs are areas of the genome that are flanked by specific DNA sequences, which contain direct repeats and are often inserted in highly conserved genes, e.g., tRNA genes. The specific DNA sequences also carry genes coding for genetic mobility such as transposases (Jackson et al., 2011). The evpQ gene (orf 02740) is spaced by 513 genes from evpP (orf 03254), the first gene of the T6SS gene cluster. Three consecutive tRNA genes and three IS903 transposase genes were found upstream of evpQ (Figure 4). Moreover, by the DNAstar analysis, the mean GC content of this MGE that evpQ localizes is 49.0%, which is much lower than that of the genome of E. piscicida PPD130/91 (59.8%). This indicates that EvpQ is encoded by horizontal transferred genomic island. Besides, TssA was also encoded by the same the GI. TssA protein is reported to play a role in coordinating T6SS inner tube/sheath assembly (Dix et al., 2018). This indicates that the genomic island is probably a pathogenicity island.
Figure 4. EvpQ is encoded by a genomic island far outsides T6SS gene cluster. Gene organization of T6SS gene cluster and the GI that encodes EvpQ. Data were from reference (Zheng and Leung, 2007) and our laboratory. Open reading frames with identifiable orthologs in the horizontal transferred fragment are labeled. Yellow arrows, T6SS effector genes; azure arrows, T6SS apparatus genes; green arrows, transposase genes; orange arrows, tRNA genes; white arrows, hypothetical genes.
RpoS inhibits E. piscicida T6SS by blocking RpoD-mediated transcription of esrB (Yin et al., 2018). EsrB positively regulates T6SS through EsrC (Tan et al., 2005; Zheng et al., 2005; Chakraborty et al., 2011). EsrC and Fur compete to bind directly to the Fur box in the promoter of evpP (Chakraborty et al., 2011). To learn whether these proteins also regulate the transcription of the novel T6SS effector EvpQ, a DNA fragment (bp -594 to -1) upstream of evpQ was inserted into the promoterless gfp shuttle vector pFPV25 to create pFPV-594 to -1. The resulting construct (pFPV-594 to -1) was introduced into E. piscicida WT, ΔrpoS, Δfur, ΔesrB, and ΔesrC strain, respectively. At 8-, 18-, and 24-h post-subculture (hps) in DMEM, the activity of this putative promoter in the aforementioned strains were tested with a microplate reader (Synergy neo2, Biotek, United States). As seen in Figure 5A, similar levels of fluorescent intensity were detected from the ΔrpoS strain and wild-type strain, whereas dramatically elevated fluorescence intensity was detected from the Δfur strain, and sharply decreased levels of fluorescence intensity were detected in the ΔesrC strain at each time point examined (8, 18, and 24 hps). These data indicate that when culturing in DMEM, evpQ is negatively regulated by Fur and positively regulated by EsrC; however, RpoS seems not to be involved in the transcription of evpQ.
Figure 5. EvpQ is under the control of EsrC, RpoS, Fur, and temperature. (A) Comparison of the transcriptional level of evpQ in WT, ΔesrC, ΔrpoS, and Δfur strains. The DNA fragment (bp -594 to -1) upstream of evpQ was inserted into the promoterless gfp shuttle vector pFPV25 to create pFPV-evpQ–594 to –1. This plasmid was then introduced into E. piscicida strains. At 8, 18, and 24 hps in DMEM, the fluorescence intensity of those E. piscicida strains were evaluated by using a microplate reader. Means ± SD from one representative experiment was shown. ***P < 0.001; **P < 0.01; NS, not significant. (B) Examination on the steady-state protein level of EvpQ in different E. piscicida strains at 8-, 18-, and 24-h post-subculture in DMEM. This experiment was repeated separately for at least three times, and one representative blot was shown. (C) The expression and secretion of EvpQ from E. piscicida wild type cultured in DMEM at different temperatures. ECPs and TCPs from similar amounts of the WT evpQ:2HA cultured at 25, 35, or 37°C were probed with anti-HA, anti-EvpC, anti-EseG, and anti-DanK antibodies. (D) The transcription levels of evpQ from E. piscicida wild type cultured in DMEM at different temperatures. The mRNA levels of evpQ from the wild-type strain cultured at 25, 35, or 37°C were examined by qRT-PCR. 16S rRNA was used as the reference gene. Transcription levels of evpQ relative to that of 16S rRNA are presented (i.e., relative fold changes in gene expression). Data are presented as means ± SD. **P < 0.01.
The steady-state protein level of EvpQ in the aforementioned strains was examined. As shown in Figure 5B, similar levels of steady-state EvpQ were detected from WT, ΔrpoS, and Δfur strain at 8 hps. At 18 or 24 hps, the steady-state protein level of EvpQ increased slightly in WT or Δfur strain, meanwhile it increased starkly in ΔrpoS strain and reached maximum at 24 hps. As a control, protein level of type III effector EseG was examined. It was found that EseG was also negatively controlled by RpoS. The highest protein level of EseG was detected in ΔrpoS strain at 8 hps, and it deceases with its growth. Equal amounts of proteins were loaded per lane as indicated by the DnaK protein level. These data indicate that both EvpQ and EseG are negatively controlled by RpoS, and intracellular EvpQ increases while EseG decreases with its growth.
Edwardsiella piscicida PhoQ sensor domain detects temperatures through a conformational change of its secondary structure, and it regulates the types III and VI secretion systems through direct activation of esrB (Chakraborty et al., 2010). E. piscicida types III and VI secretion systems are activated from 23 to 35°C, but they are suppressed at or below 20°C, at or above 37°C. Will the protein level of EvpQ be subject to temperature? To answer this question, we compared the intracellular and extracellular protein levels of EvpQ at 25, 35, and 37°C from the WT evpQ::2HA strain cultured in DMEM. As shown in Figure 5C, the expression and secretion of EvpQ decreased sharply at 35°C as compared with that at 25°C, and EvpQ almost failed to be detected from TBP and ECP when WT evpQ::2HA strain was cultured at 37°C. Meanwhile, the secretion level of T3SS effector EseG mildly decreased at 35°C as compared with that at 25°C. This indicates that T6SS effector EvpQ is more susceptible to temperature change than T3SS effector EseG.
To investigate whether the decreased steady-state protein level of EvpQ resides in the decreased transcription level of evpQ when the culture temperature increases. We compared the transcript levels of evpQ gene from the wild-type strain cultured at 25, 35, and 37°C, respectively. 16S rRNA was used as the reference gene. The transcript levels of evpQ were significantly (P < 0.01) downregulated by 265- and 21-fold, respectively, at 37 and 35°C when comparing with that at 25°C, and downregulated by 13-fold when comparing between 37 and 35°C (Figure 5D). Thus, the deceased expression level of EvpQ is due to the decreased transcription of evpQ with the elevation of culture temperature.
T6SS is a versatile machinery playing roles in both pathogenesis and interbacterial competition by translocating T6SS effectors. T6SS effectors are loaded into the inner tube or associate with the spike trimer during T6SS assembly (Silverman et al., 2013; Flaugnatti et al., 2016; Hernandez et al., 2020). Contraction of the sheath propels the inner tube/spike, which allows perforation of the target cell membrane, delivering effectors into the cytosol of target cells (Journet and Cascales, 2016). Sory and Cornelis (1994) set up a system to examine the translocation of T3SS effector. By this method, effector candidate was fused at the N-terminal of CyaA, and CyaA converts ATP into cAMP in the presence of calmodulin when the fusion protein is translocated into host cell cytoplasm. The increase in cAMP of taken as the translocation of T3SS effector candidate. In this study, EvpQ was fused at the N-terminal of CyaA (501 aa), while we failed to detect the translocation of EvpQ::CyaA into EPC cells. It is speculated that this method may not be applicable to indicate the translocation of T6SS effector. On one aspect, the large size of the fusion protein may block its translocation through T6SS inner tube; on the other aspect, the fusion of CyaA to EvpQ could have changed the ability of EvpQ to attach to spike trimer, thereof disabling its translocation. When 2HA tag (18 aa) was fused at the C-terminal of evpQ on the genome, the translocation of EvpQ::2HA (191 aa) was successfully detected by mechanic fractionation of EPC cells infected. EvpQ::2HA could have been translocated through the T6SS tail tube.
EsrB elaborately controls T6SS expression through EsrC (Zheng et al., 2005). Fur requires iron binding for its activity, and the activated Fur binds to promoters of iron-responsive genes to repress their transcription under iron replete conditions (Escolar et al., 1999). Fur was reported to represses Salmonella SPI-2 expression that is activated inside macrophages and under acidic conditions (Choi et al., 2014). In E. piscicida, Fur senses high iron concentration and binds directly to the Fur box in the promoter of evpP to inhibit EsrC’s binding to the same region (Chakraborty et al., 2011). EvpQ was negatively regulated by Fur and positively regulated by EsrC. Whether Fur and EsrC compete in binding with the promoter of EvpQ awaits further study. Moreover, we revealed in this study that the steady-state protein level of EvpQ was negatively controlled by RpoS when E. piscicida was cultured in DMEM. Accordingly, Yin et al. (2018) revealed that ETAE_2037 of E. piscicida EIB202 (renamed as EvpQ in the current study) was negatively regulated by the sigma factor RpoS when cultured in DMEM, as revealed by RNAseq. Our study failed to detect the difference in the transcription of evpQ between wild-type and ΔrpoS strains at 8 and 24 hps. This is probably due to the low sensibility of fluorescence detection system, when comparing with the RNAseq assay.
Based on references and our results, we drew a schematic diagram to summarize regulatory role of EsrC, RpoS, and Fur on EvpQ and T6SS gene cluster (Figure 6). PhoQ senses the change of the ambient temperature (23∼35°C) and transmits the signal to PhoP, and the phosphorylated PhoP binds directly to the PhoP box within the promoter region of esrB to activate its transcription (Chakraborty et al., 2010). The activated EsrB protein upregulates the transcription of T6SS through EsrC (Zheng et al., 2005: Chakraborty et al., 2010). High concentration of iron activates the Fur protein, the activated Fur binds directly to the Fur box in the promoter of T6SS effector gene evpP, and the binding of Fur inhibits the binding of EsrC to the same region (Chakraborty et al., 2011). The novel T6SS effector evpQ that is localized on the genomic island is also negatively regulated by Fur and positively regulated by EsrC; it remains to be resolved in the near future whether or not EsrC and Fur binds the promoter of evpQ directly. RpoS can block RpoD-mediated transcription of esrB, antagonizing the expression of esrB, thereof inhibiting the expression of E. piscicida T6SS proteins (Yin et al., 2018). RpoS negatively controls the expression of EvpQ. RpoS represses promoters containing a -6G in their respective discriminator sequences (Yin et al., 2018). Whether or not RpoS represses EvpQ through binding to a -6G in the promoter of evpQ awaits further study.
Figure 6. Schematic diagram of regulatory network of EsrC, RpoS, and Fur on EvpQ and T6SS gene cluster in E. piscicida PPD130/91, based on reference papers and our results from the current study. PhoQ senses the change of ambient temperature (23∼35°C) and transmits the signal to PhoP, and the phosphorylated PhoP binds directly to the PhoP box within the promoter region of esrB to activate its transcription (Chakraborty et al., 2010). The activated EsrB protein upregulates the transcription of T6SS through EsrC (Zheng et al., 2005; Chakraborty et al., 2010). High concentration of iron activates the Fur protein, and activated Fur binds directly to the Fur box in the promoter of T6SS effector gene evpP. The binding of Fur inhibits the binding of EsrC to the same region (Chakraborty et al., 2011). The EvpQ encoded by the genomic island is also negatively regulated by Fur and positively regulated by EsrC, while it remains to be resolved whether EsrC and Fur directly bind the promoter of evpQ. The sigma factor RpoS, antagonizing the expression of esrB (Yin et al., 2018), negatively controls the expression of EvpQ.
EvpQ is encoded by a genomic island, and it exists only in E. piscicida but not in any other species of the genus Edwardsiella, such as E. ictaluri, E. anguillarum, E. hoshinae, or E. tarda. Alike EvpQ, GtgE localizes on the Gifsy-2 prophage of Salmonella Typhimurium (Ho et al., 2002). S. Typhimurium can infect a broad range of vertebrate species, whereas Salmonella Typhi only infects humans. GtgE is not encoded by S. Typhi, and introducing GtgE into S. Typhi enables this human-adapted serovar to survive within non-permissive host cells (Spanò and Galán, 2012). E. ictaluri only infects fish in the siluriformes, while E. piscicida infects fish from wide orders. It is interesting to investigate in the future whether introducing EvpQ into E. ictaluri extends its hosts or not.
EvpQ shares similarity with papain-like cysteine protease AvrRpt2, which suppresses plant immunity by modulating auxin signaling and cleavage of the membrane localized defense regulator RIN4 (Axtell et al., 2003; Mackey et al., 2003; Cui et al., 2013). AvrRpt2 also specifically suppresses pathogen-associated molecular pattern (PAMP)-induced activation of MPK4 and MPK11 but not of MPK3 and MPK6 (Eschen-Lippold et al., 2016). Whether EvpQ manipulate and fine-tune MPK signaling pathway upon its translocation into fish hosts awaits further investigation.
The original contributions presented in the study are included in the article/supplementary material, further inquiries can be directed to the corresponding author.
The animal study was reviewed and approved by the Animal Ethical and Welfare Committee, Institute of Hydrobiology, Chinese Academy of Sciences.
DL, YL, XL, TH, and SS performed the experiments and prepared materials for the manuscript. HX and PN designed and supervised the experiments, and interpreted the data. DL, YL, and HX wrote the manuscript. All authors contributed to the article and approved the submitted version.
This work was funded by the National Key Research and Development Program of China (No. 2018YFD0900504) and National Natural Science Foundation of China (No. U1706205).
The authors declare that the research was conducted in the absence of any commercial or financial relationships that could be construed as a potential conflict of interest.
Abayneh, T., Colquhoun, D. J., and Sørum, H. (2013). Edwardsiella piscicida sp. nov., a novel species pathogenic to fish. J. Appl. Microbiol. 114, 644–654. doi: 10.1111/jam.12080
Axtell, M. J., Chisholm, S. T., Dahlbeck, D., and Staskawicz, B. J. (2003). Genetic and molecular evidence that the Pseudomonas syringae type III effector protein AvrRpt2 is a cysteine protease. Mol. Microbiol. 49, 1537–1546. doi: 10.1046/j.1365-2958.2003.03666.x
Casper-Lindley, C., Dahlbeck, D., Clark, E. T., and Staskawicz, B. J. (2002). Direct biochemical evidence for type III secretion-dependent translocation of the AvrBs2 effector protein into plant cells. Proc. Natl. Acad. Sci. U.S.A. 99, 8336–8341. doi: 10.1073/pnas.122220299
Chakraborty, S., Li, M., Chatterjee, C., Sivaraman, J., Leung, K. Y., and Mok, Y.-K. (2010). Temperature and Mg2+ sensing by a novel PhoP-PhoQ two-component system for regulation of virulence in Edwardsiella tarda. J. Biol. Chem. 285, 38876–38888. doi: 10.1074/jbc.m110.179150
Chakraborty, S., Sivaraman, J., Leung, K. Y., and Mok, Y. K. (2011). Two-component PhoB-PhoR regulatory system and ferric uptake regulator sense phosphate and iron to control virulence genes in type III and VI secretion systems of Edwardsiella tarda. J. Biol. Chem. 286, 39417–39430. doi: 10.1074/jbc.m111.295188
Chen, H., Yang, D., Han, F., Tan, J., Zhang, L., Xiao, J., et al. (2017). The Bacterial T6SS effector EvpP prevents NLRP3 inflammasome activation by inhibiting the Ca2+-dependent MAPK-Jnk pathway. Cell Host Microbe 21, 47–58. doi: 10.1016/j.chom.2016.12.004
Choi, E., Kim, H., Lee, H., Nam, D., Choi, J., and Shin, D. (2014). The iron-sensing fur regulator controls expression timing and levels of Salmonella pathogenicity island 2 genes in the course of environmental acidification. Infect. Immun. 82, 2203–2210.
Coombes, B. K., Lowden, M. J., Bishop, J. L., Wickham, M. E., Brown, N. F., Duong, N., et al. (2007). SseL is a Salmonella-specific translocated effector integrated into the SsrB-controlled Salmonella pathogenicity island 2 type III secretion system. Infect. Immun. 75, 574–580.
Cui, F., Wu, S., Sun, W., Coaker, G., Kunkel, B., He, P., et al. (2013). The Pseudomonas syringae type III effector AvrRpt2 promotes pathogen virulence via stimulating Arabidopsis auxin/indole acetic acid protein turnover. Plant Physiol. 162, 1018–1029. doi: 10.1104/pp.113.219659
Datsenko, K. A., and Wanner, B. L. (2000). One-step inactivation of chromosomal genes in Escherichia coli K-12 using PCR products. Proc. Natl. Acad. Sci. U.S.A. 97, 6640–6645. doi: 10.1073/pnas.120163297
Dix, S. R., Owen, H. J., Sun, R., Ahmad, A., Shastri, S., Spiewak, H. L., et al. (2018). Structural insights into the function of type VI secretion system TssA subunits. Nat. Commun. 9:4765. doi: 10.1038/s41467-018-07247-1
Edwards, R. A., Keller, L. H., and Schifferli, D. M. (1998). Improved allelic exchange vectors and their use to analyze 987P fimbria gene expression. Gene 207, 149–157. doi: 10.1016/S0378-1119(97)00619-7
Eschen-Lippold, L., Jiang, X., Elmore, J. M., Mackey, D., Shan, L., Coaker, G., et al. (2016). Bacterial AvrRpt2-like cysteine proteases block activation of the Arabidopsis mitogen-activated protein kinases, MPK4 and MPK11. Plant Physiol. 171, 2223–2238. doi: 10.1104/pp.16.00336
Escolar, L., Perez-Martin, J., and de Lorenzo, V. (1999). Opening the iron box: transcriptional metalloregulation by the Fur protein. J. Bacteriol. 181, 6223–6229. doi: 10.1128/jb.181.20.6223-6229.1999
Flaugnatti, N., Le, T. T., Canaan, S., Aschtgen, M. S., Nguyen, V. S., Blangy, S., et al. (2016). A phospholipase A1 antibacterial type VI secretion effector interacts directly with the C-terminal domain of the VgrG spike protein for delivery. Mol. Microbiol. 99, 1099–1118. doi: 10.1111/mmi.13292
Hernandez, R. E., Gallegos-Monterrosa, R., and Coulthurst, S. J. (2020). Type VI secretion system effector proteins: effective weapons for bacterial competitiveness. Cell. Microbiol. 22:e13241. doi: 10.1111/cmi.13241
Ho, T. D., Figueroa-Bossi, N., Wang, M., Uzzau, S., Bossi, L., and Slauch, J. M. (2002). Identification of GtgE, a novel virulence factor encoded on the Gifsy-2 bacteriophage of Salmonella enterica serovar Typhimurium. J. Bacteriol. 184, 5234–5239. doi: 10.1128/jb.184.19.5234-5239.2002
Jackson, R. W., Vinatzer, B., Arnold, D. L., Dorus, S., and Murillo, J. (2011). The influence of the accessory genome on bacterial pathogen evolution. Mob. Genet. Elements 1, 55–65. doi: 10.4161/mge.1.1.16432
Journet, L., and Cascales, E. (2016). The type VI secretion system in Escherichia coli and related species. EcoSal Plus. 7. doi: 10.1128/ecosalplus.esp-0009-2015
Ling, S. H. M., Wang, X. H., Xie, L., Lim, T. M., and Leung, K. Y. (2000). Use of green fluorescent protein (GFP) to track the invasive pathways of Edwardsiella tarda in the in vivo and in vitro fifish models. Microbiology 146, 7–19. doi: 10.1099/00221287-146-1-7
Liu, L. Y., Nie, P., Yu, H. B., and Xie, H. X. (2017). Regulation of type III secretion of translocon and effector proteins by the EsaB/EsaL/EsaM complex in Edwardsiella tarda. Infect. Immun. 85:e00322-17. doi: 10.1128/iai.00322-17
Lu, J. F., Wang, W. N., Wang, G. L., Zhang, H., Zhou, Y., Gao, Z. P., et al. (2016). Edwardsiella tarda EscE (Orf13 protein) is a type III secretion system-secreted protein that is required for the injection of effectors, secretion of translocators, and pathogenesis in fish. Infect. Immun. 84, 2–10. doi: 10.1128/iai.00986-15
Ma, A. T., McAuley, S., Pukatzki, S., and Mekalanos, J. J. (2009). Translocation of a Vibrio cholerae type VI secretion effector requires bacterial endocytosis by host cells. Cell Host Microbe 5, 234–243. doi: 10.1016/j.chom.2009.02.005
Mackey, D., Belkhadir, Y., Alonso, J. M., Ecker, J. R., and Dangl, J. L. (2003). Arabidopsis RIN4 is a target of the type III virulence effector AvrRpt2 and modulates RPS2-mediated resistance. Cell 112, 379–389. doi: 10.1016/S0092-8674(03)00040-0
Matsuyama, T., Kamaishi, T., Ooseko, N., Kurohara, K., and Iida, T. (2005). Pathogenicity of motile and non-motile Edwardsiella tarda to some marine fish. Fish Pathol. 40, 133–135.
Mohanty, B. R., and Sahoo, P. K. (2007). Edwardsiellosis in fish: a brief review. J. Biosci. 32, 1331–1344.
Mudgett, M. B., and Staskawicz, B. J. (1999). Characterization of the Pseudomonas syringae pv. tomato AvrRpt2 protein: demonstration of secretion and processing during bacterial pathogenesis. Mol. Microbiol. 32, 927–941. doi: 10.1046/j.1365-2958.1999.01403.x
Okuda, J., Arikawa, Y., Takeuchi, Y., Mahmoud, M. M., Suzaki, E., Kataoka, K., et al. (2006). Intracellular replication of Edwardsiella tarda in murine macrophage is dependent on the type III secretion system and induces an up-regulation of anti-apoptotic NF-kappaB target genes protecting the macrophage from staurosporine-induced apoptosis. Microb. Pathog. 41, 226–240. doi: 10.1016/j.micpath.2006.08.002
Padrós, F., Zarza, C., Dopazo, L., Cuadrado, M., and Crespo, S. (2006). Pathology of Edwardsiella tarda infection in turbot, Scophthalmus maximus (L.). J. Fish Dis. 29, 87–94.
Pukatzki, S., Ma, A. T., Revel, A. T., Sturtevant, D., and Mekalanos, J. J. (2007). Type VI secretion system translocates a phage tail spike-like protein into target cells where it cross-links actin. Proc. Natl. Acad. Sci. U.S.A. 104, 15508–15513. doi: 10.1073/pnas.0706532104
Pukatzki, S., Ma, A. T., Sturtevant, D., Krastins, B., Sarracino, D., Nelson, W. C., et al. (2006). Identification of a conserved bacterial protein secretion system in Vibrio cholerae using the Dictyostelium host model system. Proc. Natl. Acad. Sci. U.S.A. 103, 1528–1533. doi: 10.1073/pnas.0510322103
Rietsch, A., Vallet-Gely, I., Dove, S. L., and Mekalanos, J. J. (2005). ExsE, a secreted regulator of type III secretion genes in Pseudomonas aeruginosa. Proc. Natl. Acad. Sci. U.S.A. 102, 8006–8011. doi: 10.1073/pnas.0503005102
Shao, S., Lai, Q. L., Liu, Q., Wu, H. Z., Xiao, J. F., Shao, Z. Z., et al. (2015). Phylogenomics characterization of a highly virulent Edwardsiella strain ET080813T encoding two distinct T3SS and three T6SS gene clusters: propose a novel species as Edwardsiella anguillarum sp. nov. Syst. Appl. Microbiol. 38, 36–47. doi: 10.1016/j.syapm.2014.10.008
Shindo, T., and Van der Hoorn, R. A. (2008). Papain-like cysteine proteases: key players at molecular battlefields employed by both plants and their invaders. Mol. Plant Pathol. 9, 119–125. doi: 10.1111/j.1364-3703.2007.00439.x
Silverman, J. M., Agnello, D. M., Zheng, H., Andrews, B. T., Li, M., Catalano, C. E., et al. (2013). Haemolysin coregulated protein is an exported receptor and chaperone of type VI secretion substrates. Mol. Cell 51, 584–593.
Simon, R., Priefer, U., and Pühler, A. (1983). A broad host range mobilization system for in vivo genetic engineering: transposon mutagenesis in gram negative bacteria. Nat. Biotechnol. 1, 784–791.
Sory, M. P., and Cornelis, G. R. (1994). Translocation of a hybrid YopE adenylate-cyclase from Yersinia enterocolitica into HeLa cells. Mol. Microbiol. 14, 583–594. doi: 10.1111/j.1365-2958.1994.tb02191.x
Spanò, S., and Galán, J. E. (2012). A Rab32-dependent pathway contributes to Salmonella Typhi host restriction. Science 338, 960–963. doi: 10.1126/science.1229224
Srinivasa Rao, P. S., Yamada, Y., Yan, Y. P., and Leung, K. Y. (2004). Use of proteomics to identify novel virulence determinants that are required for Edwardsiella tarda pathogenesis. Mol. Microbiol. 53, 573–586. doi: 10.1111/j.1365-2958.2004.04123.x
Tan, Y. P., Zheng, J., Tung, S. L., Rosenshine, I., and Leung, K. Y. (2005). Role of type III secretion in Edwardsiella tarda virulence. Microbiology 151, 2301–2313. doi: 10.1099/mic.0.28005-0
Uzzau, S., Figueroa-Bossi, N., Rubino, S., and Bossi, L. (2001). Epitope tagging of chromosomal genes in Salmonella. Proc. Natl. Acad. Sci. U.S.A. 98, 15264–15269. doi: 10.1073/pnas.261348198
Valdivia, R. H., and Falkow, S. (1996). Bacterial genetics by flow cytometry: rapid versatile emerging pathogen of fish. Virulence 10, 555–567. doi: 10.1080/21505594.2019.1621648
Wang, X., Gong, P., Zhang, X., Wang, J., Tai, L., Wang, X., et al. (2017). NLRP3 inflammasome activation in murine macrophages caused by Neospora caninum infection. Parasit. Vectors 10:266. doi: 10.1186/s13071-017-2197-2
Wettstadt, S., and Filloux, A. (2020). Manipulating the type VI secretion system spike to shuttle passenger proteins. PLoS One 15:e0228941. doi: 10.1371/journal.pone.0228941
Wolf, K., and Mann, J. A. (1980). Poikilotherm vertebrate cell lines and viruses, a current listing for fishes. In Vitro 16, 168–179. doi: 10.1007/bf02831507
Xie, H. X., Lu, J. F., Zhou, Y., Yi, J., Yu, X. J., Leung, K. Y., et al. (2015). Identification and functional characterization of a novel Edwardsiella tarda effector EseJ. Infect. Immun. 83, 1650–1660. doi: 10.1128/IAI.02566-14
Xie, H. X., Yu, H. B., Zheng, J., Nie, P., Foster, L. J., Mok, Y. K., et al. (2010). EseG, an effector of the type III secretion system of Edwardsiella tarda, triggers microtubule destabilization. Infect. Immun. 78, 5011–5021. doi: 10.1128/iai.00152-10
Yang, G. H., Billings, G., Hubbard, T. P., Park, J. S., Leung, K. Y., Liu, Q., et al. (2017). Time-resolved transposon insertion sequencing reveals genome-wide fitness dynamics during infection. mBio 8:e01581-17. doi: 10.1128/mbio.01581-17
Yi, J., Xiao, S. B., Zeng, Z. X., Lu, J. F., Liu, L. Y., Laghari, Z. A., et al. (2016). EseE of Edwardsiella tarda augments secretion of translocon protein EseC and expression of the escC-eseE operon. Infect. Immun. 84, 2336–2344. doi: 10.1128/iai.00106-16
Yin, K., Guan, Y., Ma, R., Wei, L., Liu, B., Liu, X., et al. (2018). Critical role for a promoter discriminator in RpoS control of virulence in Edwardsiella piscicida. PLoS Pathog. 14:e1007272. doi: 10.1371/journal.ppat.1007272
Yu, X. J., Liu, M., and Holden, D. W. (2004). SsaM and SpiC interact and regulate secretion of Salmonella pathogenicity island 2 type III secretion system effectors and translocators. Mol. Microbiol. 54, 604–619. doi: 10.1111/j.1365-2958.2004.04297.x
Zhang, Q., He, T. T., Li, D. Y., Liu, L. Y., Nie, P., and Xie, H. X. (2019). The Edwardsiella piscicida type III effector EseJ suppresses expression of type 1 fimbriae, leading to decreased bacterial adherence to host cells. Infect. Immun. 87:e00187-19. doi: 10.1128/iai.00187-19
Zheng, J., and Leung, K. Y. (2007). Dissection of a type VI secretion system in Edwardsiella tarda. Mol. Microbiol. 66, 1192–1206. doi: 10.1111/j.1365-2958.2007.05993.x
Keywords: type VI secretion system, effector, EvpQ, regulation, mobile genetic element, Edwardsiella piscicida
Citation: Li DY, Liu YL, Liao XJ, He TT, Sun SS, Nie P and Xie HX (2021) Identification and Characterization of EvpQ, a Novel T6SS Effector Encoded on a Mobile Genetic Element in Edwardsiella piscicida. Front. Microbiol. 12:643498. doi: 10.3389/fmicb.2021.643498
Received: 18 December 2020; Accepted: 05 February 2021;
Published: 11 March 2021.
Edited by:
Bo Peng, Sun Yat-sen University, ChinaReviewed by:
Ka Yin Leung, Guangdong Technion-Israel Institute of Technology, ChinaCopyright © 2021 Li, Liu, Liao, He, Sun, Nie and Xie. This is an open-access article distributed under the terms of the Creative Commons Attribution License (CC BY). The use, distribution or reproduction in other forums is permitted, provided the original author(s) and the copyright owner(s) are credited and that the original publication in this journal is cited, in accordance with accepted academic practice. No use, distribution or reproduction is permitted which does not comply with these terms.
*Correspondence: Hai Xia Xie, eGllaEBpaGIuYWMuY24=
Disclaimer: All claims expressed in this article are solely those of the authors and do not necessarily represent those of their affiliated organizations, or those of the publisher, the editors and the reviewers. Any product that may be evaluated in this article or claim that may be made by its manufacturer is not guaranteed or endorsed by the publisher.
Research integrity at Frontiers
Learn more about the work of our research integrity team to safeguard the quality of each article we publish.