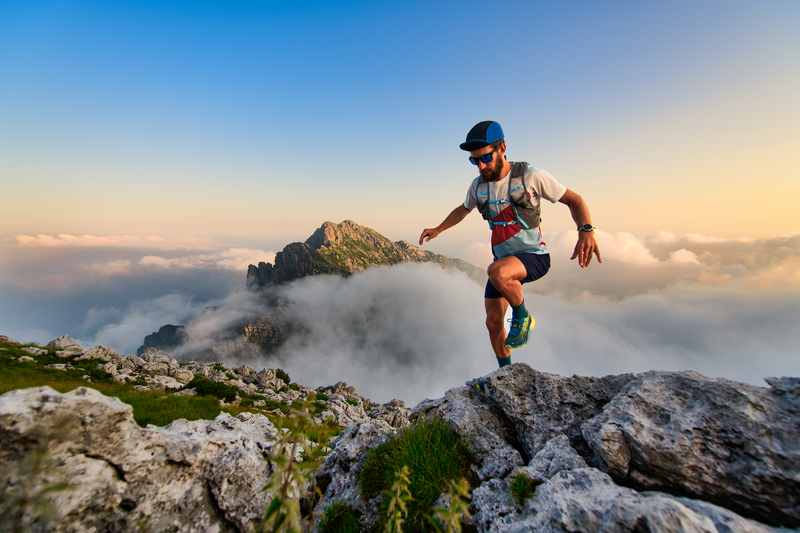
95% of researchers rate our articles as excellent or good
Learn more about the work of our research integrity team to safeguard the quality of each article we publish.
Find out more
ORIGINAL RESEARCH article
Front. Microbiol. , 25 February 2021
Sec. Systems Microbiology
Volume 12 - 2021 | https://doi.org/10.3389/fmicb.2021.641756
This article is part of the Research Topic Actinobacteria: Recent Trends in Genomics, Omics Study and Discovery of Novel Natural Products View all 7 articles
Dalbavancin, vancomycin and chlorobiphenyl-vancomycin share a high degree of structural similarity and the same primary mode of drug action. All inhibit bacterial cell wall biosynthesis through complexation with intermediates in peptidoglycan biosynthesis mediated via interaction with peptidyl-d-alanyl–d-alanine (d-Ala–d-Ala) residues present at the termini of the intermediates. VanB-type glycopeptide resistance in bacteria encodes an inducible reprogramming of bacterial cell wall biosynthesis that generates precursors terminating with d-alanyl–d-lactate (d-Ala–d-Lac). This system in Streptomyces coelicolor confers protection against the natural product vancomycin but not dalbavancin or chlorobiphenyl-vancomycin, which are semi-synthetic derivatives and fail to sufficiently activate the inducible VanB-type sensory response. We used transcriptome profiling by RNAseq to identify the gene expression signatures elucidated in S. coelicolor in response to the three different glycopeptide compounds. An integrated comparison of the results defines both the contribution of the VanB resistance system to the control of changes in gene transcription and the impact at the transcriptional level of the structural diversity present in the glycopeptide antibiotics used. Dalbavancin induces markedly more extensive changes in the expression of genes required for transport processes, RNA methylation, haem biosynthesis and the biosynthesis of the amino acids arginine and glutamine. Chlorobiphenyl-vancomycin exhibits specific effects on tryptophan and calcium-dependent antibiotic biosynthesis and has a stronger repressive effect on translation. Vancomycin predictably has a uniquely strong effect on the genes controlled by the VanB resistance system and also impacts metal ion homeostasis and leucine biosynthesis. Leaderless gene transcription is disfavoured in the core transcriptional up- and down-regulation taking place in response to all the glycopeptide antibiotics, while HrdB-dependent transcripts are favoured in the down-regulated group. This study illustrates the biological impact of peripheral changes to glycopeptide antibiotic structure and could inform the design of future semi-synthetic glycopeptide derivatives.
Glycopeptide antibiotics are important antibacterial agents for the treatment and control of infections caused by Gram-positive pathogens, particularly those that show resistance to other antibiotics. Vancomycin, a natural metabolite first discovered and isolated from cultures of the actinomycete Amycolatopsis orientalis (McCormick et al., 1955), is the pioneer compound in the glycopeptide antibiotic class. Approved for clinical use in 1958, it has since been joined by four additional structurally related natural and semi-synthetic glycopeptides, namely teicoplanin, oritavancin, dalbavancin and telavancin (reviewed in Butler et al., 2014; Blaskovich et al., 2018; Marcone et al., 2018). All these compounds currently find application in the treatment of staphylococcal, enterococcal and clostridial infections, but, as with all antibiotic chemotherapies, they are vulnerable to the development of resistance (Pootoolal et al., 2002).
High-level resistance to vancomycin occurs through an inducible mechanism that reprograms the biosynthesis of intermediates in bacterial cell wall peptidoglycan production (Bugg et al., 1991a, b; Walsh et al., 1996). The primary mode of action of glycopeptide antibiotics is the inhibition of peptidoglycan biosynthesis through complexation with biosynthetic intermediates possessing peptidyl-d-alanyl–d-alanine (d-Ala–d-Ala) residues (Barna and Williams, 1984). Complex formation prevents efficient incorporation of the intermediates into the mature peptidoglycan cell wall structure. Inducible resistance systems generate precursors terminating with d-alanyl–d-lactate (d-Ala–d-Lac) in preference to d-Ala–d-Ala and thereby enable continued peptidoglycan biosynthesis in the presence of vancomycin. Increased tolerance to vancomycin can also be achieved by non-inducible mechanisms. In Staphylococcus aureus, for example, increased cell wall thickness and changes to cell wall composition and turnover are associated with intermediate level glycopeptide antibiotic resistance (Hanaki et al., 1998a, b; Cui et al., 2003; Howden et al., 2010). Conversely, environmental factors can increase sensitivity to glycopeptide activity in highly resistant strains. The presence of high concentrations of inorganic phosphate (>0.25% w/v) sensitises Streptomyces species to vancomycin by hampering transcriptional induction of the resistance genes (Santos-Beneit and Martin, 2013; Santos-Beneit, 2018).
The emergence and spread of vancomycin resistant pathogens in hospitals around the world is an important healthcare concern. The 2019 Antibiotic Resistance Threats Report from the United States’ Centers for Disease Control and Prevention lists VanB-type vancomycin-resistant enterococci in their “serious threat” category (CDC, 2019). VanB-type resistance is defined by inducible resistance to vancomycin but susceptibility to teicoplanin and related analogues (unless vancomycin is also present). Routes to the production of new glycopeptide antibiotics with improved efficacy are continually being sought and include total chemical synthesis (Okano et al., 2014, 2017), chemical or chemoenzymatic derivatisation of glycopeptide scaffolds (Yarlagadda et al., 2015; Blaskovich et al., 2018; MishraI, Stolarzewicz et al., 2018; Tailhades et al., 2020), and the development of synthetic biology platforms (Xu et al., 2020). Successful modification of a glycopeptide structure might result from the generation of molecules with reduced ability to activate the known inducible glycopeptide resistance systems or the conferment of antibacterial activities independent of the interaction with d-Ala–d-Ala residues. There is, however, only a limited understanding of the impact that peripheral changes to the structure of glycopeptide antibiotics might have on their antibacterial activity.
Transcriptome profiling has previously been used as a method to define bacterial responses to large panels of chemically very diverse antibiotics in studies aimed at improving antibacterial discovery pipelines (Boshoff et al., 2004; Hutter et al., 2004; Jones et al., 2017; O’Rourke et al., 2020). Transcriptome profiles of the immediate response to antibiotic challenge, typically within 30-60 min, allowed classification of compounds into mechanism of action (MOA) groups which could be exploited to predict the MOA of new antibiotic candidates. Here we use a similar chemotranscriptomics approach to define the structure-activity relationships among a panel of three very closely related glycopeptide antibiotic structures – dalbavancin, vancomycin and chlorobiphenyl-vancomycin (Figure 1A) – using Streptomyces coelicolor as a biological activity reporter strain. S. coelicolor possesses a well-characterised VanB-type glycopeptide antibiotic resistance system that confers full protection against the natural product vancomycin but not against dalbavancin or chlorobiphenyl-vancomycin (Hong et al., 2004, 2005; Hutchings et al., 2006; Kwun and Hong, 2014). Vancomycin and chlorobiphenyl-vancomycin are visually very similar molecules and score highly on a pairwise Tanimoto chemical similarity index (Figure 1B). They differ, however, in the presence of the hydrophobic chlorobiphenyl group conjugated to the disaccharide moiety through the vancosamine sugar, as also found in oritavancin, which markedly increases its activity against S. coelicolor (Kwun and Hong, 2014). Dalbavancin is a lipoglycopeptide antibiotic and is the least similar of the three molecules (Figure 1). It is a semi-synthetic derivative of a teicoplanin-like natural product and is characterised by an extended lipophilic side chain attached to the monosaccharide moiety and by the presence of a dimethylaminopropyl amide side chain. The chemotranscriptomic profiling results define the impact of the structural diversity present in the glycopeptide antibiotics tested at the level of the bacterium’s transcriptional response. Functional interpretation of the different responses identifies the biological consequences of the differences in structure. A conserved response is also identified and discussed.
Figure 1. The glycopeptide antibiotics used in this study. Structures of vancomycin (Van), chlorobiphenyl-vancomycin (CbpVan) and dalbavancin (Dal), and their corresponding minimum inhibitory concentration (MIC) values against S. coelicolor in liquid cultures (Kwun and Hong, 2014) (A) and pairwise Tanimoto chemical similarity scores (B). In (A), distinctive structural features are highlighted, including the following that are also discussed in the text: N-methylleucine group (green); chlorobiphenyl group (blue); lipid side chain (orange); dimethylaminopropyl amide side chain (red).
Spores of S. coelicolor M600 were germinated and grown to mid-log phase (OD450 nm approx. 0.5) in liquid NMMP medium as described previously (Hong et al., 2004, 2005; Tran et al., 2019). Biological duplicate cultures were treated at this point by adding vancomycin (Sigma), dalbavancin or chlorobiphenyl-vancomycin to a final concentration of 10 μg/ml. A vehicle-only control (dimethylsulphoxide) in which cultures received no antibiotic treatment was also applied. Cultures were incubated for a further 30 min before harvesting for total RNA extraction. The decision to use 10 μg/ml for all three antibiotics (which possess different MICs above and below this threshold) was based on Kwun and Hong, 2014 (Kwun and Hong, 2014) where this dose produced a strong cell wall stress transcriptional response in S. coelicolor (as measured by qRT-PCR of selected reporter genes) in the 30-60 min following treatment with a panel of 26 glycopeptide antibiotics. This was irrespective of whether the compounds used possessed MICs well below or well above 10 μg/ml. A treatment of 10 μg/ml over 30 min is therefore appropriate to capture the immediate response of interest.
Total RNA was isolated from mycelium harvested from 5 ml liquid culture aliquots using the procedure previously described (Tran et al., 2019). RNA concentration, purity and integrity were determined using a NanoDrop 1C (NanoDrop Technologies) spectrophotometer and an Agilent 4200 TapeStation (Agilent Technologies). All samples passed purity quality control checks and exhibited mid-range RNA Integrity Number values. For each sample, 2 μg aliquots were subjected to ribosomal RNA depletion using the Illumina Ribo-Zero rRNA Removal Kit (Bacteria). Successful depletion was verified by analysis on the Agilent TapeStation. Strand-specific sequencing libraries were prepared using the TruSeq stranded mRNA library prep and TruSeq RNA single indexes set A (Illumina). All libraries were checked on the Agilent TapeStation using a D1000 High Sensitivity tape. Libraries were quantified, pooled and subjected to single-end 150 bp sequencing using an Illumina NextSeq500 sequencer with the Med OutPut Illumina kit 1 × 150 cycles. The raw sequencing run was demultiplexed on the Illumina BaseSpace platform, generating fastq.gz files for subsequent analysis. This data is available in the ArrayExpress database1 under accession number E-MTAB-9846.
Raw fastq.gz sequencing data was quality checked using fastqc (v.0.11.8) (Andrews, 2010) and multiqc (v1.7) (Ewels et al., 2016). Gene transcript abundances were determined by pseudoalignment of the raw sequencing reads to a reference transcriptome using kallisto (Bray et al., 2016). The reference transcriptome was generated from the sequence and annotation in genome version GCF_000203835.1_ASM20383v1, plus all the short non-coding RNA sequences (sRNA) reported in Jeong et al. (2016). Raw count data were filtered to remove lowly expressed transcripts (682 removed), and the data were normalised and tested for differential expression using the limma voom pipeline (Ritchie et al., 2015). The limma TREAT approach was applied to test changes for significance against a 50% fold-change threshold, and all p-values were corrected for multiple testing using the Benjamini and Hochberg method (McCarthy and Smyth, 2009). Each glycopeptide antibiotic treatment was referenced to the common control condition. Functional enrichment tests were performed using clusterProfiler (Yu et al., 2012) and the gene ontology Biological Process annotation for S. coelicolor present in AnnotationHub (Morgan and Shepherd, 2020). For the enrichment analyses, genes were assigned to groups according to their combined response to each antibiotic (Supplementary Table 1). Analyses for under- or over-representation of leaderless transcripts and HrdB-dependent transcripts were performed using the fisher.test function in the R stats package (R Core Team, 2020). For this, the transcript groups that showed opposing regulation in response to any two antibiotics were first removed from the data (43 transcripts removed). Gene transcripts were assigned as originating from leaderless, or 5′-UTR leadered, transcription using data for primary transcription start sites published in Jeong et al. (2016). Operon structure was included in the assignments such that, for example, where a leaderless transcript covers transcription of a three gene operon, all three genes are classed as leaderless. Where necessary, operon structure was taken from Charaniya et al. (2007). Dependence of transcription on sigma HrdB was assigned using the data published in Smidova et al. (2019). Pairwise Tanimoto similarity scores for the glycopeptide antibiotic structures were obtained from ChEBI (Hastings et al., 2016).
The global transcriptional responses of S. coelicolor cells to vancomycin, chlorobiphenyl-vancomycin and dalbavancin were captured by treating liquid cultures grown to mid-log phase in a minimal medium for 30 min with 10 μg/ml of each antibiotic. This concentration is below the minimum inhibitory concentration (MIC) for vancomycin, but above that for chlorobiphenyl-vancomycin and dalbavancin (Figure 1A). Previous work defined 30-60 min as the time required to produce a maximal cell wall stress transcriptional response in S. coelicolor to 10 μg/ml of each of a panel of 26 glycopeptide antibiotics possessing MICs in the same 0.1 to 100 μg/ml range (Kwun and Hong, 2014). Antibiotic challenge studies in Escherichia coli and S. aureus have also considered a 30 min exposure time to be ideal for observing compound-specific responses while avoiding indirect effects (Jones et al., 2017; O’Rourke et al., 2020). The experiments were performed in biological duplicate, and the results after RNA sequencing were compared to those obtained from a similar vehicle-only control treatment. Unsupervised clustering of the normalised gene transcript abundance data indicates that exposure to all three glycopeptides generates transcriptional profiles distinct from the untreated cells, but that vancomycin and chlorobiphenyl-vancomycin produce responses more similar to each other than to the response to dalbavancin (Figures 2A,B). Differential expression analysis identified 1242, 1770 and 2315 transcripts significantly differently expressed (adj.P.Val ≤ 0.05) between antibiotic and control treatments for vancomycin, chlorobiphenyl-vancomycin and dalbavancin, respectively, comprising 14–28% of the transcriptome (Supplementary Data 1). Of these, significant up or down regulation in response to all three glycopeptides was conserved for 825 transcripts (∼10% of the transcriptome) (Figures 2C,D). A total of 3040 unique gene transcripts responded significantly to at least one of the antibiotics used.
Figure 2. The transcriptional responses of S. coelicolor cells to exposure to vancomycin (Van), chlorobiphenyl-vancomycin (CbpVan) and dalbavancin (Dal). Principal components analysis (PCA) (A) and hierarchical clustering of Euclidean sample distances (B) indicate closer similarity between the data for Van and CbpVan compared to Dal. Differential expression analysis identifies gene transcripts significantly changing in abundance in response to one or more glycopeptide antibiotic compared to the control (Ctrl) (C). In (B), darker blue grid colours indicate closer similarity between pairs of samples.
Expression of the vanRSJKHAX genes (SCO3589-SCO3596) comprising the VanB-type glycopeptide resistance cluster was significantly up-regulated in response to all three glycopeptide antibiotics, but on average was induced > 32 fold more strongly in response to vancomycin (Figure 3A and Supplementary Figure 1A). Chlorobiphenyl-vancomycin and dalbavancin stimulated similar levels of expression of the resistance system. At the global level, antibiotic-induced changes in transcript abundance relative to the control correlate highly between vancomycin and chlorobiphenyl-vancomycin (R2 = 0.739) and vancomycin and dalbavancin (R2 = 0.551) but the data for the vanRSJKHAX genes is notably deviant (Figure 3A). Vancomycin and chlorobiphenyl-vancomycin, however, produce similar responses in gene transcription controlled by the extracytoplasmic function sigma factor SigE that is a major system for responding to cell wall stress in S. coelicolor (Tran et al., 2019) (Figure 3B and Supplementary Figure 1B). The response of the sigE regulon to dalbavancin was also highly similar, but slightly less well conserved. In contrast, induction of a transcriptional response associated with zinc starvation stress through physicochemical interaction of vancomycin with Zn2+ (Zarkan et al., 2016, 2017) is largely absent in dalbavancin treated cultures and is attenuated in the response to chlorobiphenyl-vancomycin (Figure 3C).
Figure 3. Differences in activation of the VanB-type resistance system (A), the SigE-controlled cell wall stress response (B) and zinc starvation stress response (C). In each panel, the diagonal line illustrates theoretical perfect correlation between the data on each x- and y-axis (slope = 1). In (A), global correlations between the changes induced by each antibiotic treatment are shown (R2 and p-values).
Previous mapping of transcriptional start sites in the S. coelicolor genome identified a notable proportion of gene transcripts, about 20%, that do not possess a 5′-UTR region but start at or adjacent to their translational start sites (Jeong et al., 2016). Such leaderless transcription of genes can impact both the transcriptional and post-transcriptional regulation of gene product formation. Genes whose transcription was significantly altered in response to glycopeptide antibiotic treatment and for which transcription start site data is available were analysed to determine any preference for leadered or leaderless transcription (Table 1). Interestingly, leaderless transcripts are significantly under-represented in the group of genes significantly up-regulated in response to all three glycopeptides and also in the groups down-regulated in response to two or more of the antibiotics used. In contrast, leaderless transcripts are significantly over-represented in the group of genes whose transcription was not changed significantly following exposure to any of the antibiotic treatments.
Table 1. Leaderless transcripts are significantly under-represented in genes responding to glycopeptide antibiotic stress.
The initiation of gene transcription in bacteria requires both RNA polymerase and a specific sigma factor that can recognise the promoter sequence and mediate initiation. The principal sigma factor in S. coelicolor is sigma factor HrdB and is required for cell viability. The regulon of genes controlled by HrdB includes those required for primary metabolism and vegetative cell growth (Smidova et al., 2019). Genes dependent on HrdB for their transcription are significantly over-represented in the groups of genes down-regulated in response to at least one of the glycopeptide antibiotics (Table 2), consistent with a decrease in active growth following the treatments. This corresponds with a significant > 2-fold decrease in transcription of sigma factor HrdB (SCO5820) itself in response to all the glycopeptides studied (Supplementary Data 1). Interestingly, HrdB-dependent transcripts are also significantly under-represented in the group of genes whose expression is not affected by any antibiotic treatment (Table 2).
Table 2. Transcripts down-regulated in response to glycopeptide antibiotic stress are significantly enriched for control by HrdB-dependent promoters.
The significant changes in gene transcription occurring uniquely in response to only one of the three glycopeptide antibiotics studied are summarised in Figure 4, while significant responses to two or more antibiotics are presented in Figure 5. Functional enrichment analysis of the different groups of genes present in this data, based on the S. coelicolor gene ontology biological process (GO BP) annotation, highlights the cellular processes that are affected by the changes in gene expression observed (Figures 4, 5 and Supplementary Data 2). Strikingly, amino acid biosynthesis emerges as a process that is affected differently by each glycopeptide antibiotic. Genes uniquely up-regulated in response to dalbavancin are significantly enriched for amino acid biosynthesis (GO: 0008632 3.00e-03), predominantly those required for glutamate and arginine biosynthesis (GO:0009084 1.06e-06 and GO:0006526 6.74e-04, respectively) (Figure 4 and Supplementary Figure 2). In contrast, up-regulation of tryptophan biosynthesis is uniquely associated with chlorobiphenyl-vancomycin (GO:0000162 1.11e-02), and up-regulation of leucine metabolism is similarly associated with vancomycin treatment (GO:0006551 2.90e-03) (Figure 4 and Supplementary Figure 2). Genes required for histidine biosynthesis are significantly enriched in the group of genes down-regulated in response to both vancomycin and dalbavancin but not to chlorobiphenyl-vancomycin (GO:0000105 5.48e-05) (Figure 5 and Supplementary Figure 2).
Figure 4. Heatmap summarising the transcripts uniquely changing significantly in response to only one of the glycopeptide antibiotics used, relative to the control treatment. Significant biological process categories from gene ontology (GO) functional enrichment analysis of each group of genes are shown to the right (“ns” denotes cases where no significant categories were identified). Complete GO enrichment analysis results are provided in Supplementary Data 2.
Figure 5. Heatmap summarising the transcripts changing significantly in response to two or more of the glycopeptide antibiotics used relative to the control treatment. Significant biological process categories from gene ontology (GO) functional enrichment analysis of each group of genes are shown to the right (“ns” denotes cases where no significant categories were identified). Only groups containing more than 10 transcripts are labelled. Complete GO enrichment analysis results are provided in Supplementary Data 2.
Dalbavancin elicits the most extensive changes in gene transcription of all the glycopeptides studied, with 1109 genes uniquely up- or down-regulated (Figure 2C). The uniquely up-regulated genes are significantly enriched for transport functions (GO:0006810 3.00e-03) and include gene products required for amino acid import, and sugar, phosphate and oligopeptide transport (Figure 4 and Supplementary Figure 3). Genes required for pyrimidine nucleoside biosynthesis are also enriched in this category (GO:0046134 4.64e-03) (Figure 4 and Supplementary Figure 3). The uniquely down-regulated genes are significantly enriched for functions related to haem biosynthesis (GO:0006783 8.67e-06) and the methylation of RNA (GO:0001510 4.27e-04) (Figure 4 and Supplementary Figure 3).
The translational apparatus is considered a limiting factor for the growth of bacterial cells, such that growth rate is directly proportional to the number of ribosomes in a cell. Genes uniquely down-regulated in response to chlorobiphenyl-vancomycin are significantly associated with translation (GO:0006412 2.23e-09) suggesting a more pronounced effect on growth than vancomycin or dalbavancin (Figure 4 and Supplementary Figure 4A). A detrimental effect on ribosome biogenesis is largely absent in the response to vancomycin, but some down-regulation is evident following dalbavancin treatment. The group of genes significantly repressed in the presence of dalbavancin and chlorobiphenyl-vancomycin, but not vancomycin, is also significantly enriched for the translation GO BP category (GO:0006412 9.90e-07) (Figure 5 and Supplementary Figure 4B).
The response conserved between all three glycopeptide antibiotics studied included the significant induction of transcription of 661 genes and the concomitant repression of 164 genes (Figure 2C). Functional enrichment analysis of the up-regulated genes identifies a reorganisation of cellular metabolism associated with cell wall biogenesis, peptidoglycan synthesis, protein catabolism and polysaccharide biosynthesis (Figure 5). A GO category related to transcription initiation is also significantly over-represented in this up-regulated set of genes (GO:0006352 4.04e-04), and these correspond to 16 genes encoding alternative RNA polymerase sigma factors (Supplementary Figure 4C). Each alternative sigma factor recognises a different promoter sequence to that controlled by the primary sigma factor HrdB and thus each specifies initiation of transcription at distinct sets of genes different from the HrdB-dependent gene transcription that is predominantly required for primary metabolism and vegetative growth.
Short non-coding RNA transcripts (sRNAs) form part of the molecular machinery that regulates bacterial gene expression. Jeong et al. (Jeong et al., 2016) have previously identified 230 sRNAs in S. coelicolor that are putatively involved in transcriptional and post-transcriptional regulation of gene product formation, although their roles are not currently well understood. In this work, glycopeptide antibiotic stress caused significant changes in the transcription of 79 sRNAs (Figure 6). This included 21 that were reproducibly induced or repressed by all three glycopeptides studied, but also examples of sRNAs changing uniquely in response to only one.
Vancomycin, chlorobiphenyl-vancomycin and dalbavancin induce distinct transcriptional responses in the VanB-type resistant streptomycete S. coelicolor, despite sharing the same primary mode of antibiotic action and a high degree of chemical similarity. Vancomycin molecules bind Zn2+ ions via interaction with nitrogen and oxygen atoms in the N-terminal methylleucine moiety, a phenomenon initially observed due to the identification of a strong zinc-starvation response by exposure to vancomycin in S. coelicolor (Zarkan et al., 2016, 2017). The absence of a zinc-starvation response following dalbavancin treatment (Figure 3C) correlates with the absence of this methylleucine group in the structure of dalbavancin. Although the zinc-binding methylleucine feature is conserved in chlorobiphenyl-vancomycin, treatment with this glycopeptide produces a much weaker zinc-starvation response compared to vancomycin, presumably due to the presence of the bulky hydrophobic chlorobiphenyl group. Full interaction of vancomycin with Zn2+ proceeds via polymerisation of vancomycin dimers and the chlorobiphenyl group may compromise this dimerisation process. Interestingly, vancomycin also uniquely causes a significant up-regulation of a set of four transcription units encoding putative functions in iron ion homeostasis, suggesting a broader influence of the vancomycin structural features on metal ion homeostasis (Figure 4 and Supplementary Figure 4D).
The chlorobiphenyl group present in the glycopeptide antibiotic oritavancin is reported to perturb cell membrane integrity in Gram-positive bacteria through increased interaction with the lipid bilayer, providing an additional mode of antibiotic activity (Zhanel et al., 2012). While there was no direct evidence for membrane perturbation discernible in the transcriptome data characterising the response of S. coelicolor to chlorobiphenyl-vancomycin, a broader repressive effect on gene transcription required for production of the translational apparatus compared to both vancomycin and dalbavancin was detected (Figure 4 and Supplementary Figure 4). In addition, chlorobiphenyl-vancomycin instigated a unique up-regulation in genes annotated to tryptophan biosynthesis (Figure 4 and Supplementary Figure 2B). This corresponds to the trp2 locus that is within the cda cluster of biosynthetic genes specifying production of the calcium-dependent antibiotic CDA and supplies tryptophan precursors for synthesis of CDA (Hojati et al., 2002). The trp1 locus associated with tryptophan auxotrophy (SCO2036, SCO2037, SCO2039, SCO2043) was not up-regulated, but trpA (SCO2036) was uniquely significantly down-regulated in response to chlorobiphenyl-vancomycin (Supplementary Data 1). The switching on of CDA biosynthesis in S. coelicolor has been proposed to form part of a programmed cell death response (Novotna et al., 2003; Virolle, 2020), and it is interesting to note that significant up-regulation of the cda cluster genes occurs uniquely in response to chlorobiphenyl-vancomycin (Supplementary Figure 5A). A general down-regulation of translation coupled with activation of programmed cell death would be consistent with a more potent activity for chlorobiphenyl-vancomycin, perhaps related to membrane depolarisation.
Dalbavancin is the least structurally similar of the three antibiotics studied (Figure 1) and S. coelicolor cells exposed to dalbavancin were also the least similar at the level of their transcriptome profiles (Figure 2). Important structural features of dalbavancin include the long lipid side chain attached to the monosaccharide moiety and the dimethylaminopropyl amide side chain. The lipid side chain is associated with its activity against VanB-type resistant bacteria where its lipophilic interaction with the cell membrane is proposed to prevent sensing of the glycopeptide by the VanB-type VanS sensor kinase protein that resides in the membrane (Dong et al., 2002; Kwun and Hong, 2014). A marked induction of the VanS-dependent transcription of the vanRSJKHAX genes in the vancomycin resistance cluster in response to dalbavancin was still, however, observed in this study, but was ∼30-fold weaker than that produced by vancomycin itself. This reduced level of activation, also seen with chlorobiphenyl-vancomycin, is presumably insufficient to enable survival. Crystal structure analysis indicates that the positively charged dimethylaminopropyl amide group of dalbavancin remains free after interaction with its d-Ala–d-Ala target, permitting additional interactions to take place with negatively charged molecules such as phospholipid head groups of the bacterial membrane (Economou et al., 2012). Interestingly, transcripts uniquely up-regulated in response to dalbavancin are significantly enriched for transport processes, including the pstABC phosphate transport locus which is ∼2.5-fold induced (Supplementary Figure 3A). Such changes to activated transport mechanisms likely represent compensatory mechanisms acting against the reduced bioavailability of important nutrients or increased damage to the cell envelope. In addition to the general up-regulation of a broad range of transport systems, the dalbavancin response is also characterised by unique effects on amino acid biosynthesis, pyrimidine biosynthesis, RNA methylation and haem biosynthesis (Figure 4 and Supplementary Figures 2A, 3). While these effects are currently difficult to rationalise it is clear that the divergent structure of dalbavancin elicits a more divergent transcriptional response in S. coelicolor, relative to vancomycin or chlorobiphenyl-vancomycin.
A core signature response common to all three glycopeptides was evident, centred around activation of the SigE-regulated cell wall stress response, induction of genes encoding a raft of 16 alternative RNA polymerase sigma factors, an up-regulation of genes with functions related to cell wall biogenesis and reorganisation and an increase in transcripts encoding protein catabolic functions. Interestingly, leaderless transcripts were significantly under-represented amongst both the up and down regulated genes in the core response. Leaderless gene transcription is widespread in bacteria, especially in Actinobacteria where ∼20% of genes are leaderless (Zheng et al., 2011). Absence of a 5′-UTR leader sequence can impact the rate of transcript synthesis and degradation, through altered mRNA stability and reduced opportunities for interaction with regulatory sRNA molecules and also the rate of its translation, through a requirement for a different mechanism of translational initiation and again via the reduced opportunity for interactions with regulatory sRNA. A rigorous assessment of these features in Mycobacerium smegmatis, however, failed to identify a consistent global trend differentiating rates of transcription and translation by leadered or leaderless transcription (Nguyen et al., 2020). The observed preference for leadered transcripts in the induced response to glycopeptide antibiotic stress, and the enrichment of leaderless transcripts in those remaining unchanged following antibiotic challenge (Table 1), suggests that in S. coelicolor the presence of a 5′-UTR may give more reliable dynamic control over gene product formation. Interestingly, this is also observed in the response of S. coelicolor to heat shock stress (Bucca et al., 2018). The conserved elements of the response to all glycopeptides are perhaps surprising given the different outcomes of the different treatments: cells ultimately survive vancomycin exposure and recover active growth, while chlorobiphenyl-vancomycin and dalbavancin treatment leads to stasis and cell death. This is consistent with the selection of the 30 min time period to capture antibiotic-specific responses and avoid indirect effects: these fates have presumably yet to be arrived at in this study, and the cells are transitioning through a common response instigated to try to adapt and survive.
Transcripts significantly down-regulated in response to glycopeptide stress also tend to be dependent on the major vegetative sigma factor HrdB, while those not responding have a tendency to be HrdB-independent (Table 2). This is consistent with the significant down-regulation in transcription of hrdB (SCO5820) in response to all three glycopeptides. Concomitant up-regulation of expression of 16 alternative sigma factor genes, including hrdD (SCO3202) and eight extracytoplasmic function sigma factors, including sigE (SCO3356) and bldN (SCO3323) (Supplementary Figure 4C), can also be envisaged to decrease the proportion of RNA polymerase associating with HrdB and promote transcription initiation away from HrdB-controlled genes. The S. coelicolor genome encodes 65 sigma factors, an unusually large number for a bacterium, most of which are alternative sigma factors controlling transcription of regulons yet to be determined. The results here elucidate the biological role of some of these as stress induced alternative sigma factors. Interestingly, leaderless transcripts are significantly under-represented in HrdB-dependent transcripts (and conversely over-represented in those that are HrdB-independent) (Supplementary Table 2).
Assessing structure-activity relationships through genome-wide profiling depends on the quality of the genome annotation available for the reporter organism. For chemicals with activities against eukaryotic cells, the yeast Saccharomyces cerevisiae is used extensively for this purpose via chemogenomics techniques, relying on the existence of one of the most extensive functional annotations of any genome (Gasch et al., 2000; Giaever et al., 2004; Andrusiak et al., 2012; Lee et al., 2014). The annotation of bacterial genomes, with the possible exception of E. coli, has not yet reached the same level of maturity, and the genome of S. coelicolor is limited both by the presence of a large proportion of genes with no known function and the dependence on computationally inferring functions based on the annotations of homologs present in better studied organisms. Despite these limitations, the current study was able to usefully differentiate the activities of three closely related glycopeptide antibiotic structures.
Transcriptome profiling has a track record in distinguishing bacterial responses to chemically very diverse antibiotics from different mode of action groups. Here we show that it can also usefully be employed to study structure-activity relationships within very closely related compounds possessing the same principal mode of action. The divergent responses observed for the three different glycopeptide antibiotics tested, centred around a core set of changes common to all, indicate that in addition to their shared mode of action, each can have its own additional influences on bacterial physiology that are related to the peripheral differences in their structures. While only a partial interpretation of these differences has been possible – limited by an incomplete understanding of the functional role of all the genes in the S. coelicolor genome – this work indicates the utility of the approach. As the functional annotation of bacterial genomes matures, finer grained interpretations of structure-activity relationships will become possible, providing important context for efforts aimed at designing modifications to improve the activities of useful antibiotics.
This data is available in the ArrayExpress database (http://www.ebi.ac.uk/arrayexpress) under accession number E-MTAB-9846.
H-JH and CS conceived and designed the study. H-JH performed the antibiotic challenge experiments. GB performed the transcriptome sequencing. AH undertook the data processing and analysis. All authors contributed to the writing of the manuscript and to interpretation of the data.
This work was partly funded by the BBSRC, United Kingdom (NPRONET NIBB award POC023 to CS).
The authors declare that the research was conducted in the absence of any commercial or financial relationships that could be construed as a potential conflict of interest.
Dalbavancin and chlorobiphenyl-vancomycin were a kind gift from Daniel Kahne and his coworkers.
The Supplementary Material for this article can be found online at: https://www.frontiersin.org/articles/10.3389/fmicb.2021.641756/full#supplementary-material
Andrews, S. (2010). fastqc: A Quality Control Tool for High Throughput Sequence Data (v.0.11.8). Available online at: http://www.bioinformatics.babraham.ac.uk/projects/fastqc/
Andrusiak, K., Piotrowski, J. S., and Boone, C. (2012). Chemical-genomic profiling: systematic analysis of the cellular targets of bioactive molecules. Bioorg. Med. Chem. 20, 1952–1960. doi: 10.1016/j.bmc.2011.12.023
Barna, J. C., and Williams, D. H. (1984). The structure and mode of action of glycopeptide antibiotics of the vancomycin group. Annu. Rev. Microbiol. 38, 339–357. doi: 10.1146/annurev.mi.38.100184.002011
Blaskovich, M. A. T., Hansford, K. A., Butler, M. S., Jia, Z., Mark, A. E., and Cooper, M. A. (2018). Developments in glycopeptide antibiotics. ACS Infect. Dis. 4, 715–735.
Boshoff, H. I., Myers, T. G., Copp, B. R., McNeil, M. R., Wilson, M. A., and Barry, C. E. III (2004). The transcriptional responses of Mycobacterium tuberculosis to inhibitors of metabolism: novel insights into drug mechanisms of action. J. Biol. Chem. 279, 40174–40184.
Bray, N. L., Pimentel, H., Melsted, P., and Pachter, L. (2016). Near-optimal probabilistic RNA-seq quantification. Nat. Biotechnol. 34, 525–527. doi: 10.1038/nbt.3519
Bucca, G., Pothi, R., Hesketh, A., Moller-Levet, C., Hodgson, D. A., Laing, E. E., et al. (2018). Translational control plays an important role in the adaptive heat-shock response of Streptomyces coelicolor. Nucleic Acids Res. 46, 5692–5703. doi: 10.1093/nar/gky335
Bugg, T. D., Dutka-Malen, S., Arthur, M., Courvalin, P., and Walsh, C. T. (1991a). Identification of vancomycin resistance protein VanA as a D-alanine:D-alanine ligase of altered substrate specificity. Biochemistry 30, 2017–2021. doi: 10.1021/bi00222a002
Bugg, T. D., Wright, G. D., Dutka-Malen, S., Arthur, M., Courvalin, P., and Walsh, C. T. (1991b). Molecular basis for vancomycin resistance in Enterococcus faecium BM4147: biosynthesis of a depsipeptide peptidoglycan precursor by vancomycin resistance proteins VanH and VanA. Biochemistry 30, 10408–10415. doi: 10.1021/bi00107a007
Butler, M. S., Hansford, K. A., Blaskovich, M. A., Halai, R., and Cooper, M. A. (2014). Glycopeptide antibiotics: back to the future. J. Antibiot. (Tokyo) 67, 631–644. doi: 10.1038/ja.2014.111
Charaniya, S., Mehra, S., Lian, W., Jayapal, K. P., Karypis, G., and Hu, W. S. (2007). Transcriptome dynamics-based operon prediction and verification in Streptomyces coelicolor. Nucleic Acids Res. 35, 7222–7236. doi: 10.1093/nar/gkm501
Cui, L., Ma, X., Sato, K., Okuma, K., Tenover, F. C., Mamizuka, E. M., et al. (2003). Cell wall thickening is a common feature of vancomycin resistance in Staphylococcus aureus. J. Clin. Microbiol. 41, 5–14. doi: 10.1128/jcm.41.1.5-14.2003
Dong, S. D., Oberthur, M., Losey, H. C., Anderson, J. W., Eggert, U. S., Peczuh, M. W., et al. (2002). The structural basis for induction of VanB resistance. J. Am. Chem. Soc. 124, 9064–9065. doi: 10.1021/ja026342h
Economou, N. J., Nahoum, V., Weeks, S. D., Grasty, K. C. I, Zentner, J., Townsend, T. M., et al. (2012). A carrier protein strategy yields the structure of dalbavancin. J. Am. Chem. Soc. 134, 4637–4645. doi: 10.1021/ja208755j
Ewels, P., Magnusson, M., Lundin, S., and Kaller, M. (2016). MultiQC: summarize analysis results for multiple tools and samples in a single report. Bioinformatics 32, 3047–3048. doi: 10.1093/bioinformatics/btw354
Gasch, A. P., Spellman, P. T., Kao, C. M., Carmel-Harel, O., Eisen, M. B., Storz, G., et al. (2000). Genomic expression programs in the response of yeast cells to environmental changes. Mol. Biol. Cell 11, 4241–4257. doi: 10.1091/mbc.11.12.4241
Giaever, G., Flaherty, P., Kumm, J., Proctor, M., Nislow, C., Jaramillo, D. F., et al. (2004). Chemogenomic profiling: identifying the functional interactions of small molecules in yeast. Proc. Natl. Acad. Sci. U.S.A. 101, 793–798. doi: 10.1073/pnas.0307490100
Hanaki, H., Kuwahara-Arai, K., Boyle-Vavra, S., Daum, R. S., Labischinski, H., and Hiramatsu, K. (1998a). Activated cell-wall synthesis is associated with vancomycin resistance in methicillin-resistant Staphylococcus aureus clinical strains Mu3 and Mu50. J. Antimicrob. Chemother. 42, 199–209. doi: 10.1093/jac/42.2.199
Hanaki, H., Labischinski, H., Inaba, Y., Kondo, N., Murakami, H., and Hiramatsu, K. (1998b). Increase in glutamine-non-amidated muropeptides in the peptidoglycan of vancomycin-resistant Staphylococcus aureus strain Mu50. J. Antimicrob. Chemother. 42, 315–320. doi: 10.1093/jac/42.3.315
Hastings, J., Owen, G., Dekker, A., Ennis, M., Kale, N., Muthukrishnan, V., et al. (2016). ChEBI in 2016: improved services and an expanding collection of metabolites. Nucleic Acids Res. 44, D1214–D1219.
Hojati, Z., Milne, C., Harvey, B., Gordon, L., Borg, M., Flett, F., et al. (2002). Structure, biosynthetic origin, and engineered biosynthesis of calcium-dependent antibiotics from Streptomyces coelicolor. Chem. Biol. 9, 1175–1187. doi: 10.1016/s1074-5521(02)00252-1
Hong, H. J., Hutchings, M. I., Hill, L. M., and Buttner, M. J. (2005). The role of the novel Fem protein VanK in vancomycin resistance in Streptomyces coelicolor. J. Biol. Chem. 280, 13055–13061. doi: 10.1074/jbc.m413801200
Hong, H. J., Hutchings, M. I., Neu, J. M., Wright, G. D., Paget, M. S., and Buttner, M. J. (2004). Characterization of an inducible vancomycin resistance system in Streptomyces coelicolor reveals a novel gene (vanK) required for drug resistance. Mol. Microbiol. 52, 1107–1121. doi: 10.1111/j.1365-2958.2004.04032.x
Howden, B. P., Davies, J. K., Johnson, P. D., Stinear, T. P., and Grayson, M. L. (2010). Reduced vancomycin susceptibility in Staphylococcus aureus, including vancomycin-intermediate and heterogeneous vancomycin-intermediate strains: resistance mechanisms, laboratory detection, and clinical implications. Clin. Microbiol. Rev. 23, 99–139. doi: 10.1128/cmr.00042-09
Hutchings, M. I., Hong, H. J., and Buttner, M. J. (2006). The vancomycin resistance VanRS two-component signal transduction system of Streptomyces coelicolor. Mol. Microbiol. 59, 923–935. doi: 10.1111/j.1365-2958.2005.04953.x
Hutter, B., Schaab, C., Albrecht, S., Borgmann, M., Brunner, N. A., Freiberg, C., et al. (2004). Prediction of mechanisms of action of antibacterial compounds by gene expression profiling. Antimicrob. Agents Chemother. 48, 2838–2844. doi: 10.1128/aac.48.8.2838-2844.2004
Jeong, Y., Kim, J. N., Kim, M. W., Bucca, G., Cho, S., Yoon, Y. J., et al. (2016). The dynamic transcriptional and translational landscape of the model antibiotic producer Streptomyces coelicolor A3(2). Nat. Commun. 7:11605.
Jones, M. B., Nierman, W. C., Shan, Y., Frank, B. C., Spoering, A., Ling, L., et al. (2017). Reducing the bottleneck in discovery of novel antibiotics. Microb. Ecol. 73, 658–667. doi: 10.1007/s00248-016-0889-3
Kwun, M. J., and Hong, H. J. (2014). The activity of glycopeptide antibiotics against resistant bacteria correlates with their ability to induce the resistance system. Antimicrob. Agents Chemother. 58, 6306–6310. doi: 10.1128/aac.03668-14
Lee, A. Y., St Onge, R. P., Proctor, M. J. I, Wallace, M., Nile, A. H., Spagnuolo, P. A., et al. (2014). Mapping the cellular response to small molecules using chemogenomic fitness signatures. Science 344, 208–211.
Marcone, G. L., Binda, E., Berini, F., and Marinelli, F. (2018). Old and new glycopeptide antibiotics: from product to gene and back in the post-genomic era. Biotechnol. Adv. 36, 534–554. doi: 10.1016/j.biotechadv.2018.02.009
McCarthy, D. J., and Smyth, G. K. (2009). Testing significance relative to a fold-change threshold is a TREAT. Bioinformatics 25, 765–771. doi: 10.1093/bioinformatics/btp053
McCormick, M. H., McGuire, J. M., Pittenger, G. E., Pittenger, R. C., and Stark, W. M. (1955). Vancomycin, a new antibiotic. I. Chemical and biologic properties. Antibiot. Annu. 3, 606–611.
Mishra, N. M. I, Stolarzewicz, D., Cannaerts, J., Schuermans, R., Lavigne, Y., Looz, B., et al. (2018). Iterative chemical engineering of vancomycin leads to novel vancomycin analogs with a high in vitro therapeutic index. Front. Microbiol. 9:1175. doi: 10.3389/fmicb.2018.01175
Morgan, M., and Shepherd, L. (2020). AnnotationHub: Client to Access AnnotationHub Resources. R package Version 2.20.0.
Nguyen, T. G., Vargas-Blanco, D. A., Roberts, L. A., and Shell, S. S. (2020). The impact of leadered and leaderless gene structures on translation efficiency, transcript stability, and predicted transcription rates in Mycobacterium smegmatis. J. Bacteriol. 202:e00746-19.
Novotna, J., Vohradsky, J., Berndt, P., Gramajo, H., Langen, H., Li, X. M., et al. (2003). Proteomic studies of diauxic lag in the differentiating prokaryote Streptomyces coelicolor reveal a regulatory network of stress-induced proteins and central metabolic enzymes. Mol. Microbiol. 48, 1289–1303. doi: 10.1046/j.1365-2958.2003.03529.x
Okano, A., Isley, N. A., and Boger, D. L. (2017). Total syntheses of vancomycin-related glycopeptide antibiotics and key analogues. Chem. Rev. 117, 11952–11993. doi: 10.1021/acs.chemrev.6b00820
Okano, A., Nakayama, A., Schammel, A. W., and Boger, D. L. (2014). Total synthesis of [Psi[C(horizontal lineNH)NH]Tpg(4)]vancomycin and its (4-chlorobiphenyl)methyl derivative: impact of peripheral modifications on vancomycin analogues redesigned for dual D-Ala-D-Ala and D-Ala-D-Lac binding. J. Am. Chem. Soc. 136, 13522–13525. doi: 10.1021/ja507009a
O’Rourke, A., Beyhan, S., Choi, Y., Morales, P., Chan, A. P., Espinoza, J. L., et al. (2020). Mechanism-of-action classification of antibiotics by global transcriptome profiling. Antimicrob. Agents Chemother. 64:e01207-19.
Pootoolal, J., Neu, J., and Wright, G. D. (2002). Glycopeptide antibiotic resistance. Annu. Rev. Pharmacol. Toxicol. 42, 381–408.
R Core Team, (2020). R: A Language and Environment For Statistical Computing. Vienna: R Foundation for Statistical Computing.
Ritchie, M. E., Phipson, B., Wu, D., Hu, Y., Law, C. W., Shi, W., et al. (2015). limma powers differential expression analyses for RNA-sequencing and microarray studies. Nucleic Acids Res. 43:e47. doi: 10.1093/nar/gkv007
Santos-Beneit, F. (2018). Genome sequencing analysis of Streptomyces coelicolor mutants that overcome the phosphate-depending vancomycin lethal effect. BMC Genomics 19:457. doi: 10.1186/s12864-018-4838-z
Santos-Beneit, F., and Martin, J. F. (2013). Vancomycin resistance in Streptomyces coelicolor is phosphate-dependent but is not mediated by the PhoP regulator. J. Glob. Antimicrob. Resist. 1, 109–113. doi: 10.1016/j.jgar.2013.03.003
Smidova, K., Zikova, A., Pospisil, J., Schwarz, M., Bobek, J., and Vohradsky, J. (2019). DNA mapping and kinetic modeling of the HrdB regulon in Streptomyces coelicolor. Nucleic Acids Res. 47, 621–633. doi: 10.1093/nar/gky1018
Tailhades, J., Zhao, Y., Ho, Y. T. C., Greule, A. I, Schoppet, A. M., Kulkarni, K., et al. (2020). A chemoenzymatic approach to the synthesis of glycopeptide antibiotic analogues. Angew. Chem. Int. Ed. Engl. 59, 10899–10903. doi: 10.1002/anie.202003726
Tran, N. T., Huang, X., Hong, H. J., Bush, M. J., Chandra, G., Pinto, D., et al. (2019). Defining the regulon of genes controlled by sigma(E), a key regulator of the cell envelope stress response in Streptomyces coelicolor. Mol. Microbiol. 112, 461–481. doi: 10.1111/mmi.14250
Virolle, M. J. (2020). A challenging view: antibiotics play a role in the regulstion of the energetic metabolism of the producing bacteria. Antibiotics (Basel) 9:83. doi: 10.3390/antibiotics9020083
Walsh, C. T., Fisher, S. L. I, Park, S., Prahalad, M., and Wu, Z. (1996). Bacterial resistance to vancomycin: five genes and one missing hydrogen bond tell the story. Chem. Biol. 3, 21–28. doi: 10.1016/s1074-5521(96)90079-4
Xu, M., Wang, W., Waglechner, N., Culp, E. J., Guitor, A. K., and Wright, G. D. (2020). GPAHex-A synthetic biology platform for Type IV-V glycopeptide antibiotic production and discovery. Nat. Commun. 11:5232.
Yarlagadda, V., Sarkar, P., Manjunath, G. B., and Haldar, J. (2015). Lipophilic vancomycin aglycon dimer with high activity against vancomycin-resistant bacteria. Bioorg. Med. Chem. Lett. 25, 5477–5480. doi: 10.1016/j.bmcl.2015.10.083
Yu, G., Wang, L. G., Han, Y., and He, Q. Y. (2012). clusterProfiler: an R package for comparing biological themes among gene clusters. OMICS 16, 284–287. doi: 10.1089/omi.2011.0118
Zarkan, A., Macklyne, H. R., Chirgadze, D. Y., Bond, A. D., Hesketh, A. R., and Hong, H. J. (2017). Zn(II) mediates vancomycin polymerization and potentiates its antibiotic activity against resistant bacteria. Sci. Rep. 7:4893.
Zarkan, A., Macklyne, H. R., Truman, A. W., Hesketh, A. R., and Hong, H. J. (2016). The frontline antibiotic vancomycin induces a zinc starvation response in bacteria by binding to Zn(II). Sci. Rep. 6:19602.
Zhanel, G. G., Schweizer, F., and Karlowsky, J. A. (2012). Oritavancin: mechanism of action. Clin. Infect. Dis. 54 (Suppl. 3), S214–S219.
Keywords: glycopeptide, antibiotic, resistance, vancomycin, dalbavancin, chemotranscriptomics, Streptomyces
Citation: Hesketh A, Bucca G, Smith CP and Hong H-J (2021) Chemotranscriptomic Profiling Defines Drug-Specific Signatures of the Glycopeptide Antibiotics Dalbavancin, Vancomycin and Chlorobiphenyl-Vancomycin in a VanB-Type-Resistant Streptomycete. Front. Microbiol. 12:641756. doi: 10.3389/fmicb.2021.641756
Received: 14 December 2020; Accepted: 05 February 2021;
Published: 25 February 2021.
Edited by:
Sergio Sanchez, Universidad Nacional Autónoma de México (UNAM), MexicoCopyright © 2021 Hesketh, Bucca, Smith and Hong. This is an open-access article distributed under the terms of the Creative Commons Attribution License (CC BY). The use, distribution or reproduction in other forums is permitted, provided the original author(s) and the copyright owner(s) are credited and that the original publication in this journal is cited, in accordance with accepted academic practice. No use, distribution or reproduction is permitted which does not comply with these terms.
*Correspondence: Colin P. Smith, Z2Vub21pY3NAY3BzbWl0aC5uZXQ=; Hee-Jeon Hong, aGVlLWplb24uaG9uZ0Bicm9va2VzLmFjLnVr
Disclaimer: All claims expressed in this article are solely those of the authors and do not necessarily represent those of their affiliated organizations, or those of the publisher, the editors and the reviewers. Any product that may be evaluated in this article or claim that may be made by its manufacturer is not guaranteed or endorsed by the publisher.
Research integrity at Frontiers
Learn more about the work of our research integrity team to safeguard the quality of each article we publish.