- 1Division of Biological and Environmental Science and Engineering, Red Sea Research Center, King Abdullah University of Science and Technology, Thuwal, Saudi Arabia
- 2Department of Biology, University of Konstanz, Konstanz, Germany
- 3Laboratory for Biological Geochemistry, School of Architecture, Civil and Environmental Engineering, École Polytechnique Fédérale de Lausanne, Lausanne, Switzerland
- 4Center for Advanced Surface Analysis, Institute of Earth Sciences, University of Lausanne, Lausanne, Switzerland
Aiptasia is an emerging model organism to study cnidarian symbioses due to its taxonomic relatedness to other anthozoans such as stony corals and similarities of its microalgal and bacterial partners, complementing the existing Hydra (Hydrozoa) and Nematostella (Anthozoa) model systems. Despite the availability of studies characterizing the microbiomes of several natural Aiptasia populations and laboratory strains, knowledge on basic information, such as surface topography, bacterial carrying capacity, or the prospect of microbiome manipulation is lacking. Here we address these knowledge gaps. Our results show that the surface topographies of the model hydrozoan Hydra and anthozoans differ substantially, whereas the ultrastructural surface architecture of Aiptasia and stony corals is highly similar. Further, we determined a bacterial carrying capacity of ∼104 and ∼105 bacteria (i.e., colony forming units, CFUs) per polyp for aposymbiotic and symbiotic Aiptasia anemones, respectively, suggesting that the symbiotic status changes bacterial association/density. Microbiome transplants from Acropora humilis and Porites sp. to gnotobiotic Aiptasia showed that only a few foreign bacterial taxa were effective colonizers. Our results shed light on the putative difficulties of transplanting microbiomes between cnidarians in a manner that consistently changes microbial host association at large. At the same time, our study provides an avenue to identify bacterial taxa that exhibit broad ability to colonize different hosts as a starting point for cross-species microbiome manipulation. Our work is relevant in the context of microbial therapy (probiotics) and microbiome manipulation in corals and answers to the need of having cnidarian model systems to test the function of bacteria and their effect on holobiont biology. Taken together, we provide important foundation data to extend Aiptasia as a coral model for bacterial functional studies.
Introduction
Corals constitute the foundation species of reef ecosystems that provide a habitat for about a third of all marine life (Fisher et al., 2015), but anthropogenic-driven climate change is now one of the main drivers of coral reef loss (Hughes et al., 2017b, 2018a,b): about half of the Great Barrier Reef corals were lost over the last 30 years (Dietzel et al., 2020) and a 70–99% coral reef decline is projected even under a 1.5–2°C warming, one of the more benign climate change scenarios (Allen et al., 2018). Therefore, it is important to find solutions that can improve coral resilience and mitigate the negative effects of ongoing ocean warming, ocean acidification, and ocean deoxygenation (Allemand and Osborn, 2019; Hughes et al., 2020). Importantly, corals are cnidarian holobionts that consist of the coral animal host, intracellular microalgal symbionts (Symbiodiniaceae), and associated bacteria among many other organisms that all contribute to the stress tolerance and resilience of this metaorganism (Rohwer et al., 2002; Rosenberg et al., 2007; Bang et al., 2018; LaJeunesse et al., 2018; Pogoreutz et al., 2020; Voolstra and Ziegler, 2020). Besides the broad importance of Symbiodiniaceae, which reside within the coral cells and cover almost the entire energy needs of the coral host through provision of photosynthates (Muscatine, 1990; Trench, 1993), bacteria presumably play important roles in metabolism, immunity, and environmental adaptation of the coral host (Ziegler et al., 2017; Ziegler et al., 2019; Robbins et al., 2019; Voolstra and Ziegler, 2020). However, functional studies that detail the specific contributions of specific microbial taxa are still rare, partially due to methodological limitations (Cooke et al., 2019; Robbins et al., 2019). One suggested avenue to elucidate host-bacteria interactions in cnidarians is the use of model organisms, in particular using gnotobiotic (i.e., bearing few remaining known bacteria) or axenic (i.e., bacteria-/germ-free) host systems that allow controlled exposure or provision of cultured bacterial isolates (Jaspers et al., 2019).
Among Cnidarians, the hydrozoan Hydra is one of the few models where a germ-free (axenic) closed life cycle is available, which allows the detailed study of associated bacteria and the functions they contribute to the metaorganism, demonstrating the power of such systems to elucidate host-bacterial interactions (Fraune and Bosch, 2007; Fraune et al., 2009; Fraune et al., 2014; Franzenburg et al., 2013b; Augustin et al., 2017; Wein et al., 2018). Similarly, the anthozoan Nematostella vectensis is becoming established as a cnidarian model to study host-bacterial interactions (Har et al., 2015; Domin et al., 2018). However, both systems lack the ability to engage in symbioses with microalgal symbionts of the family Symbiodiniaceae (LaJeunesse et al., 2018). Therefore, they may not comprise an ideal model for corals, since association with Symbiodiniaceae affects bacterial assemblage (Ainsworth et al., 2015; Röthig et al., 2016a; Lawson et al., 2018; Maire et al., 2021). To this end, the sea anemone Aiptasia is gaining increasing traction as a coral model due to harboring the same or similar Symbiodiniaceae as scleractinian corals, its simplicity of culturing and clonal propagation, and the fact that Aiptasia anemones can be kept indefinitely in symbiotic and aposymbiotic states (i.e., with and without their microalgal partners) (Weis et al., 2008; Voolstra, 2013), allowing to study the mechanistic underpinnings of the cnidarian-dinoflagellate symbiosis in detail (Baumgarten et al., 2015; Biquand et al., 2017; Cziesielski et al., 2018; Rädecker et al., 2018; Gegner et al., 2019; Simona et al., 2019). Of note, the name Aiptasia refers to the colloquial model system name, given that different researchers across the world use different strains, and likely species (Weis et al., 2008; Baumgarten et al., 2015; Oakley et al., 2015; Biquand et al., 2017; Dungan et al., 2020). The current proposed species name is Exaiptasia diaphana (Dungan et al., 2020), previously Exaiptasia pallida (Grajales and Rodríguez, 2014). Following the notion of Aiptasia as a model to study the coral-algal symbiosis (Baumgarten et al., 2015), its suitability as a model to study coral-bacterial interactions was suggested (Röthig et al., 2016a). Studies that describe bacterial association of several wild-type and lab-cultured strains could show that (i) microbial assemblages of lab-cultured Aiptasia are comparable, (ii) bacterial associations are somewhat “plastic” pending environmental differences and association with or without algal symbionts, and (iii) an overall similarity in the taxonomic composition of microbiomes of corals and Aiptasia (Röthig et al., 2016a; Brown et al., 2017; Herrera et al., 2017; Dungan et al., 2020). However, a detailed examination of the surface ectoderm topography, bacterial carrying capacity, and the prospect of microbiome manipulation (e.g., in the form of microbiome transplants) to highlight similarities to corals and demonstrate the efficacy of the Aiptasia system as a tool to study bacterial interactions was missing.
In the current work, our aim was to address these knowledge gaps and provide a foundation for Aiptasia to be used as a model for the study of coral-bacterial interactions. To do this, we characterized tissue surface topographies of Aiptasia and the Hydra and Nematostella cnidarian model systems and subsequently compared them to three scleractinian corals, in the context of the surface ectoderm as a bacterial habitat. In addition, we determined the bacterial carrying capacity in symbiotic and aposymbiotic Aiptasia anemones as a frame of comparison to reef-building corals and to provide a scale of reference for sequencing-based bacterial community studies. Last, using bacteria-depleted sea anemones, we conducted microbiome transplant experiments using coral microbiomes to assess the suitability of this method as a tool to study function of coral-associated bacteria and as a means to assess the ability of changing microbial host association to aid environmental adaptation of coral holobionts.
Materials and Methods
Animal Rearing
Aposymbiotic and symbiotic Aiptasia of the clonal strain CC7 were generated and reared as described previously (Baumgarten et al., 2015) with some modifications. Aposymbiotic animals were generated through repeated 4 h cold−shocks at 4°C and treatment with 50 μM of the photosynthetic inhibitor diuron (Sigma−Aldrich, United States). Aposymbiotic animals were maintained in the dark for >3 years and are routinely inspected by fluorescence microscopy (Leica DMI3000 B) to confirm the absence of Symbiodiniaceae. Symbiotic anemones were generated by infecting aposymbiotic animals with strain SSB01 (Breviolum minutum, former Clade B) (Xiang et al., 2013). Of note, the algal symbiont strain SSB01 is not the native symbiont of Aiptasia CC7 but has been previously used as a stable and characterized Aiptasia host-algal symbiont combination (Röthig et al., 2016a; Wolfowicz et al., 2016; Simona et al., 2019). To obtain symbiotic CC7-SSB01 Aiptasia, individual anemones were exposed to 105 Symbiodiniaceae cells/ml for 24 h, fed with Artemia nauplii after 48 h, and seawater was exchanged thereafter. All Aiptasia anemones were kept in autoclaved natural seawater and reared in 1 L tanks at a 12 h:12 h light:dark cycle at 20–40 μmol photons m2 s1 of photosynthetically active radiation at 25°C in an I-36LLVL incubator (Percival Scientific Inc., United States). Nematostella vectensis polyps were reared in half liter tanks in half strength artificial seawater at room temperature. Polyps of Hydra magnipapillata strain 105 were reared in half liter tanks in commercial spring water at room temperature. Nematostella and Hydra animals were fed Artemia nauplii twice a week.
Ectoderm Surface Analysis Using Electron Microscopy
We compared ectoderm surface topography of different cnidarian classes, namely Anthozoa (corals, Aiptasia, Nematostella vectensis) with Hydrozoa (Hydra magnipapillata), using Scanning Electron Microscopy (SEM). Coral colony fragments of Stylophora pistillata, Acropora humilis, and Porites sp. were collected from the central Red Sea at 6 m depth at the Al Fahal forereef (22°15.100′N, 38°57.386′E) by SCUBA and maintained in an open water aquaria system until use (CMOR, KAUST), whereas Aiptasia, N. vectensis, and H. magnipapillata animals were available from cultured lab strains. Three fragments (in the case of corals) or three polyps (in the case of Aiptasia, Nematostella, Hydra) were transferred to 24-well plates for processing. Coral fragments and model system cnidarians were left to expand in ∼2% magnesium chloride in artificial seawater (ASW) or fresh water for Hydra, or half strength ASW for Nematostella for 15 min, respectively. After that, specimens were fixed in ∼2.5% glutaraldehyde in 0.1 M cacodylate buffer at 4°C overnight. Samples were then washed in 0.1 M cacodylate buffer (pH 7.2–7.4) thrice for 15 min each and post fixed in 1% osmium tetroxide solution in 0.1 M cacodylate buffer for 1 h in the dark. Samples were further washed thrice in ddH2O for 15 min each and proceeded for dehydration in an EtOH gradient: 30, 50, 70, 90, and 100%, 15 min each step, with two final incubations in 100% EtOH, also for 15 min. The drying process was initiated by transferring the samples to a 1:2 solution of hexamethyldisilazane (HMDS):100% EtOH (v/v) for 20 min, then to a fresh solution of 2:1 HMDS:100% EtOH for 20 min, 100% HMDS for 20 min, and a final incubation in 100% HMDS. Samples were left to dry loosely covered in the chemical hood for the HMDS to slowly evaporate overnight. Each fragment was then mounted on an SEM specimen mount head pin and sputter coated with 4 nm of platinum/palladium or Iridium. All samples were imaged using a Teneo Volume Scope electron microscope (FEI, United States) operating at 1–3 kV.
Complementary to the SEM analyses, we imaged Aiptasia specimens by Transmission Electron Microscopy (TEM). Briefly, Aiptasia polyps were fixed in 2.5% glutaraldehyde in 0.1 M cacodylate buffer (pH 7.4) for 12 h at 4°C and post fixed with 1% osmium tetroxide in 0.1 M cacodylate buffer for 1 h in the dark at 4°C. After three washes in ddH2O, the sample blocks were dehydrated through EtOH and acetone, infiltrated with a mixture of epoxy resin (Electron Microscopy Sciences, United States) and acetone, followed by a final embedding in pure resin. A Leica EM UC6 Ultramicrotome (Leica Microsystems, Germany) was used to cut 150 nm thin sections from the resin block. Finally, thin sections were collected on a 200 mesh copper grid, stained with 1% uranyl acetate (Electron Microscopy Sciences, United States) and Reynold’s lead citrate (Electron Microscopy Sciences, United States). Images were acquired using a Titan CT transmission electron microscope (Thermo Fisher Scientific, United States) operating at 300 kV.
To investigate the presence of bacteria inside the mucus layer using TEM, we modified the standard resin embedding protocol by introducing an agarose embedding step with subsequent cryosectioning of the specimens. Aiptasia anemones were prepared as follows: Individual polyps were chemically fixed in phosphate buffer (0.1 M pH 7.4) with 9% sucrose, containing 4% formaldehyde and 2.5% glutaraldehyde. Fixation was done at room temperature for 2 h after which samples were embedded in 4% aqueous agarose to ease the manipulation. Then, polyps were cut into ∼4 mm3 pieces and post stained in 2% osmium aqueous solution for 1 h in the dark. After rinsing in water, samples were dehydrated in a series of ethanol concentrations, ranging from 10 to 100%, then infiltrated with EPON resin (Electron Microscopy Sciences, United States), before polymerization at 60°C for 48 h. Thin sections of 80 nm were cut and mounted onto a Formvar film-coated, carbon-stabilized 100 mesh copper finder grid (Electron Microscopy Sciences, United States). Sections were post stained with UranyLess (Electron Microscopy Sciences, United States) and lead citrate (Electron Microscopy Sciences, United States) before being imaged with a Tecnai-12 transmission electron microscope (Thermo Fisher Scientific, United States) operating at 100 kV with a FEI eagle camera (Thermo Fisher Scientific, United States) using TIA software (Thermo Fisher Scientific, United States). Contrast, brightness, and sharpness of acquired images were adjusted using Adobe Photoshop.
Generation of Gnotobiotic Aiptasia
Bacteria-depleted Aiptasia polyps were generated using a previously developed protocol consisting of a depletion priming step, followed by an antibiotic treatment, and subsequent recovery from the antibiotic cocktail (Costa et al., 2019). For the depletion priming step, polyps were transferred to 500 ml plastic tanks, reared in 0.22 μm filtered ASW (same light and temperature regime as described above) and fed with sterile decapsulated Artemia nauplii for 4 weeks. For the antibiotic treatment, anemones were transferred to petri dishes and washed repeatedly with ASW individually and incubated in antibiotic solution (50 μg/ml of Carbenicillin, Chloramphenicol, Rifampicin, and Nalidixic acid in ASW) for 15 min. Polyps were then transferred to 24-well tissue culture plates (Corning Costar, United States), one polyp per well, under sterile conditions, and incubated with antibiotic solution for 7 days, with daily media exchange, at a 12 h:12 h light/dark cycle in an incubator (20–40 μmol photons m–2 s–1 of photosynthetically active radiation) at 25°C. After 7 days, anemones were given 24 h for recovery in ASW before microbiome inoculation (see below). Effective bacterial depletion using this protocol was validated using culture-dependent and -independent techniques (Costa et al., 2019): bacterial depletion of treated Aiptasia polyps was confirmed by absence of colony forming units (CFUs) after plating anemone lysates on Marine Agar and subsequent incubation for at least 5 days. In addition, DNA extracted using the DNeasy Blood & Tissue Kit (Qiagen, Germany) from treated Aiptasia anemone lysates were used for PCR amplification of the 16S rRNA gene (95°C for 15 min, followed by 30 cycles of 30 s at 95°C, 90 s at 55°C, and 90 s at 72°C, followed by a final extension for 10 min at 72°C using the 16S universal primer pair 27F-1492R) and subsequent confirmation of absence of a PCR product by means of electrophoresis on an agarose gel.
Microbial Carrying Capacity
We determined microbial carrying capacity of apo- and symbiotic control Aiptasia polyps (i.e., untreated) as well as of anemones after antibiotic treatment and microbiome transplants. To do this, single polyps from all experimental conditions were placed in 1.5 ml tubes under sterile conditions and 300 μl of ASW were added before the polyps were lysed using a motorized pestle and mortar. Animal lysates and ASW (negative control) were diluted 10-, 100-, and 1000-fold, and 50 μl were plated on Marine Agar (Difco Marine Agar 2216, BD Biosciences, United States) and incubated at 25°C for 24–48 h or 5 days in the case of antibiotic treated anemones, with subsequent counting of bacterial colonies. For statistical analysis, colony counts were log-transformed, normality was tested using the Shapiro–Wilk test, and a one-way ANOVA was conducted. In case of statistical significance, a Dunnett post hoc test was conducted. An unpaired t-test was used to assess for significant differences between aposymbiotic and symbiotic control conditions. In order to account for putative polyp size differences, CFU counts per polyp were normalized to the host total protein for each polyp, determined using a Micro BCA protein assay kit (Pierce, United States), and the same statistical analysis as above was conducted.
Microbiome Inocula
Fragments of Acropora humilis and Porites sp. were collected from the nearshore reef Tahala (22°15.7812′N, 39°3.099′E) (central Red Sea, Saudi Arabia) and processed on the same day. Fragments from three colonies per coral species were collected and combined prior to inoculation (see below). Each coral fragment was placed in a sterile Ziploc bag with 10 ml of ASW, and coral tissue was air blasted off the skeleton using a sterile 1 ml barrier tip inserted to a rubber hose connected to a bench air-pressure outlet. The slurry obtained from fragments from a given coral species were combined and transferred to a 50 ml polypropylene tube and the volume was adjusted to 50 ml with ASW to reduce viscosity. The slurry was homogenized for 30 s using an Ultra Turrax T18 homogenizer (IKA, Germany) and split in 25 ml preparations per tube. Control inocula were prepared using between 10 and 15 apo- and symbiotic Aiptasia polyps, respectively, in 30 ml of ASW, homogenized as described above, and homogenized a second time with a MicroDisTec homogenizer 125 (Thermo Fisher Scientific, United States) to ensure complete maceration. The final volume of the lysate was adjusted to 50 ml with ASW and split in 25 ml preparations.
Microbiome inocula were further processed in a biosafety cabinet, using sterile work practices. Lysates were centrifuged using a swing-bucket rotor at 500 g for 5 min to pellet zooxanthellae. The supernatant was collected and an aliquot was taken for visual inspection on an inverted epifluorescence microscope. All lysates were centrifuged once, except for the Porites sp. inoculum, which had one extra centrifugation step to completely remove visible traces of the zooxanthellae. The supernatants were pooled for each inoculum type and centrifuged at 3220 g for 30 min to pellet bacteria. The resulting pellet was resuspended in 25 ml of ASW and centrifuged twice. Pellets were resuspended in 15 ml of ASW and a 1 ml aliquot was set aside for quantification of bacteria.
Bacteria were quantified using BacLight Red Bacterial stain (Thermo Fisher Scientific, United States). Aliquots from each inoculum were stained using 1 μM of dye for 10 min and counted using a BD FACSCanto II flow cytometer (BD Biosciences, United States). Gates for bacterial counts were first defined using 1 μm and 2 μm reference beads (Thermo Fisher Scientific, United States) in forward scatter (FSC) vs. side scatter (SSC) and then by using Aiptasia bacterial cultures stained with BacLight Red and acquired in the PerCP-PI channel. Bacterial numbers were calculated after gravimetric calibration of the flow rates and using the positive events acquired using the defined gating strategy. Based on determined counts, inocula were diluted to 5 × 105 bacterial cells/ml and 1 ml was used per polyp. In parallel to the flow cytometry-based bacterial counts, an aliquot of all final inocula was plated on Marine Agar (Difco Marine Agar 2216, BD Biosciences, United States) for CFU counts. Bacterial densities of inocula are provided in Supplementary Table 1.
Microbiome Transplants
For the microbiome transplants, we assessed 30 apo- and symbiotic anemones each across five experimental conditions using six biological replicates (60 anemones in total). Experimental conditions were as follows: (1) untreated control anemones from rearing tanks (APO and SYM), (2) gnotobiotic anemones after 1 day of recovery from antibiotic treatment (APO_AB and SYM_AB), (3) Acropora microbiome inoculum (APO+ACRinoc and SYM+ACRinoc), (4) Porites microbiome inoculum (APO+PORinoc and SYM+PORinoc), (5) Aiptasia microbiome inoculum (APO+APOinoc and SYM+SYMinoc). Anemones were kept in 24-well plates, 1 polyp per well, and gnotobiotic anemones were inoculated by adding 1 ml of the respective microbiome inoculum to the well (final volume of 1 ml, 5 × 105 bacterial cells/ml) and subsequent incubation for 3 days. After that, anemones were washed twice with ASW and kept in ASW for 4 additional days before being collected (7 days after microbiome transplantation).
RNA Isolation, cDNA Synthesis, and 16S rRNA Amplicon Sequencing
RNA-based 16S rRNA amplicon sequencing was conducted on five biological replicates from each experimental condition and symbiotic state of the microbiome transplant experiment (see above), in addition to no template DNA extraction and no template PCR negative controls. For RNA isolation, the Qiagen AllPrep DNA/RNA kit (Qiagen, Germany) was used. Briefly, 600 μl of RLT Plus buffer were added to 150 μl of fresh lysate (or artificial seawater for the negative control) followed by snap freezing of the tubes in liquid nitrogen and storage at −80°C until extraction, following the manufacturer’s instructions. RNA quantity and integrity were determined using Qubit (Thermo Fisher Scientific, United States) and BioAnalyzer (Agilent Technologies, United States), respectively. Total RNA was DNase-treated prior to reverse-transcription using the SuperScript First-Strand Synthesis System (Invitrogen, United States), according to the manufacturer’s instructions. For amplification of 16S rRNA amplicons from cDNA, the primers 784F and 1061R (Andersson et al., 2008; Bayer et al., 2013) with MiSeq 16S adapter sequences were used (forward: 5′-TCGTCGGCAGCGTCAGATGTGTATAAGAGACAGAGGA TTAGATACCCTGGTA-3′; reverse: 5′-GTCTCGTGGGCTCGG A GA TGT GTA TA AG A G A C A G CR R C A C GA G CTGACGAC -3′; Illumina overhang adaptor sequences are underlined). PCR reactions were performed in triplicate using the Qiagen Multiplex PCR kit (Qiagen, Germany) with 2 μl of cDNA and a primer concentration of 0.5 μM in a reaction volume of 20 μL. PCRs were performed as follows: 1 cycle at 95°C for 15 min, 27 cycles each at 95°C for 30 s, 55°C for 90 s, and 72°C for 30 s, followed by a final extension step at 72°C for 10 min. Triplicate PCRs for each sample were pooled and cleaned with Illustra ExoProStar (GE Healthcare, United States). Samples were subsequently indexed (eight PCR cycles) using Nextera XT barcode sequencing adapters (Illumina, United States). Indexed PCR products were normalized using Invitrogen SequalPrep normalization plates (Thermo Fisher Scientific, United States) and pooled in equimolar ratios. Pooled samples were checked for the presence of primer dimers on a BioAnalyzer (Agilent Technologies, United States) before sequencing. The library was sequenced at the KAUST Bioscience Core Lab using 2 × 300 bp at 6 pM with 20% phiX on the Illumina MiSeq (version 3 chemistry) according to the manufacturer’s specifications.
16S rRNA Amplicon Analysis
Sequence reads were demultiplexed and adapters and barcodes were removed. Resulting sequences were then processed using mothur v.1.39.5 (Schloss et al., 2009). Briefly, paired-end sequences were assembled using the “make.contigs” command, subsequently trimmed to exclude sequences <200 bp, and rare sequences (appearing once across the entire sequencing dataset) were removed. The remaining sequences were then aligned to the SILVA database (version 132) using “align.seqs,” then pre-clustered allowing a 2 nt difference, and finally chimeric sequences were removed using VSEARCH (Rognes et al., 2016). Taxonomical classification was done using the Greengenes (release gg_13_5_99, May 2013) and SILVA (release 138, December 2016) databases. Eukaryotic, archaeal, mitochondrial, and chloroplast sequences were removed prior to OTU clustering using a 97% similarity cutoff. Putative contaminants were determined based on their abundance in negative controls and removed from all samples. An OTU was considered a contaminant if: [Σ relative abundance OTUi in negative controls]/[Σ relative abundance OTUi in all samples] > 0.1. Beta diversity was examined via principal coordinate analysis (PCoA) of Bray-Curtis dissimilarity distances of log10(x + 1) OTU abundances (Supplementary Data 1) using the phyloseq package (McMurdie and Holmes, 2013). A permutational multivariate analysis of variance (PERMANOVA) was carried out using the “adonis” function on dissimilarity Bray-Curtis distances of relative OTU abundances to test for differences between conditions and across symbiotic states. Pairwise PERMANOVA tests were conducted using an R wrapper function for multilevel pairwise comparisons (Martinez Arbizu, 2019). To determine overlap of bacterial taxa across microbiome transplants, we determined OTUs that were present across all samples for a given experimental treatment (i.e., APO, APO+APOinoc, ACR+ACRinoc, APO+PORinoc, SYM, SYM+SYMinoc, SYM+ACRinoc, and SYM+PORinoc) and overlapping taxa were represented using the package VennDiagram (Chen and Boutros, 2011).
Results
Distinct Ectoderm Surface Topographies Across Cnidarians
We compared surface topographies using scanning electron microscopy (SEM) of three model system cnidarians (Hydra, Nematostella, and Aiptasia) and three coral species (Stylophora pistillata, Acropora humilis, Porites sp.) as a first proxy to determine microbial association (Figure 1). The ectodermal epithelium of the hydrozoan Hydra was composed of a smooth surface with few cilia (i.e., 5–10 μm long hair-like plasma membrane projections, made of microtubules in a 9 + 2 ultrastructure arrangement) (Figure 1A). By comparison, Hydra tentacles showed increased ciliation (and a higher density of villi), coinciding with increased mucus and detritus retention, but also exhibited smooth(er) tissue patches scattered across the tentacles, where some bacteria were found to be attached (Figure 1B). The ectodermal epithelium surfaces of column and tentacle of N. vectensis and Aiptasia were highly similar (Figures 1C–F). We observed extensive villi (i.e., smaller, numerous plasma membrane projections, lacking microtubules) coverage in the body column of both polyps, with villi protruding from ectodermal cells ranging between 1 and 2 μm in length (Figures 1C,E) and bigger cilia ranging from 4 to 6 μm in the tentacle region (Figures 1D,F). In contrast to Hydra, we did not detect any bacteria on the column surface or tentacle regions. This suggests two things: (1) bacteria are rather rare on the surface ectoderm and may be largely constrained to the surface mucus layer in anthozoans, and (2) bacteria may be more abundant on the surface ectoderm in the hydrozoan Hydra than in anthozoans. This is corroborated by a complementary TEM analysis of the Aiptasia ectoderm, showing bacteria above the ciliary/villi band, inside the electron dense mucus layer (Figure 2 and Supplementary Figure 1). The preserved surface mucus layer was estimated around 5–15 μm, extending beyond the villi. Of further note, the surface ectoderms of apo- and symbiotic Aiptasia were indiscriminate (Supplementary Figure 2).
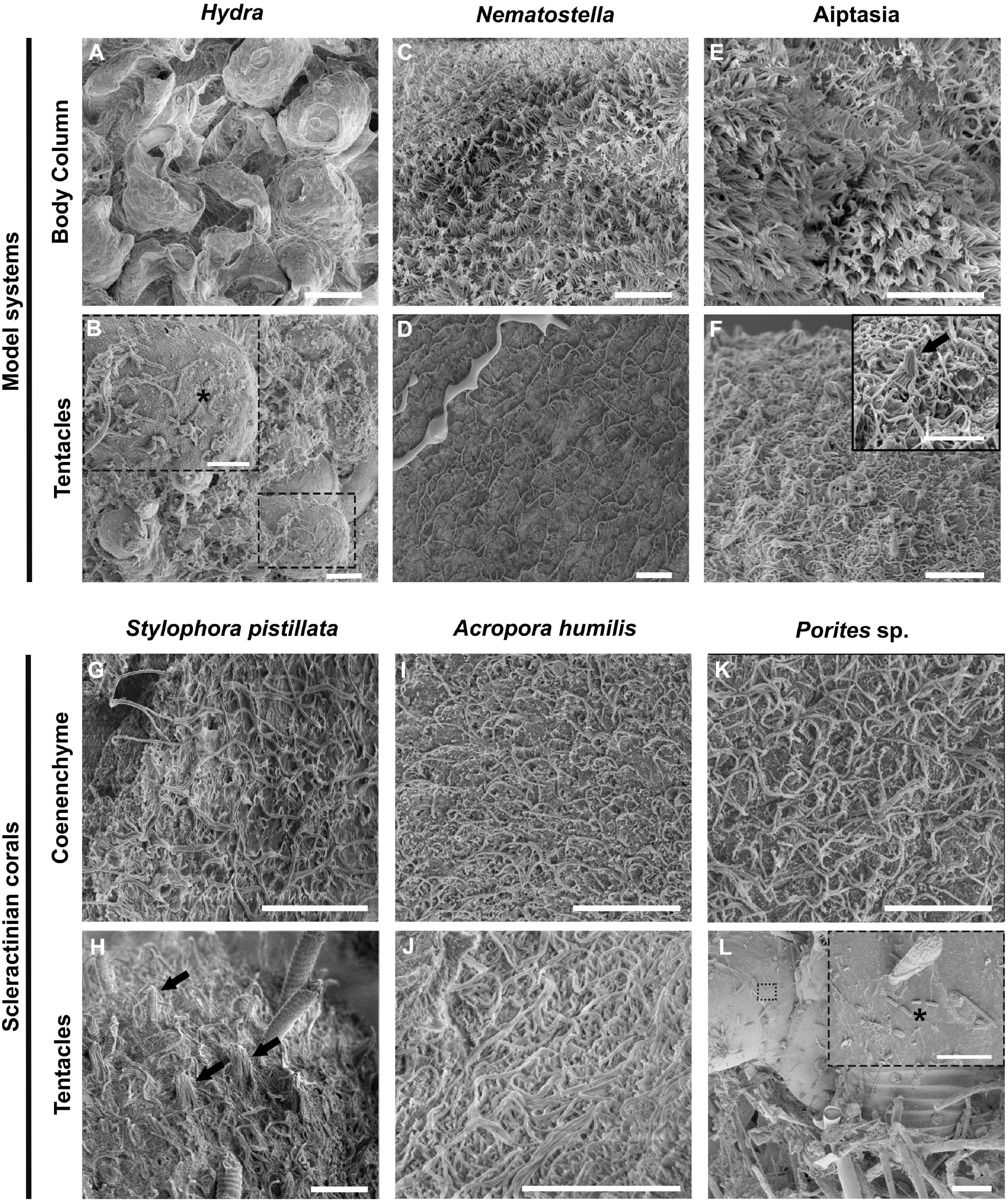
Figure 1. Ultrastructural comparison of the surface topography of Hydra and Anthozoans. Representative scanning electron microscopy (SEM) images of three model cnidarians (A–F) and three reef building corals (G–L) show differences between the hydrozoan Hydra and anthozoans, but an overall similar topography across anthozoans. (A,B) Hydra column and tentacle surface topography. (C,D) Nematostella column and tentacle surface topography. (E,F) Aposymbiotic Aiptasia column and tentacle surface topography. (G,H) Stylophora pistillata coenenchyme and tentacle. (I,J) Acropora humilis coenenchyme and tentacle. Noticeable are the discharged cnidocysts in the tentacle. (K,L) Porites sp. coenenchyme and fouled surface topography. Note the presence of microeukaryotes trapped in the fouled region, to which bacteria seem attached. Dashed line boxes denote regions where bacteria can be seen (black asterisks). Black arrows denote mechanoreceptor bundles. Scale bars: (A–K): 10 μm; (L): 100 μm; boxes in (B,F,L): 5 μm.
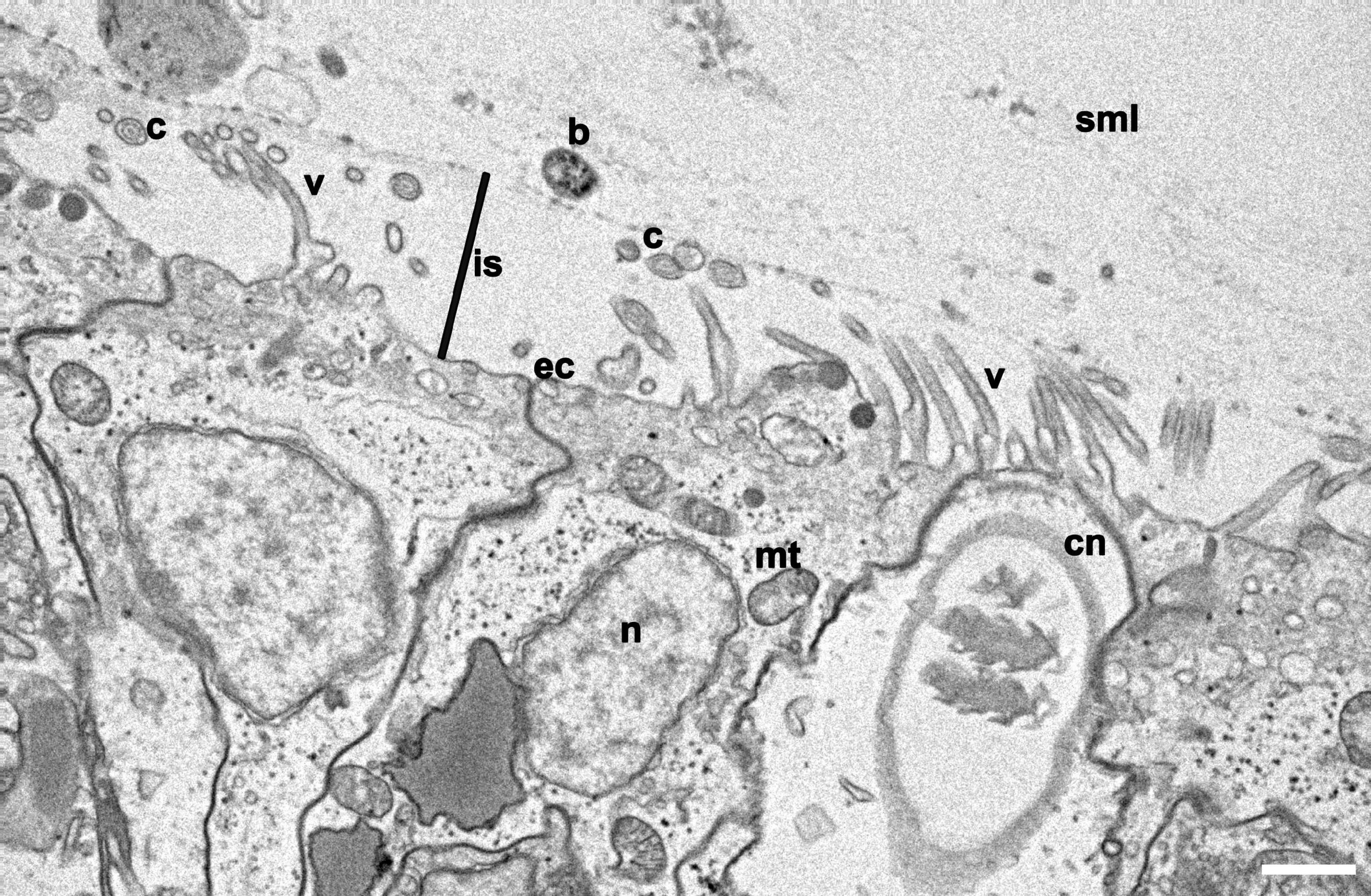
Figure 2. Cross section of the surface ectoderm of Aiptasia. Representative transmission electron microscopy (TEM) micrograph of Aiptasia shows the villi coverage on the epidermis and the interspace between villi and the surface mucus layer (SML), where a bacterium can be identified. b, bacterium; c, cilia; cn, cnidocyte; ec, ectoderm; is, Ectoderm-mucus interspace; mt, mitochondria; n, nucleus; sml, surface mucus layer; v, villus. Scale bar- 1 μm.
We also analyzed the ectodermal epithelium topography of the tentacles and coenenchyme (i.e., coral tissue between the polyps) of the three scleractinian corals Stylophora pistillata, Acropora humilis, and Porites sp. (Figures 1G–I). We observed extensive villi coverage with longer cilia ranging from 4 to 6 μm extending from the epidermis in all corals. Surface topographies were similar to N. vectensis and Aiptasia, but distinct from Hydra. In the case of Porites sp., a thick mucus sheet was visible on top of the coenenchyme, intercalated with fouled areas (Supplementary Figure 3). Further, the surface mucus layer of Porites sp. seemed distinct from S. pistillata and A. humilis, creating a more compact mucus sheet that persisted chemical fixation and several washing steps. Such sheet-like mucus appearances are described for Porites compressa, as well as the observation of the presence of fouled regions (Johnston and Rohwer, 2007). Contrary to the coral surface ectoderm that seemed devoid of bacteria, many bacteria were found attached to the smooth epithelial surfaces of other eukaryotes that aggregated in fouled regions of Porites sp. (Figure 1L), corroborating the notion that the ciliated surface of anthozoans plays a role in preventing bacterial adhesion.
Distinct Bacterial Carrying Capacity of Apo- and Symbiotic Aiptasia
To determine the putative carrying capacity of Aiptasia anemones, we obtained CFU counts from apo- and symbiotic control anemones that were subsequently compared to the different experimental conditions, i.e., antibiotic treated and microbiome inoculated animals (Figure 3 and Supplementary Tables 2, 3). The average carrying capacity of aposymbiotic Aiptasia anemones was determined as 4.25 × 104 CFUs/polyp (control animals). When normalized to protein biomass, we obtained 1.59 × 105 CFUs/mg host protein. The carrying capacity of symbiotic anemones was estimated at 2.02 × 105 CFUs/polyp and 1.10 × 106 CFUs/mg host protein, respectively (control animals). Thus, the number of CFUs was higher in symbiotic polyps by about an order of magnitude compared to aposymbiotic polyps (unpaired t-test, P = 0.025, Supplementary Table 4). We did not obtain CFUs from antibiotic-treated anemones, confirming successful bacterial depletion (Figure 3B). Interestingly, in some cases (re-)infection of gnotobiotic Aiptasia with microbiome inocula of either aposymbiotic Aiptasia or corals increased the carrying capacity of aposymbiotic animals. For instance, aposymbiotic anemones exposed to Porites sp. inoculum exhibited a significant increase in CFU counts to an average of 1.85 × 105 CFUs/polyp (Dunnett’s test P < 0.05, Figure 3B and Supplementary Tables 2, 4). Conversely, inoculation of symbiotic anemones (SYM+ACRinoc and SYM+PORinoc) did not result in a significant increase in CFU counts (Dunnett’s test P > 0.3, Figure 3B and Supplementary Tables 2, 4). Overall, the carrying capacity of Aiptasia polyps with ∼5 mm of oral disk was between 104 and 105 CFUs for apo- and symbiotic anemones, respectively. After microbiome inoculation the carrying capacity was at about 105 CFUs/polyp, irrespective of the symbiotic condition.
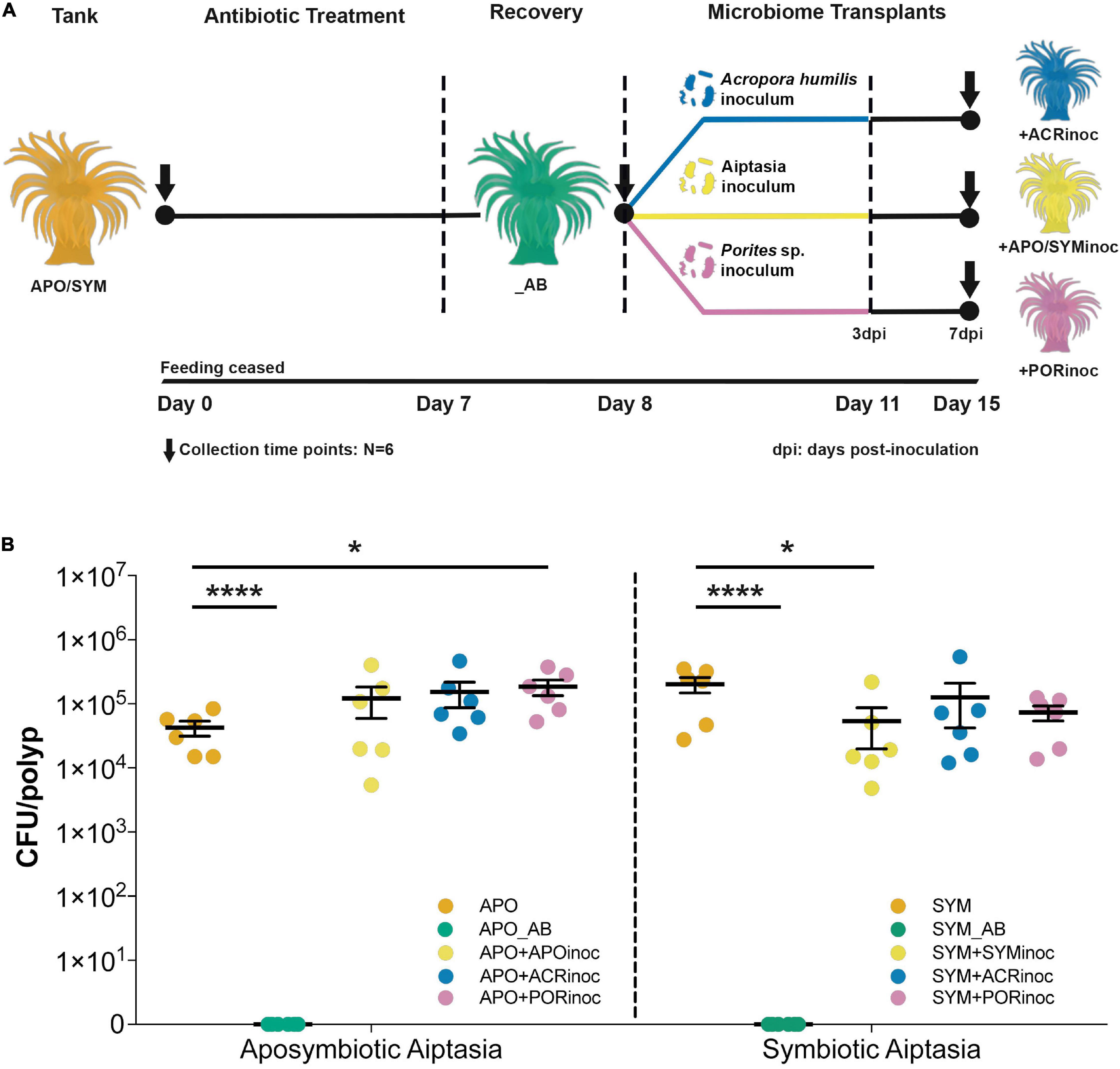
Figure 3. Gnotobiotic Aiptasia, microbiome transplants, and carrying capacity of Aiptasia sea anemones. (A) Overview of the microbiome transplant experiment. Anemones underwent antibiotic treatment for 7 days, followed by a 1-day recovery. Animals were then inoculated with preparations of Acropora humilis, Porites sp., and Aiptasia microbiomes for 3 days, after which inocula were removed (water exchange) and animals were incubated for 4 additional days (total of 7 days). Dotted vertical lines denote a change in the external environment. (B) Carrying capacity of Aiptasia sea anemones. CFU counts were determined across all experimental conditions and for apo- (left) and symbiotic (right) anemones separately. Depicted is the mean and standard error for the respective experimental condition (*P ≤ 0.05; ****P < 0.0001). APO/SYM, apo- and symbiotic control anemones; APO_AB/SYM_AB, 7-day antibiotic-treated anemones with a subsequent 1-day recovery; APO+APOinoc, APO microbiome transplantation after antibiotic treatment; SYM+SYMinoc, SYM microbiome transplantation after antibiotic treatment; APO/SYM+ACRinoc, Acropora humilis microbiome transplantation after antibiotic treatment (7 days after inoculation); APO/SYM+PORinoc, Porites sp. microbiome transplantation after antibiotic treatment (7 days after inoculation).
Bacterial Community Composition of Native and Inoculated Aiptasia
We employed RNA-based 16S amplicon sequencing to assess active bacterial community composition and dynamics (in contrast to the resident community based on DNA-based 16S sequencing) associated with Aiptasia under the various experimental treatments (Figure 4). Bacterial community composition differed significantly between treatments (PERMANOVA, P = 0.001) (Supplementary Table 5). As previously reported based on DNA-based 16S marker gene sequencing (Röthig et al., 2016a), bacterial communities of apo- and symbiotic Aiptasia anemones were different (PERMANOVA, P = 0.015, Figure 4B). For this reason, we clustered apo- and symbiotic samples separate to resolve differences within apo- and symbiotic groups as a result of the treatments (Figure 4A). For both groups, antibiotic-treated anemones (APO_AB and SYM_AB) were clearly separated from control anemones (APO and SYM) and both were different from gnotobiotic anemones re-inoculated with microbial communities (with the exception of SYM+SYMinoc that closely clustered with SYM anemones) (Figure 4A).
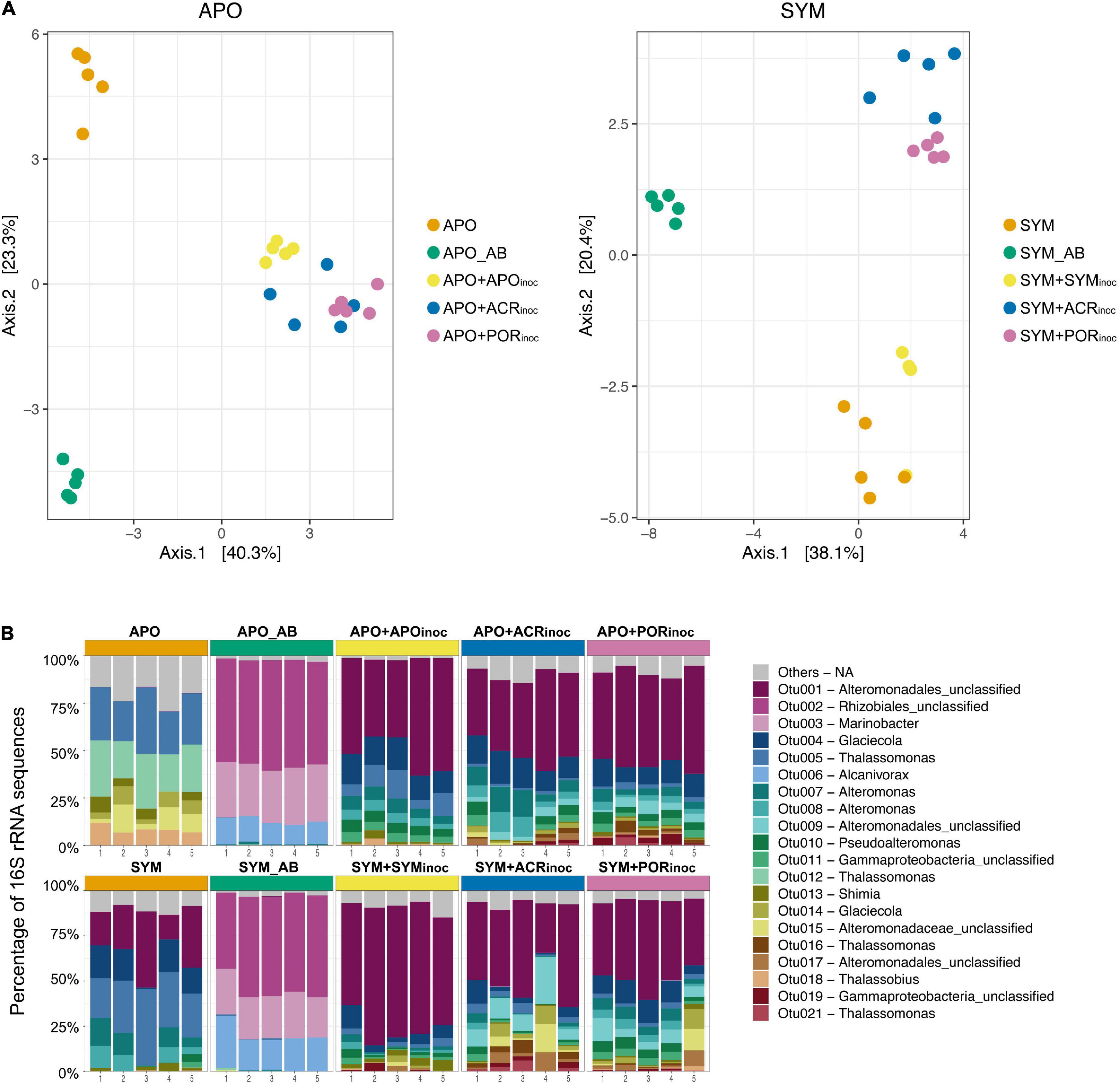
Figure 4. Bacterial community composition and dynamics of Aiptasia polyps 7 days after microbiome transplantation. (A) Principal Coordinate Analysis (PCoA) based on Bray-Curtis distances of OTU log10(x + 1)-transformed abundances. (B) 16S rRNA amplicon-based active bacterial community composition at the OTU level. Depicted are the 20 most abundant (i.e., active) OTUs across all samples (refer to Figure 3A for an overview over time points sampled). Remaining OTUs are grouped as “Others.” APO/SYM, apo- and symbiotic control anemones; APO_AB/SYM_AB, 7-day antibiotic-treated anemones with a subsequent 1-day recovery; APO+APOinoc, APO microbiome inoculation after antibiotic treatment; SYM+SYMinoc, SYM microbiome inoculation after antibiotic treatment; APO/SYM+ACRinoc, Acropora humilis microbiome inoculation after antibiotic treatment (7 days after inoculation); APO/SYM+PORinoc, Porites sp. microbiome inoculation after antibiotic treatment (7 days after inoculation).
The bacterial assemblage of antibiotic-treated anemones (APO_AB and SYM_AB) was markedly different from the microbiomes of other anemones in that it was considerably less complex. This was highlighted by the notion that >90% of the active community in apo- and symbiotic anemones was comprised of the same three bacterial taxa (OTU0002, OTU0003, and OTU0006) affiliated to the order Rhizobiales and the genera Marinobacter and Alcanivorax (Figure 4B). Conversely, 75% of the active community of aposymbiotic control anemones was comprised of only six bacterial taxa (OTUs 0005, 0012, 0013, 0014, 0015, 0018, Figure 4B), which were largely absent in the antibiotic treated Aiptasia. Notably, >70% of the bacterial communities of all inoculated aposymbiotic anemones were dominated by the same taxa regardless of the inoculum. The dominant bacterial taxa were representatives of the order Alteromonadales (OTU0001, OTU0004, OTU0007, OTU0008), which were barely detected in aposymbiotic control anemones. For the symbiotic anemones, the active bacterial community of control polyps was dominated by a few bacterial taxa of the order Alteromonadales and the genera Glaciecola and Thalassomonas (Figure 4B). Similarly, microbiome transplanted symbiotic Aiptasia resembled each other and were dominated by bacteria of the order Alteromonadales (OTU0001, OTU0004, OTU0007, OTU0008, OTU0009), just as the microbiome transplanted aposymbiotic Aiptasia. Thus, despite the differences in microbial community composition of apo- and symbiotic control anemones, microbiome transplanted gnotobiotic apo- and symbiotic anemones look much more alike with regard to their microbiome.
To get better insight into the bacterial taxa that were present in Aiptasia after microbiome transplantation, we compared the active bacterial community of control conditions (APO/SYM) and treatment conditions (Figure 5 and Supplementary Table 6). This comparison shows that all inoculated anemones (+APOinoc, +SYMinoc, +ACRinoc, and +PORinoc) harbored bacterial taxa that were already present (active) in control anemones as well as “novel” (i.e., previously undetected) bacteria. This may point to potential carryover from gnotobiotic anemones (not all bacteria were depleted). In the case of coral microbiome inoculations, we observed association with novel bacteria from the coral microbiomes (as determined by the comparison to APO and SYM anemones, Figure 5 and Supplementary Table 6). In particular, two bacteria taxa, of the genus Thalassomonas (OTU0016) and an unclassified Alteromonadales (OTU0021), are worthwhile mentioning because they were both found to be abundant (i.e., active) in coral microbiome transplanted Aiptasia (i.e., APO+ACRinoc, APO+PORinoc, SYM+ACRinoc, and SYM+PORinoc), but absent from Aiptasia transplanted with their own microbiomes (i.e., APO+APOinoc and SYM+SYMinoc) (Supplementary Table 6). Both OTUs are putative coral microbiome bacteria that were able to colonize apo- and symbiotic Aiptasia. On the contrary, a putative coral microbiome taxon from the genus Glaciecola (OTU0195) was only found associated with symbiotic anemones. Further, we found two Gammaproteobacteria (OTU0067 and OTU0099) to be specifically prevalent in anemones inoculated with Porites sp. microbiome preparations.
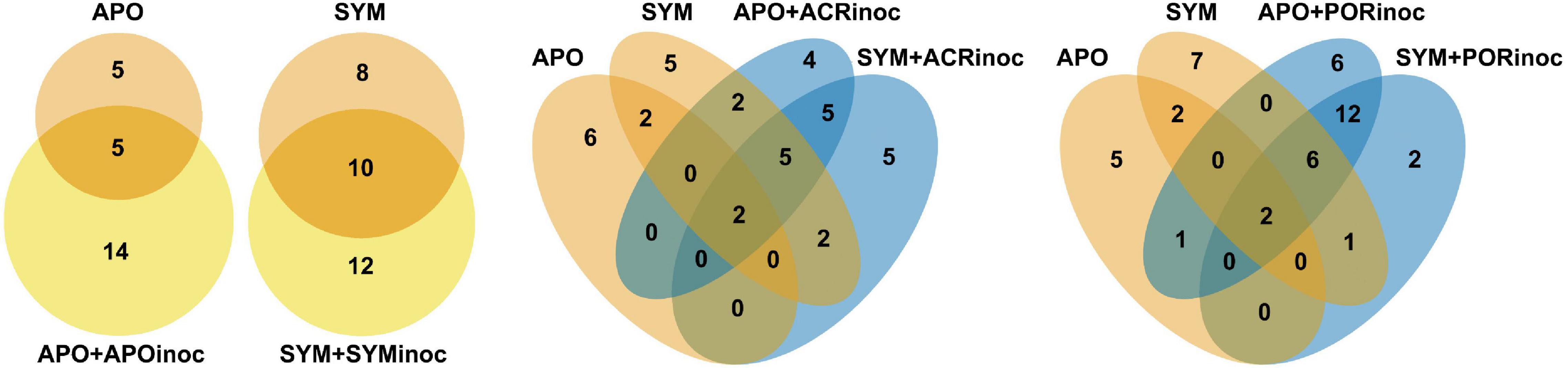
Figure 5. Bacterial taxa of control and microbiome transplanted Aiptasia. Venn diagrams representing the number of active OTUs ubiquitously present in all control anemones (APO/SYM), shared between APO/SYM anemones and transplanted anemones (APO+APOinoc, APO+ACRinoc, APO+PORinoc and SYM+SYMinoc, SYM+ACRinoc, SYM+PORinoc), and ubiquitously present in transplanted anemones 7 days after the microbiome transplantation. In the absence of sequenced inocula we chose to assess bacterial taxa that were consistently present in coral inoculated Aiptasia, but not present in control Aiptasia, as an indication for bacteria of coral origin. APO/SYM, apo- and symbiotic control anemones; APO_AB/SYM_AB, 7-day antibiotic-treated anemones with a subsequent 1-day recovery; APO+APOinoc, APO microbiome transplantation after antibiotic treatment; SYM+SYMinoc, SYM microbiome transplantation after antibiotic treatment; APO/SYM+ACRinoc, Acropora humilis microbiome transplantation after antibiotic treatment (7 days after inoculation); APO/SYM+PORinoc, Porites sp. microbiome transplantation after antibiotic treatment (7 days after inoculation).
Discussion
It is becoming increasingly clear that the specific composition and abundance of certain bacterial species affect host health and fitness (McFall-Ngai et al., 2013). At the same time, recent studies highlight that bacterial associations are not stable over time, but rather, assemble flexibly following fluctuations in the prevailing environment(s) (Roder et al., 2015; Ziegler et al., 2017; Ziegler et al., 2019; Voolstra and Ziegler, 2020). On the one hand, such observations support the notion that microbiome community changes can support host ecological adaption to environmental change (Reshef et al., 2006; Voolstra and Ziegler, 2020). On the other hand, the premise of flexibility gives rise to the idea of probiotics, i.e., inoculation with beneficial bacteria to support metaorganism stress resilience (Peixoto et al., 2017, 2019, 2021). The latter is gaining attention with regards to reef-building corals given the alarming loss of coral reef cover over recent decades (Hughes et al., 2017a, 2020). However, besides the acknowledged importance of coral-associated bacteria (Bang et al., 2018; van Oppen and Blackall, 2019) and the promise of coral probiotics to work in principle (Rosado et al., 2019), we are still missing answers to many of the basic questions surrounding cnidarian microbiome building principles and the underlying mechanistic aspects. Aiptasia is an emerging model system to study cnidarian symbioses, and here we set out to build a foundation for bacterial functional studies using the Aiptasia model by characterizing surface topography, carrying capacity, and assessing the prospect of microbiome transplants.
Our results show pronounced differences in the ultrastructure of the surface ectoderm between Hydra and anthozoans with implications for microbial association. SEM analyses of Hydra, N. vectensis, Aiptasia, and three scleractinian coral species suggest that bacterial epibionts in anthozoans are (more) restricted to the surface mucus layer (SML) in comparison to hydrozoans. Notably, Hydra anemones feature a smooth surface ectoderm where the epithelial glycocalyx (i.e., the pericellular matrix made of glycoproteins and glycolipids that cover the plasma membrane of the epithelium) promotes bacterial attachment and lacks extensive ectodermal ciliation that may prevent bacterial adhesion (Kesimer et al., 2013; Fraune et al., 2014; Wein et al., 2018). Other hydrozoans such as Ectopleura crocea and Cladonema sp. have shown colonizing epibionts attached to the glycocalyx, which ranged from 200 nm to 1 μm in thickness from the hydroid ectoderm to the epibiont, and their surface topography was also shown to be smoother, resembling that of Hydra (di Camillo et al., 2012; Abouna et al., 2015). Although Hydra can be considered a derived hydrozoan due to its life history (e.g., lack of a medusoid stage and freshwater habitat), imaging results from the current and other studies (e.g., di Camillo et al., 2012; Abouna et al., 2015) support the general notion that hydrozoans and anthozoans differ in their surface topographies, with anthozoans featuring a ciliated, rough surface, and a more defined ectoderm-SML separation in contrast to hydrozoans that seem to exhibit a smooth(er) less ciliated surface. It has been shown that the SML of corals is highly dynamic, with SML being cyclically shed (Bythell and Wild, 2011). This is thought to prevent pathogen colonization from the surrounding environment (Shnit-Orland and Kushmaro, 2009; Glasl et al., 2016), but might also explain the flexible microbial association of corals commonly found across different environments (Roder et al., 2015; Röthig et al., 2016b, 2017; Ziegler et al., 2017; Voolstra and Ziegler, 2020) and may contribute to the efficacy of coral probiotics (Peixoto et al., 2017, 2021). At a more basal level, the difference in surface topography between hydrozoans and anthozoans argues for the need of having distinct cnidarian models to reflect the implied microbial association differences. For the two anthozoan models, N. vectensis and Aiptasia, there was little difference in terms of ectodermal topography and bacterial colonization between the two organisms, both at the column and tentacle level. However, microalgal symbionts in the family Symbiodiniaceae (LaJeunesse et al., 2018) were shown to putatively contribute to the composition of coral mucus through their exudates (Brown and Bythell, 2005; Nelson et al., 2013), which affect bacterial association (Matthews et al., 2020), and Symbiodiniaceae were also shown to harbor themselves specific bacteria (Maire et al., 2021). As such, Aiptasia is a model system not only for the study of coral-algal symbiosis, but also for the study of bacterial associations and for testing the capacity for microbiome manipulation as further discussed below.
We were surprised by the lack of observed bacterial colonization at the surface ectoderm of Aiptasia (anthozoans more generally) using SEM, which was readily apparent in Hydra using the same technique. As alluded to above, this might be a consequence of surface topography differences, which ultimately affect bacterial colonization, but also differences in how the preparation affects sample integrity. In the case of Hydra, the accessible glycocalyx was preserved during SEM preparation, whereas the mucus of the anthozoans species was lost by employing a classical SEM sample protocol. Indeed, a modified TEM protocol developed to preserve the mucus layer also confirmed the presence of bacteria in the surface mucus layer of Aiptasia polyps, but not in the ectodermal layer or glycocalyx (Supplementary Figure 1). This highlights the need for continuous development and improvements of protocols to visualize bacterial association (Wada et al., 2016). For instance, TEM analyses may be combined with CARD-FISH staining for improved specificity and visualization of Aiptasia- and coral-associated bacteria besides the exploration of other methods (Kesimer et al., 2013).
Complementary to the visual assessment of bacterial abundance, we determined a bacterial carrying capacity of ∼ 104 to 105 bacterial cells per Aiptasia polyp, pending on the symbiotic state. This number is similar to the bacterial density reported for Hydra (Wein et al., 2018) and may roughly equate to the ∼106 bacterial cells/cm2 coral tissue previously reported (Koren and Rosenberg, 2006; Garren and Azam, 2012). It is interesting to note that our bacterial capacity was an order of magnitude higher for symbiotic (105 bacterial cells/polyp) in comparison to aposymbiotic (104 bacterial cells/polyp) anemones. This difference may arise from the additional niche space provided by the symbiosome, which was previously shown to harbor bacteria (Ainsworth et al., 2015), and through association with Symbiodiniaceae, which harbor their own microbial community (Deines et al., 2020; Maire et al., 2021). Visualization and enumeration of cnidarian-associated bacteria is still relatively rare (Neave et al., 2017; Cooke et al., 2019), in part because of the difficulties associated with fluorescent staining techniques in corals (Wada et al., 2016). As such, we relied on CFU counts, which avoid many of these difficulties but at the cost of media selectivity, as highlighted by the discrepancy between absence of CFUs in marine media and amplicon sequencing-based detection of some bacterial taxa. Literature perusal suggests that the most prevalent bacterial taxa detected in gnotobiotic anemones using sequencing (OTU0002, OTU0003, and OTU0006) cannot grow on our employed media and therefore escape CFU counting (Brooijmans et al., 2009; Yetti et al., 2015; Wang et al., 2016). Nevertheless, the antibiotic-treated anemones were highly bacteria depleted as more than 95% of the sequencing reads represented only three OTUs, which correspond to less than 5% of the active taxa detected in control anemones. As such, we consider animals treated with our gnotobiotic protocol (Costa et al., 2019) highly bacteria depleted. Besides the availability of axenic or gnotobiotic animals, it is desirable to have bacterial isolates with fluorescent reporter plasmids for more accurate estimation, which also allows for tracking location and abundance of said bacteria, as shown in Hydra (Wein et al., 2018). On this note, viability-qPCR (Emerson et al., 2017) may comprise a molecular method for enumeration of the density of active bacteria, although we found that it requires significant optimization due to taxon-specific differences with regard to dye permeability. As alluded to above and recently (Röthig et al., 2016a), 75% of the relative microbial abundance is comprised of only a handful of bacterial taxa, making Aiptasia a “non-complex” coral model for microbiome studies with the promise to obtain bacterial isolates for functional testing and manipulation (Röthig et al., 2016a).
Our RNA-based sequencing analysis revealed that the community of active bacteria was less diverse in comparison to the DNA-based microbial community (Röthig et al., 2016a): we identified an average of 38 and 64 active bacterial taxa associated with apo- and symbiotic anemones, while DNA-based analysis of the standing community retrieved 109 and 118 distinct bacteria, respectively. This may seem like a stark difference; however, it is not straight-forward to compare RNA- and DNA-based bacterial communities. Since abundance estimates are relative, “absence” in the RNA-based community analysis merely indicates that we could identify less bacteria (at the current sequencing depth) and that there is a putative difference between the bacterial community that is “present” (DNA) and the one that is “active” (RNA). Nevertheless, we compared the overlap in OTU-assigned taxonomies at the genus level for CC7 from Röthig et al. (2016a) and this study, which showed that 65% (APO) and 84% (SYM) of the identified bacterial genera in the RNA-based analysis were also found in the DNA-based analysis. Notably, such comparisons have inherent biases due to taxonomic redundancies and differences in the taxonomic classification between different OTU datasets.
To begin to explore the prospect of Aiptasia microbiome manipulation as a tool to interrogate bacterial function and test the effect of probiotics on holobiont biology, we conducted a series of inoculations/transplants on gnotobiotic Aiptasia with microbiomes from control anemones and from two coral species. Acropora humilis was chosen because its microbiome is highly uneven and dominated by bacteria of the genus Endozoicomonas, which could be used for tracking of the microbial transplant, since Aiptasia CC7 seems devoid of Endozoicomonas (Röthig et al., 2016a). However, we did not detect Endozoicomonas in the microbiome of inoculated anemones (i.e., SYM+ACRinoc, APO+ACRinoc). This suggests that Endozoicomonas exhibit high (coral) host specificity, despite their broad and prevalent distribution across marine invertebrates (Neave et al., 2016, 2017; Rossbach et al., 2019). Moreover, Endozoicomonas reside within coral tissues (Neave et al., 2016, 2017), which may explain their absence after microbiome transplantation, because mucus-associated bacteria may be easier to transfer than tissue-associated bacteria. Such differences putatively provide important insight regarding the choice of bacteria targeted for microbiome transplants. In contrast to A. humilis, Porites sp. was chosen as a donor because it is considered an environmentally resilient species with a more even and diverse microbiome (Hadaidi et al., 2017; Robbins et al., 2019). As such, successful microbiome transplantation would allow for subsequent testing of altered stress susceptibility. First off, our results show that all transplanted animals harbor a significantly different active bacterial community when compared to control anemones with the general notion that inoculated Aiptasia resemble each other more than control animals (Figure 4 and Supplementary Table 5). This is not highly surprising given that formation of an established microbial community may take time and likely goes through processes of inter-bacterial communication, host-bacterial communication, and winnowing, all of which presumably affect microbiome composition (Franzenburg et al., 2013a; Bernasconi et al., 2019; Shibl et al., 2020). As such, microbial consortia associated with Aiptasia after microbiome transplantation might represent a mix of specific bacteria administered with the inocula and opportunistic, environmentally present bacteria (e.g., resistant bacteria that survived the antibiotic treatment and were attached to the wells of the rearing plate), in particular copiotrophs. Copiotrophs are known to rapidly (re)populate carbon-rich environments such as the surface mucus layer (Nelson et al., 2013; McDevitt-Irwin et al., 2017; Cárdenas et al., 2018; Hadaidi et al., 2019) and as such may “drive” initial microbial repopulation dynamics. This makes the long(er)-term tracking of microbiome assemblage dynamics after transplantation important. It also suggests that antibiotic treated Aiptasia without any subsequent inoculation should be included in future experimental designs as controls for determining load and type of “residual” bacteria. In addition, future experiments should also include microbiome transplantations of untreated Aiptasia, resembling current coral probiotics procedures (Peixoto et al., 2021). This would provide further insight regarding repopulation dynamics and constraints.
Many of the taxa found in control Aiptasia established themselves again after microbiome inoculation, such as bacteria in the genus Thalassomonas (OTU0005, OTU0012), Glaciecola (OU0014), Thalassobius (OTU0018), or Alteromonas (OTU0007) (Supplementary Table 6). Microbiome transplantations with inocula from A. humilis and Porites sp. were successful to the extent that (at least) some foreign bacterial taxa (some of which were specific to the coral species from which the inoculum was obtained) were present (active) in Aiptasia polyps, as evidenced by their detection 7 days after microbiome transplantation. Notably, unavailability of sequenced inocula and coral native microbiomes within the current study limited the extent to which we could identify bacterial taxa with broad host compatibility, suitable for cross-species microbiome manipulation. Due to this, we considered bacterial taxa that were consistently present in coral-inoculated but not present in control Aiptasia as of putative coral origin. Besides treatment-specific differences, we commonly found bacterial taxa belonging to the Alteromonadaceae, Rhodobacteraceae, and Gammaproteobacteria to be transferred. This is encouraging given that bacteria from these taxonomic affiliations are commonly found in coral microbiomes (Roder et al., 2014; Neave et al., 2017; Ziegler et al., 2017, 2019).
At large, our results suggest that cross-species microbiome manipulation via transplantation is possible (to a certain extent). That is to say, Aiptasia is a suitable system to test the function of (at least some) coral bacteria and their effect on holobiont biology. The obvious next step is to test for altered holobiont phenotypes, e.g., increased or decreased stress resilience, using a standardized framework (Voolstra et al., 2020) after microbial transplantation with subsequent elucidation of the underlying mechanism(s). In addition, to achieve consistent and stable changes of microbial host consortia, an improved understanding of inocula persistence, dispersion, location, bacterial load, and any putative long-term effects is wanted. Aiptasia seems like the model system of choice, given its taxonomic relatedness and physiological similarities with regard to Symbiodiniaceae association, microbiome composition, and surface and tissue complexity.
Conclusion
Bacteria affect the health and fitness of their hosts. Given the worldwide decline of corals and the reefs they build, a better understanding of the function of bacteria and their potential manipulation is important. To achieve this, the development of coral model systems is imperative. Here we set the foundation for Aiptasia as a model for the study of coral-bacterial interactions. We show that the surface ectoderm topography is highly similar between Aiptasia and corals, in line with overall similarities in the microbiome composition established previously. Building on these prospects, we have developed a protocol for the generation of gnotobiotic Aiptasia and determined the bacterial carrying capacity to perform microbiome manipulation experiments. Our results support the principal suitability of Aiptasia to microbiome manipulation and its putative ability to incorporate foreign bacterial species. Although more work is needed, our study is a first step and provides an avenue to study the function of self and foreign bacteria as well as to explore the mechanisms underlying microbial acquisition, association, and host specificity. Future work should incorporate standardized testing to elucidate the effect of altered microbiomes on holobiont phenotypes as well as the generation of fluorescently labeled bacterial isolates, which will allow for spatial/temporal tracking and enumeration of bacterial associates. The work presented here provides a foundation and we will continue to develop Aiptasia as a coral model for bacterial functional studies.
Data Availability Statement
Data determined in this study are available under NCBI BioProject PRJNA665377 (https://www.ncbi.nlm.nih.gov/bioproject/665377). Scripts used for data analysis, curation, and plotting are available at https://github.com/ajcardenasb/Aiptasia_microbiome_manipulation. Abundant bacterial OTU reference sequences are available under GenBank Accession numbers MW577132 – MW577168 (https://www.ncbi.nlm.nih.gov/nuccore/?term=MW577132:MW577168[accn]).
Author Contributions
CV, RC, and AC conceived and designed the experiments, analyzed the data, and wrote the manuscript. RC and AC collected and processed the samples. RC, AC, and CL-F generated the data. RC, AC, CL-F, GT, and CV generated the figures. RC, AC, CL-F, GT, AM, and CV interpreted the data. CL-F, AM, and CV provided tools, reagents, and methods. All authors contributed to the article and approved the submitted version.
Funding
Research reported in this publication was supported by KAUST baseline funds to CV.
Conflict of Interest
The authors declare that the research was conducted in the absence of any commercial or financial relationships that could be construed as a potential conflict of interest.
Acknowledgments
We acknowledge the KAUST Imaging and Characterization Core Lab for their support in TEM and SEM analysis and the KAUST Bioscience Core Lab (BCL) for their support with the NGS data generation. We would like to thank all collaborators who tested the bacteria depletion protocol and provided feedback. We would also like to thank Carol Buitrago-Lopez and Hagen Gegner for their help with the coral collection and maintenance. Hydra magnipapillata and Nematostella vectensis were kindly provided by Prof. Takashi Gojobori’s group.
Supplementary Material
The Supplementary Material for this article can be found online at: https://www.frontiersin.org/articles/10.3389/fmicb.2021.637834/full#supplementary-material
References
Abouna, S., Gonzalez-Rizzo, S., Grimonprez, A., and Gros, O. (2015). First description of sulphur-oxidizing bacterial symbiosis in a cnidarian (Medusozoa) living in sulphidic shallow-water environments. PLoS One 10:e0127625. doi: 10.1371/journal.pone.0127625
Ainsworth, T. D., Krause, L., Bridge, T., Torda, G., Raina, J.-B., Zakrzewski, M., et al. (2015). The coral core microbiome identifies rare bacterial taxa as ubiquitous endosymbionts. ISME J. 9, 2261–2274. doi: 10.1038/ismej.2015.39
Allemand, D., and Osborn, D. (2019). Ocean acidification impacts on coral reefs: from sciences to solutions. Reg. Stud. Mar. Sci. 28:100558. doi: 10.1016/j.rsma.2019.100558
Allen, M. R., Dube, O. P., Solecki, W., Aragón-Durand, F., Cramer, W., Humphreys, S., et al. (2018). “Framing and context,” in Global Warming of 1.5°C. An IPCC Special Report on the Impacts of Global Warming of 1.5°C Above Pre-Industrial Levels and Related Global Greenhouse Gas Emission Pathways, in the Context of Strengthening the Global Response to the Threat of Climate Change, Sustainable Development, and Efforts to Eradicate Poverty, eds V. Masson-Delmotte et al. (Geneva: IPCC).
Andersson, A. F., Lindberg, M., Jakobsson, H., Bäckhed, F., Nyrén, P., and Engstrand, L. (2008). Comparative analysis of human gut microbiota by barcoded pyrosequencing. PLoS One 3:e2836. doi: 10.1371/journal.pone.0002836
Augustin, R., Schröder, K., Murillo Rincón, A. P., Fraune, S., Anton-Erxleben, F., Herbst, E.-M., et al. (2017). A secreted antibacterial neuropeptide shapes the microbiome of Hydra. Nat. Commun. 8:698. doi: 10.1038/s41467-017-00625-1
Bang, C., Dagan, T., Deines, P., Dubilier, N., Duschl, W. J., Fraune, S., et al. (2018). Metaorganisms in extreme environments: do microbes play a role in organismal adaptation? Zoology 127, 1–19. doi: 10.1016/j.zool.2018.02.004
Baumgarten, S., Simakov, O., Esherick, L. Y., Liew, Y. J., Lehnert, E. M., Michell, C. T., et al. (2015). The genome of Aiptasia, a sea anemone model for coral symbiosis. Proc. Natl. Acad. Sci. U.S.A. 112:201513318. doi: 10.1073/pnas.1513318112
Bayer, T., Neave, M. J., Alsheikh-Hussain, A., Aranda, M., Yum, L. K., Mincer, T., et al. (2013). The microbiome of the red sea coral stylophora pistillata is dominated by tissue-associated endozoicomonas bacteria. Appl. Environ. Microbiol. 79, 4759–4762. doi: 10.1128/AEM.00695-13
Bernasconi, R., Stat, M., Koenders, A., Paparini, A., Bunce, M., and Huggett, M. J. (2019). Establishment of coral-bacteria symbioses reveal changes in the core bacterial community with host ontogeny. Front. Microbiol. 10:1529. doi: 10.3389/fmicb.2019.01529
Biquand, E., Okubo, N., Aihara, Y., Rolland, V., Hayward, D. C., Hatta, M., et al. (2017). Acceptable symbiont cell size differs among cnidarian species and may limit symbiont diversity. ISME J. 11, 1702–1712. doi: 10.1038/ismej.2017.17
Brooijmans, R. J. W., Pastink, M. I., and Siezen, R. J. (2009). Hydrocarbon-degrading bacteria: the oil-spill clean-up crew. Microb. Biotechnol. 2, 587–594. doi: 10.1111/j.1751-7915.2009.00151.x
Brown, B. E., and Bythell, J. C. (2005). Perspectives on mucus secretion in reef corals. Mar. Ecol. Prog. Ser. 296, 291–309. doi: 10.3354/meps296291
Brown, T., Otero, C., Grajales, A., Rodriguez, E., and Rodriguez-Lanetty, M. (2017). Worldwide exploration of the microbiome harbored by the cnidarian model, exaiptasia pallida (Agassiz in Verrill, 1864) indicates a lack of bacterial association specificity at a lower taxonomic rank. PeerJ 5:e3235. doi: 10.7717/peerj.3235
Bythell, J. C., and Wild, C. (2011). Biology and ecology of coral mucus release. J. Exp. Mar. Biol. Ecol. 408, 88–93. doi: 10.1016/j.jembe.2011.07.028
Cárdenas, A., Neave, M. J., Haroon, M. F., Pogoreutz, C., Rädecker, N., Wild, C., et al. (2018). Excess labile carbon promotes the expression of virulence factors in coral reef bacterioplankton. ISME J. 12, 59–76. doi: 10.1038/ismej.2017.142
Chen, H., and Boutros, P. C. (2011). VennDiagram: a package for the generation of highly-customizable venn and euler diagrams in R. BMC Bioinformatics 12:35. doi: 10.1186/1471-2105-12-35
Cooke, I., Mead, O., Whalen, C., Boote, C., Moya, A., Ying, H., et al. (2019). Molecular techniques and their limitations shape our view of the holobiont. Zoology 137:125695. doi: 10.1016/j.zool.2019.125695
Costa, R. M., Cárdenas, A., and Voolstra, C. R. (2019). Protocol for Bacterial Depletion of Aiptasia Anemones - Towards the Generation of Gnotobiotic/germ-Free Cnidarian Host Animals V.2. Protocols IO. Available online at: dx.doi.org/10.17504/protocols.io.7mrhk56.
Cziesielski, M. J., Liew, Y. J., Cui, G., Schmidt-Roach, S., Campana, S., Marondedze, C., et al. (2018). Multi-omics analysis of thermal stress response in a zooxanthellate cnidarian reveals the importance of associating with thermotolerant symbionts. Proc. R. Soc. B Biol. Sci. 285:20172654. doi: 10.1098/rspb.2017.2654
Deines, P., Hammerschmidt, K., and Bosch, T. C. G. (2020). Microbial species coexistence depends on the host environment. mBio 11:e807-20. doi: 10.1128/mBio.00807-20
di Camillo, C. G., Luna, G. M., Bo, M., Giordano, G., Corinaldesi, C., and Bavestrello, G. (2012). Biodiversity of prokaryotic communities associated with the ectoderm of Ectopleura crocea (Cnidaria, Hydrozoa). PLoS One 7:e39926. doi: 10.1371/journal.pone.0039926
Dietzel, A., Bode, M., Connolly, S. R., and Hughes, T. P. (2020). Long-term shifts in the colony size structure of coral populations along the great barrier reef. Proc. Biol. Sci. 287:20201432. doi: 10.1098/rspb.2020.1432
Domin, H., Zurita-Gutiérrez, Y. H., Scotti, M., Buttlar, J., Humeida, U. H., and Fraune, S. (2018). Predicted bacterial interactions affect in vivo microbial colonization dynamics in Nematostella. Front. Microbiol. 9:728. doi: 10.3389/fmicb.2018.00728
Dungan, A. M., Hartman, L. M., Tortorelli, G., Belderok, R., Lamb, A. M., Pisan, L., et al. (2020). Exaiptasia diaphana from the great barrier reef: a valuable resource for coral symbiosis research. Symbiosis 80, 195–206. doi: 10.1007/s13199-020-00665-0
Emerson, J. B., Adams, R. I., Román, C. M. B., Brooks, B., Coil, D. A., Dahlhausen, K., et al. (2017). Schrödinger’s microbes: tools for distinguishing the living from the dead in microbial ecosystems. Microbiome 5:86. doi: 10.1186/s40168-017-0285-3
Fisher, R., O’Leary, R. A., Low-Choy, S., Mengersen, K., Knowlton, N., Brainard, R. E., et al. (2015). Species richness on coral reefs and the pursuit of convergent global estimates. Curr. Biol. 25, 500–505. doi: 10.1016/j.cub.2014.12.022
Franzenburg, S., Fraune, S., Altrock, P. M., Künzel, S., Baines, J. F., Traulsen, A., et al. (2013a). Bacterial colonization of Hydra hatchlings follows a robust temporal pattern. ISME J. 7, 781–790. doi: 10.1038/ismej.2012.156
Franzenburg, S., Walter, J., Künzel, S., Wang, J., Baines, J. F., Bosch, T. C. G., et al. (2013b). Distinct antimicrobial peptide expression determines host species-specific bacterial associations. Proc. Natl. Acad. Sci. U.S.A. 110, E3730–E3738.
Fraune, S., Anton-Erxleben, F., Augustin, R., Franzenburg, S., Knop, M., Schröder, K., et al. (2014). Bacteria-bacteria interactions within the microbiota of the ancestral metazoan Hydra contribute to fungal resistance. ISME J. 9, 1543–1556. doi: 10.1038/ismej.2014.239
Fraune, S., and Bosch, T. C. G. (2007). Long-term maintenance of species-specific bacterial microbiota in the basal metazoan Hydra. Proc. Natl. Acad. Sci. U.S.A. 104, 13146–13151. doi: 10.1073/pnas.0703375104
Fraune, S., Bosch, T. C. G., and Augustin, R. (2009). Exploring host-microbe interactions in Hydra. Microbe Magazine 4, 457–462. doi: 10.1128/microbe.4.457.1
Garren, M., and Azam, F. (2012). Corals shed bacteria as a potential mechanism of resilience to organic matter enrichment. ISME J. 6, 1159–1165. doi: 10.1038/ismej.2011.180
Gegner, H. M., Rädecker, N., Ochsenkühn, M., Barreto, M. M., Ziegler, M., Reichert, J., et al. (2019). High levels of floridoside at high salinity link osmoadaptation with bleaching susceptibility in the cnidarian-algal endosymbiosis. Biol. Open 8:bio045591. doi: 10.1242/bio.045591
Glasl, B., Herndl, G. J., and Frade, P. R. (2016). The microbiome of coral surface mucus has a key role in mediating holobiont health and survival upon disturbance. ISME J. 10, 2280–2292. doi: 10.1038/ismej.2016.9
Grajales, A., and Rodríguez, E. (2014). Morphological revision of the genus Aiptasia and the family Aiptasiidae (Cnidaria, Actiniaria, Etridioidea). Zootaxa 3826, 55–100. doi: 10.11646/zootaxa.3826.1.2
Hadaidi, G., Gegner, H. M., Ziegler, M., and Voolstra, C. R. (2019). Carbohydrate composition of mucus from scleractinian corals from the central Red Sea. Coral Reefs 38, 21–27. doi: 10.1007/s00338-018-01758-5
Hadaidi, G., Röthig, T., Yum, L. K., Ziegler, M., Arif, C., Roder, C., et al. (2017). Stable mucus-associated bacterial communities in bleached and healthy corals of Porites lobata from the Arabian Seas. Sci. Rep. 7:45362. doi: 10.1038/srep45362
Har, J. Y., Helbig, T., Lim, J. H., Fernando, S. C., Reitzel, A. M., Penn, K., et al. (2015). Microbial diversity and activity in the Nematostella vectensis holobiont: insights from 16S rRNA gene sequencing, isolate genomes, and a pilot-scale survey of gene expression. Front. Microbiol. 6:818. doi: 10.3389/fmicb.2015.00818
Herrera, M., Ziegler, M., Voolstra, C. R., and Aranda, M. (2017). Laboratory-cultured strains of the sea anemone exaiptasia reveal distinct bacterial communities. Front. Mar. Sci. 4:115. doi: 10.3389/fmars.2017.00115
Hughes, D. J., Alderdice, R., Cooney, C., Kühl, M., Pernice, M., Voolstra, C. R., et al. (2020). Coral reef survival under accelerating ocean deoxygenation. Nat. Clim. Chang. 10, 296–307. doi: 10.1038/s41558-020-0737-9
Hughes, T. P., Anderson, K. D., Connolly, S. R., Heron, S. F., Kerry, J. T., Lough, J. M., et al. (2018a). Spatial and temporal patterns of mass bleaching of corals in the Anthropocene. Science 359, 80–83. doi: 10.1126/science.aan8048
Hughes, T. P., Kerry, J. T., Baird, A. H., Connolly, S. R., Dietzel, A., Eakin, C. M., et al. (2018b). Global warming transforms coral reef assemblages. Nature 556, 492–496. doi: 10.1038/s41586-018-0041-2
Hughes, T. P., Barnes, M. L., Bellwood, D. R., Cinner, J. E., Cumming, G. S., Jackson, J. B. C., et al. (2017a). Coral reefs in the Anthropocene. Nature 546, 82–90. doi: 10.1038/nature22901
Hughes, T. P., Kerry, J. T., Álvarez-Noriega, M., Álvarez-Romero, J. G., Anderson, K. D., Baird, A. H., et al. (2017b). Global warming and recurrent mass bleaching of corals. Nature 543, 373–377. doi: 10.1038/nature21707
Jaspers, C., Fraune, S., Arnold, A. E., Miller, D. J., Bosch, T. C. G., and Voolstra, C. R. (2019). Resolving structure and function of metaorganisms through a holistic framework combining reductionist and integrative approaches. Zoology 133, 81–87. doi: 10.1016/j.zool.2019.02.007
Johnston, I. S., and Rohwer, F. (2007). Microbial landscapes on the outer tissue surfaces of the reef-building coral Porites compressa. Coral Reefs 26, 375–383. doi: 10.1007/s00338-007-0208-z
Kesimer, M., Ehre, C., Burns, K. A., Davis, C. W., Sheehan, J. K., and Pickles, R. J. (2013). Molecular organization of the mucins and glycocalyx underlying mucus transport over mucosal surfaces of the airways. Mucosal Immunol. 6, 379–392. doi: 10.1038/mi.2012.81
Koren, O., and Rosenberg, E. (2006). Bacteria associated with mucus and tissues of the coral Oculina patagonica in summer and winter. Appl. Environ. Microbiol. 72, 5254–5259. doi: 10.1128/AEM.00554-06
LaJeunesse, T. C., Parkinson, J. E., Gabrielson, P. W., Jeong, H. J., Reimer, J. D., Voolstra, C. R., et al. (2018). Systematic revision of symbiodiniaceae highlights the antiquity and diversity of coral endosymbionts. Curr. Biol. 28, 2570.e6–2580.e6. doi: 10.1016/j.cub.2018.07.008
Lawson, C. A., Raina, J., Kahlke, T., Seymour, J. R., and Suggett, D. J. (2018). Defining the core microbiome of the symbiotic. Environ. Microbiol. Rep. 10, 7–11. doi: 10.1111/1758-2229.12599
Maire, J., Girvan, S. K., Barkla, S. E., Perez-Gonzalez, A., Suggett, D. J., Blackall, L. L., et al. (2021). Intracellular bacteria are common and taxonomically diverse in cultured and in hospite algal endosymbionts of coral reefs. ISME J. doi: 10.1038/s41396-021-00902-4 Online ahead of print
Martinez Arbizu, P. (2019). pairwiseAdonis: Pairwise Multilevel Comparison using Adonis. R Package Version 0.3 1.
Matthews, J. L., Raina, J. B., Kahlke, T., Seymour, J. R., van Oppen, M. J. H., and Suggett, D. J. (2020). Symbiodiniaceae-bacteria interactions: rethinking metabolite exchange in reef-building corals as multi-partner metabolic networks. Environ. Microbiol. 22, 1675–1687. doi: 10.1111/1462-2920.14918
McDevitt-Irwin, J. M., Baum, J. K., Garren, M., and Vega Thurber, R. L. (2017). Responses of coral-associated bacterial communities to local and global stressors. Front. Mar. Sci. 4:262. doi: 10.3389/fmars.2017.00262
McFall-Ngai, M., Hadfield, M. G., Bosch, T. C. G., Carey, H. V., Domazet-Lošo, T., Douglas, A. E., et al. (2013). Animals in a bacterial world, a new imperative for the life sciences. Proc. Natl. Acad. Sci. U.S.A. 110, 3229–3236.
McMurdie, P. J., and Holmes, S. (2013). Phyloseq: an r package for reproducible interactive analysis and graphics of microbiome census data. PLoS One 8:e61217. doi: 10.1371/journal.pone.0061217
Muscatine, L. (1990). “The role of symbiotic algae in carbon and energy flux in reef corals,” in Ecosystems of the World, ed. Z. Dubinsky (Amsterdam: Elsevier).
Neave, M. J., Apprill, A., Ferrier-Pagès, C., and Voolstra, C. R. (2016). Diversity and function of prevalent symbiotic marine bacteria in the genus Endozoicomonas. Appl. Microbiol. Biotechnol. 100, 8315–8324. doi: 10.1007/s00253-016-7777-0
Neave, M. J., Rachmawati, R., Xun, L., Michell, C. T., Bourne, D. G., Apprill, A., et al. (2017). Differential specificity between closely related corals and abundant Endozoicomonas endosymbionts across global scales. ISME J. 11, 186–200. doi: 10.1038/ismej.2016.95
Nelson, C. E., Goldberg, S. J., Wegley Kelly, L., Haas, A. F., Smith, J. E., Rohwer, F., et al. (2013). Coral and macroalgal exudates vary in neutral sugar composition and differentially enrich reef bacterioplankton lineages. ISME J. 7, 962–979. doi: 10.1038/ismej.2012.161
Oakley, C. A., Ameismeier, M. F., Peng, L., Weis, V. M., Grossman, A. R., and Davy, S. K. (2015). Symbiosis induces widespread changes in the proteome of the model cnidarian Aiptasia. Cell Microbiol. 18, 1009–1023. doi: 10.1111/cmi.12564
Peixoto, R. S., Rosado, P. M., Leite, D. C., de, A., Rosado, A. S., and Bourne, D. G. (2017). Beneficial microorganisms for corals (BMC): proposed mechanisms for coral health and resilience. Front. Microbiol. 8:341. doi: 10.3389/fmicb.2017.00341
Peixoto, R. S., Sweet, M., and Bourne, D. G. (2019). Customized medicine for corals. Front. Mar. Sci. 6:1–5. doi: 10.3389/fmars.2019.00686
Peixoto, R. S., Sweet, M., Villela, H. D. M., Cardoso, P., Thomas, T., Voolstra, C. R., et al. (2021). Coral probiotics: premise, promise, prospects. Annu. Rev. Anim. Biosci. 9, 265–288. doi: 10.1146/annurev-animal-090120-115444
Pogoreutz, C., Voolstra, C. R., Rädecker, N., Weis, V., Cardenas, A., and Raina, J.-B. (2020). “The coral holobiont highlights the dependence of cnidarian animal hosts on their associated microbes,” in Cellular Dialogues in the Holobiont, eds T. C. Bosch and M. G. Hadfield (Boca Raton, FL: CRC Press), 91–118. doi: 10.1201/9780429277375
Rädecker, N., Raina, J. B., Pernice, M., Perna, G., Guagliardo, P., Kilburn, M. R., et al. (2018). Using Aiptasia as a model to study metabolic interactions in cnidarian-symbiodinium symbioses. Front. Physiol. 9:214. doi: 10.3389/fphys.2018.00214
Reshef, L., Koren, O., Loya, Y., Zilber-Rosenberg, I., and Rosenberg, E. (2006). The coral probiotic hypothesis. Environ. Microbiol. 8, 2068–2073. doi: 10.1111/j.1462-2920.2006.01148.x
Robbins, S. J., Singleton, C. M., Chan, C. X., Messer, L. F., Geers, A. U., Ying, H., et al. (2019). A genomic view of the reef-building coral Porites lutea and its microbial symbionts. Nat. Microbiol. 4, 2090–2100. doi: 10.1038/s41564-019-0532-4
Roder, C., Arif, C., Daniels, C., Weil, E., and Voolstra, C. R. (2014). Bacterial profiling of white plague disease across corals and oceans indicates a conserved and distinct disease microbiome. Mol. Ecol. 23, 965–974. doi: 10.1111/mec.12638
Roder, C., Bayer, T., Aranda, M., Kruse, M., and Voolstra, C. R. (2015). Microbiome structure of the fungid coral Ctenactis echinata aligns with environmental differences. Mol. Ecol. 24, 3501–3511. doi: 10.1111/mec.13251
Rognes, T., Flouri, T., Nichols, B., Quince, C., and Mahé, F. (2016). VSEARCH: a versatile open source tool for metagenomics. PeerJ 2016, 1–22. doi: 10.7717/peerj.2584
Rohwer, F., Seguritan, V., Azam, F., and Knowlton, N. (2002). Diversity and distribution of coral-associated bacteria. Mar. Ecol. Prog. Ser. 243, 1–10. doi: 10.3354/meps243001
Rosado, P. M., Leite, D. C. A., Duarte, G. A. S., Chaloub, R. M., Jospin, G., Nunes da Rocha, U., et al. (2019). Marine probiotics: increasing coral resistance to bleaching through microbiome manipulation. ISME J. 13, 921–936. doi: 10.1038/s41396-018-0323-6
Rosenberg, E., Koren, O., Reshef, L., Efrony, R., and Zilber-Rosenberg, I. (2007). The role of microorganisms in coral health, disease and evolution. Nat. Rev. Microbiol. 5, 355–362. doi: 10.1038/nrmicro1635
Rossbach, S., Cardenas, A., Perna, G., Duarte, C. M., and Voolstra, C. R. (2019). Tissue-specific microbiomes of the red sea giant clam Tridacna maxima highlight differential abundance of endozoicomonadaceae. Front. Microbiol. 10:2661. doi: 10.3389/fmicb.2019.02661
Röthig, T., Costa, R. M., Simona, F., Baumgarten, S., Torres, A. F., Radhakrishnan, A., et al. (2016a). Distinct bacterial communities associated with the coral model Aiptasia in aposymbiotic and symbiotic states with symbiodinium. Front. Mar. Sci. 3:234. doi: 10.3389/fmars.2016.00234
Röthig, T., Ochsenkühn, M. A., Roik, A., van der Merwe, R., and Voolstra, C. R. (2016b). Long-term salinity tolerance is accompanied by major restructuring of the coral bacterial microbiome. Mol. Ecol. 25, 1308–1323. doi: 10.1111/mec.13567
Röthig, T., Roik, A., Yum, L. K., and Voolstra, C. R. (2017). Distinct bacterial microbiomes associate with the deep-sea coral Eguchipsammia fistula from the red sea and from aquaria settings. Front. Mar. Sci. 4:259. doi: 10.3389/fmars.2017.00259
Schloss, P. D., Westcott, S. L., Ryabin, T., Hall, J. R., Hartmann, M., Hollister, E. B., et al. (2009). Introducing mothur: open-source, platform-independent, community-supported software for describing and comparing microbial communities. Appl. Environ. Microbiol. 75, 7537–7541. doi: 10.1128/AEM.01541-09
Shibl, A. A., Isaac, A., Ochsenkühn, M. A., Cárdenas, A., Fei, C., Behringer, G., et al. (2020). Diatom modulation of select bacteria through use of two unique secondary metabolites. Proc. Natl. Acad. Sci. U.S.A. 117, 27445–27455. doi: 10.1073/pnas.2012088117
Shnit-Orland, M., and Kushmaro, A. (2009). Coral mucus-associated bacteria: a possible first line of defense. FEMS Microbiol. Ecol. 67, 371–380. doi: 10.1111/j.1574-6941.2008.00644.x
Simona, F., Zhang, H., and Voolstra, C. R. (2019). Evidence for a role of protein phosphorylation in the maintenance of the cnidarian–algal symbiosis. Mol. Ecol. 28, 5373–5386. doi: 10.1111/mec.15298
Trench, R. K. (1993). Microalgal-invertebrate symbioses - a review. Endocytobiosis Cell Res. 9, 135–175.
van Oppen, M. J. H., and Blackall, L. L. (2019). Coral microbiome dynamics, functions and design in a changing world. Nat. Rev. Microbiol. 17, 557–567. doi: 10.1038/s41579-019-0223-4
Voolstra, C. R. (2013). A journey into the wild of the cnidarian model system Aiptasia and its symbionts. Mol. Ecol. 22, 4366–4368. doi: 10.1111/mec.12464
Voolstra, C. R., Buitrago-López, C., Perna, G., Cárdenas, A., Hume, B. C. C., Rädecker, N., et al. (2020). Standardized short-term acute heat stress assays resolve historical differences in coral thermotolerance across microhabitat reef sites. Glob. Chang. Biol. 26, 4328–4343. doi: 10.1111/gcb.15148
Voolstra, C. R., and Ziegler, M. (2020). Adapting with microbial help: microbiome flexibility facilitates rapid responses to environmental change. BioEssays 42:e2000004. doi: 10.1002/bies.202000004
Wada, N., Pollock, F. J., Willis, B. L., Ainsworth, T., Mano, N., and Bourne, D. G. (2016). In situ visualization of bacterial populations in coral tissues: pitfalls and solutions. PeerJ 2016:e2424. doi: 10.7717/peerj.2424
Wang, G., Tang, M., Wu, H., Dai, S., Li, T., Chen, C., et al. (2016). Pyruvatibacter mobilis gen. Nov., sp. nov., a marine bacterium from the culture broth of Picochlorum sp. 122. Int. J. Syst. Evol. Microbiol. 66, 184–188. doi: 10.1099/ijsem.0.000692
Wein, T., Dagan, T., Fraune, S., Bosch, T. C. G., Reusch, T. B. H., and Hülter, N. F. (2018). Carrying capacity and colonization dynamics of Curvibacter in the hydra host habitat. Front. Microbiol. 9:443. doi: 10.3389/fmicb.2018.00443
Weis, V. M., Davy, S. K., Hoegh-Guldberg, O., Rodriguez-Lanetty, M., and Pringle, J. R. (2008). Cell biology in model systems as the key to understanding corals. Trends Ecol. Evol. 23, 369–376. doi: 10.1016/j.tree.2008.03.004
Wolfowicz, I., Baumgarten, S., Voss, P. A., Hambleton, E. A., Voolstra, C. R., Hatta, M., et al. (2016). Aiptasia sp. larvae as a model to reveal mechanisms of symbiont selection in cnidarians. Sci. Rep. 6:32366. doi: 10.1038/srep32366
Xiang, T., Hambleton, E. A., Denofrio, J. C., Pringle, J. R., and Grossman, A. R. (2013). Isolation of clonal axenic strains of the symbiotic dinoflagellate Symbiodinium and their growth and host specificity. J. Phycol. 49, 447–458. doi: 10.1111/jpy.12055
Yetti, E., Thontowi, A., Yopi, Y., and Lisdiyanti, P. (2015). Screening of marine bacteria capable of degrading various polyaromatic hydrocarbons. Squalen Bull. Mar. Fish. Postharvest Biotechnol. 10, 121–127. doi: 10.15578/squalen.v10i3.123
Ziegler, M., Grupstra, C. G. B., Barreto, M. M., Eaton, M., BaOmar, J., Zubier, K., et al. (2019). Coral bacterial community structure responds to environmental change in a host-specific manner. Nat. Commun. 10:3092. doi: 10.1038/s41467-019-10969-5
Keywords: coral model system, Exaiptasia diaphana, metaorganism, holobiont, microbiome, symbiosis, gnotobiotic, axenic
Citation: Costa RM, Cárdenas A, Loussert-Fonta C, Toullec G, Meibom A and Voolstra CR (2021) Surface Topography, Bacterial Carrying Capacity, and the Prospect of Microbiome Manipulation in the Sea Anemone Coral Model Aiptasia. Front. Microbiol. 12:637834. doi: 10.3389/fmicb.2021.637834
Received: 04 December 2020; Accepted: 19 February 2021;
Published: 08 April 2021.
Edited by:
Aram Mikaelyan, North Carolina State University, United StatesReviewed by:
Brittany Leigh, Vanderbilt University, United StatesElizabeth Hambleton, University of Vienna, Austria
Copyright © 2021 Costa, Cárdenas, Loussert-Fonta, Toullec, Meibom and Voolstra. This is an open-access article distributed under the terms of the Creative Commons Attribution License (CC BY). The use, distribution or reproduction in other forums is permitted, provided the original author(s) and the copyright owner(s) are credited and that the original publication in this journal is cited, in accordance with accepted academic practice. No use, distribution or reproduction is permitted which does not comply with these terms.
*Correspondence: Christian R. Voolstra, Y2hyaXMudm9vbHN0cmFAZ21haWwuY29t