- 1State Key Laboratory of Wheat and Maize Crop Science, College of Plant Protection, Henan Agricultural University, Zhengzhou, China
- 2Bureau of Agriculture and Rural Affairs of Qixian, Kaifeng, China
Insect resistance to Bacillus thuringiensis (Bt) insecticidal proteins has rapidly evolved with the expansion of the planting area of transgenic Bt crops. Pyramiding RNA interference (RNAi) and Bt in crops is urgently needed to counter the rapid increase in pest resistance. The ideal “pyramid” strategy simultaneously targets different action pathways that exert synergetic effects on each other. Here, we identified a dephosphatase, namely, Helicoverpa armigera calcineurin (HaCAN), which might enhance the insecticidal activity of Cry1Ac against Helicoverpa armigera by regulating immune gene expression via dephosphatase activity, but not by acting as a receptor. Notably, blocking enzyme activity or knocking down endogenous HaCAN significantly promoted the enhancement in Cry1Ac toxicity to insect larvae and cells. Correspondingly, the increase in HaCAN activity reduced the cytotoxicity of Cry1Ac as shown by the heterologous expression of HaCAN. Our results provide a probable that HaCAN is an important candidate gene for pyramiding RNAi and Cry1Ac crops to control cotton bollworm.
Introduction
Transgenic Bacillus thuringiensis (Bt) Cry1Ac crops have provided significantly economic and environmental benefits since they were first introduced in 1996 for the control of major Lepidopteran pests (Mendelsohn et al., 2003; Hutchison et al., 2010; Tabashnik et al., 2010; Naranjo, 2011; Lu et al., 2012; Downes et al., 2017). Therefore, over the past 22 years, Bt crops have been planted by millions of farmers from 1 million in 1996 to over 100 million in 2017 and 2018 (ISAAA, 2018). However, the extensive planting of Bt crops has led to the increase in the selection pressure for Bt resistance, and led to the increasingly rapid evolution of pest resistance. Field-evolved resistance to Bt toxins in some insect pests reduces the benefits of Bt (Tabashnik and Carrière, 2017).
The simultaneous expression of multiple Bt toxins in crops has been considered as a principal strategy for insecticide resistance management to delay pest adaptation (Brévault et al., 2013). However, the durability and efficacy of such Bt crops are reduced by antagonism or cross resistance between Bt toxins (Carrière et al., 2015, 2016). Thus, RNA interference (RNAi) is a promising new alternative for the effective management of insect pests, particularly Bt-resistant insects (Mamta and Rajam, 2017; Ni et al., 2017). RNAi (targeting JHAMT or JHBP) and simultaneous Cry1Ac expression in cotton can be used to control Cry1Ac-resistant cotton bollworm (Ni et al., 2017). However, associated studies have showed that in Chilo suppressalis larvae, the knockdown of ATPase subunit A increases resistance to Cry2Aa- and Cry1Ca-expressing rice (Qiu et al., 2019). Therefore, the ideal “pyramid” strategy should simultaneously target different action pathways with synergetic effects on each other. The knockout of an immune gene Sl 102 in Spodoptera littoralis was recently verified to improve the effect of spray applications of the Bt-based biopesticide (XentariTM) (Caccia et al., 2020). In fact, feeding with Bt toxins themselves activates a series of immune signal pathways (Chai et al., 2008; Xia et al., 2016; Portugal et al., 2017). Meanwhile, the genes that are involved in the immune pathway can significantly affect insect development (Luo and Dearolf, 2010; Wei et al., 2019a). Thus, immune genes have attracted the attention of researchers, and are considered as candidate genes for the RNAi + Bt for insect control strategy.
Some immune genes, such as p38, jnk, C-type lectins, and HSP70 that participate in insect defense and immune responses, are reportedly involved in Bt toxicity (Huffman et al., 2004; Chai et al., 2008; Cancino-Rodezno et al., 2010; Oppert et al., 2012; Xia et al., 2016; Portugal et al., 2017). Nevertheless, the roles of these immune genes in combination with Bt toxins in insect control remain elusive. In fact, although many immune-related genes in Helicoverpa armigera have been reported (Wang et al., 2010), their functions in Bt toxicity are rarely reported. Our previous study screened one important Ca2+-dependent phosphatase, calcineurin (CAN), which serves as an immune activator by binding with relish, a key transcription factor of the immune deficiency (IMD) pathway, to affect the development of cotton bollworm by regulating antimicrobial peptide (AMP) expression (Wei et al., 2019a). CAN has been identified as a dephosphatase of protein phosphatase 2B family and participates in various biological pathways (Furman and Norris, 2014), including immunity pathways (Bueno et al., 2002; Kang et al., 2007; Furman and Norris, 2014; Ballesteros-Martinez et al., 2017). In mammals, CAN is a mediator that participates in innate immunity and anaphylaxis (Bueno et al., 2002; Kang et al., 2007; Ballesteros-Martinez et al., 2017). In insects, Gram-negative bacteria can induce CAN activity to promote the production of relish, and activated relish subsequently induces the expression of antimicrobial genes (Dijkers and O’Farrell, 2007; Li and Dijkers, 2015).
To consider the potential value of the use of CAN in the RNAi + Bt strategy, we investigated CAN role in the insecticidal activity of Cry1Ac against cotton bollworm. In this study, we used the CAN-specific inhibitor, FK506 to block CAN activity, and tested the insecticidal activity of Cry1Ac against cotton bollworm through a diet bioassay. The expression level and enzyme activity of CAN in larvae after Cry1Ac treatment were analyzed. We examined the binding characteristics of HaCAN and Cry1Ac by using the split-ubiquitin based membrane yeast two-hybrid (MYTH) system (Stagljar et al., 1998; Möckli et al., 2007; Snider et al., 2010). We also conducted in vitro gain and loss of function analyses by expressing endogenous HaCAN and HaCAN double-stranded RNA (dsRNA) in Sf9 cells and Helicoverpa zea midgut cells, respectively. Our findings indicated that HaCAN was not a receptor of Cry1Ac, but was rather an activator of the immune gene. HaCAN was involved in the insecticidal activity of Cry1Ac against cotton bollworm. Our results provided a probable that HaCAN is an important candidate gene for pyramiding RNAi and Cry1Ac in crops to control cotton bollworm.
Materials and Methods
Insects and Cell Lines
Helicoverpa armigera larvae were purchased on October 2018 (Henan Jiyuan Baiyun Industry Co., Ltd.) and maintained in the laboratory condition with artificial diet (Zhao et al., 2009).
Helicoverpa zea midgut cell line RP-HzGUT-AW1 (MG) was generously provided by Dr. Xianchun Li (University of Arizona, Tucson, AZ, United States) (Goodman et al., 2004). Spodoptera frugiperda cell line (Sf9) and MG cell line were routinely maintained at a constant temperature incubator, and the culture mediums were prepared as the description in Wei et al. (2016, 2018a, 2019b).
Bt Toxins
Cry1Ac protoxin and activated Cry1Ac were purchased from Beijing General Pest Biotech Research Co., Ltd.1. The activated Cry1Ac proteins were re-dissolved in Excell 420 insect serum-free medium or Sf-900 II SFM media following the previous description (Wei et al., 2016).
Bioassays
We tested the susceptibility of second instar larvae to Cry1Ac (2 μg/mL, about the 40% mortality for second instar larvae) toxins or FK506 (APEBIO; 10, 20, and 50 μM) singly or in Cry1Ac (2 μg/mL) + FK506 (10, 20, and 50 μM) combinations. Before the bioassay, the newly molting second instar larvae were first starved for 12 h before diet bioassay. The 50 mM pH 10.0 Na2CO3 (dissolve Cry1Ac, as a buffer control to adjust the mortality of Cry1Ac treatment), DMSO (dissolve FK506, as a buffer control to adjust the mortality of FK506 treatments), and Na2CO3 + FK506 (as a buffer control to adjust the mortality of Cry1Ac + FK506 treatments) treatments were used as buffer controls, and the same volumes of buffer as that of the treatments were used. All these toxins or inhibitors were mixed with artificial diet. For each treatment (single toxin, combination, or control), 48–64 same size second instar larvae (16 larvae for each replicate) were transferred to the wells (one larva per well) in 24-well plates. The larvae mortalities daily until 7 days’ post-exposure to these above treatments were recorded.
The Analysis of the Expression and Activity of CAN Induced by Cry1Ac
To investigate the effect of feeding with 25 μg/mL Cry1Ac (about the 30% mortality for fifth instar larvae) on HaCAN expression, 10 larvae (fifth instar) were treated with three biological replicates. After feeding at different time (6, 12, 24, 48, and 72 h), the larvae were collected and corresponding midgut tissues were harvested.
To analyze the effect of feeding with Cry1Ac, FK506, and Cry1Ac + FK506 on CAN activity, the new molting of fifth instar larvae was selected. 12 h after starvation, the larvae were refed with the artificial diet containing 25 μg/mL Cry1Ac (about the 30% mortality for fifth instar larvae), 50 μM FK506, and Cry1Ac (25 μg/mL) + FK506 (50 μM) for 72 h, respectively. The 50 mM pH 10.0 Na2CO3 (dissolve Cry1Ac) and DMSO (dissolve FK506) were used as buffer controls. The larvae were collected and the midgut tissues were harvested.
Membrane Yeast Two-Hybrid (MYTH) Screen Assay
A full open reading frame (ORF) of Cry1Ac was PCR-amplified from Bt HD73 plasmids, using the gene-specific primers pBT3-N-Cry1Ac (forward: ATCGAATTCCTGCAG GGCCATTACGGCCATGGATAACAATCCGAACATC; reverse: TACTTACCATGGGGCCGAGGCGGCCCTATTCCTCCATAAG GAGTAATTCC; with the SfiI restriction site). After the SfiI digestion of the PCR products, Cry1Ac was cloned into the bait vector pBT3-N (DUALsystems BioTech). HaCAN was cloned into expression vector pPR3-N (Figure 4B), the construction of HaCAN was prepared in our previous study, and it had been shown that pPR3-N-CAN can express in yeast cells and work well (Wei et al., 2019a).
Four treatments were introduced into competent cells of the Yeast strain NMY51 (DUAL systems BioTech) with lithium acetate (LiAc)/PEG-mediated transformation, including 100 ng pTSU2-APP and 100 ng pNubG-Fe65, 100 ng pBT3-N-Cry1Ac and 100 ng pOst1-NubI, 100 ng pBT3-N-Cry1Ac and 100 ng pPR3-N, and 100 ng pBT3-N-Cry1Ac and 100 ng pPR3-N-HaCAN. The detail of co-transformation method followed the previous description (Wei et al., 2019a). Then, the interaction pairs were screened on four SD (Synthetic Defined Drop-out Medium) plates as the previous description (Wei et al., 2019a). Finally, we photographed the colonies of each prey and bait construct pair on the above four plates.
Plasmid of HaCAN-GFP-pIEx Constructs
The whole ORF was cloned into pIEx-RFP vector [kindly provided by Professor Xiaofan Zhao (Shandong University, School of Life Sciences)]. First, we designed gene-specific primers of HaCAN in Supplementary Table S1. HaCAN ORF was amplified with the primers HaCAN-5′ Sac I and HaCAN-5′ Bgl II and template HaCAN-pMD-18T DNA (Wei et al., 2019a). PCR products were cloned into pGEM®-T vector (Promega, Madison, WI, United States), followed by restriction enzyme digestion with Sac I and Bgl II (New England Biolabs, Ipswich, MA, United States). The pIEx-RFP vector was also digested with Sac I and Bgl II at 37°C overnight. Ligation reaction of pIEx-RFP with HaCAN was carried out at 16°C overnight and was transfected to DH5α cells. Recombinant plasmids were extracted for subsequent use.
Double-Strand RNA Synthesis
HaCAN and EGFP dsRNA were synthesized using the MEGAscript RNAi kit (Ambion, Vilnius, Lithuania) as the description of using gene-specific primers containing T7 polymerase sites (all the related primers are listed in Supplementary Table S1) to prepare the templates for dsRNA synthesis (Du et al., 2012, 2017). A 466 bp cDNA fragment of HaCAN was PCR-amplified from HaCAN-pMD-18T (Wei et al., 2019a) to generate the template for in vitro dsRNA synthesis. Amino acid sequence analysis indicated that CAN in H. armigera shared 99% amino acid identity with H. zea CAN homolog (unpublished data). The same fragment of HaCAN and HzCAN can be used in H. zea MG cells to test the function of CAN. The enhanced green fluorescent protein (EGFP) template (a negative control) was prepared as the previous descriptions in Du et al. (2012). The synthesized dsRNAs of HaCAN and EGFP were then eluted in DEPC water and dsRNA concentrations were measured by a BioPhotometer (Eppendorf, Hauppauge, NY, United States) (Du et al., 2017).
Transient Transfection and Cell Bioassay
Sf9 and H. zea midgut cells were seeded onto a 12-well plate (costar, 9 × 105cells/well), allowing cells to attach for 3 h. Then, cells were transiently (5 h) transfected with 1.5 μg/well pIEx-RFP-CAN plasmid (or 50 mM DsCAN) using FuGENE HD Tran section Reagent (Promega; 8 μL per well). Empty pIEx-RFP (or DsEGFP) vector was used as control. Five hours after transfection, transfection mixture was removed and replaced by the newly supplemented medium (according to the description in Wei et al., 2016, 2018a, 2019b). Sixty-four hours after cell maintenance at 28°C, the cells that transfected pIEx-RFP-CAN or pIEx-RFP were observed under the fluorescence microscope (Zeiss, LSM780) to check the expression of proteins. After 64 h post-transfection, the cells were also collected and the concentration of cells was measured with hemocytometer by using trypan blue; then the treated cells were reseeded in a 96-well micro-plate (costar) for 100 μL of cells (10,000 cells), allowing cells to attach about 2 h. We tested the cytotoxicity of the treated cells by using Cry1Ac toxin (100 μg/mL for expressing HaCAN and 50 μg/mL for knockdown of HaCAN) for 4 h; finally, the cell mortality was calculated under the inverted microscope (OPTIKA IM-5) (Wei et al., 2016, 2018a, 2019b). The remaining treated cells of each independent transfection were collected, centrifuged at 1000 × g for 10 min, washed with an equal volume of cold PBS buffer for three times, and then stored them at −80°C for subsequent RNA or protein extraction to make sure the successful transfection.
Protein Extraction and Western Blot
The cell proteins were extracted using 100 μL RIPA lysis buffer (Wei et al., 2016) with 10 μL PMSF (to prevent the degradation of proteins) and 25 μL 5× SDS loading buffer (Wei et al., 2016); 20 μL of protein mixture was separated on 10% sodium dodecyl sulfate polyacrylamide gel electrophoresis (SDS-PAGE) and electroblotted to 0.45 μm polyvinylidene difluoride (PVDF) (Solarbio) membrane in 25 mM Tris, 192 mM glycine, 10% methanol, PH 8.3. The membrane was blocked with PBST (137 mM NaCl, 2.7 mM KCl, 10 mM Na2HPO4, 2 mM KH2PO4, pH 7.4, 0.1% Tween 20) and 5% fat-free milk (Sangon, Shanghai) for 1 h at 25°C. Then mouse-anti-RFP (1:1000) was added to the blocking buffer; 1 h after incubation at 25°C, the membrane was washed for five times (5 min for each time) using PBST. Then sheep anti-mouse secondary antibody (Beijing ComWin Biotech Co., Ltd.) (1:10,000) was added to PBST for 1 h at 25°C with constant shaking. Finally, immune-band was detected with immobilon western electrochemiluminescence HRP substrate (ECL, Abbkine) and with Tanon-4600 photographic.
Determination of CAN Activity
The dephosphorylase activity of CAN was tested using a Calcineurin Activity Assay Kit [cat. no. ab139461; Abcam (Cambridge, MA, United States)] according to the previously described methods (Li et al., 2017; Zhao et al., 2018).
RT-PCR
Total RNA was extracted from collected cells and midgut samples with Total RNA Isolation Mini kit (Ambion, Vilnius, Lithuania). The resultant RNA samples were treated with DNase I (Fermentas, EU) to remove potential DNA contamination. RNA samples were reverse transcribed into first-strand cDNA with First-Strand cDNA Synthesis Kit (Fermentas, EU).
To test the expression levels of HaCAN, qRT-PCR was carried out using Applied Biosystems 7500 Fast Real-Time PCR system (ABI, Carlsbad, CA, United States). H. armigera ribosomal protein gene 18S (RP18S) and EF1-α were used as reference genes (Du et al., 2017; Wei et al., 2019a). qRT-PCR reaction system, thermocycler conditions, melting curve analysis, and the calculation method for expression level of the HaCAN followed previously described methods (Liu et al., 2015; Wei et al., 2019a). The primers used for the RT-qPCR in the methods are listed in Supplementary Table S1; the sizes of amplicons, amplification efficiency, and annealing temperature of each pair of primers are also listed in Supplementary Table S1.
Statistical Analysis
We compared the expected mortality for each combination of FK506 and Cry1Ac with the observed mortalities of each combination to evaluate the interactions between FK506 and Cry1Ac (Tabashnik et al., 2013; Wei et al., 2015). The expected mortality of each combination is calculated using the following formula:
where S(Cry1Ac)OBS is the corrected observed survival rate under the treatment of Cry1Ac and S(FK506)OBS is the corrected observed survival rate under the treatment of FK506. The differences between expected and observed mortalities of each combination were analyzed by Student’s t-test (DPSSOFT: DPS7.05).
Significant difference in the expression level of HaCAN between CK (buffer control) and treatments (feeding Cry1Ac) at each different time point was compared with Student’s t-test (DPSSOFT: DPS7.05).
Significant difference in the enzyme activities of HaCAN at different treatments was compared with the Tukey’s test with P < 0.05 (DPSSOFT: DPS7.05).
Cell mortality was calculated with the Abbott formula (Abbott, 1925) as the description in Wei et al. (2016, 2019b). Significant differences in cell mortalities, relative expression level, and enzyme activities of HaCAN between CAN-GFP-pIEx (dsCAN) and GFP-pIEx (dsEGFP) were compared using Student’s t-test (DPSSOFT: DPS7.05).
Results
HaCAN Expression Was Induced by Cry1Ac
We investigated the expression levels of HaCAN transcript at 6, 12, 24, 48, and 72 h after feeding with Cry1Ac. Comparison with the corresponding controls revealed that HaCAN transcript had significantly increased (6 h, P = 0.0001; 12 h, P = 0.0018; 24 h, P = 0.0001; 48 h, P = 0.0099; 72 h, P = 0.0001) (Figure 1). Notably, a 6.53-fold increase in the expression level of HaCAN transcript was observed at 72 h after Cry1Ac treatment (Figure 1).
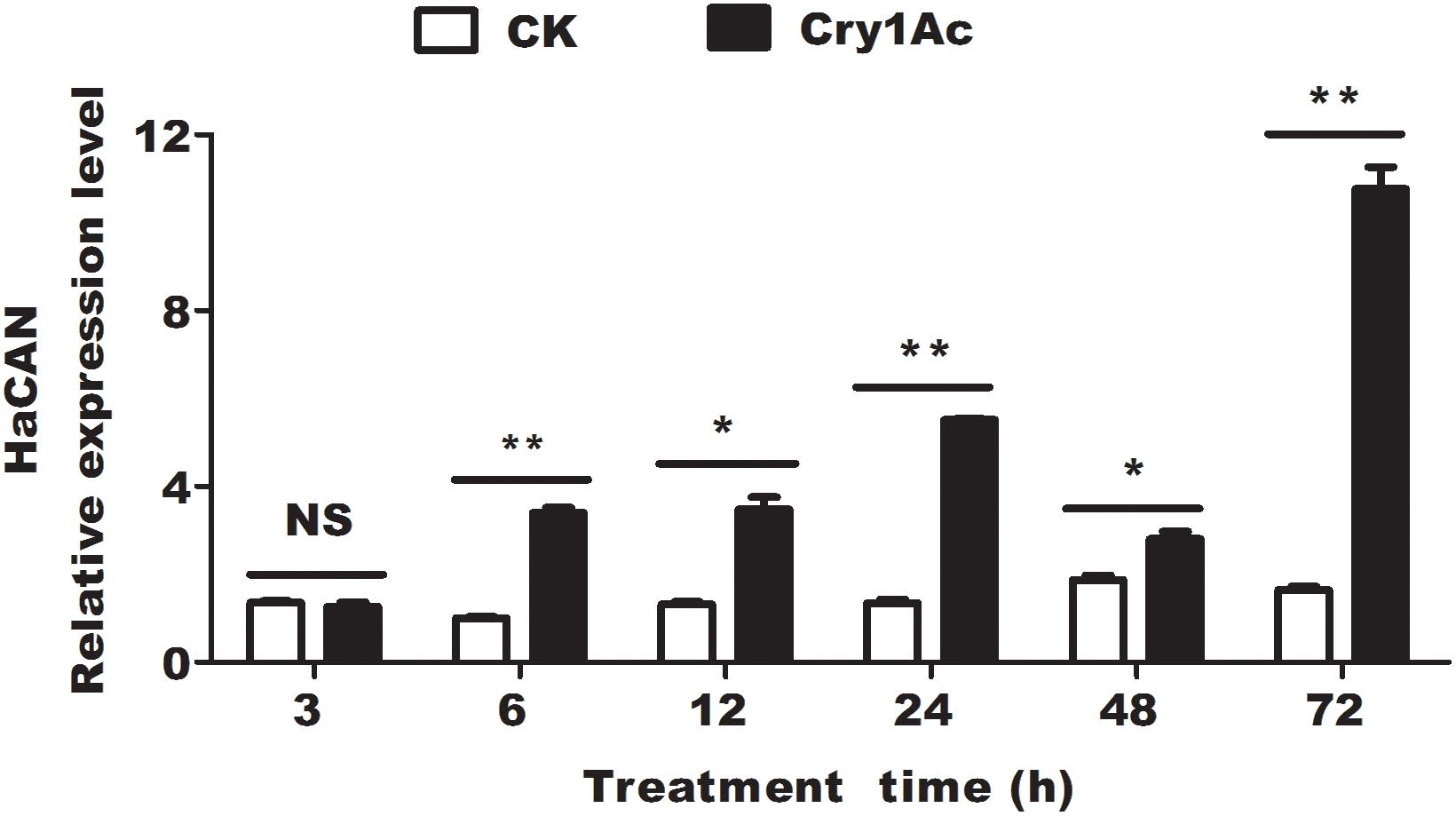
Figure 1. The effect of feeding with CAN inhibitor FK506 on the expression level of HaCAN. Each error bar represents the standard error of the mean from three biological replicates. Significant differences between CK (buffer control) and treatments (feeding Cry1Ac) at different time points are indicated with asterisks (*P < 0.05, based on Student’s t-tests, DPS7.05).
HaCAN Activity Was Induced by Cry1Ac and Inhibited by FK506
FK506 feeding caused the significant suppression of HaCAN activity by comparing with the buffer control at 72 h. Feeding with 25 μg/mL Cry1Ac led to a significant increase in HaCAN activity. However, the increase in HaCAN activity induced by Cry1Ac was attenuated by FK506 (P = 0.0001; Figure 2).
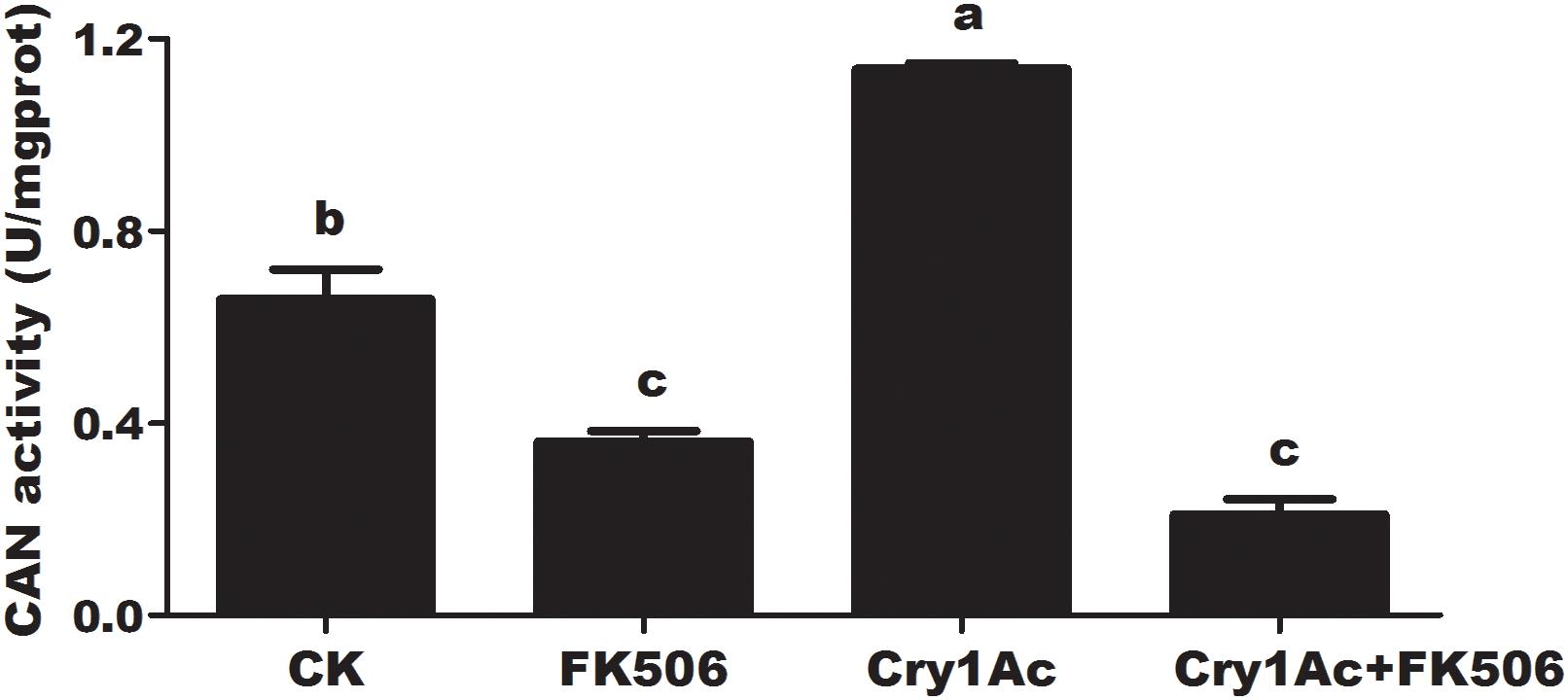
Figure 2. The effect of feeding with FK506, Cry1Ac, and Cry1Ac + FK506 on HaCAN activity in the midgut of larvae. Each error bar represents the standard error of the mean from three biological replicates. Significant differences among the different treatments are indicated with different lowercase letters (P < 0.05, based on Tukey’s test, DPS7.05).
Inhibition of HaCAN Activity Increased the Insecticidal Activity of Cry1Ac to Larvae
To investigate the effect of FK506 on Cry1Ac toxicity, FK506 was added to an artificial diet containing Cry1Ac. Feeding with 10 μM FK506 + Cry1Ac and 20 μM FK506 + Cry1Ac resulted in considerably higher observed corrected mortalities than expected corrected mortalities (Figure 3). When the larvae were fed on artificial diets containing a high dose of FK506 (50 μM FK506 + Cry1Ac), the observed corrected mortality reached 78.05%, whereas the expected corrected mortality was only 42.18% (Figure 3). The mixture of 50 μM FK506 + Cry1Ac significantly improved the insecticidal activity of Cry1Ac against cotton bollworm (P = 0.0473) (Figure 3).
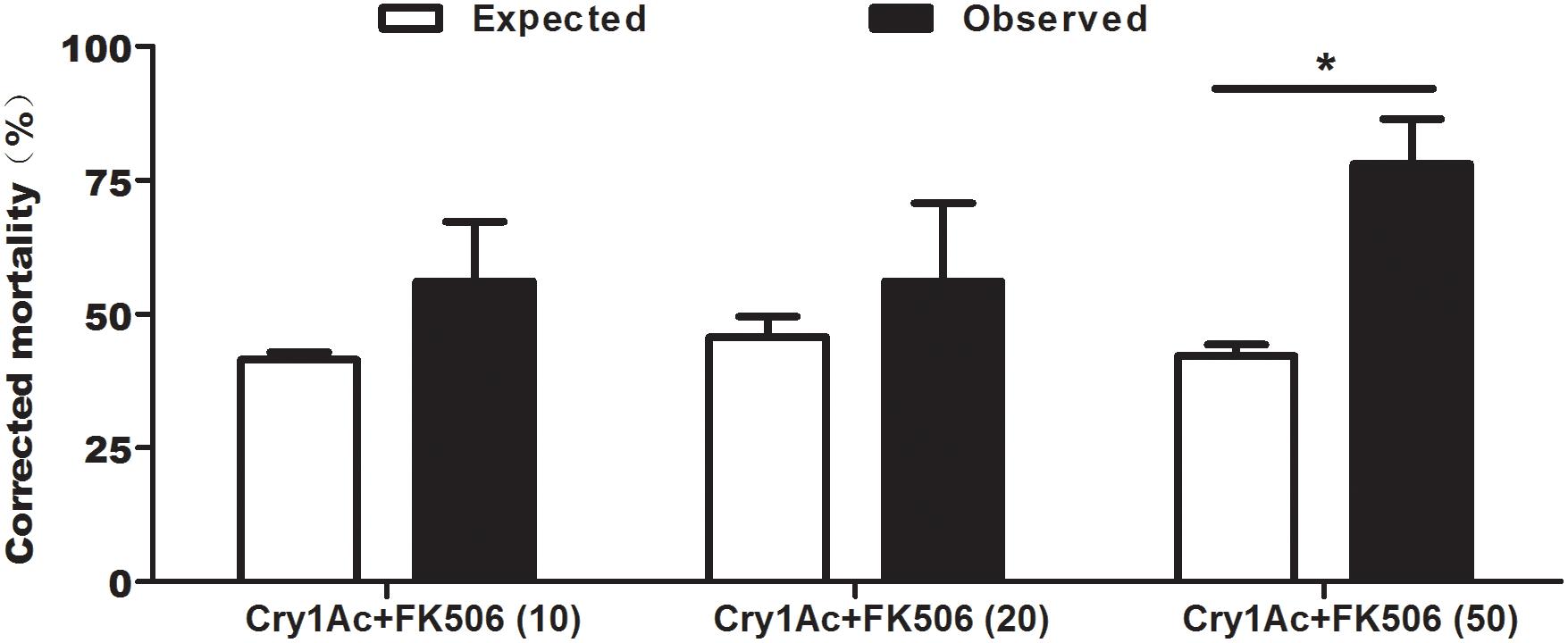
Figure 3. Expected versus observed mortalities caused by the combinations of Cry1Ac and FK506 against H. armigera. Each error bar represents the standard error of the mean from three biological replicates. 10, 20, and 50 indicate the concentrations of FK506 in μM. Asterisk shows significant differences between observed and expected mortality at P < 0.05 level (based on Student’s t-tests, DPS7.05).
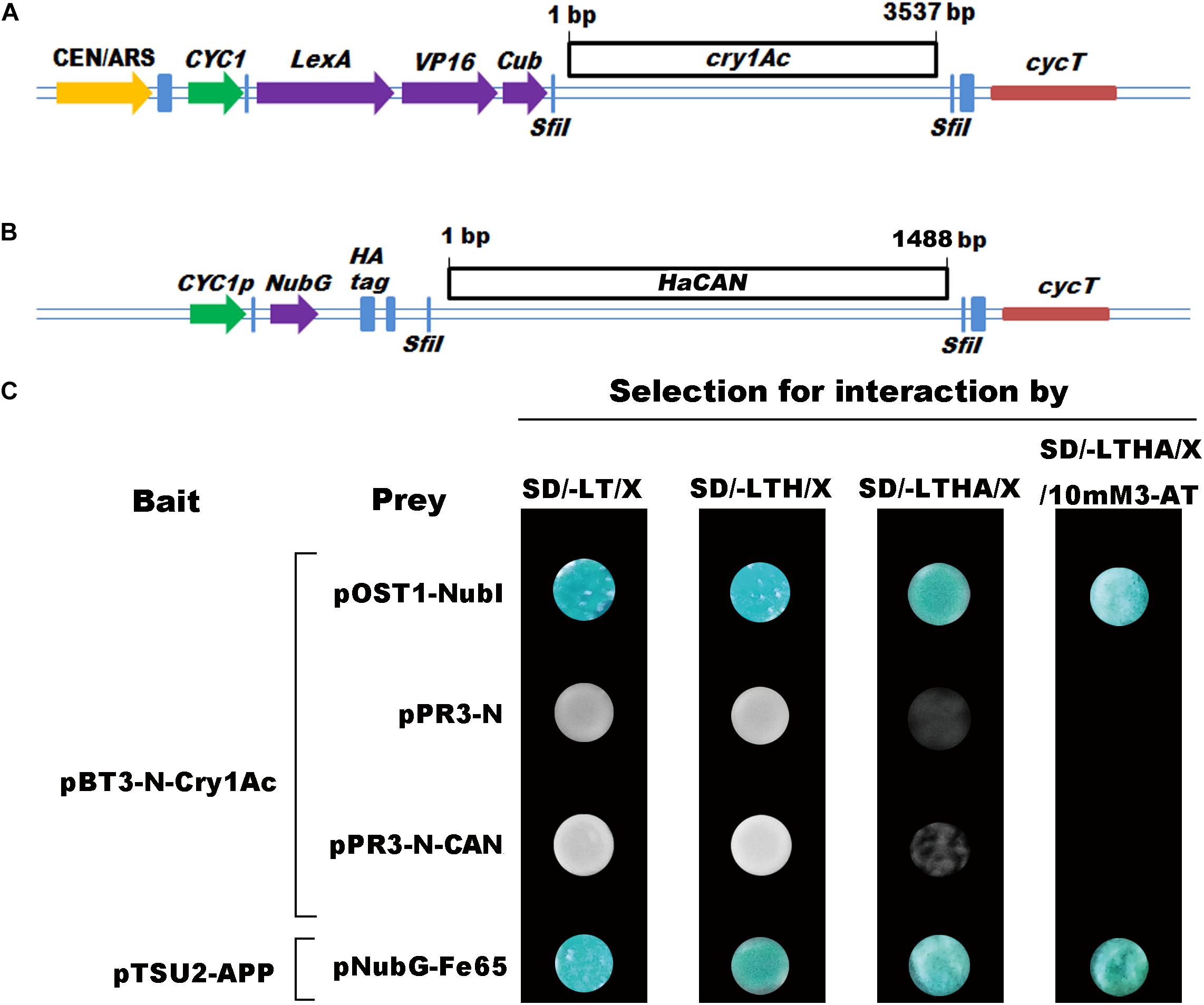
Figure 4. The interactions between Cry1Ac and HaCAN based on yeast two-hybrid system. Diagrammatic representation of the bait (A) and prey (B) constructs. SD/-LT/X (SD-leucine-tryptophan + 40 mg/L X-Gal media) (X-Gal soluted in N,N-dimethylformamide), SD/-LTH/X (SD-leucine-tryptophan-histidine + 40 mg/L X-Gal media), SD/-LTHA/X (SD-leucine-tryptophan-histidine-adenine + 40 mg/L X-Gal media), and SD/-LTHA/X/10mM3-AT (SD-leucine-tryptophan-histidine-adenine + 40 mg/L X-Gal media + 10 mM 3-AT) plates, respectively. (C) Representative growth of NMY51 yeast cells co-transformed with one of the seven pairs of prey and bait constructs on four SD medium plates with increasing selection stringency. The treatment of pTSU2-APP and pNubG-Fe65 was positive control. The treatment of pBT3-N-Cry1Ac and pPR3-N was negative control.
Interaction Between HaCAN and Cry1Ac
Cry1Ac was inserted into the pBT3-N bait vector, which was fused with the C-terminal half of ubiquitin (Cub) and an artificial transcription factor (LexA-VP6) (Figure 4A). Before the interactions, the positive control of the transformants of pTSU2-APP and pNubG-Fe65 was found to work effectively (Figure 4C; Yu et al., 2015). This result verified the utility of the split-ubiquitin-based MYTH system to identify protein–protein interactions, including cytosolic proteins. The transformants of pBT3-N-Cry1Ac and pOst1-NubI grew well and turned blue in β-galactosidase assay on SD/-LT/X (X-α-Gal dissolved in N, N-dimethylformamide), SD/-LTH/X, SD/-LTHA/X, and SD/-LTHA/X/10mM3-AT plates (Figure 4C). These results showed that plasmids of pBT3-N-Cry1Ac worked well in this experiment. The transformations of pBT3-N-Cry1Ac and pPR3-N did not turn blue in the β-galactosidase assay of SD/-LT/X, SD/-LTH/X, SD/-LTHA/X, and SD/-LTHA/X/10mM3-AT plates (Figure 4C), which indicated that Cry1Ac could not interact with the NubG fused non-sense peptide. In our previous study, we prepared a HaCAN construct (Figure 4B) and showed that pPR3-N-CAN can be expressed in yeast cells and work well (Wei et al., 2019a). We had verified the interaction between Cry1Ac and HaABCC2, a known receptor of Cry1Ac by co-transforming the Cry1Ac bait construct pBT3-N-Cry1Ac and the HaABCC2 prey construct pPR3-N-HaABCC2 (our submitted paper), which indicated that this split-ubiquitin MYTH system could be used to identify the interaction between Bt toxins and receptors. The transformants of pBT3-N-Cry1Ac and pPR3-N-HaCAN did not turn blue in β-galactosidase assay of SD/-LT/X, SD/-LTH/X, SD/-LTHA/X, and SD/-LTHA/X/10 mM 3-AT plates. This result indicated that Cry1Ac and HaCAN could not interact directly (Figure 4C).
Heterologous Expression of HaCAN Affected Cry1Ac Cytotoxicity Against Sf9 Cells
pIEx-RFP-CAN and pIEx-RFP (empty vector) plasmids were transfected into Sf9 cells. Red fluorescence was observed under a fluorescence microscope, indicating that the corresponding protein had been successfully expressed in the cells (Figures 5A,B). After the successfully expression of HaCAN in Sf9 cells, the HaCAN proteins were detected in transfected cells as shown by Western blot results (Figure 5C). Correspondingly, HaCAN activity was also significantly improved (Figure 5D), compared with that in the control (P = 0.001) (Figure 5D). Importantly, the overexpression of HaCAN led to a significant reduction in cell susceptibility to Cry1Ac (Figure 5E) (P = 0.0347). Photographs also showed that the overexpression of HaCAN in Sf9 cells can could reduce the cytotoxicity of Cry1Ac (Figures 5F,G).
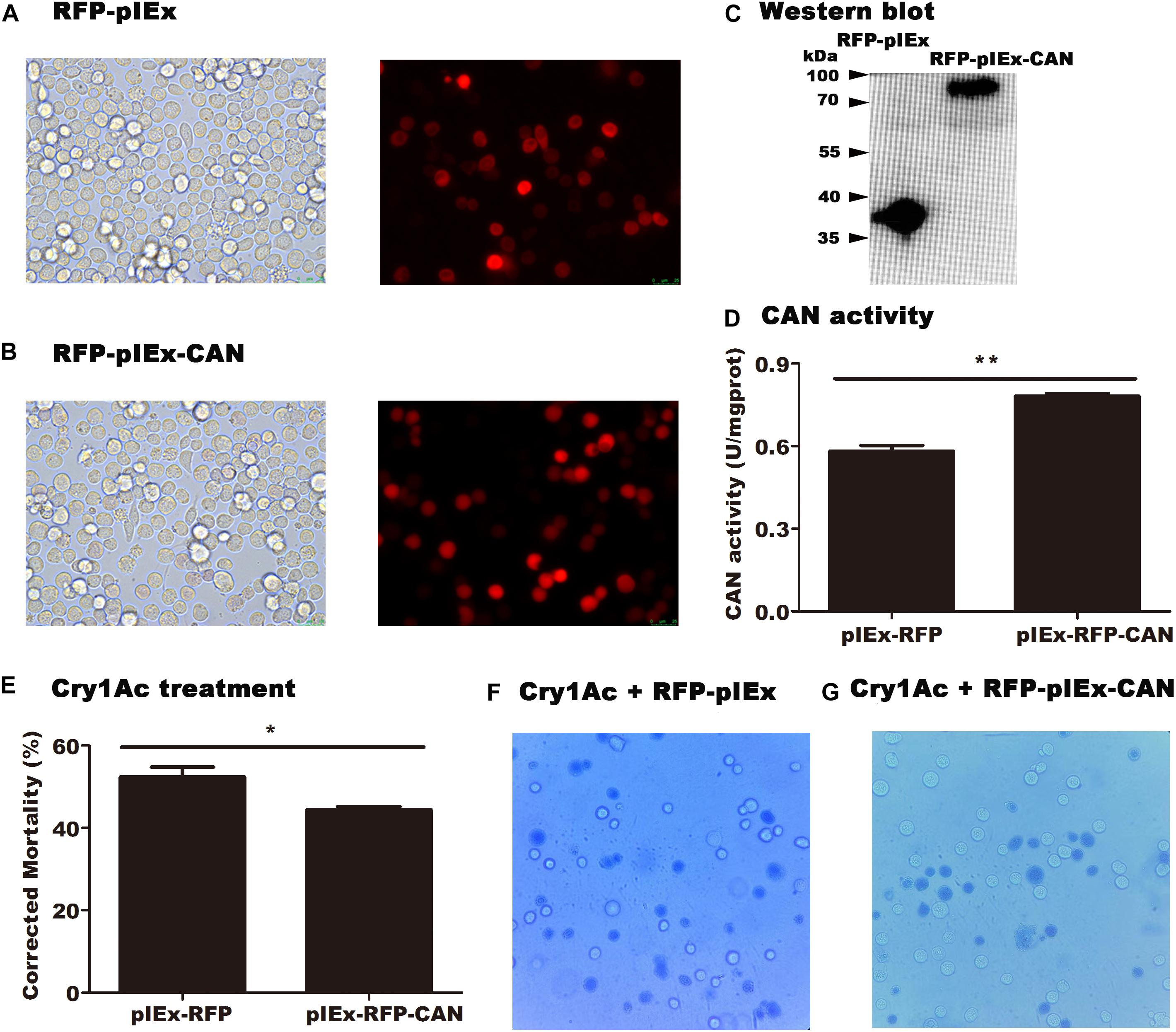
Figure 5. Impact of the heterologously expressed HaCAN on the cytotoxicity of Cry1Ac to midgut cells. (A) The Sf9 cells that transfected GFP-pIEx (empty vector). (B) The Sf9 cells that transfected GFP-pIEx-CAN. (C) Western blot analysis of HaCAN expression in the transfected cells. (D) The determination of HaCAN activity. (E) Cell mortalities caused by 100 μg/mL activated Cry1Ac. (F,G) Photographs are representative of 400x views of the two treatments under an inverted microscope (OPTIKA IM-5). The blue cells represent dead cells, which were stained blue by trypan blue. Each error bar represents the standard error of the mean from three transfection replicates. Asterisks show significant differences in expression levels, enzyme activities, and mortalities between each treatment (P < 0.05, Student’s t-test, DPSSOFT: DPS7.05).
Suppressing HaCAN Increased the Susceptibility of Midgut Cells to Cry1Ac
qRT-PCR detected a significant reduction in the mRNA level of HaCAN in midgut cells transfected with 50 nM HaCAN dsRNA (Figure 6A) compared with that in the control transfected with 50 nM EGFP dsRNA control (P = 0.0017) (Figure 6A).
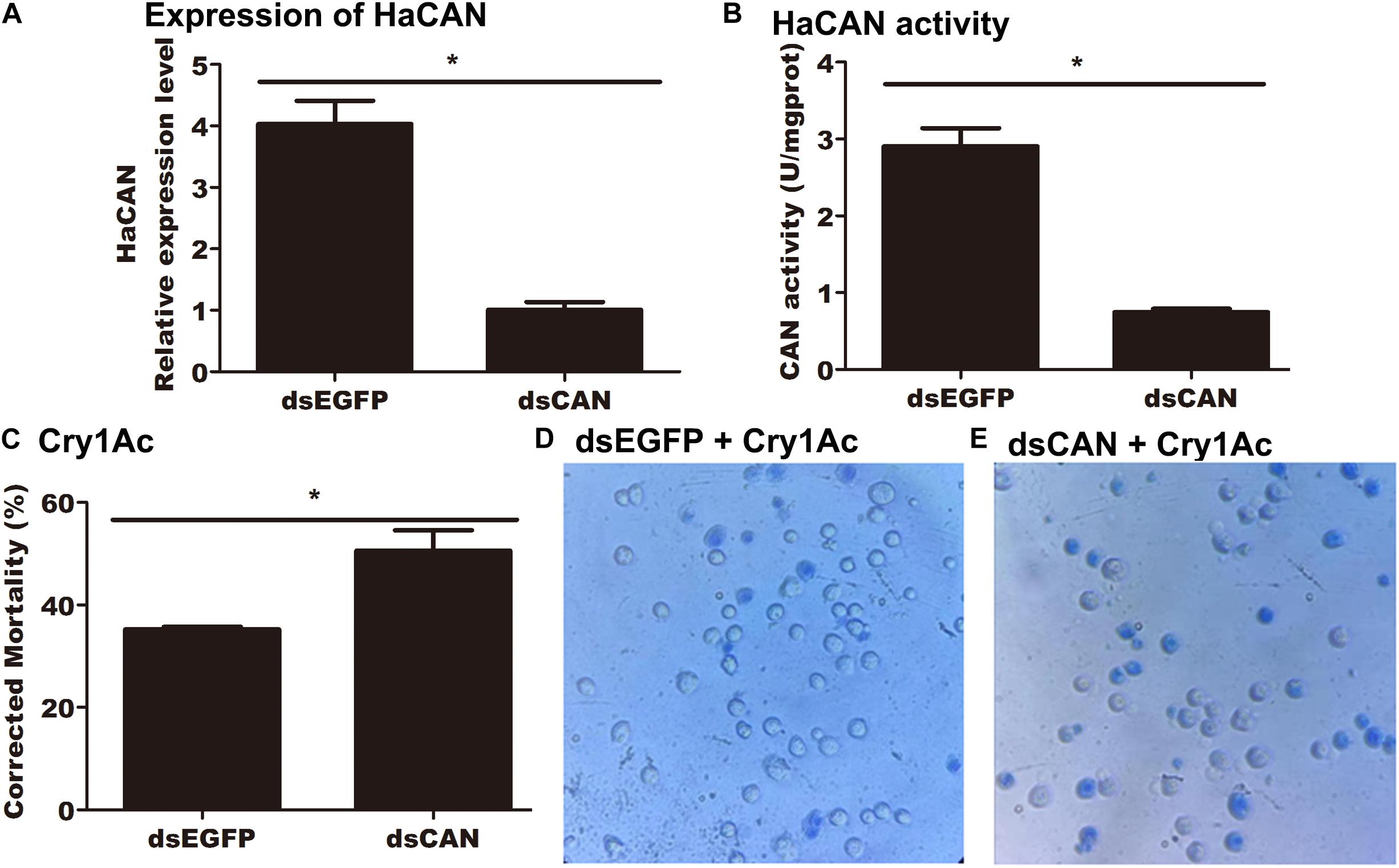
Figure 6. Impact of silencing HaCAN on the cytotoxicity of Cry1Ac to midgut cells. (A) Expression levels of HaCAN in the midgut cells. (B) The determination of HaCAN activity. (C) Cell mortalities caused by 50 μg/mL activated Cry1Ac. (D,E) Photographs are representative of 400x views of the two treatments of the cell lines under an inverted microscope (OPTIKA IM-5). The dead cells were stained blue by trypan blue. Each error bar represents the standard error of the mean from three transfection replicates. Asterisks show significant differences in expression levels, enzyme activities, and mortalities between each treatment (P < 0.05, Student’s t-test, DPSSOFT: DPS7.05).
HaCAN activity was measured after the successful knockdown of HaCAN by RNAi in midgut cells. The result demonstrated that HaCAN activity in the treated cells was significantly reduced in comparison with that in the control cells (P = 0.0008) (Figure 6B).
HaCAN dsRNA-transfected midgut cells were further treated with 50 μg/mL of activated Cry1Ac. The results demonstrated that the cells transfected with HaCAN dsRNA showed significantly higher mortality than those transfected with EGFP dsRNA (increased by 15.39%) (P = 0.0349) (Figure 6C). Photographs also showed that the decrease in HaCAN mRNA in midgut cells resulted in the increase in the cytotoxicity of Cry1Ac to cells (Figures 6D,E).
Discussion
To study the mode of action of Bt, more attention was paid to explore the functional binding receptors with Bt toxin. To date, four main types of receptors, including cadherin, aminopeptidase, alkaline phosphatase, and ATP-binding cassette (ABC) transporters, have been identified (Wei et al., 2019c) on the basis of their capabilities to bind with Bt proteins and their involvement in Bt resistance. However, differentially expressed genes in biochemical pathways, including the hydrolase, digestive, catalytic, immune, and detoxification pathways, may be enriched by genomic or proteomic response or resistance to Bt (Chen et al., 2010; Yuan et al., 2011; Lei et al., 2014; Nanoth Vellichirammal et al., 2015; Xia et al., 2016; Zhang et al., 2017; Wei et al., 2018b). Importantly, these differentially expressed genes such as heat shock proteins (HSPs) and V-ATPase subunits, also participate in the toxicity of Bt to insects (Yuan et al., 2011; Xia et al., 2016; Zhang et al., 2017; Rubio-Infante et al., 2018). In Plutella xylostella, Hsp90 can bind with Cry1A proteins to form a positive loop to protect the Cry protoxin from gut protease degradation (García-Gómez et al., 2019). In the present study, HaCAN was significantly upregulated by Cry1Ac (Figure 1). Investigations on the Cry1Ac-induced genes may help elucidate the mode of action of Bt and avoid the development of resistance.
In addition, this study also confirmed that protein phosphorylation process might participate in Bt toxicity. As reported, the phosphorylated activation of MAPK p38, which acts as a defense response, is involved in the initial response to CF1 cells after intoxication with Cry1A toxins (Portugal et al., 2017). Furthermore, the Cry1Ac protoxin can bind with HSP70 on the cell surface, and thereby participate in Cry1Ac protoxin-induced macrophage activation to reduce Cry1Ac protoxin-induced ERK1 phosphorylation (García-Gómez et al., 2019). A further in-depth study on P. xylostella demonstrated that the altered protein phosphorylation levels of p38, JNK, and ERK in the MAPK signaling cascade may possibly trigger the differential expression of aminopeptidase N and other midgut receptors, thereby leading to insect resistance to Cry1Ac (Guo et al., 2020). These studies hinted that protein kinase in signal transduction is involved in Bt toxicity. In the present study, we found for the first time that a dephosphorylase, HaCAN, affected Cry1Ac insecticide activity (Figures 2, 3, 5, 6).
The findings reported in this paper showed that although HaCAN affected the insecticidal activity of Cry1Ac against cotton bollworm (Figures 3, 5, 6), HaCAN was not a target receptor of Cry1Ac (Figure 4). However, how HaCAN defends the insect against Bt toxin damage remains a question? In yeast and mammal, CAN activates the transcription factor nuclear factor of activated T cells (NFAT) via dephosphorylation, therefore regulating the expression of AMPs and other immune-related genes through the Toll or IMD pathways (Aramburu et al., 2000). In Drosophila, CAN activity can be induced by Gram-negative bacteria (Dijkers and O’Farrell, 2007; Li and Dijkers, 2015), leading to relish activation and finally promoting antimicrobial gene expression through the IMD or Toll pathway (Meng et al., 1999; Dijkers and O’Farrell, 2007; Xu et al., 2012; Li and Dijkers, 2015; Yang et al., 2015). Our previous findings indicated that CAN can bind with relish to regulate the expressions of AMPs (attacin, cecropin D, and gloverin) when the insect injected with Gram-negative bacteria in H. armigera (Wei et al., 2019a). However, a study confirmed that AMPs produced by Trichoplusia ni in response to Bt toxins are not Gram-specific (Tamez-Guerra et al., 2008). Importantly, in insect detoxification reactions to Bt, AMPs are induced in response to Bt challenge and also affect the insecticidal activity of Bt to insect (Ma et al., 2005a,b; Hwang and Kim, 2011). The knockdown of gloverin, an AMP, significantly increases the toxicity of Bt to Spodoptera exigua larvae (Hwang and Kim, 2011). Bt-resistant Galleria mellonella has a more intact midgut than susceptible insects because it secretes antimicrobial factors (such as gloverin and cecropin) into the gut lumen for the external mitigation of Bt (Dubovskiy et al., 2016). Just as it reported, one of the insect defense mechanisms against Bt toxins is the activation of the cellular detoxification reaction, which reduces the amount of Bt endotoxin within the lumen (Rahman et al., 2004, 2006; Ma et al., 2005a,b; Hernández-Martínez et al., 2010). Therefore, we speculated that HaCAN might regulate the expression of AMPs in the insect against Bt toxin damage. However, a more detailed mechanism underlying HaCAN and AMPs defense against Cry1Ac needs to be studied in the future.
But what is important that HaCAN acts as an important gene in the development of cotton bollworm (Wei et al., 2019a). The data obtained in the present study indicated that “pyramids” of RNAi (targeting CAN) and Cry1Ac crop might work well to control cotton bollworm because the low expression or enzyme activity of HaCAN could increase the insecticidal activity of Cry1Ac (Figures 2, 3, 5, 6). In addition, this transgenic strategy might be an ideal combination, because the HaCAN-mediated dephosphorylation of acetyl-coA carboxylase regulates pheromone biosynthesis activating neuropeptide-induced sex pheromone biosynthesis (Du et al., 2017) and mating behavior in adults (Zhao et al., 2018). Considering the important roles of HaCAN in the larvae and adults, it provided a good clue that CAN might be an excellent candidate gene for use in “pyramids” strategy by the simultaneous expression of RNAi (targeting CAN) and Cry1Ac in crop to control cotton bollworm. However, the detailed effects of RNAi (targeting CAN) and Cry1Ac in larvae and adults should be evaluated in the future.
Data Availability Statement
The raw data supporting the conclusions of this article will be made available by the authors, without undue reservation.
Author Contributions
JW, QT, MD, and SA conceived and designed the experiments and wrote the manuscript. XY, SY, SL, JC, XL, and JW conducted the experiments and analyzed the data. All authors read and approved the manuscript.
Funding
This study was supported by the National Natural Science Foundation of China (Grant 31802016 and 31601904), Henan Agricultural Research System (S2014-11-G06), Young Talents Promotion Project in Henan Province (Grant 2019HYTP014), the Youth Innovation Foundation of Henan Agricultural University (Grant KJCX2018A14), Science and Technology Planning Project of Henan Province of China (202102110072), and Young Talents Foundation of Henan Agricultural University.
Conflict of Interest
The authors declare that the research was conducted in the absence of any commercial or financial relationships that could be construed as a potential conflict of interest.
Supplementary Material
The Supplementary Material for this article can be found online at: https://www.frontiersin.org/articles/10.3389/fmicb.2021.634619/full#supplementary-material
Footnotes
References
Abbott, W. S. (1925). A method of computing the effectiveness of an insecticide. Ecol. Entomol. 18, 265–267. doi: 10.1093/jee/18.2.265a
Aramburu, J., Rao, A., and Klee, C. B. (2000). Calcineurin: from structure to function. Curr. Top. Cell. Regul. 36, 237–295. doi: 10.1016/s0070-2137(01)80011-x
Ballesteros-Martinez, C., Mendez-Barbero, N., Montalvo-Yuste, A., Jensen, B. M., Gomez-Cardenosa, A., and Klitfod, L. (2017). Endothelial regulator of calcineurin 1 promotes barrier integrity and modulates histamine-induced barrier dysfunction in anaphylaxis. Front. Immunol. 8:1323. doi: 10.3389/fimmu.2017.01323
Brévault, T., Heuberger, S., Zhang, M., Ellers-Kirk, C., Ni, X., Masson, L., et al. (2013). Potential shortfall of pyramided transgenic cotton for insect resistance management. Proc. Natl. Acad. Sci. U.S.A. 15, 5806–5811. doi: 10.1073/pnas.1216719110
Bueno, O. F., Brandt, E. B., Rothenberg, M. E., and Molkentin, J. D. (2002). Defective T cell development and function in calcineurin A -deficient mice. Proc. Natl. Acad. Sci. U.S.A. 99, 9398–9403. doi: 10.1073/pnas.152665399
Caccia, S., Astarita, F., Barra, E., Di, L. I., Varricchio, P., and Pennacchio, F. (2020). Enhancement of Bacillus thuringiensis toxicity by feeding Spodoptera littoralis larvae with bacteria expressing immune suppressive dsRNA. J. Pest Sci. 93, 303–314. doi: 10.1007/s10340-019-01140-6
Cancino-Rodezno, A., Alexander, C., Villaseñor, R., Pacheco, S., Porta, H., Pauchet, Y., et al. (2010). The mitogen-activated protein kinase p38 is involved in insect defense against Cry toxins from Bacillus thuringiensis. Insect Biochem. Mol. Biol. 40, 58–63. doi: 10.1016/j.ibmb.2009.12.010
Carrière, Y., Crickmore, N., and Tabashnik, B. E. (2015). Optimizing pyramided transgenic Bt crops for sustainable pest management. Nat. Biotechnol. 33, 161–168. doi: 10.1038/nbt.3099
Carrière, Y., Fabrick, J. A., and Tabashnik, B. E. (2016). Can pyramids and seed mixtures delay resistance to Bt crops? Trends Biotechnol. 34, 291–302. doi: 10.1016/j.tibtech.2015.12.011
Chai, L., Tian, Y., Yang, D., Wang, J., and Zhao, X. (2008). Molecular cloning and characterization of a c-type lectin from the cotton bollworm, Helicoverpa armigera. Dev. Comp. Immunol. 32, 71–83. doi: 10.1016/j.dci.2007.04.006
Chen, L., Liang, G., Zhang, J., Wu, K., Guo, Y., and Rector, B. G. (2010). Proteomic analysis of novel Cry1Ac binding proteins in Helicoverpa armigera (Hübner). Arch. Insect Biochem. Physiol. 73, 61–73. doi: 10.1002/arch.20340
Dijkers, P. F., and O’Farrell, P. H. (2007). Drosophila calcineurin promotes induction of innate immune responses. Curr. Biol. 17, 2087–2093. doi: 10.1016/j.cub.2007.11.001
Downes, S., Kriticos, D., Parry, H., Paull, C., Schellhorn, N., and Zalucki, M. P. (2017). A perspective on management of Helicoverpa armigera: transgenic Bt cotton, IPM, and landscapes. Pest Manag. Sci. 73, 485–492. doi: 10.1002/ps.4461
Du, M., Liu, X., Ma, N., Liu, X., Wei, J., Yin, X., et al. (2017). Calcineurin-mediated dephosphorylation of acetyl-coA carboxylase is required for PBAN-induced sex pheromone biosynthesis in Helicoverpa armigera. Mol. Cell. Proteomics. 16, 2138–2152. doi: 10.1074/mcp.ra117.000065
Du, M., Zhang, S., Zhu, B., Yin, X., and An, S. (2012). Identification of adiacylglycerol acyltransferase 2 gene involved in pheromone biosynthesis activating neuropeptide stimulated pheromone production in Bombyx mori. J. Insect Physiol. 58, 699–703. doi: 10.1016/j.jinsphys.2012.02.002
Dubovskiy, I. M., Grizanova, E. V., Whitten, M. M. A., Mukherjee, K., Greig, C., Alikina, T., et al. (2016). Immuno-physiological adaptations confer wax moth Galleria mellonella resistance to Bacillus thuringiensis. Virulence 7, 860–870. doi: 10.1080/21505594.2016.1164367
Furman, J. L., and Norris, C. M. (2014). Calcineurin and glial signaling: neuroinflammation and beyond. J. Neuroinflamm. 11:158.
García-Gómez, B. I., Cano, S. N., Zagal, E. E., Dantán-Gonzalez, E., Bravo, A., and Soberón, M. (2019). Insect Hsp90 chaperone assists Bacillus thuringiensis Cry toxicity by enhancing protoxin binding to the receptor and by protecting protoxin from gut protease degradation. mBio 10:e02775-19.
Goodman, C. L., Wang, A. A., Nabli, H., McIntosh, A. H., Wittmeyer, J. L., and Grasela, J. J. (2004). Development and partial characterization of Heliothine cell lines from embryonic and differentiated tissues. In vitro Cell. Dev. Biol. Anim. 40, 89–94. doi: 10.1290/1543-706x(2004)040<0089:dapcoh>2.0.co;2
Guo, Z., Kang, S., Sun, D., Gong, L., Zhou, J., Qin, J., et al. (2020). MAPK-dependent hormonal signaling plasticity contributes to overcoming Bacillus thuringiensis toxin action in an insect host. Nat. Commun. 11:3003.
Hernández-Martínez, P. L., Naseri, B., Navarro-Cerrillo, G., Escriche, B., Ferré, J., and Herrero, S. (2010). Increase in midgut microbiota load induces an apparent immune priming and increases tolerance to Bacillus thuringiensis. Environ. Microbiol. 12, 2730–2737.
Huffman, D. L., Abrami, L., Sasik, R., Corbeil, J., van der Goot, F. G., and Aroian, R. V. (2004). Mitogen-activated protein kinase pathways defend against bacterial pore-forming toxins. Proc. Natl. Acad. Sci. U.S.A. 101, 10995–11000. doi: 10.1073/pnas.0404073101
Hutchison, W. D., Burkness, E. C., Mitchell, P. D., Moon, R. D., Leslie, T. W., and Fleischer, S. J. (2010). Area wide suppression of European corn borer with Bt maize reaps savings to non-Bt maize growers. Science 330, 222–225. doi: 10.1126/science.1190242
Hwang, J., and Kim, Y. (2011). RNA interference of an antimicrobial peptide, gloverin, of the beet armyworm, Spodoptera exigua, enhances susceptibility to Bacillus thuringiensis. J. Invertebr. Pathol. 108, 194–200. doi: 10.1016/j.jip.2011.09.003
ISAAA (2018). Global Status of Commercialized Biotech/GM Crops in 2018: Biotech Crops Continue to Help Meet the Challenges of Increased Population and Climate Change. ISAAA Brief No. 54. Ithaca, NY: ISAAA.
Kang, Y., Kusler, B., Otsuka, M., Hughes, M., Suzuki, N., Suzuki, S., et al. (2007). Calcineurin negatively regulates TLR-mediated activation pathways. J. Immunol. 179, 4598–4607. doi: 10.4049/jimmunol.179.7.4598
Lei, Y., Zhu, X., Xie, W., Wu, Q., Wang, S., Guo, Z., et al. (2014). Midgut transcriptome response to a Cry toxin in the diamondback moth, Plutella xylostella (Lepidoptera: Plutellidae). Gene 533, 180–187. doi: 10.1016/j.gene.2013.09.091
Li, Y., and Dijkers, P. F. (2015). Specific calcineurin isoforms are involved in Drosophila toll immune signaling. J. Immunol. 194, 168–176. doi: 10.4049/jimmunol.1401080
Li, Z., Ni, C., Xia, C., Jaw, J., Wang, Y., Cao, Y., et al. (2017). Calcineurin/nuclear factor−κB signaling mediates isoflurane−induced hippocampal neuroinflammation and subsequent cognitive impairment in aged rats. Mol. Med. Rep. 15, 201–209. doi: 10.3892/mmr.2016.5967
Liu, S., Wang, M., and Li, X. (2015). Over expression of tyrosine hydroxylase and dopa decarboxylase associated with pupal melanization in Spodoptera exigua. Sci. Rep. 5:11273.
Lu, Y., Wu, K., Jiang, Y., Guo, Y., and Desneux, N. (2012). Widespread adoption of Bt cotton and insecticide decrease promotes biocontrol services. Nature 487, 362–365. doi: 10.1038/nature11153
Luo, H., and Dearolf, C. R. (2010). The JAK/STAT pathway and Drosophila development. Bioessays 23, 1138–1147.
Ma, G., Roberts, H., Sarjan, M., Featherstone, N., Lahnstein, J., Akhurst, R., et al. (2005a). Is the mature endotoxin Cry1Ac from Bacillus thuringiensis inactivated by a coagulation reaction in the gut lumen of resistant Helicoverpa armigera larvae? Insect Biochem. Mol. Biol. 35, 729–739. doi: 10.1016/j.ibmb.2005.02.011
Ma, G., Sarjan, M., Preston, C., Asgari, S., and Schmidt, O. (2005b). Mechanisms of inducible resistance against Bacillus thuringiensis endotoxins in invertebrates. Insect Sci. 12, 319–330. doi: 10.1111/j.1005-295x.2005.00039.x
Mamta, B., and Rajam, M. V. (2017). RNAi technology: a new platform for crop pest control. Physiol. Mol. Biol. Plants 23, 487–501. doi: 10.1007/s12298-017-0443-x
Mendelsohn, M., Kough, J., Vaituzis, Z., and Matthews, K. (2003). Are Bt crops safe? Nat. Biotechnol. 21, 1003–1009. doi: 10.1038/nbt0903-1003
Meng, X., Khanuja, B. S., and Ip, Y. T. (1999). Toll receptor-mediated Drosophila immune response requires Dif, an NF- kappaB factor. Gene Dev. 13, 792–797. doi: 10.1101/gad.13.7.792
Möckli, N., Deplazes, A., Hassa, P. O., Zhang, Z., Peter, M., Hottiger, M. O., et al. (2007). Yeast split-ubiquitin-based cytosolic screening system to detect interactions between transcriptionally active proteins. Biotechniques 42, 725–730. doi: 10.2144/000112455
Nanoth Vellichirammal, N., Wang, H., Eyun, S., Moriyama, E. N., Coates, B. S., Miller, N. J., et al. (2015). Transcriptional analysis of susceptible and resistant European corn borer strains and their response to Cry1F protoxin. BMC Genomics 16:558. doi: 10.1186/s12864-015-1751-6
Naranjo, S. E. (2011). Impacts of Bt transgenic cotton on integrated pest management. J. Agric. Food Chem. 59, 5842–5851.
Ni, M., Ma, W., Wang, X., Gao, M., Dai, Y., Wei, X., et al. (2017). Next-generation transgenic cotton: pyramiding RNAi and Bt counters insect resistance. Plant Biotechnol. J. 15, 1204–1213. doi: 10.1111/pbi.12709
Oppert, B., Dowd, S. E., Bouffard, P., Li, L., Conesa, A., Lorenzen, M. D., et al. (2012). Transcriptome profiling of the intoxication response of Tenebrio molitor larvae to Bacillus thuringiensis Cry3Aa protoxin. PLoS One 7:e34624. doi: 10.1371/journal.pone.0034624
Portugal, L., Muñóz-Garay, C., Dl, M. D. C., Soberón, M., and Bravo, A. (2017). Toxicity of cry1a toxins from Bacillus thuringiensis to CF1 cells does not involve activation of adenylate cyclase/pka signaling pathway. Insect Biochem. Mol. Biol. 80, 21–31. doi: 10.1016/j.ibmb.2016.11.004
Qiu, L., Sun, Y., Jiang, Z., Yang, P., Liu, H., Zhou, H., et al. (2019). The midgut V-ATPase subunit A gene is associated with toxicity to crystal 2Aa and crystal 1Ca-expressing transgenic rice in Chilo suppressalis. Insect Mol. Biol. 28, 520–527.
Rahman, M. M., Ma, G., Roberts, H. L., and Schmidt, O. (2006). Cell-free immune reactions in insects. J. Insect Physiol. 52, 754–762. doi: 10.1016/j.jinsphys.2006.04.003
Rahman, M. M., Roberts, H. L., Sarjan, M., Asgari, S., and Schmidt, O. (2004). Induction and transmission of Bacillus thuringiensis tolerance in the flour moth Ephestia kuehniella. Proc. Natl. Acad. Sci. U.S.A. 101, 2696–2699. doi: 10.1073/pnas.0306669101
Rubio-Infante, N., Ilhuicatzi-Alvarado, D., Torres-Martínez, M., Reyes-Grajeda, J. P., Nava-Acosta, R., González-González, E., et al. (2018). The macrophage activation induced by Bacillus thuringiensis Cry1Ac protoxin involves ERK1/2 and p38 pathways and the interaction with cell-surface-HSP70. J. Cell. Biochem. 119, 580–598. doi: 10.1002/jcb.26216
Snider, J., Kittanakom, S., Curak, J., and Stagljar, I. (2010). Split-ubiquitin based membrane yeast two-hybrid (MYTH) system: a powerful tool for identifying protein-protein interactions. J. Vis. Exp. 36:1698.
Stagljar, I., Korostensky, C., Johnsson, N., and te Heesen, S. (1998). A genetic system based on split-ubiquitin for the analysis of interactions between membrane proteins in vivo. Proc. Natl. Acad. Sci. U.S.A. 95, 5187–5192. doi: 10.1073/pnas.95.9.5187
Tabashnik, B. E., and Carrière, Y. (2017). Surge in insect resistance to transgenic crops and prospects for sustainability. Nat. Biotechnol. 35, 926–935. doi: 10.1038/nbt.3974
Tabashnik, B. E., Fabrick, J. A., Unnithan, G. C., Yelich, A. J., Masson, L., Zhang, J., et al. (2013). Efficacy of genetically modified Bt toxins alone and in combinations against pink bollworm resistant to Cry1Ac and Cry2Ab. PLoS One 8:e80496. doi: 10.1371/journal.pone.0080496
Tabashnik, B. E., Sisterson, M. S., Ellsworth, P. C., Dennehy, T. J., Antilla, L., Liesner, L., et al. (2010). Suppressing resistance to Bt cotton with sterile insect releases. Nat. Biotechnol. 28, 1304–1307. doi: 10.1038/nbt.1704
Tamez-Guerra, P., Valadez-Lira, J. A., Alcocer-González, J. M., Oppert, B., Gomez-Flores, R., Tamez-Guerra, R., et al. (2008). Detection of genes encoding antimicrobial peptides in Mexican strains of Trichoplusia ni (Hübner) exposed to Bacillus thuringiensis. J. Invertebr. Pathol. 98, 218–227. doi: 10.1016/j.jip.2008.02.008
Wang, Q., Liu, Y., He, H., Zhao, X., and Wang, J. (2010). Immune responses of Helicoverpa armigera to different kinds of pathogens. BMC Immunol. 11:9. doi: 10.1186/1471-2172-11-9
Wei, J., Guo, Y., Liang, G., Wu, K., Zhang, J., Tabashnik, B. E., et al. (2015). Cross-resistance and interactions between Bt toxins Cry1Ac and Cry2Ab against the cotton bollworm. Sci. Rep. 5:7714.
Wei, J., Li, L., Yao, S., Zhou, S., Liu, X., Du, M., et al. (2019a). Calcineurin-modulated antimicrobial peptide expression is required for the development of Helicoverpa armigera. Front. Physiol. 10:1312. doi: 10.3389/fphys.2019.01312
Wei, J., Liang, G., Wu, K., Gu, S., Guo, Y., Ni, X., et al. (2018a). Cytotoxicity and binding profiles of activated Cry1Ac and Cry2Ab to three insect cell lines. Insect Sci. 25, 655–666. doi: 10.1111/1744-7917.12451
Wei, J., Yang, S., Chen, L., Liu, X., Du, M., An, S., et al. (2018b). Transcriptomic responses to different Cry1Ac selection stresses in Helicoverpa armigera. Front. Physiol. 9:1653. doi: 10.3389/fphys.2018.01653
Wei, J., Zhang, M., Liang, G., and Li, X. (2019b). Alkaline phosphatase2 is a functional receptor of Cry1Ac but not Cry2Ab in Helicoverpa zea. Insect Mol. Biol. 28, 372–379. doi: 10.1111/imb.12556
Wei, J., Zhang, M., Liang, G., Wu, K., Guo, Y., Ni, X., et al. (2016). APN1 is a functional receptor of Cry1Ac but not Cry2Ab in Helicoverpa zea. Sci. Rep. 6:19179.
Wei, J., Zhang, Y., and An, S. (2019c). The progress in insect cross-resistance among Bacillus thuringiensis toxins. Arch. Insect Biochem. Physiol. 12:e21547.
Xia, J., Guo, Z., Yang, Z., Zhu, X., Kang, S., Yang, X., et al. (2016). Proteomics-based identification of midgut proteins correlated with Cry1Ac resistance in Plutella xylostella. Pestic. Biochem. Physiol. 132, 108–117. doi: 10.1016/j.pestbp.2016.01.002
Xu, X., Zhong, X., Yi, H., and Yu, X. Q. (2012). Manduca sexta gloverin binds microbial components and is active against Bacteria and Fungi. Dev. Comp. Immunol. 38, 275–284. doi: 10.1016/j.dci.2012.06.012
Yang, J., Wang, X., Tang, S., Shen, Z., and Wu, J. (2015). Peptidoglycan recognition protein S2 from silkworm integument: characterization, microbe-Induced expression, and involvement in the immune-deficiency pathway. J. Insect Sci. 15, 20. doi: 10.1093/jisesa/iev007
Yu, Y., Li, Y., and Zhang, Y. (2015). Screening of APP interaction proteins by DUAL membrane yeast two-hybrid system. Int. J. Exp. Pathol. 8, 2802–2808.
Yuan, C., Ding, X., Xia, L., Yin, J., Huang, S., and Huang, F. (2011). Proteomic analysis of BBMV in Helicoverpa armigera midgut with and without Cry1Ac toxin treatment. Biocontrol. Sci. Technol. 21, 139–151. doi: 10.1080/09583157.2010.527318
Zhang, T., Coates, B. S., Wang, Y., Wang, Y., Bai, S., Wang, Z., et al. (2017). Down-regulation of aminopeptidase N and ABC transporter subfamily G transcripts in Cry1Ab and Cry1Ac resistant Asian corn borer, Ostrinia furnacalis (Lepidoptera: Crambidae). Int. J. Biol. Sci. 13, 835–851. doi: 10.7150/ijbs.18868
Zhao, W., Li, L., Zhang, Y., Liu, X., Wei, J., Xie, Y., et al. (2018). Calcineurin is required for male sex pheromone biosynthesis and female acceptance. Insect Mol. Biol. 27, 373–382. doi: 10.1111/imb.12379
Keywords: Helicoverpa armigera, calcineurin, Cry1Ac, membrane yeast two-hybrid, cell toxicity
Citation: Wei J, Yao X, Yang S, Liu S, Zhou S, Cen J, Liu X, Du M, Tang Q and An S (2021) Suppression of Calcineurin Enhances the Toxicity of Cry1Ac to Helicoverpa armigera. Front. Microbiol. 12:634619. doi: 10.3389/fmicb.2021.634619
Received: 28 November 2020; Accepted: 25 January 2021;
Published: 11 February 2021.
Edited by:
Baltasar Escriche, University of Valencia, SpainReviewed by:
Lin Jin, Nanjing Agricultural University, ChinaGemei Liang, Chinese Academy of Agricultural Sciences, China
Copyright © 2021 Wei, Yao, Yang, Liu, Zhou, Cen, Liu, Du, Tang and An. This is an open-access article distributed under the terms of the Creative Commons Attribution License (CC BY). The use, distribution or reproduction in other forums is permitted, provided the original author(s) and the copyright owner(s) are credited and that the original publication in this journal is cited, in accordance with accepted academic practice. No use, distribution or reproduction is permitted which does not comply with these terms.
*Correspondence: Mengfang Du, ZHVtZW5nZmFuZ0AxNjMuY29t; Qingbo Tang, cWluZ2JvdGFuZ0AxMjYuY29t; Shiheng An, YW5zaGloZW5nQGFsaXl1bi5jb20=