- 1IFREMER-Phycotoxins Laboratory, Nantes, France
- 2IFREMER-DYNECO Pelagos, Plouzané, France
- 3Sorbonne Université, CNRS, UMR 7144 Adaptation et Diversité en Milieu Marin, Station Biologique de Roscoff, Roscoff, France
Paralytic shellfish poisoning (PSP) is a human foodborne syndrome caused by the consumption of shellfish that accumulate paralytic shellfish toxins (PSTs, saxitoxin group). In PST-producing dinoflagellates such as Alexandrium spp., toxin synthesis is encoded in the nuclear genome via a gene cluster (sxt). Toxin production is supposedly associated with the presence of a 4th domain in the sxtA gene (sxtA4), one of the core genes of the PST gene cluster. It is postulated that gene expression in dinoflagellates is partially constitutive, with both transcriptional and post-transcriptional processes potentially co-occurring. Therefore, gene structure and expression mode are two important features to explore in order to fully understand toxin production processes in dinoflagellates. In this study, we determined the intracellular toxin contents of twenty European Alexandrium minutum and Alexandrium pacificum strains that we compared with their genome size and sxtA4 gene copy numbers. We observed a significant correlation between the sxtA4 gene copy number and toxin content, as well as a moderate positive correlation between the sxtA4 gene copy number and genome size. The 18 toxic strains had several sxtA4 gene copies (9–187), whereas only one copy was found in the two observed non-toxin producing strains. Exploration of allelic frequencies and expression of sxtA4 mRNA in 11 A. minutum strains showed both a differential expression and specific allelic forms in the non-toxic strains compared with the toxic ones. Also, the toxic strains exhibited a polymorphic sxtA4 mRNA sequence between strains and between gene copies within strains. Finally, our study supported the hypothesis of a genetic determinism of toxin synthesis (i.e., the existence of several genetic isoforms of the sxtA4 gene and their copy numbers), and was also consistent with the hypothesis that constitutive gene expression and moderation by transcriptional and post-transcriptional regulation mechanisms are the cause of the observed variability in the production of toxins by A. minutum.
Introduction
The paralytic shellfish poisoning (PSP) syndrome is caused by the consumption of shellfish contaminated by toxins of the saxitoxin group (STX-group), also named paralytic shellfish toxins (PSTs) (Bricelj and Shumway, 1998). In mammals, these toxins act as blockers of voltage-dependent Na+ channels, inhibiting the transmission of neuronal signals (Cusick and Sayler, 2013). The first symptoms are tingling sensations in the lips, tongue and throat, and numbness of the face, which may progress in the most severe and acute cases of intoxication to paralysis, respiratory arrest or cardiovascular shock leading to death (Zhang et al., 2013; Hurley et al., 2014).
Among the 16 cyanobacterial and dinoflagellate genera PST producers (Yunes et al., 2009; Wiese et al., 2010; Borges et al., 2015; Savela et al., 2015; Caruana and Amzil, 2018), two species (Alexandrium minutum and Alexandrium pacificum) are known to form recurrent toxic blooms in shallow confined coastal waters along the French coasts (Giacobbe et al., 1996; Abdenadher et al., 2012; Anderson et al., 2012; Dhib et al., 2013; Guallar et al., 2017; Condie et al., 2019). STXs account for 57 molecules that include (Wiese et al., 2010) non-sulfated (NeoSTX, STX), mono-sulfated (GTX1/4, GTX2/3, B1, B2), di-sulfated (C1, C2, C4) or decarbamoylated (dcGTX2/3 and dcSTX) derivatives (Masselin et al., 2001; Touzet et al., 2007b; Masseret et al., 2009; Laabir et al., 2011, 2013; Hii et al., 2016). Highly toxic strains are correlated with the presence of low levels of sulfonate derivatives, i.e., STX, NeoSTX, and GTX1/4 (Botana et al., 2017). In addition, the toxin content per cell results from physiological (Anderson et al., 1990), environmental (such as abiotic and biotic factors (Hwang and Lu, 2000; Grzebyk et al., 2003; Laabir et al., 2011, 2013; Aguilera-Belmonte et al., 2013) and genetic factors (Kellmann et al., 2010; Stüken et al., 2015).
The candidate gene cluster for the PST biosynthetic pathway (sxt) was first identified in the 1980s from a cyanobacterial strain that produces PSTs, Cylindrospermopsis raciborskii T3 (Kellmann et al., 2008b). Thirty catalytic functions correspond to twenty-six proteins clustered within a single 35 kb genomic region (Kellmann et al., 2008a,b). Eight of these proteins, encoded by the sxtA, sxtB, sxtD, sxtG, sxtS, sxtH/T, sxtU, and sxtI genes, are directly involved in PST synthesis in cyanobacteria leading to the production of certain STX derivatives (dcSTX and/or STX) (Kellmann et al., 2008b; Moustafa et al., 2009; Hackett et al., 2013). Then sxtI allows the conversion from dcSTX to STX, while other proteins coded by the sxtL, sxtN, sxtO, sxtR, sxtX, sxtW, sxtZ, sxtPER, and sxtACT genes are involved in the synthesis of other PST analogs and in the transport of the toxin (Zhang et al., 2014, 2017; Wang et al., 2015; Verma et al., 2019). Although cyanobacteria and dinoflagellates are not closely related phylogenetically (prokaryotic vs. eukaryotic organisms), homologs of the cyanobacteria sxt gene cluster have been found in toxic dinoflagellates (Stüken et al., 2011; Hackett et al., 2013; Verma et al., 2019).
The sxtA gene is involved in the first step of toxin synthesis. However, whereas one unique and long mRNA version of this gene exists in cyanobacteria, two isoforms potentially in multiple copies are detected in the toxic dinoflagellates A. fundyense, A. minutum, A. catenella, and/or A. pacificum. These isoforms consist of one “short” isoform encoding three catalytic domains (sxtA1-3) (excluding a 4th domain that has no homolog in existing databases [amino acids 822–976, (Le Gac et al., 2016)] and a “long” one encoding four catalytic domains (sxtA1-4) (Stüken et al., 2011; Wang et al., 2020a). Based on the presence of the sxtA4 domain, it is thought that the two isoforms are also present in A. affine, A. australiense, A. ostenfeldii, A. tamarense and the toxic dinoflagellates G. catenatum and P. bahamense (Stüken et al., 2011; Suikkanen et al., 2013; Murray et al., 2015; Wang et al., 2020a). The sxtA4 domain is also found in the transcriptome of two non-toxic dinoflagellates, Prorocentrum micans and Cochlodinium polykrikoides, but is composed of a different sequence than those found in toxic Alexandrium species (Wang et al., 2020a,b).
Early on, it was suspected that the “long” mRNA isoform bearing the specific 4th sxtA domain (or sxtA4) was essential for toxin production, due to their under-expression in the non-toxic mutant A. pacificum ACHK-NT (Zhang et al., 2014; Verma et al., 2019). Suikkanen et al. (2013) additionally observed that the saxitoxin-producing strain of A. ostenfeldii contained sxtA4 whereas the spirolide-producing strain of A. ostenfeldii did not. Furthermore, it has been shown that the presence of the sxtA4 gene is essential for toxin production, and that the sxtA4 copy numbers (CPNs) was strongly correlated with the toxin content in 15 strains of A. minutum. However, no correlation was found between the sxtA4 CPNs and PSP content in three strains of A. ostenfeldii (Savela et al., 2016).
In field surveys, sxtA4 qPCR assays are found to be effective for identifying PST-producing dinoflagellates from mixed samples (Murray et al., 2011, 2019; Gao et al., 2015; Penna et al., 2015; Ruvindy et al., 2018). Indeed, the presence of sxtA4 seems to be a putative proxy to reveal the presence of toxic cells in blooms since there is a strong correlation between the detection of sxtA4 and the presence of toxins in A. ostenfeldii and A. pacificum (Murray et al., 2011; Savela et al., 2016). However, it has been reported that several strains (one strain of A. tamarense, two strains of A. australiense and one mutant of A. pacificum) possess sxtA4 without producing toxin (Murray et al., 2011, 2015; Stüken et al., 2011; Zhang et al., 2014), challenging the use of the sxtA4 copy number as a proxy for the presence or level of toxin production.
The relationship between copy number of the sxtA4 domain and toxin content remains unclear and, so far, the available results suggest that the determination of toxin production is complex, and involves constitutive gene expression associated with processes regulating the level of toxin production (Yang et al., 2010; Wiese et al., 2014; Zhang et al., 2017; Akbar et al., 2018). The use of several gene expression modes, including both transcriptional and post-transcriptional processes, are suspected in dinoflagellates (Hackett et al., 2013; McLean, 2013; Roy and Morse, 2013). Although this sxtA4 domain is highly conserved between cyanobacteria (i.e., Cylindrospermopsis raciborskii, Anabaena circinalis, Lyngbya wollei, Aphanizomenon flos-aquae) and PST-producing dinoflagellates, multiple variants and highly variable gene copy numbers (CPN) of this 4th sxtA domain have been reported both between and within dinoflagellate species, raising questions regarding the universality of a gene regulation mode (Murray et al., 2011; Stüken et al., 2011, 2015; Savela et al., 2016; Mendoza-Flores et al., 2018; Verma et al., 2019).
In this study, we investigate how genetic determinism influences PST production in two Alexandrium species: (i) by describing the intra- and inter-specific variability of the Alexandrium strains in terms of either their genetic characteristics (gene CPN, expression, genetic variants) or their toxin production (content and profile) between twenty European strains of A. pacificum and A. minutum, (ii) by comparing the genome size and the 4th sxtA domain copy number to the toxin production and, (iii) based on the transcriptomic data generated for A. minutum, we additionally compare this toxin production to the sxtA4 gene expression level and allelic frequencies in eleven strains. The goal of our study is to provide a better understanding of the toxin production in Alexandrium, in particular to clarify whether the presence of sxtA4 is a criterion for PST production in Alexandrium and whether there is a relationship between sxtA4 CPN and toxin content.
Materials and Methods
Strains and Culture Conditions
Monoclonal and xenic cultures of A. minutum and A. pacificum were established from European coast samples (Thau lagoon in French Mediterranean Sea, Atlantic Ocean and English Channel) (Table 1), and were identified by morphological criteria and by partial rRNA sequences ((ITS1, 5.8S, ITS2) for A. minutum (Dia et al., 2014; Le Gac et al., 2016) and the large subunit (LSU) for A. pacificum (Supplementary Table 1). Batch cultures were maintained in a autoclaved, filtered natural Mediterranean seawater (at a salinity of 38) and English Channel seawater (at a salinity of 35) enriched with L1 nutrients without the addition of silica (Guillard and Hargraves, 1993). They were exposed to a 12:12-h light: dark cycle and a photon flux density of 100 μmol photons m–2 s–1 (cool-white fluorescent light; Osram, Munich, Germany) at 18°C ± 1°C. The origins of the strains are listed in Table 1. Counts were performed using a particle counter (Beckman-Coulter Multisizer 3, Fullerton, CA, United States).
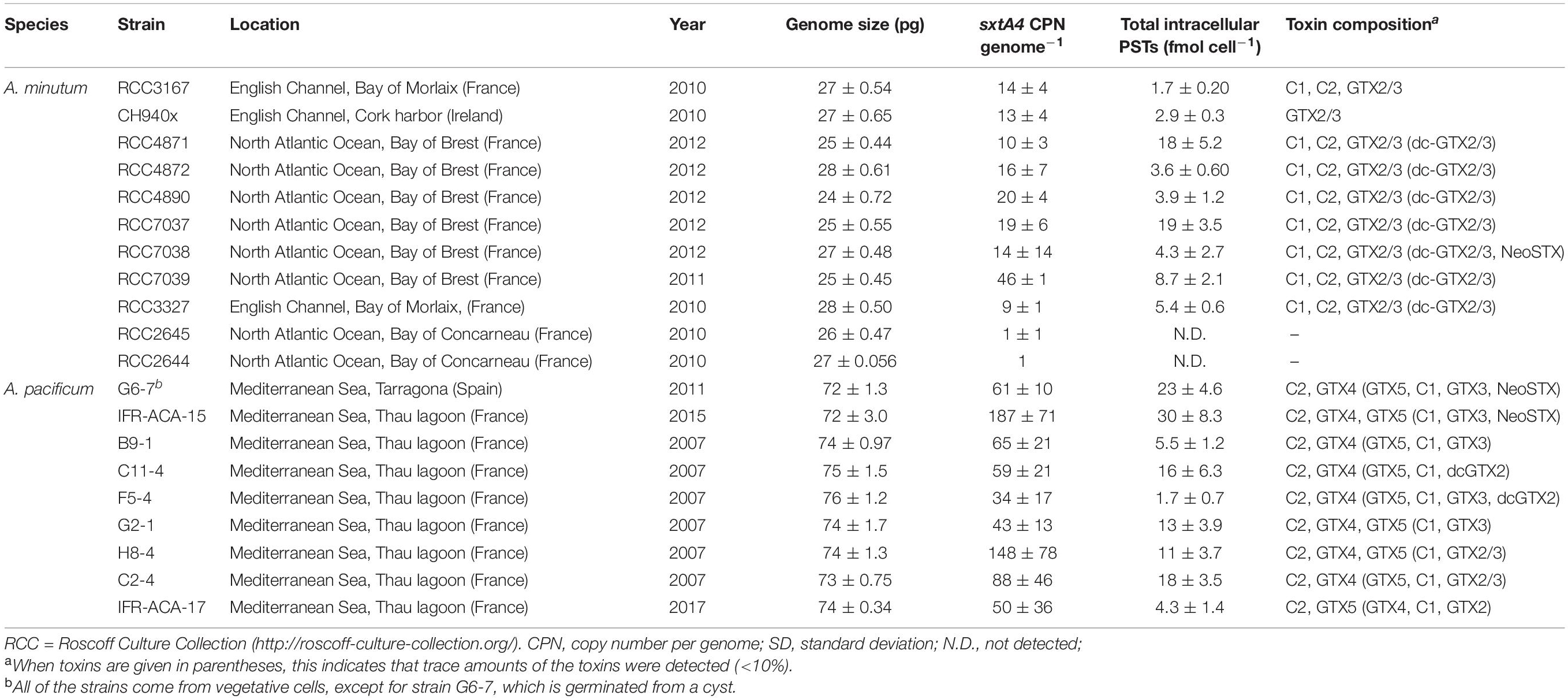
Table 1. List of the A. minutum and A. pacificum strains used, with their genome size, sxtA4 CPN per genome and toxin composition content (means ± SD, n = 3).
Batch Culture of Alexandrium Strains
Batch cultures in the late exponential growth phase were prepared in order to limit CPN variations over the growth phases (Stüken et al., 2011). Triplicate batch cultures of the Alexandrium strains were inoculated at an initial density of 5,000 cells mL–1 in 2 L Erlenmeyer flasks and incubated for 9–10 days for A. minutum and 6 days for A. pacificum to reach the end of the exponential growth phase (Supplementary Figure 1). At this time, samples were taken to measure the PSTs, CPN, and cell density.
PST Analysis by Liquid Chromatography/Fluorescence Detection (LC/FLD)
Centrifugation was performed at 3,000 g for 8 min at 4°C in order to harvest 1.7 × 106± 5.4 × 105 A. minutum cells and 7.8 × 105± 2.4 × 104 A. pacificum cells. The pellets were extracted using the protocol described by Caruana et al. (2020). The toxin analyses were performed by LC/FLD based on the method given by Van De Riet et al. (2009) with slight modifications. Only the toxins with available standards were targeted. The mono-sulfated (GTX1/GTX4, GTX2/GTX3, B1, B2), decarbamoylated (dcGTX2/dcGTX3) and dcSTX, NeoSTX and STX toxins were separated using a reverse phase chromatography column (Zorbax Bonus RP, 3.5 μm, 4.6 × 150 mm) with a flow rate of 0.8 mL min–1. The di-sulfated toxins (C1, C2) were separated using a reverse phase chromatography column (BetaBasic 8, 5 μm, 4.6 × 250 mm) with a flow rate of 0.8 mL min–1. The pH and/or column temperature was/were optimized to separate dc-GTX2/dcGTX3, GTX5 (B1) and C1/C2. The toxin concentrations were quantified using a 6-point calibration curve of the reference standards from CNRC (Halifax, NS, Canada). Limits of detection (LOD) and quantification (LOQ) were provided in Supplementary Table 2 and strains showing no detectable traces of searched toxins were considered non-producing PST strains or non-toxic.
gDNA Isolation and Quantification
A polycarbonate 12-μm pore-size filter (Nucleopore track-etched membrane, Whatman, Fisher Scientific, Pittsburgh, PA, United States) was used to aseptically filter 1.7 × 106± 5.4 × 105 A. minutum cells and 7.8 × 105± 2.4 × 104 A. pacificum cells. The cells were then rinsed three times with sterile L1 medium. Total gDNA was isolated from the 12 μm filter using the Nucleospin Plant II kit (Macherey Nagel, Hoerdt, France), according to the manufacturer’s instructions with minor modifications. These modifications involved resuspending each filter in 1 mL of PL1 buffer, using a vortex for 3 min and incubating for 60 min at 65°C with 25 μL RNase A. The silica membrane was washed three times with PW1 and PW2 and then dried 5 min at 65°C. Sample elution was performed twice after an incubation with 25 μL of PE buffer (5 min at 65°C). The gDNA was quantified using a NanoDrop® spectrophotometer (A260/A280, NanoDrop® Spectrophotometer ND-1000, Labtech International Ltd., Ringmer, United Kingdom). The quality of the gDNA was examined on a 1% agarose gel (25 min, 100V) stained with GelRedTM (Biotium, United States).
sxtA4 Genomic Amplification and Cloning
Genomic amplifications were performed using 10 ng of isolated gDNA, 300 nM of primers (Supplementary Table 3) and 1× GoTaq® Green Master Mix (Promega, Madison, WI, United States). After denaturation at 95°C for 2 min, amplification consisted of 35 cycles for 30 s at 95°C, 45 s at 67°C and 50 s at 72°C. A final step of elongation was done at 72°C for 5 min. The amplified DNA fragments were visualized on 0.8% agarose (25 min, 100V) stained with GelRedTM, cut out and cleaned using the PCR Clean-up Gel Extraction kit (Macherey Nagel, Hoerdt, France) according to the manufacturer’s instructions. After a quantification and quality control step, the PCR products were cloned into a pGEM-T Easy Vector (Promega, Madison, WI, United States) according to the manufacturer’s instructions and transfected into JM109 E. coli competent cells (Promega, Madison, WI, United States). Following blue/white screening, recombinant plasmid DNA was extracted using the Plasmid DNA purification kit (Macherey Nagel, Hoerdt, France) according to the manufacturer’s instructions and then sequenced (Eurofins MWG Operon, Ebersberg, Germany).
Sequence Analysis and Primer Designs
The nucleotide and protein bioinformatic analyses of the recombinant plasmids were performed using Vector NTI 9.1.0 (Invitrogen, Invitrogen Corporation, Carlsbad, CA, United States). Similarity searches between the sequenced recombinant plasmids were done with BLASTN and BLASTX sequence alignments against the nucleotide and protein sequences in the available databases from GenBank1. Specific qPCR primers sxtA4 were designed with Primer Express 3.0 (Applied Biosystems, Carlsbad, CA, United States) in the clone similarity sequence between A. minutum and A. pacificum, respectively (Supplementary Table 4). The sequence data from this article can be found in the GenBank/EMBL databanks and are listed in Supplementary Table 4 with their accession numbers.
Quantitative PCR Experiments (qPCR)
All of the quantitative PCRs (qPCR) were performed on an MX3000p qPCR system (Agilent Technologies Inc., Santa Clara, CA, United States) with 96-well polypropylene plates (Agilent). All of the qPCR reactions were run with Brilliant III Ultra-Fast SYBR® Green qPCR Master Mix (Agilent), using 1× SYBR® Green qPCR Master Mix, 300 nM of each primer (Supplementary Table 3) (sxt072-MinuPaci/sxt0073) and a known quantity of gDNA (1 and 5 ng) or recombinant plasmid DNA. The amplification cycle consisted of a hot start, 3 min at 95°C, amplification, 40 cycles for 50 s at 95°C and 20 s at 60°C. The specificity of the PCR amplification was checked using a heat dissociation protocol, one cycle for 1 min at 95°C, 30 s at 60°C and 30 s at 95°C after the final cycle of PCR. For all of the qPCR experiments, standard curves were obtained using a 10-fold dilution series (ca. 0–1.107 copies) of Spe I linearized recombinant plasmids (New England BioLabs, Ipswich, United Kingdom). The sxtA4 inserts were generated from purified PCR products from the A. minutum and A. pacificum strains RCC2645, IFR-ACA-15 and B9-1 (Supplementary Table 4). The sxtA4 purified PCR products containing ∼8.104 and 8.105 copies, were used as internal standards to verify qPCR efficiency. Three biological replicates were performed, each in four technical replicates. A negative control without DNA was included for each PCR mix. All of the assays showed a R2 > 0.98 with mean efficiency of 104% (min 102%, max 109%).
sxtA4 Copy Number Determination
The DNA found in the purified PCR products, samples and linearized recombinant plasmids was quantified with the Quant-iTTMPicoGreen® dsDNA assay kit (Invitrogen, Carlsbad, CA, United States) according to the manufacturer’s instructions. For the sxtA4 genes, the standard curves were established by relating the Log10 number of copies to the threshold cycle number (Ct). The linear regression equation was determined using the MX3000p qPCR system where Iplate is the intercept and S is the slope. According to the linear regression equation, the copy numbers in each reaction (CPNr) were calculated as described by Stüken et al. (2015), with CPr corresponding to the crossing point of the individual reaction. Subsequently, the copy numbers in each reaction per ng (CPNng) were estimated as follows: where DNAin is the amount of input DNA in ng. The copy numbers per genome (CPNG) were calculated as follows: where Gsize is the measured genome size of the strain in pg.
sxtA4 Analyses in the A. minutum Transcriptomic Database
The A. minutum strain-specific sequence and expression levels of sxtA4 were obtained from a previously published RNAseq dataset (Le Gac et al., 2016). Each strain was collected at the end of the exponential phase and the mRNA was sequenced on an Illumina Hiseq PE 2× (100 bp). Normalized expression values are calculated as 2^rlog (coverage). The FreeBayes software (Garrison and Marth, 2012) was used to identify genetic variants. As A. minutum strains are haploid in our culture conditions, allelic variants within strains reflect the presence of genetically divergent gene copies within a given strain. Biallelic variants were considered when displaying a quality criterion >40 and when covered more than 20 times in each strain. For each variant site, the reference allelic frequency was calculated as the number of reads corresponding to the reference allele (i.e., as in the reference transcriptome) divided by the total number of reads covering the site. In the A. minutum reference transcriptome (https://doi.org/10.17882/45445), the sxtA4 reference sequence was comp112540_c0_seq1 (Supplementary Figure 3).
Genome Size Measurements
Genome size measurements were estimated by flow cytometry using the modified protocol described by Marie et al. (2000), and samples were extracted with minor modifications detailed in Supplementary material (Supplementary Table 5). The samples were analyzed using a FACS Canto II system equipped with a 488 nm laser. The fluorescence emission of the IP was collected by the orange photomultiplier equipped with a 610 LP filter. Each sample was analyzed for 3 min at a rate of 55 μL min–1. Three analyses were performed for each sample. The average of the first peak of the culture was compared with that of the reference on the distribution resulting from the orange channel. The subsequent quantity of DNA was then calculated using the following formula: DNAquantity(Gbp) = (Meansample/Meanreference)×6.8, where 6.8 is the DNA content in picograms (pg) of diploid human cells. In order to convert the number of nucleotide pairs to picograms, the following formula given by Dolezel et al. (2003) is used: DNAcontent(pg) = genomesize(Gbp)/0.978.
Data and Statistical Analyses
The statistical analyses were performed using RStudio 1.2.1578. All of the values were expressed as mean ± standard deviation (SD). The differences were considered significant when p < 0.05. A Student’s t-test was applied to identify statistically significant differences in the sxtA4 normalized expression between the PST strain group and the non-PST strain group and to test the difference in genome size between the two species. The comparisons between the sxtA4 CPNs and PSTs in both species were performed using the non-parametric Mann-Whitney U test (Mann and Whitney, 1947), as it was shown that the distribution was not normal. To test the various relationships between genome size, gene CPNs and total PSTs, Spearman’s rank correlation coefficient (S) was used (Chan, 2003; Akoglu, 2018).
Results
Saxitoxin-Group Profiles
Among the 20 strains analyzed, two strains of A. minutum isolated from Concarneau Bay (France) were not toxic (RCC2644 and RCC2645), meaning that the targeted PST toxins for which there is a standard were not detected by our LC/FLD analysis (LOD values are detailed in Supplementary Table 1). Concerning toxic strains at the end of the exponential growth phase, the cellular toxin quota in the A. pacificum cells ranged from 1.7 ± 0.70 to 30 ± 8.3 fmol cell–1 (14 ± 3.7 fmol cell–1); this was comparable to the level found in the PST-producing A. minutum strains, which ranged from 1.7 ± 0.20 to 19 ± 3.5 fmol cell–1 (7.4 ± 1.8 fmol cell–1) (Table 1). Four main STX analogs were detected in the A. minutum strains (C1, C2, GTX2, GTX3), with the notable exception of strain CH940x which only contained GTX2 and GTX3 (Figure 1). Traces of Neo-SXT (RCC7038 only) and dcGTX2/3 (all of the A. minutum strains) were detected in all of the strains, with the exception of the RCC3167 and CH940x strains, which did not contain any A. pacificum strains contained C2 and GTX4, with the exception of strain C2-4 which contained C2 and GTX5 as dominant toxins and only traces of GTX4. Traces of dc-GTX2, GTX3, C1 and Neo-STX accounted for less than 10% of the total toxin content in the other strains.
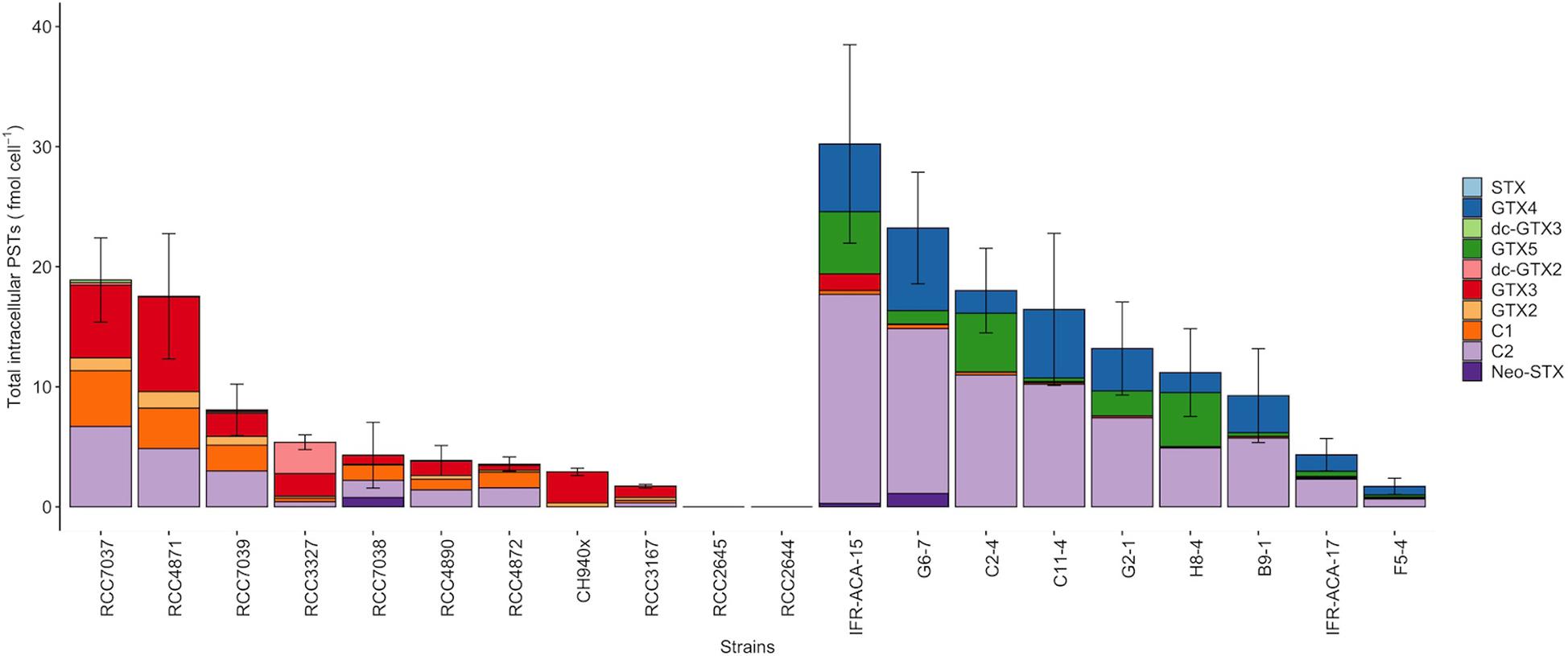
Figure 1. Intracellular STX-group content in the Alexandrium minutum (left) and A. pacificum (right) strains (including Neo-STX, dc-GTX2/3, GTX2/3, C1, C2, GTX1/4) (means ± SD, n = 3. Error bars represent standard deviations).
sxtA4 Copy Number per Genome
The sxtA4 gene was amplified from all of the strains (toxin-producing and non-producing ones) but different copy numbers were detected. The sxtA4 CPNs were significantly higher in A. pacificum compared with A. minutum (U test, W = 2, p < 0.001) (Figure 2). We counted between 9 and 46, and between 34 and 187 genomic copies of sxtA4 in the toxic strains of A. minutum and A. pacificum, respectively (Table 1). In the two non-toxic A. minutum strains (RCC2644 and RCC2645), we counted a single sxtA4 gene copy per genome. An overall positive relationship was statistically supported between the sxtA4 CPN per genome and the total toxin content per cell (Spearman test, ρ = 0.58, S = 15085, p < 0.001) (Figure 3A). However, this correlation was more robust for A. minutum than for A. pacificum (Spearman test; A. minutum, ρ = 0.45, S = 3308, p < 0.01; A. pacificum, ρ = 0.47, S = 1736, p < 0.05). The RCC7037 and RCC4871 A. minutum strains contrasted with this general rule as they had high PST contents but a somewhat mid-range CPN value (Figure 3B). No correlations were detected between the sxtA4 CPN and the year of isolation or geographical origin of the strains. For instance, the sxtA4 CPN ranged from 34 ± 17 to 187 ± 71 in eight strains of A. pacificum isolated in the same year (2017) and from the same location (Thau Lagoon, Mediterranean Sea).
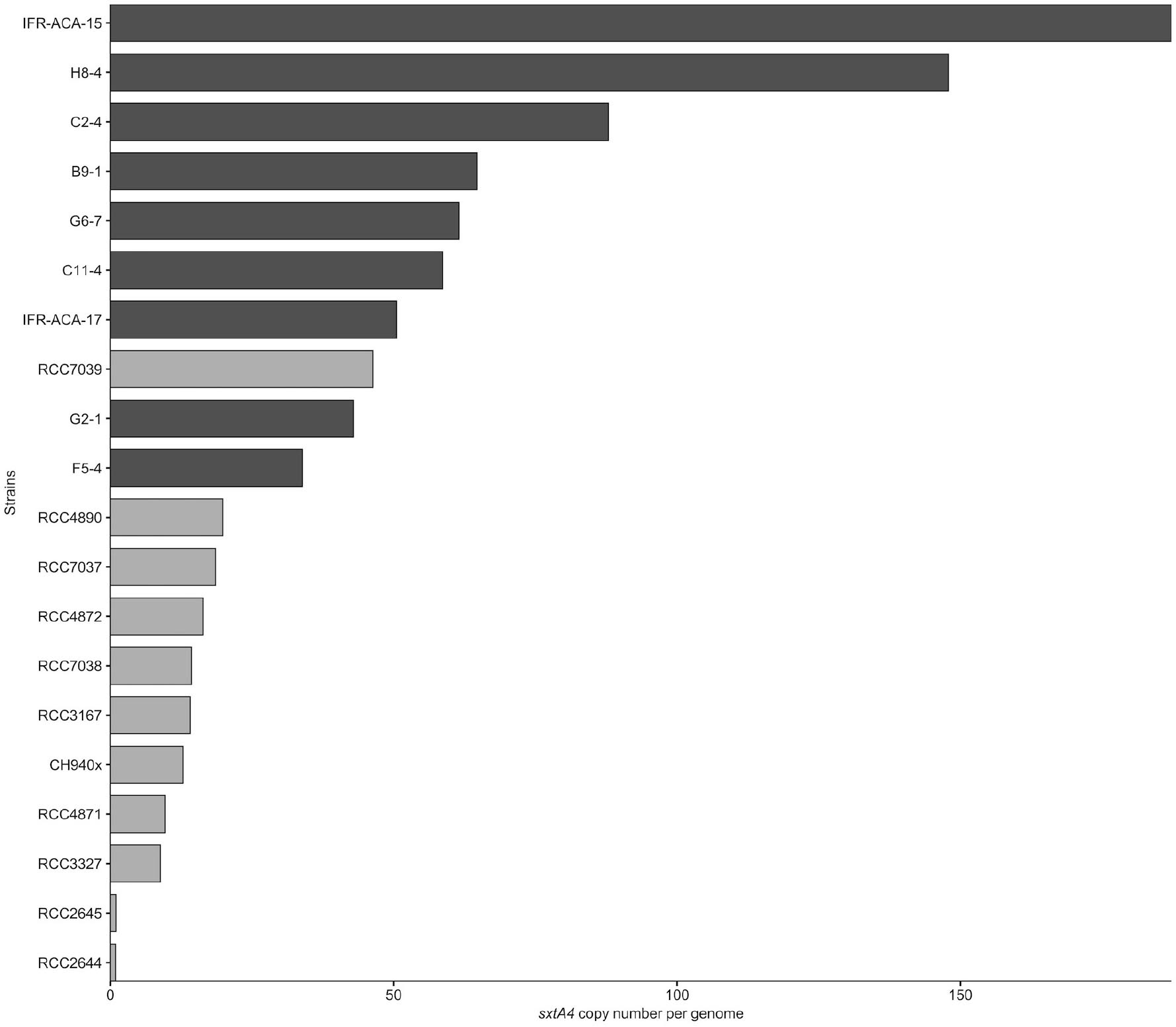
Figure 2. Average genomic sxtA4 copy number in the A. minutum (light gray) and A. pacificum (dark gray) strains (n = 3).
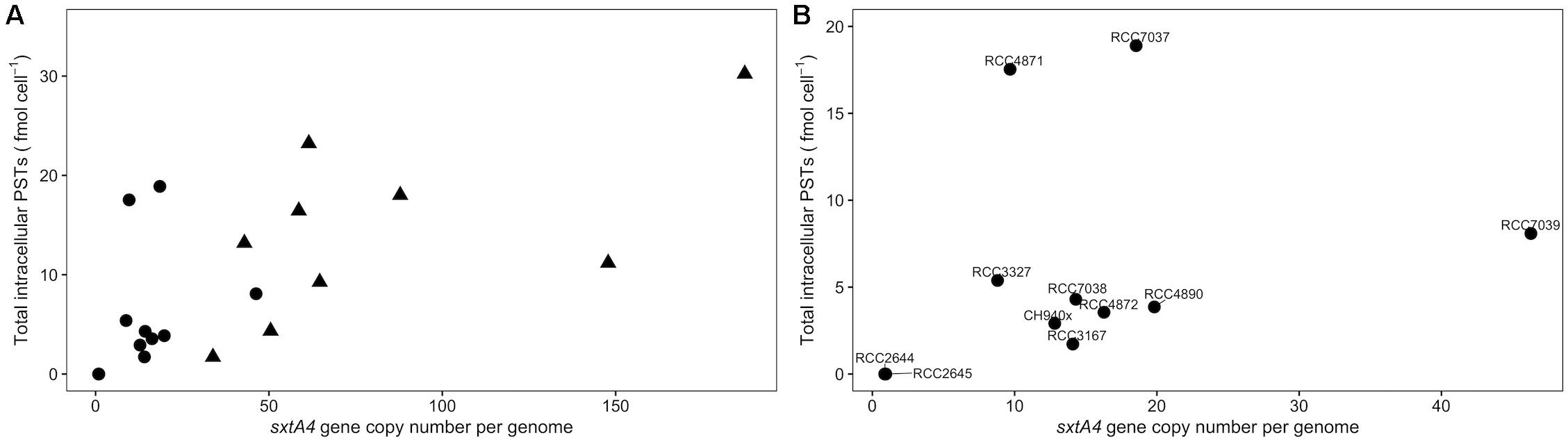
Figure 3. Scatterplots of the relationship between (A) the total intracellular toxins and the sxtA4 copy number per genome in all of the strains (ρ = 0.58, S = 15085, p = 1.5 × 10– 6), and (B) specifically for A. minutum (ρ = 0.65, S = 3308, p = 0.009).
Genome Size
Alexandrium pacificum had a genome size that was three times larger than that of A. minutum (24 ± 0.70 and 76 ± 1.2 pg, respectively), with low intraspecific variation (t-test, t = 66.6, df = 14.5, p < 0.001) (Table 1). These values are in the range of those previously reported for other Alexandrium species, e.g., from 21.8 pg in A. andersonii to up to 204.5 pg in certain strains of A. tamarense (LaJeunesse et al., 2005; Figueroa et al., 2014; Casabianca et al., 2017). A positive correlation was found between the genome size and sxtA4 CPNs when the 20 strains were considered (Spearman’s coefficient, ρ = 0.58, S = 15817, p < 0.001) (Figure 4). However, this correlation would no longer be supported if only a single species was considered due to a difference in the genomic size between A. minutum and A. pacificum (Supplementary Figure 2B).
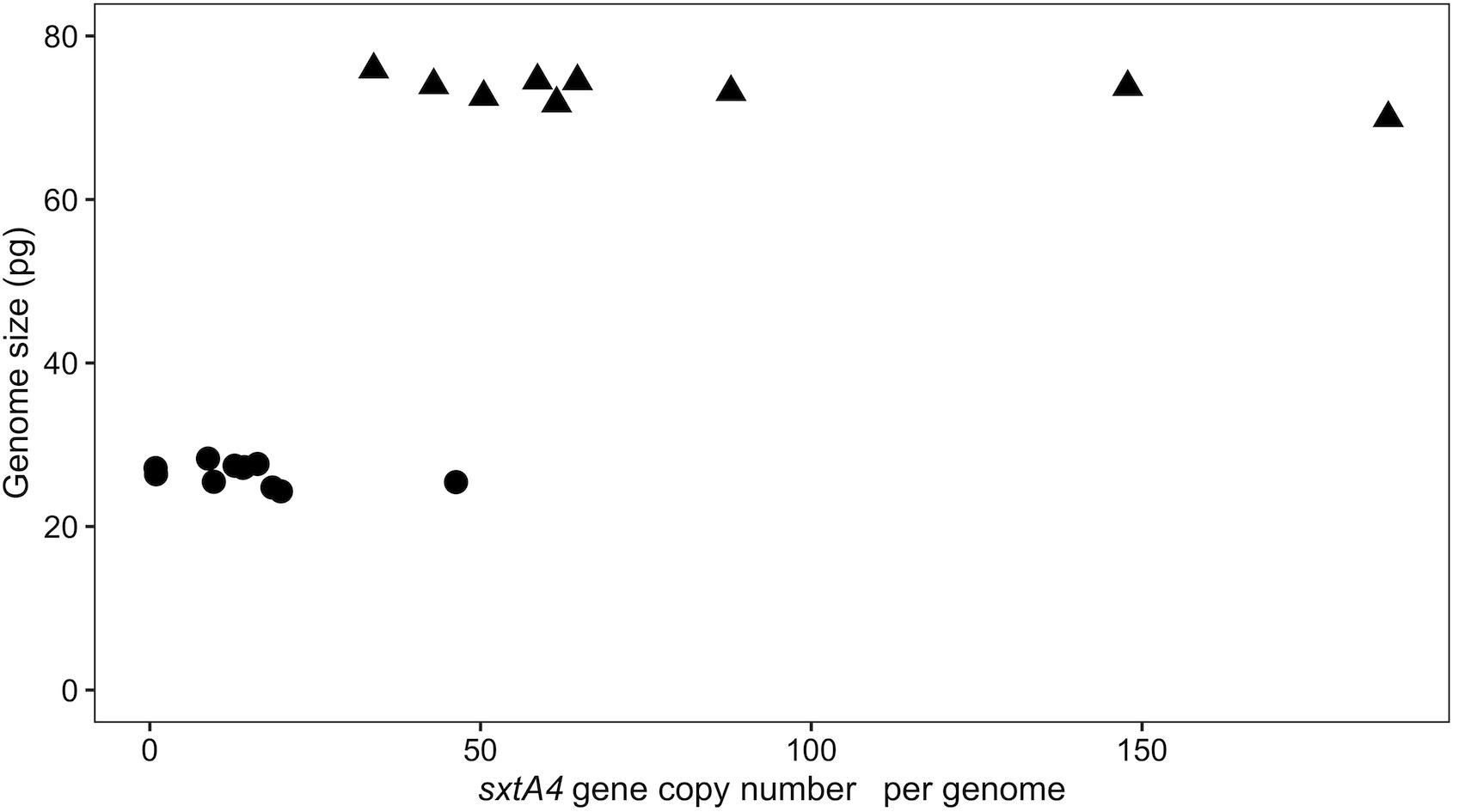
Figure 4. Scatterplots of the correlation between genome size and the sxtA4 CPN (ρ = 0.58, S = 564, p = 0.009) for the A. minutum (circles) and A. pacificum (triangles) strains.
A. minutum sxtA4 Gene Expression and Genetic Variation
The sxtA4 gene expression level was examined in two non-toxic strains and nine toxic strains. The sxtA4 expression in the non-toxic strains was significantly lower than in the toxic strains (1733 ± 371.4 vs. 3257 ± 513.9, respectively, t-test, p < 0.05) (Figure 5). In all of the eleven A. minutum strains investigated, a total of 30 variable sites were detected on comparison with the reference sxtA4 sequence (Figure 6). The nine toxic strains exhibited polymorphic sxtA4 copies with some conserved parts and variable sites that differed between the strains. The toxic strains had between two to five invariable sites (i.e., reference allelic frequency of 0 or 1). For instance, the CH940x strain, which bore five invariable sites, only produced two toxins (GTX2 and GTX3). Whereas the nine PST-producing strains were polymorphic, the two non-producing strains had a monomorphic sxtA4 gene, which was identical for the two strains. We also noted that two genetic positions (positions 456 and 1189) in the sxtA4 mRNA sequence tended to display fixed differences between the PST-producing and non-producing strains. In these A. minutum strains, a strong significant positive correlation was found between the sxtA4 CPN per genome and the normalized sxtA4 expression (ρ = 0.73, S = 58.63, p = 0.01) (Figure 7).
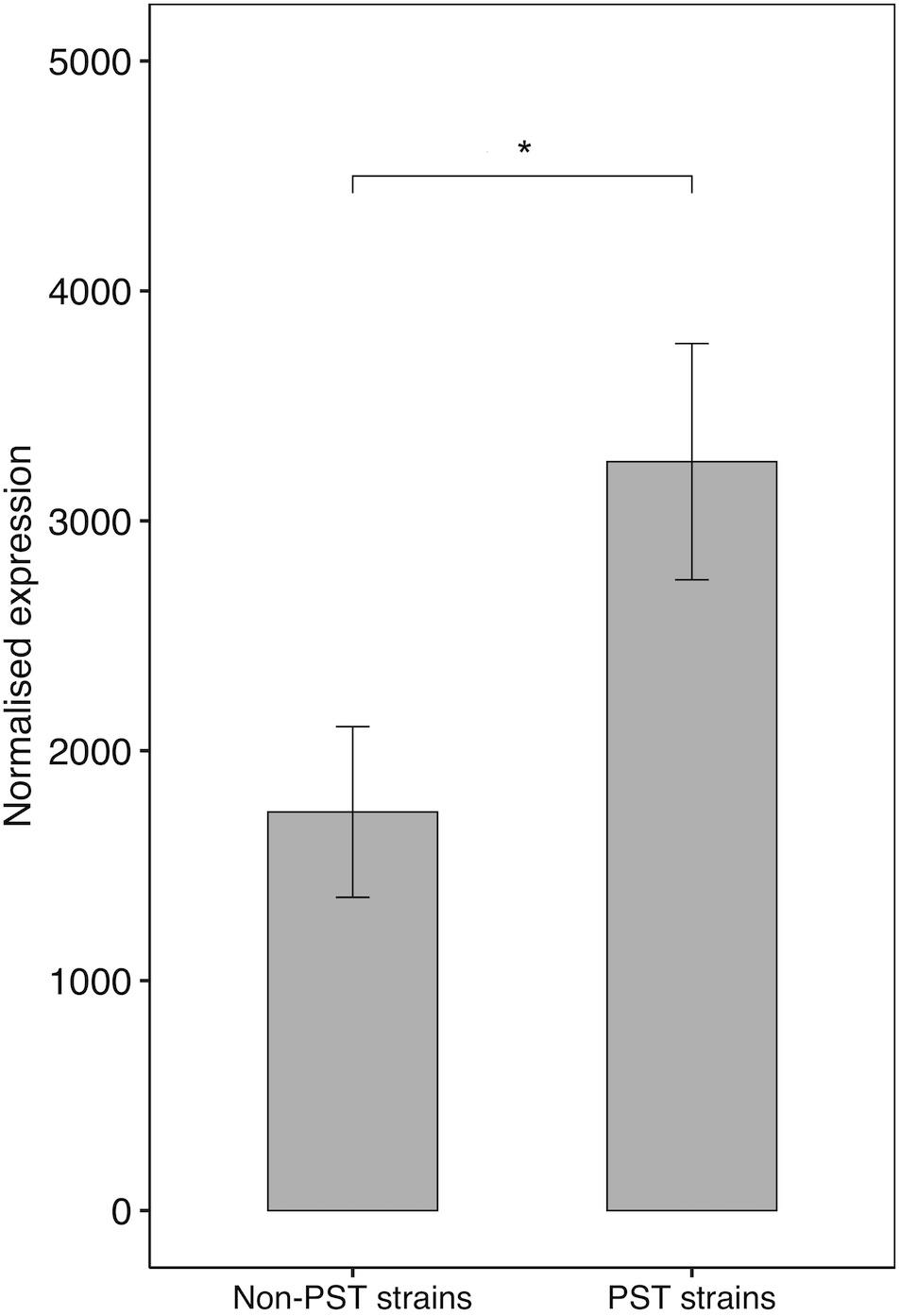
Figure 5. SxtA4 normalized expression (from comp112540_c0_seq1) in the non-PST producing A. minutum strains (RCC2644 and RCC2645) and the PST-producing A. minutum strains (RCC3327, RCC7038, CH940x, RCC3167, RCC7037, RCC4871, RCC7039, RCC4872, and RCC4890). T-test, *p < 0.05. Error bars represent the standard deviations.
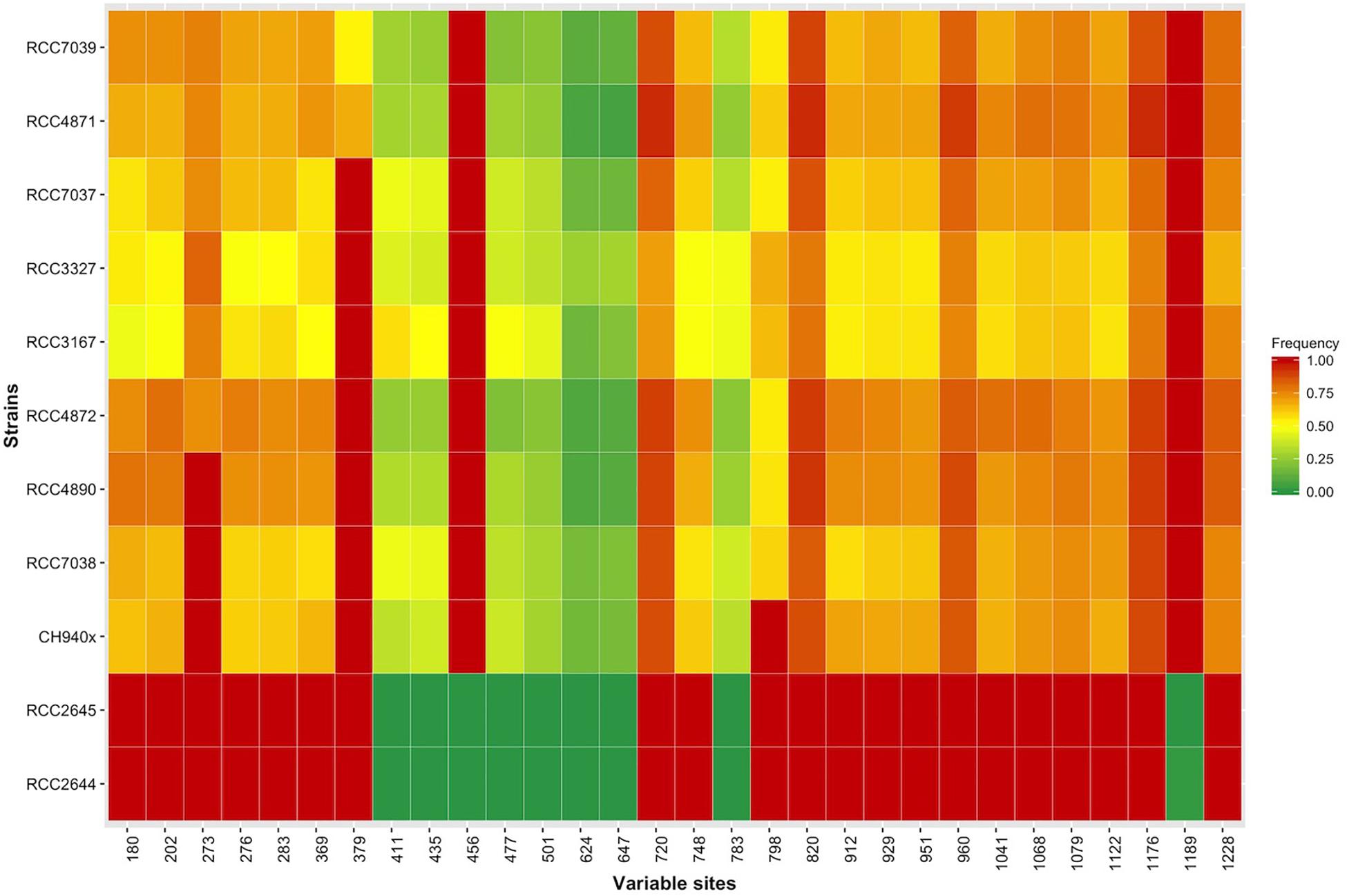
Figure 6. Heatmap showing the allelic frequencies at the 30 genetically variable sites in the sxtA4 sequence (comp112540_c0_seq1) for each A. minutum strain.
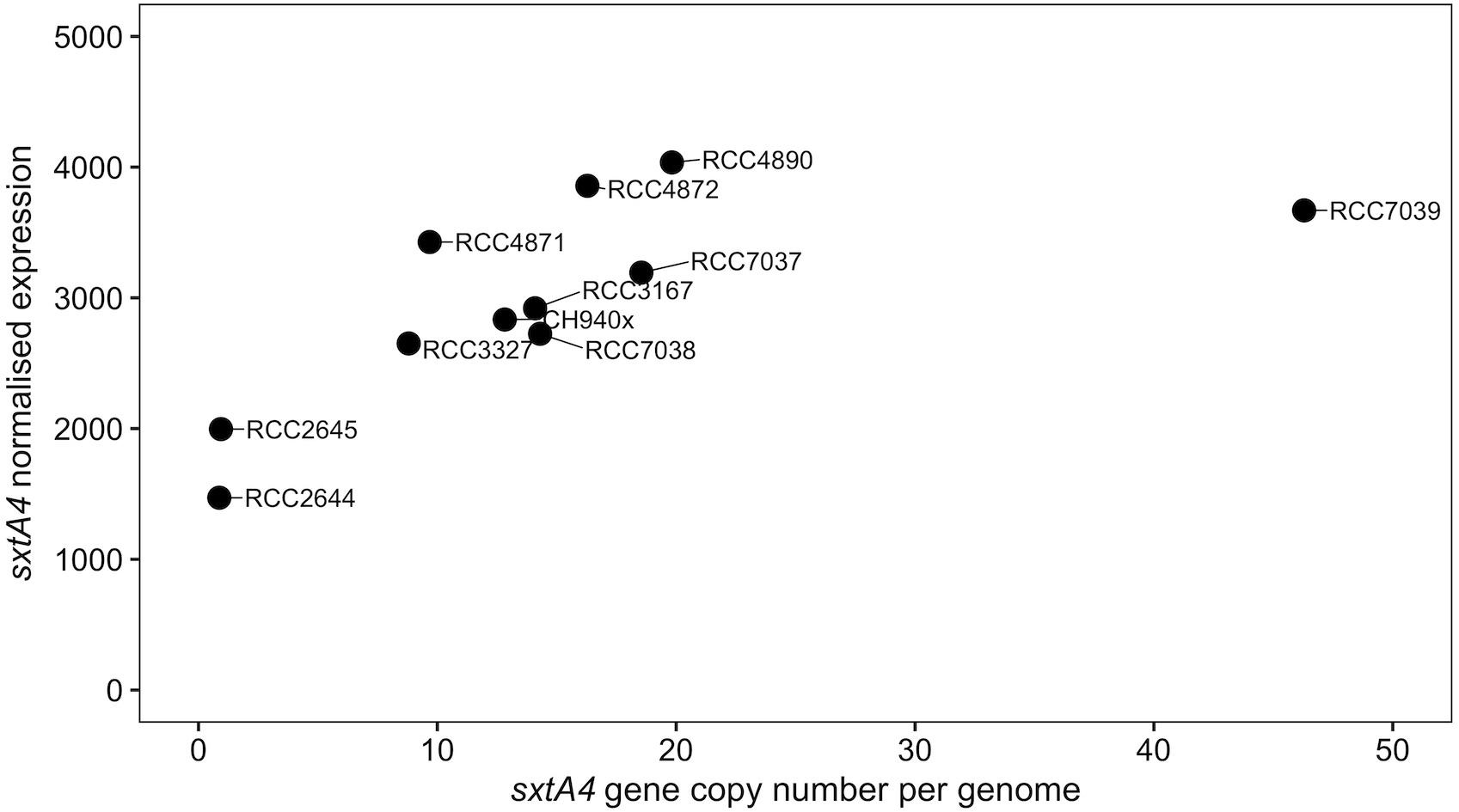
Figure 7. Scatterplot of the correlation between the sxtA4 copy number per genome and the sxtA4 normalized expression in the A. minutum strains (ρ = 0.82, S = 38, p = 0.003).
Discussion
Interspecific Variability in Toxin Phenotype
Alexandrium minutum and A. pacificum are two well established species that differ in their toxin profiles (Balech, 1995; Laabir et al., 2007). The dominant saxitoxin isoforms found here in the French A. pacificum strains (GTX4, C2, and/or GTX5) are in line with the toxin profiles reported for the various strains found in the North Mediterranean Sea (Laabir et al., 2013; Lugliè et al., 2017), and differ from populations of the South Mediterranean Sea (dominated by GTX6 or C1 or GTX1, Hadjadji et al., 2020), Australian (yielded mostly GTX5 and GTX6, GTX1/4 and C1/2, Ajani et al., 2017) and Asian populations (Laabir et al., 2013). Similarly, the species A. minutum also presented diverse toxin profiles that were categorized into five clusters by Lewis et al. (2018). All of the strains originating from northwestern France (Morlaix and Brest) corresponded well with cluster 4, which gathered A. minutum species from Northern Europe characterized by a significant amount of C1/2 and GTX2/GTX3 toxins (Grzebyk et al., 2003; Nascimento et al., 2005; Touzet et al., 2007a,b, 2008). The Cork strain likely belonged to cluster 2, which included strains producing high levels of GTX2/3 from Northern Europe, especially from Ireland. Two strains from Concarneau Bay did not contain any targeted toxins (RCC2644 and RCC2645). It is uncommon to find non-toxic strains within primarily toxic species, although this had already been reported in A. australiense, A. pacificum, A. ostenfeldii, A. minutum, and A. affine (Nguyen-Ngoc, 2004; Touzet et al., 2007a; Brown et al., 2010; Yang et al., 2010; Murray et al., 2012; Suikkanen et al., 2013; Zou et al., 2014). However, RCC2644 and RCC2645 were still highlighted as being independent genetic entities from other A. minutum strains originating from Cork harbor or the coasts in northwestern France (Le Gac et al., 2016). One possible scenario explaining this situation would be an ancestral divergence via complete isolation followed by a secondary contact involving gene flow (Le Gac et al., 2016). The presence of a ventral pore on the right side of the 10 plate was pointed out as a useful diagnostic phenotypic characteristic that can be used to identify this subgroup of A. minutum strains originating from Concarneau (southern Brittany, France). Our study highlights an absence of known toxin production as another likely distinctive feature of this subgroup of A. minutum strains, which could constitute a 6th cluster in the A. minutum populations. Both A. minutum and A. pacificum exhibited various toxin profiles among the studied populations and across their biogeographical distributions, potentially calling their descriptions as unique species into question.
Variability in sxtA4 Copy Numbers and Genome Size
Considering the A. minutum and A. pacificum strains all together, our results suggest a continuum between genome size, gene copy number and toxin content. Hence, in the larger cells of A. pacificum, the larger genome contained more elevated sxtA4 CPNs and a higher toxin content than in the smaller cells of A. minutum. A significant difference in the number of sxtA4 gene copies was observed here between the species, with a four-fold lower sxtA4 CPNs in the toxin-producing strains of A. minutum (9–46) than A. pacificum strains (34–187). These observations are congruent with previous publications reporting variable sxtA4 CPNs depending on the strains and species (Table 2). The sxtA4 CPNs for A. minutum reported in this study (9–46 genomic copies) were higher than those reported by Stüken et al. (2015), (1.5 to 10.8 genomic sxtA4 CPN). By contrast, similar values were reported for A. pacificum in this study (34–187 sxtA4 CPN) and for the Australian strains (100–280 sxtA4 CPN) (Murray et al., 2011, 2015; Stüken et al., 2011). In Gymnodinium catenatum, which produces PST concentrations up to 122 fmol cell–1 (Montoya et al., 2006), 74 to 143 copies cell–1 were reported in one strain for different growth phases (Mendoza-Flores et al., 2018). No correlation was detected here, between the sxtA4 CPN and the year of isolation or geographical origin of the strains (for instance, 34–187 sxtA4 CPNs for eight A. pacificum strains isolated in Thau Lagoon in 2017).
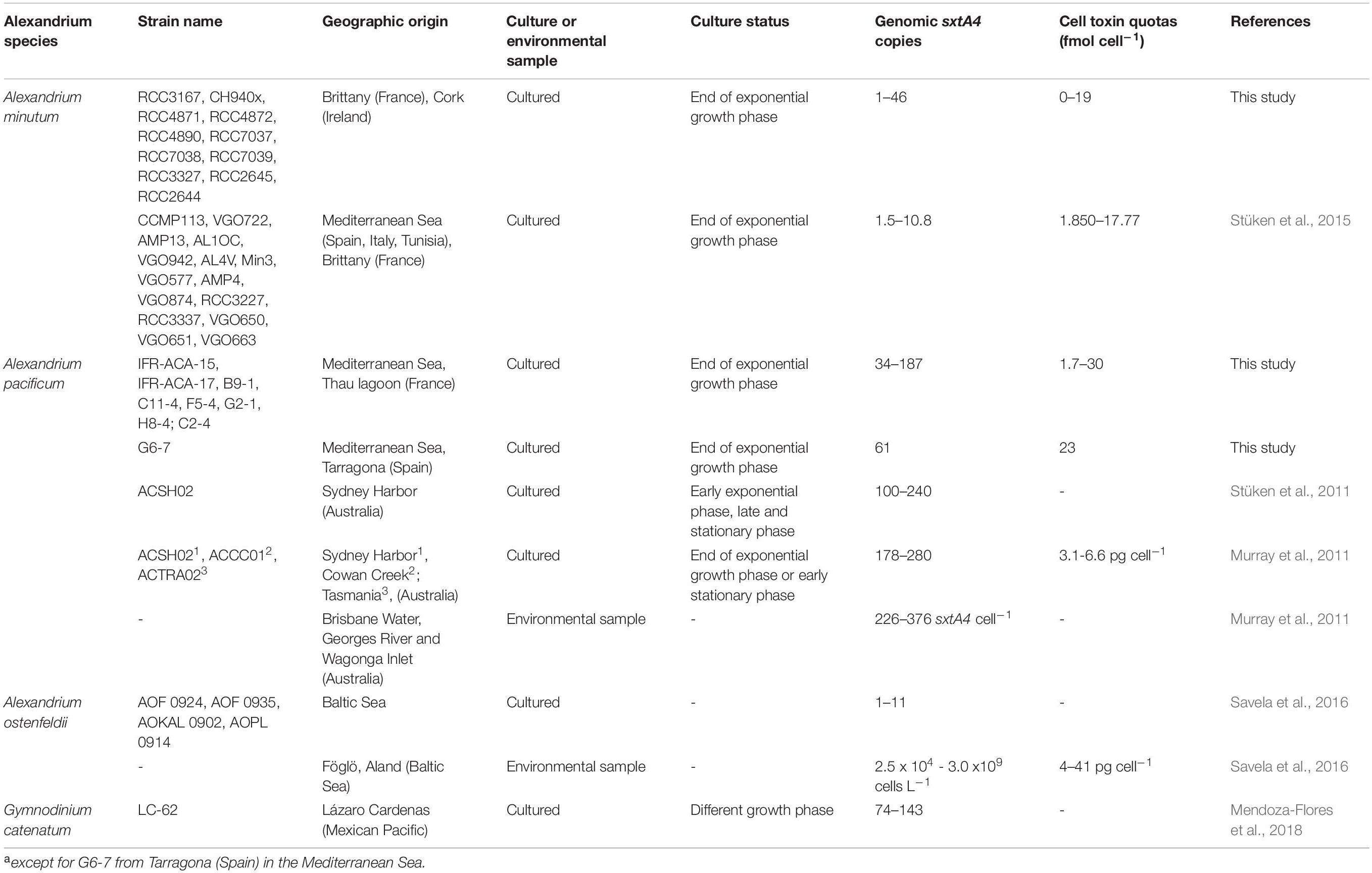
Table 2. sxtA4 CPN per genome and toxin content from PST-producing species reported in the literature.
The numerous sxtA4 copies found in these two species propose that toxin genes were conserved throughout the genomic replication occurring during the evolution of dinoflagellates, in particular A. minutum and A. pacificum species (Lin, 2011). The multiple gene copies are not restricted to toxins in dinoflagellates, and the number of gene copies may be elevated and highly variable both within and between species. This was the case for gene encoding rRNA (2,489,800 ± 550,967 A. pacificum CNR-ACATS3 and 1345 ± 780 in A. taylori CBA-1), luciferase (44–160 copies), the peridinin-chlorophyll a-binding protein (36–5000), protein kinase (30), actin (>113 gene copies), proliferating cell nuclear antigens (191.87 ± 32.13) and even form II RuBisCO (117 ± 40, 148 ± 16), which were detected in a wide range of copy-number interspecies of the genera Alexandrium, Protoceratium, Prorocentrum, and Lingulodinium (Le et al., 1997; Salois and Morse, 1997; Zhang and Lin, 2003; Liu and Hastings, 2005; Galluzzi et al., 2010; Shi et al., 2013; Hou et al., 2019). Further, the comparison of sxtA4 gene copy number with the copy number of other genes in A. minutum and A. pacificum would give a better comprehension on the conservation and evolution of toxin genes in the whole genome.
The results of our study support the hypothesis of a direct link between sxtA4 CPN and toxin content in the species A. minutum and A. pacificum as proposed by some authors on A. minutum (Stüken et al., 2015). This indicated that the sxtA4 CPN partially determined the amount of toxins produced in these species. However, with the exception of three strains (A. minutum RCC7037, RCC7039, and A. pacificum H8-4) that showed a weak correlation between the sxtA4 CPN and the toxin content. Moreover the correlation does not exist for some other Alexandrium species such as A. ostenfeldii strains, which contain a high level of intracellular toxin (4 to 41 pg cell–1) but only a few copies of the sxtA4 gene (∼ 6 copies per genome), indicating no direct relationships between PST production and cellular sxtA4 copies in this species (Savela et al., 2016). The accumulation of non-functional sxtA4 copies and/or additional regulation processes at the transcriptional and post-transcriptional levels might also explain a divergence between the number of sxtA4 copies and the quantity of toxins produced in A. minutum and A. pacificum.
The genome size appeared to have a lower intra-specific variability (24–27 and 72–76 pg), compared with what was observed for the toxin content (1.7–19 and 1.7–30 fmol cell–1) and sxtA4 CPN (9–46 and 34–187) within the A. minutum vs. A. pacificum strains, respectively, indicating other evolutionary constraints for genome size. The genome size of dinoflagellates is particularly large compared with other free-living Myzozoa (chromerids, up to 193 Mb; LaJeunesse et al., 2005; Stüken et al., 2015; Woo et al., 2015), but is rather homogeneous within strains of a single species in our data. This observation can be explained by a process accumulating genomic material while maintaining a rather constant genome size. The interspecific variations in genome sizes, DNA content and the copy number of sxtA4 genes between A. minutum and A. pacificum have been potentially explained by genome duplications and polyploidization (Loper et al., 1980; Stüken et al., 2015). However, polyploidy was refuted based on genome analysis of four Symbiodinium species (Lin et al., 2015; Liu et al., 2018), and would considerably scatter the genome size values within a given species. Other evolutionary processes may explain a constrained but large genome size and intraspecific variabilities in terms of CPNs in dinoflagellates such as segmental duplication by unequal crossover, may be favored by the permanently condensed dinoflagellate chromosomes, and/or retroposition mechanisms via the reverse transcription of mRNAs and their re-integration within the genome (Smith, 1976; Jaeckisch et al., 2011; Hou et al., 2019).
The presence of the 4th domain of the sxtA gene in the genome of Alexandrium spp. seems to be a good proxy for the capacity of a given strain to produce saxitoxins, with few exceptions reported so far (i.e., A. minutum RCC2644, RCC2645; A. australiense ATCJ33, ATEB01; A. tamarense CCMP1771; and the mutant A. pacificum ACHK-NT) (Murray et al., 2011; Stüken et al., 2011; Zhang et al., 2014). In the European A. minutum and A. pacificum strains, the genomic copy number of sxtA4 also appears to be a good indicator of the toxin production level, tough it remains an ambiguous marker for other species. Moreover, given that there is a potential relationship between the genome size, the gene copy number and toxin quotas in dinoflagellates, the compilation of a larger dataset on PST-producing strains of dinoflagellates is encouraged so as to further assess this observation.
sxtA4 Expression and Isoforms in A. minutum
The nine toxin-producing strains with several (>9) sxtA4 copies were genetically polymorphic, with several divergent sxtA4 copies co-occurring within a given strain. The observed variability in the toxin phenotypes (in terms of content) may result from the polymorphism of the sxtA4 copies that exists among the strains. Also, the polymorphism seems to be a crucial feature for toxin production, since a higher number of fixed sites was associated with lower toxin diversity (as found in the strain CH940x).
We reported two novel strains of non-toxic A. minutum (RCC2644 and RCC2645) bearing a unique sxtA4 copy. Two mutations, located at two distinct positions (456 and 1186), could be used to distinguish between toxin- and non-toxin producers. These mutations did not disrupt the transcription into mRNA since the sxtA4 gene was expressed in the two non-toxic strains, though in fewer copies. These results demonstrate that the absence of targeted toxin production in strains RCC2644 and RC2645 was not due to the absence of domain 4 of the sxtA gene but to the presence of an atypical isoform of sxtA4 in a small number of copies.
Otherwise, a positive relationship was observed between the sxtA4 CPN and the mRNA expression levels in this study and, for the first time, a direct relationship between sxtA4 mRNA expression and the toxin content was observed for all A. minutum strains. Again, this indicates that the sxtA4 CPN in the genome may contribute in determining the amount of toxins produced by A. minutum species, an observation that supports constitutive gene expression.
Moreover, cases of discrepancies between the CPN and toxin amounts suggested that transcription processes play also a major role in the regulation of STX-group synthesis, as already suggested by Bachvaroff and Place (2008). For instance, here, four-fold higher sxtA4 CPNs in A. pacificum was associated with only 1.5-fold higher toxin content than in A. minutum. Also as detailed above, our results showed a weak correlation for three strains (A. minutum RCC7037 and RCC7039, A. pacificum H8-4) and similarly, another study reported no correlation for A. ostenfeldii, in which a high level of intracellular toxin content (4 to 41 pg cell–1) was associated with a low sxtA4 CPN (∼6 copies per genome) (Savela et al., 2016).
Furthermore, although Zhang et al. (2014) reported the down-regulation of sxtA4 mRNA associated with the absence of toxin production in the mutant ACHK-NT, most studies were unable to identify a correlation between the expression level and the amount of toxin, suggesting the concomitant participation of other expression-modifying mechanisms such as translational and post-translational mechanisms. For instance, no significant variations in the expression level of toxin-related genes were observed in A. pacificum strain ACHK-T (sxtB, sxtD, sxtF/M, sxtG, sxtH/T, sxtI, sxtO, sxtP, sxtU, sxtW, sxtX, sxtZ, sxtPER) (Zhang et al., 2017) or in A. pacificum ACCC01 (sxtA4) during the toxin-producing phases (Wiese et al., 2014). Similarly, no significant variations in the expression level were found in the two strains of toxin-producing cyanobacteria, Aphanizomenon gracile (sxtA, sxtM, sxtPer) and Raphidiopsis brookii (sxtU, sxtI) (Vico et al., 2016; Cirés et al., 2017). Moreover, no correlation was found between the toxins and the mRNA amounts for sxtA1 and sxtG in A. minutum (Perini et al., 2014).
In dinoflagellates, only 5 to 30% of the genes seem to be regulated at the transcription level; the remaining genes are supposedly regulated post-transcriptionally (Lidie et al., 2005; Erdner and Anderson, 2006). Involved processes could be mRNA editing (Scott, 1995; Lin et al., 2002, 2007; Zauner et al., 2004; Dang and Green, 2009; Mungpakdee et al., 2014), the participation of small interfering RNA (RNAsi), recently discovered in dinoflagellates (Zhang and Lin, 2019), which might affect sxtA4 expression. RNAsi hybridizes to mRNA, which leads to its degradation and alters gene expression, either by suppressing gene expression or through a gene regulatory network.
In addition to transcriptional mechanisms, translational or post-translational mechanisms (phosphorylation, methylation, and glycosylation, protein cleavage) could modify the transport of mRNAs, the translation of mRNAs into proteins, or the protein molecule itself (Akbar et al., 2020). For instance, in dinoflagellates several translational and post-translational mechanisms have been found to regulate proteins involved in bioluminescence (Mittag et al., 1994, 1998), and NADP-ICDH in circadian rhythms (Akimoto et al., 2005) in Lingulodinium polyedrum and in the cell cycle in Karenia brevis (Brunelle and Van Dolah, 2011).
Hence, several transcriptional and post-transcriptional processes may co-exist in dinoflagellates and explain why the expression of a single sxtA4 gene copy in A. minutum, even at a low level, does not lead to the production of saxitoxin in our two non-toxic strains. Nevertheless, in our French toxic strains of A. minutum, all of the potential regulation mechanisms were minimized in favor of constitutive gene expression for toxin production.
Conclusion
This study provides new data on the involvement of the sxtA4 gene in toxin production, as well as a deeper understanding of STX-group synthesis in dinoflagellates. Correlations were observed by comparing data from two independent experiments at the transcriptomic and genomic levels on the same strains of A. minutum (analyses of sxtA4 transcripts vs. analyses of the number of copies of the sxtA4 gene and the toxin content). In particular, we observed that the number of sxtA4 gene copies, the presence of genetic isoforms, and the level of the sxtA4 mRNAs expression profiles determined the toxin content of a strain. However, it remains to distinguish between the presence and the functionality of sxtA4 copies to explain potential discrepancies between high gene copy number and toxin content. Moreover, non-toxin producers are genetically distinct from other A. minutum strains, with a unique sxtA4 gene allele (isoform) detected in a single copy. Expression of this gene still occurs, although to a lesser extent compared with other toxin-producing strains. These findings are promising and need to be further screened on a larger panel of non-toxic strains to determine the products of this sxtA4 isoform. Moreover, the polymorphism observed in the sxtA4 copies, both among strains and within one strain, is likely to be related to the interspecies variability in the toxin content. Further investigations of the Alexandrium sxt genes are warranted to understand how sxt genes work together to produce diverse toxin profiles in Alexandrium.
Nonetheless, the inconsistencies encountered in some strains in terms of the CPN, expression rate and toxin contents highlight the genomic complexity of dinoflagellates, which likely regulates their gene expression at the genomic, transcriptional and translational levels. Understanding the underlying mechanisms will hopefully provide a better explanation of the observed intra- and interspecies phenotypic diversity. In the future, the expression level of PST-producing dinoflagellates should be compared with proteomic and metabolic analyses. Whereas at the environmental level, recent qPCR tests targeting the sxtA4 gene have been proven to be sensitive and to produce efficient results in terms of estimating the abundance of toxic Alexandrium cells, our results highlight the relevance of developing probes that target the various sxtA4 isoforms in order to identify toxic and non-toxic individuals within the same population.
Data Availability Statement
The datasets presented in this study can be found in online GenBank/EMBL repository. The accession number(s) can be found in the in theSupplementary Material (Supplementary Table 4).
Author Contributions
SG, M-ML, AC, LG, and ZA conceived, designed, and reviewed the study. SG carried out all experimental work, acquired, analyzed, interpreted data, and drafted the manuscript. M-ML and EB participated in the experimental design of the qPCR analysis. ML performed the transcriptomic analysis. G-AR conceived and validated the toxins analysis method. DM analyzed the genome size. FM isolated and maintained the strains. All authors contributed to the writing and editing article and approved the submitted version.
Conflict of Interest
The authors declare that the research was conducted in the absence of any commercial or financial relationships that could be construed as a potential conflict of interest.
Acknowledgments
We are authors are grateful to the IFREMER Institute and the Regional Council of the Région des Pays de La Loire for the Ph.D. funding of SG. We would like to thank, Enora Briand for their assistance in the design of experimentation and their support in molecular biology, Estelle Masseret and Ester Garces for providing several A. pacificum strains. We also acknowledged Eric Abadie, Nicolas Chomérat and Gwenaël Bilien from IFREMER for the isolation and identification of several Alexandrium strains.
Supplementary Material
The Supplementary Material for this article can be found online at: https://www.frontiersin.org/articles/10.3389/fmicb.2021.613199/full#supplementary-material
Footnotes
References
Abdenadher, M., Hamza, A., Fekih, W., Hannachi, I., Zouari Bellaaj, A., Bradai, M. N., et al. (2012). Factors determining the dynamics of toxic blooms of Alexandrium minutum during a 10-year study along the shallow southwestern Mediterranean coasts. Estuar. Coast. Shelf Sci. 106, 102–111. doi: 10.1016/j.ecss.2012.04.029
Aguilera-Belmonte, A., Inostroza, I., Carrillo, K. S. S., Franco, J. M. M., Riobó, P., Gómez, P. I. I., et al. (2013). The combined effect of salinity and temperature on the growth and toxin content of four Chilean strains of Alexandrium catenella (Whedon and Kofoid) Balech 1985 (Dinophyceae) isolated from an outbreak occurring in southern Chile in 2009. Harmful Algae 23, 55–59. doi: 10.1016/j.hal.2012.12.006
Ajani, P., Harwood, D. T., and Murray, A. (2017). Recent trends in marine phycotoxins from Australian coastal waters. Mar. Drugs 15:33. doi: 10.3390/md15020033
Akbar, M., Ahmad, A., Usup, G., and Bunawan, H. (2018). RNA-Seq as an emerging tool for marine dinoflagellate transcriptome analysis: process and challenges. Processes 6:5. doi: 10.3390/pr6010005
Akbar, M., Mohd Yusof, N. Y. Y., Tahir, N. I. I., Ahmad, A., Usup, G., Sahrani, F. K. K., et al. (2020). Biosynthesis of saxitoxin in marine dinoflagellates: an omics perspective. Mar. Drugs 18, 103. doi: 10.3390/md18020103
Akimoto, H., Kinumi, T., and Ohmiya, Y. (2005). Circadian rhythm of a TCA cycle enzyme is apparently regulated at the translational level in the dinoflagellate Lingulodinium polyedrum. J. Biol. Rhythms 20, 479–489. doi: 10.1177/0748730405280811
Akoglu, H. (2018). User’s guide to correlation coefficients. Turkish J. Emerg. Med. 18, 91–93. doi: 10.1016/j.tjem.2018.08.001
Anderson, D. M., Alpermann, T. J., Cembella, A. D., Collos, Y., Masseret, E., and Montresor, M. (2012). The globally distributed genus Alexandrium: multifaceted roles in marine ecosystems and impacts on human health. Harmful Algae 14, 10–35. doi: 10.1016/j.hal.2011.10.012
Anderson, D. M., Kulis, D. M., Sullivan, J. J., Hall, S., and Lee, C. (1990). Dynamics and physiology of saxitoxin production by the dinoflagellates Alexandrium spp. Mar. Biol. 524, 511–524.
Bachvaroff, T. R., and Place, A. R. (2008). From stop to start: tandem gene arrangement, copy number and Trans-splicing sites in the dinoflagellate Amphidinium carterae. PLoS One 3:e2929. doi: 10.1371/journal.pone.0002929
Balech, E. (1995). The Genus Alexandrium Halim (Dinoflagellata). Cork: Sherkin Island Marine Station.
Borges, H. L. F. F., Branco, L. H. Z. Z., Martins, M. D., Lima, C. S., Barbosa, P. T., Lira, G. A. S. T., et al. (2015). Cyanotoxin production and phylogeny of benthic cyanobacterial strains isolated from the northeast of Brazil. Harmful Algae 43, 46–57. doi: 10.1016/j.hal.2015.01.003
Botana, L. M., Hess, P., Munday, R., Nathalie, A., DeGrasse, S. L., Feeley, M., et al. (2017). Derivation of toxicity equivalency factors for marine biotoxins associated with Bivalve Molluscs. Trends Food Sci. Technol. 59, 15–24. doi: 10.1016/j.tifs.2016.09.015
Bricelj, V. M., and Shumway, S. E. (1998). Paralytic shellfish toxins in bivalve molluscs: occurrence, transfer kinetics, and biotransformation. Fish. Sci. 6, 315–383. doi: 10.1080/10641269891314294
Brown, L., Bresnan, E., Graham, J., Lacaze, J. P., Turrell, E., and Collins, C. (2010). Distribution, diversity and toxin composition of the genus Alexandrium (dinophyceae) in Scottish waters. Eur. J. Phycol. 45, 375–393. doi: 10.1080/09670262.2010.495164
Brunelle, S. A., and Van Dolah, F. M. (2011). Post-transcriptional regulation of S-Phase genes in the dinoflagellate, karenia brevis. J. Eukaryot. Microbiol. 58, 373–382. doi: 10.1111/j.1550-7408.2011.00560.x
Caruana, A. M. N., and Amzil, Z. (2018). “Microalgae and toxins,” in Microalgae in Health and Disease Prevention, eds I. A. Levine and J. Fleurence, (Amsterdam: Elsevier Inc.), 263–305. doi: 10.1016/B978-0-12-811405-6.00013-X
Caruana, A. M. N., Le Gac, M., Hervé, F., Rovillon, G. A., Geffroy, S., Malo, F., et al. (2020). Alexandrium pacificum and Alexandrium minutum: harmful or environmentally friendly? Mar. Environ. Res. 160:105014. doi: 10.1016/j.marenvres.2020.105014
Casabianca, S., Cornetti, L., Capellacci, S., Vernesi, C., and Penna, A. (2017). Genome complexity of harmful microalgae. Harmful Algae 63, 7–12. doi: 10.1016/j.hal.2017.01.003
Cirés, S., Delgado, A., González-Pleiter, M., and Quesada, A. (2017). Temperature influences the production and transport of saxitoxin and the expression of sxt genes in the cyanobacterium aphanizomenon gracile. Toxins (Basel) 9:322. doi: 10.3390/toxins9100322
Condie, S. A., Oliver, E. C. J., and Hallegraeff, G. M. (2019). Environmental drivers of unprecedented Alexandrium catenella dinoflagellate blooms off eastern Tasmania, 2012–2018. Harmful Algae 87:101628. doi: 10.1016/j.hal.2019.101628
Cusick, K. D., and Sayler, G. S. (2013). An overview on the marine neurotoxin, saxitoxin: genetics, moleculartargets, methods of detection and ecological functions. Mar. Drugs 11, 991–1018. doi: 10.3390/md11040991
Dang, Y., and Green, B. R. (2009). Substitutional editing of Heterocapsa triquetra chloroplast transcripts and a folding model for its divergent chloroplast 16S rRNA. Gene 442, 73–80. doi: 10.1016/j.gene.2009.04.006
Dhib, A., Frossard, V., Turki, S., and Aleya, L. (2013). Dynamics of harmful dinoflagellates driven by temperature and salinity in a northeastern Mediterranean lagoon. Environ. Monit. Assess. 185, 3369–3382. doi: 10.1007/s10661-012-2797-4
Dia, A., Guillou, L., Mauger, S., Bigeard, E., Marie, D., Valero, M., et al. (2014). Spatiotemporal changes in the genetic diversity of harmful algal blooms caused by the toxic dinoflagellate Alexandrium minutum. Mol. Ecol. 23, 549–560. doi: 10.1111/mec.12617
Dolezel, J., Bartos, J., Voglmayr, H., and Greilhuber, J. (2003). Letter to the editor. Cytometry 51A, 127–128. doi: 10.1002/cyto.a.10013
Erdner, D. L., and Anderson, D. M. (2006). Global transcriptional profiling of the toxic dinoflagellate Alexandrium fundyense using massively parallel signature sequencing. BMC Genomics 7:88. doi: 10.1186/1471-2164-7-88
Figueroa, R. I., Cuadrado, A., Stüken, A., Rodríguez, F., and Fraga, S. (2014). Ribosomal DNA organization patterns within the dinoflagellate genus Alexandrium as revealed by FISH: life cycle and evolutionary implications. Protist 165, 343–363. doi: 10.1016/j.protis.2014.04.001
Galluzzi, L., Bertozzini, E., Penna, A., Perini, F., Garcés, E., and Magnani, M. (2010). Analysis of rRNA gene content in the Mediterranean dinoflagellate Alexandrium catenella and Alexandrium taylori: implications for the quantitative real-time PCR-based monitoring methods. J. Appl. Phycol. 22, 1–9. doi: 10.1007/s10811-009-9411-3
Gao, Y., Yu, R. C., Murray, S. A., Chen, J. H., Kang, Z. J., Zhang, Q. C., et al. (2015). High specificity of a quantitative PCR assay targeting a saxitoxin gene for monitoring toxic algae associated with paralytic shellfish toxins in the Yellow Sea. Appl. Environ. Microbiol. 81, 6973–6981. doi: 10.1128/AEM.00417-15
Garrison, E., and Marth, G. (2012). Haplotype-based variant detection from short-read sequencing. arXiv [Preprint] Available online at: http://arxiv.org/abs/1207.3907
Giacobbe, M. G., Oliva, F. D., and Maimone, G. (1996). Environmental factors and seasonal occurrence of the dinoflagellate Alexandrium minutum, a PSP potential producer, in a Mediterranean lagoon. Estuar. Coast. Shelf Sci. 42, 539–549. doi: 10.1006/ecss.1996.0035
Grzebyk, D., Béchemin, C., Ward, C. J., Vérité, C., Codd, G. A., and Maestrini, S. Y. (2003). Effects of salinity and two coastal waters on the growth and toxin content of the dinoflagellate Alexandrium minutum. J. Plankton Res. 25, 1185–1199. doi: 10.1093/plankt/fbg088
Guallar, C., Bacher, C., and Chapelle, A. (2017). Global and local factors driving the phenology of Alexandrium minutum (Halim) blooms and its toxicity. Harmful Algae 67, 44–60. doi: 10.1016/j.hal.2017.05.005
Guillard, R., and Hargraves, P. (1993). Stichochrysis immobilis is a diatom, not a chrysophyte. Substance Use Misuse 32, 234–236. doi: 10.3109/10826087609056170
Hackett, J. D., Wisecaver, J. H., Brosnahan, M. L., Kulis, D. M., Anderson, D. M., Bhattacharya, D., et al. (2013). Evolution of saxitoxin synthesis in cyanobacteria and dinoflagellates. Mol. Biol. Evol. 30, 70–78. doi: 10.1093/molbev/mss142
Hadjadji, I., Laabir, M., Frihi, H., Collos, Y., Shao, Z. J., Berrebi, P., et al. (2020). Unsuspected intraspecific variability in the toxin production, growth and morphology of the dinoflagellate Alexandrium pacificum R.W. Litaker (Group IV) blooming in a South Western Mediterranean marine ecosystem, Annaba Bay (Algeria). Toxicon 180, 79–88. doi: 10.1016/j.toxicon.2020.04.005
Hii, K. S., Lim, P. T., Kon, N. F., Takata, Y., Usup, G., and Leaw, C. P. (2016). Physiological and transcriptional responses to inorganic nutrition in a tropical Pacific strain of Alexandrium minutum: implications for the saxitoxin genes and toxin production. Harmful Algae 56, 9–21. doi: 10.1016/j.hal.2016.04.005
Hou, Y., Ji, N., Zhang, H., Shi, X., Han, H., and Lin, S. (2019). Genome size-dependent pcna gene copy number in dinoflagellates and molecular evidence of retroposition as a major evolutionary mechanism. J. Phycol. 55, 37–46. doi: 10.1111/jpy.12815
Hurley, W., Wolterstorff, C., MacDonald, R., and Schultz, D. (2014). Paralytic shellfish poisoning: a case series. West. J. Emerg. Med. 15, 378–381. doi: 10.5811/westjem.2014.4.16279
Hwang, D. F., and Lu, Y. H. (2000). Influence of environmental and nutritional factors on growth, toxicity, and toxin profile of dinoflagellate Alexandrium minutum. Toxicon 38, 1491–1503. doi: 10.1016/S0041-0101(00)00080-5
Jaeckisch, N., Yang, I., Wohlrab, S., Glöckner, G., Kroymann, J., Vogel, H., et al. (2011). Comparative genomic and transcriptomic characterization of the toxigenic marine dinoflagellate Alexandrium ostenfeldii. PLoS One 6:e28012. doi: 10.1371/journal.pone.0028012
Kellmann, R., Michali, T. K., and Neilan, B. A. (2008a). Identification of a saxitoxin biosynthesis gene with a history of frequent horizontal gene transfers. J. Mol. Evol. 67, 526–538. doi: 10.1007/s00239-008-9169-2
Kellmann, R., Mihali, T. K., Young, J. J., Pickford, R., Pomati, F., and Neilan, B. A. (2008b). Biosynthetic intermediate analysis and functional homology reveal a saxitoxin gene cluster in cyanobacteria. Appl. Environ. Microbiol. 74, 4044–4053. doi: 10.1128/AEM.00353-08
Kellmann, R., Stüken, A., Orr, R. J. S., Svendsen, H. M., and Jakobsen, K. S. (2010). Biosynthesis and molecular genetics of polyketides in marine dinoflagellates. Mar. Drugs 8, 1011–1048. doi: 10.3390/md8041011
Laabir, M., Amzil, Z., Lassus, P., Masseret, E., Tapilatu, Y., De Vargas, R., et al. (2007). Viability, growth and toxicity of Alexandrium catenella and Alexandrium minutum (Dinophyceae) following ingestion and gut passage in the oyster Crassostrea gigas. Aquat. Living Resour. 20, 51–57. doi: 10.1051/alr:2007015
Laabir, M., Collos, Y., Masseret, E., Grzebyk, D., Abadie, E., Savar, V., et al. (2013). Influence of environmental factors on the paralytic shellfish toxin content and profile of Alexandrium catenella (Dinophyceae) isolated from the Mediterranean Sea. Mar. Drugs 11, 1583–1601. doi: 10.3390/md11051583
Laabir, M., Jauzein, C., Genovesi, B., Masseret, E., Grzebyk, D., Cecchi, P., et al. (2011). Influence of temperature, salinity and irradiance on the growth and cell yield of the harmful red tide dinoflagellate Alexandrium catenella colonizing Mediterranean waters. J. Plankton Res. 33, 1550–1563. doi: 10.1093/plankt/fbr050
LaJeunesse, T. C., Lambert, G., Andersen, R. A., Coffroth, M. A., and Galbraith, D. W. (2005). Symbiodinium (Pyrrhophyta) genome sizes (DNA content) are smallest among dinoflagellates. J. Phycol. 41, 880–886. doi: 10.1111/j.0022-3646.2005.04231.x
Le, Q. H., Markovic, P., Hastings, J. W., Jovine, R. V. M., and Morse, D. (1997). Structure and organization of the peridinin-chlorophyll a-binding protein gene in Gonyaulax polyedra. Mol. Gen. Genet. 255, 595–604. doi: 10.1007/s004380050533
Lewis, A. M., Coates, L. N., Turner, A. D., Percy, L., Lewis, J., Michael Lewis, A., et al. (2018). A review of the global distribution of Alexandrium minutum (Dinophyceae) and comments on ecology and associated paralytic shellfish toxin profiles, with a focus on Northern Europe. J. Phycol. 598, 581–598. doi: 10.1111/jpy.12768
Le Gac, M., Metegnier, G., Chomérat, N., Malestroit, P., Quéré, J., Bouchez, O., et al. (2016). Evolutionary processes and cellular functions underlying divergence in Alexandrium minutum. Mol. Ecol. 25, 5129–5143. doi: 10.1111/mec.13815
Lidie, K. B., Ryan, J. C., Barbier, M., and Van Dolah, F. M. (2005). Gene expression in Florida red tide dinoflagellate Karenia brevis: analysis of an expressed sequence tag library and development of DNA microarray. Mar. Biotechnol. 7, 481–493. doi: 10.1007/s10126-004-4110-6
Lin, S. (2011). Genomic understanding of dinoflagellates. Res. Microbiol. 162, 551–569. doi: 10.1016/j.resmic.2011.04.006
Lin, S., Cheng, S., Song, B., Zhong, X., Lin, X., Li, W., et al. (2015). The Symbiodinium kawagutii genome illuminates dinoflagellate gene expression and coral symbiosis. Science 350, 691–694. doi: 10.1126/science.aad0408
Lin, S., Zhang, H., and Gray, M. W. (2007). “RNA editing in Dinoflagellates and its implications for the evolutionary history of the editing machinery,” in RNA and DNA Editing: Molecular Mechanisms and Their Integration into Biological Systems, ed. H. C. Smith, (Hoboken, NJ: Wiley Online Library), 280–309. doi: 10.1002/9780470262269.ch13
Lin, S., Zhang, H., Spencer, D. F., Norman, J. E., and Gray, M. W. (2002). Molecular cloning and expression. J. Mol. Biol. 320, 727–739. doi: 10.1016/S0022-2836(02)00468-0
Liu, H., Stephens, T. G., González-Pech, R. A., Beltran, V. H., Lapeyre, B., Bongaerts, P., et al. (2018). Symbiodinium genomes reveal adaptive evolution of functions related to coral-dinoflagellate symbiosis. Commun. Biol. 1:95. doi: 10.1038/s42003-018-0098-3
Liu, L., and Hastings, J. W. (2005). Novel and rapidly diverging intergenic sequences between tandem repeats of the luciferase genes in seven dinoflagellate species. J. Phycol. 42, 96–103. doi: 10.1111/j.1529-8817.2006.00165.x
Loper, C. L., Steidinger, K. A., and Walker, L. M. (1980). A simple chromosome spread technique for unarmored Dinoflagellates and implications of polyploidy in algal cultures. Am. Microsc. Soc. 99, 343–346.
Lugliè, A., Giacobbe, M. G., Riccardi, E., Bruno, M., Pigozzi, S., Mariani, M. A., et al. (2017). Paralytic shellfish toxins and cyanotoxins in the mediterranean: new data from Sardinia and Sicily (Italy). Microorganisms 5:72. doi: 10.3390/microorganisms5040072
Mann, H. B., and Whitney, D. R. (1947). On a test of whether one of two random variables is stochastically larger than the other. Ann. Math. Stat. 18, 50–60. doi: 10.1214/aoms/1177730491
Marie, D., Simon, N., Guillou, L., Partensky, F., and Vaulot, D. (2000). DNA, RNA analysis of phytoplankton by flow cytometry. Curr. Protoc. Cytom. Chapter 11:Unit11.12.
Masselin, P., Amzil, Z., Abadie, E., Nézan, E., Le Bec, C., Carreras, A., et al. (2001). “Paralytic shellfish poisoning on the french mediterranean coast in autumn 1998 : Alexandrium “Tamarense complex” (Dinophyceae) as causative agent,” in Proceedings of the 9th International Conference on Harmful Algal Blooms, Hobart, Australia, 7–11 February 2000, Vol. 53, eds G. M. Hallegraeff, S. Blackburn, I, C. J. Bolch, and R. J. Lewis, (Paris: Intergovernmental Oceanographic Commission of UNESCO), 1689–1699. doi: 10.1088/1751-8113/44/8/085201
Masseret, E., Grzebyk, D., Nagai, S., Genovesi, B., Lasserre, B., Laabir, M., et al. (2009). Unexpected genetic diversity among and within populations of the toxic dinoflagellate Alexandrium catenella as revealed by nuclear microsatellite markers. Appl. Environ. Microbiol. 75, 2037–2045. doi: 10.1128/AEM.01686-08
McLean, T. I. (2013). “Eco-omics”: a review of the application of genomics, transcriptomics, and proteomics for the study of the ecology of harmful algae. Microb. Ecol. 65, 901–915. doi: 10.1007/s00248-013-0220-5
Mendoza-Flores, A., Leyva-Valencia, I., Band-Schmidt, C. J., Galindo-Sánchez, C. E., and Bustillos-Guzmán, J. J. (2018). Identification of the gene sxtA (Domains sxtA1 and sxtA4) in Mexican strains of Gymnodinium catenatum (Dinophyceae) and their evolution. Front. Mar. Sci. 5:289. doi: 10.3389/fmars.2018.00289
Mittag, M., Lee, D. H., and Hastings, J. W. (1994). Circadian expression of the luciferin-binding protein correlates with the binding of a protein to the 3’ untranslated region of its mRNA. Proc. Natl. Acad. Sci. U.S.A. 91, 5257–5261. doi: 10.1073/pnas.91.12.5257
Mittag, M., Li, L., and Woodland Hastings, J. (1998). The mRNA level of the circadian regulated Gonyaulax luciferase remains constant over the cycle. Chronobiol. Int. 15, 93–98. doi: 10.3109/07420529808998673
Montoya, N. G., Akselman, R., Carignan, M. O., and Carreto, J. I. (2006). Pigment profile and toxin composition during a red tide of Gymnodinium catenatum Graham and Myrionecta rubra (Lohman) Jankowski in coastal waters off Mar del Plata, Argentina. Afr. J. Mar. Sci. 28, 199–202. doi: 10.2989/18142320609504147
Moustafa, A., Loram, J. E., Hackett, J. D., Anderson, D. M., Plumley, F. G., and Bhattacharya, D. (2009). Origin of saxitoxin biosynthetic genes in cyanobacteria. PLoS One 4:e5758. doi: 10.1371/journal.pone.0005758
Mungpakdee, S., Shinzato, C., Takeuchi, T., Kawashima, T., Koyanagi, R., Hisata, K., et al. (2014). Massive gene transfer and extensive RNA editing of a symbiotic dinoflagellate plastid genome. Genome Biol. Evol. 6, 1408–1422. doi: 10.1093/gbe/evu109
Murray, S. A., Diwan, R., Orr, R. J. S., Kohli, G. S., and John, U. (2015). Gene duplication, loss and selection in the evolution of saxitoxin biosynthesis in alveolates. Mol. Phylogenet. Evol. 92, 165–180. doi: 10.1016/j.ympev.2015.06.017
Murray, S. A., Ruvindy, R., Kohli, G. S., Anderson, D. M., and Brosnahan, M. L. (2019). Evaluation of sxtA and rDNA qPCR assays through monitoring of an inshore bloom of Alexandrium catenella Group 1. Sci. Rep. 9:14532. doi: 10.1038/s41598-019-51074-3
Murray, S. A., Wiese, M., Neilan, B. A., Orr, R. J. S., de Salas, M., Brett, S., et al. (2012). A reinvestigation of saxitoxin production and sxtA in the “non-toxic” Alexandrium tamarense Group V clade. Harmful Algae 18, 96–104. doi: 10.1016/j.hal.2012.05.001
Murray, S. A., Wiese, M., Stüken, A., Brett, S., Kellmann, R., Hallegraeff, G., et al. (2011). SxtA-based quantitative molecular assay to identify saxitoxin-producing harmful algal blooms in marine waters. Appl. Environ. Microbiol. 77, 7050–7057. doi: 10.1128/AEM.05308-11
Nascimento, S. M., Purdie, D. A., Lilly, E. L., Larsen, J., and Morris, S. (2005). Toxin profile, pigment composition, and large subunit rDNA phylogenetic analysis of an Alexandrium minutum (Dinophyceae) strain isolated from the fleet lagoon, United Kingdom. J. Phycol. 41, 343–353. doi: 10.1111/j.1529-8817.2005.03088.x
Nguyen-Ngoc, L. (2004). An autecological study of the potentially toxic dinoflagellate Alexandrium affine isolated from Vietnamese waters. Harmful Algae 3, 117–129. doi: 10.1016/S1568-9883(03)00062-3
Penna, A., Perini, F., Dell’Aversano, C., Capellacci, S., Tartaglione, L., Giacobbe, M. G., et al. (2015). The sxt gene and paralytic shellfish poisoning toxins as markers for the monitoring of toxic Alexandrium species blooms. Environ. Sci. Technol. 49, 14230–14238. doi: 10.1021/acs.est.5b03298
Perini, F., Galluzzi, L., Dell’Aversano, C., Iacovo, E. D., Tartaglione, L., Ricci, F., et al. (2014). SxtA and sxtG gene expression and toxin production in the mediterranean Alexandrium minutum (Dinophyceae). Mar. Drugs 12, 5258–5276. doi: 10.3390/md12105258
Roy, S., and Morse, D. (2013). Transcription and maturation of mRNA in Dinoflagellates. Microorganisms 1, 71–99. doi: 10.3390/microorganisms1010071
Ruvindy, R., Bolch, C. J., MacKenzie, L., Smith, K. F., and Murray, S. A. (2018). qPCR assays for the detection and quantification of multiple paralytic shellfish toxin-producing species of Alexandrium. Front. Microbiol. 9:3153. doi: 10.3389/fmicb.2018.03153
Salois, P., and Morse, D. (1997). Characterization and molecular phylogeny of a protein kinase cDNA from the dinoflagellate Gonyaulax (Dinophyceae). J. Phycol. 33, 1063–1072. doi: 10.1111/j.0022-3646.1997.01063.x
Savela, H., Harju, K., Spoof, L., Lindehoff, E., Meriluoto, J., Vehniäinen, M., et al. (2016). Quantity of the dinoflagellate sxtA4 gene and cell density correlates with paralytic shellfish toxin production in Alexandrium ostenfeldii blooms. Harmful Algae 52, 1–10. doi: 10.1016/j.hal.2015.10.018
Savela, H., Spoof, L., Perala, N., Preede, M., Lamminma, U., Nybom, S., et al. (2015). Detection of cyanobacterial sxt genes and paralytic shellfish toxins in freshwater lakes and brackish waters on Aland Islands, Finland. Harmful Algae 46, 1–10. doi: 10.1016/j.hal.2015.04.005
Scott, J. (1995). A place in the world for RNA editing. Cell 81, 833–836. doi: 10.1016/0092-8674(95)90002-0
Shi, X., Zhang, H., and Lin, S. (2013). Tandem repeats, high copy number and remarkable diel expression rhythm of form II RuBisCO in Prorocentrum donghaiense (Dinophyceae). PLoS One 8:e71232. doi: 10.1371/journal.pone.0071232
Smith, G. P. (1976). Evolution of repeated DNA sequences by unequal crossover. Science 191, 528–535. doi: 10.1126/science.1251186
Stüken, A., Orr, R. J. S., Kellmann, R., Murray, S. A., Neilan, B. A., and Jakobsen, K. S. (2011). Discovery of nuclear-encoded genes for the neurotoxin saxitoxin in dinoflagellates. PLoS One 6:e20096. doi: 10.1371/journal.pone.0020096
Stüken, A., Riobó, P., Franco, J., Jakobsen, K. S., Guillou, L., and Figueroa, R. I. (2015). Paralytic shellfish toxin content is related to genomic sxtA4 copy number in Alexandrium minutum strains. Front. Microbiol. 6:404. doi: 10.3389/fmicb.2015.00404
Suikkanen, S., Kremp, A., Hautala, H., and Krock, B. (2013). Paralytic shellfish toxins or spirolides? The role of environmental and genetic factors in toxin production of Alexandrium ostenfeldii complex. Harmful Algae 26, 52–59. doi: 10.1016/j.hal.2013.04.001
Touzet, N., Franco, J. M., and Raine, R. (2007a). Characterization of nontoxic and toxin-producing strains of Alexandrium minutum (Dinophyceae) in Irish coastal waters. Appl. Environ. Microbiol. 73, 3333–3342. doi: 10.1128/AEM.02161-06
Touzet, N., Franco, J. M., and Raine, R. (2007b). Influence of inorganic nutrition on growth and PSP toxin production of Alexandrium minutum (Dinophyceae) from Cork Harbour, Ireland. Toxicon 50, 106–119. doi: 10.1016/j.toxicon.2007.03.001
Touzet, N., Franco, J. M., and Raine, R. (2008). PSP toxin analysis and discrimination of the naturally co-occurring Alexandrium tamarense and A. minutum (Dinophyceae) in Cork Harbour, Ireland. Aquat. Microb. Ecol. 51, 285–299. doi: 10.3354/ame01189
Van De Riet, J. M., Gibbs, R. S., Chou, F. W., Muggah, P. M., Rourke, W. A., Burns, G., et al. (2009). Liquid chromatographic post-column oxidation method for analysis of paralytic shellfish toxins in mussels, clams, scallops, and oysters: single-laboratory validation. J. AOAC Int. 92, 1690–1704.
Verma, A., Barua, A., Ruvindy, R., Savela, H., Ajani, P. A., and Murray, S. A. (2019). The genetic basis of toxin biosynthesis in Dinoflagellates. Microorganisms 7, 1–29. doi: 10.3390/microorganisms7080222
Vico, P., Aubriot, L., Martigani, F., Rigamonti, N., Bonilla, S., and Piccini, C. (2016). Influence of nitrogen availability on the expression of genes involved in the biosynthesis of saxitoxin and analogs in Cylindrospermopsis raciborskii. Harmful Algae 56, 37–43. doi: 10.1016/j.hal.2016.04.008
Wang, D. Z., Zhang, S. F., Zhang, Y., and Lin, L. (2015). Paralytic shellfish toxin biosynthesis in cyanobacteria and dinoflagellates: a molecular overview. J. Proteomics 135, 132–140. doi: 10.1016/j.jprot.2015.08.008
Wang, H., Guo, R., Lim, W. A., Allen, A. E., and Ki, J. S. (2020a). Comparative transcriptomics of toxin synthesis genes between the non-toxin producing dinoflagellate Cochlodinium polykrikoides and toxigenic Alexandrium pacificum. Harmful Algae 93:101777. doi: 10.1016/j.hal.2020.101777
Wang, H., Kim, H., and Ki, J. S. (2020b). Transcriptome survey and toxin measurements reveal evolutionary modification and loss of saxitoxin biosynthesis genes in the dinoflagellates Amphidinium carterae and Prorocentrum micans. Ecotoxicol. Environ. Saf. 195, 110474. doi: 10.1016/j.ecoenv.2020.110474
Wiese, M., D’Agostino, P. M., Mihali, T. K., Moffitt, M. C., and Neilan, B. A. (2010). Neurotoxic alkaloids: saxitoxin and its analogs. Mar. Drugs 8, 2185–2211. doi: 10.3390/md8072185
Wiese, M., Murray, S. A., Alvin, A., and Neilan, B. A. (2014). Gene expression and molecular evolution of sxtA4 in a saxitoxin producing dinoflagellate Alexandrium catenella. Toxicon 92, 102–112. doi: 10.1016/j.toxicon.2014.09.015
Woo, Y. H., Ansari, H., Otto, T. D., Linger, C. M. K., Olisko, M. K., Michálek, J., et al. (2015). Chromerid genomes reveal the evolutionary path from photosynthetic algae to obligate intracellular parasites. eLife 4:e6974. doi: 10.7554/eLife.06974
Yang, I., John, U., Beszteri, S., Glöckner, G., Krock, B., Goesmann, A., et al. (2010). Comparative gene expression in toxic versus non-toxic strains of the marine dinoflagellate Alexandrium minutum. BMC Genomics 11:248. doi: 10.1186/1471-2164-11-248
Yunes, J. S., De La Rocha, S., Giroldo, D., Silveira, S. B. D., Comin, R., Bicho, M. D. S., et al. (2009). Release of carbohydrates and proteins by a subtropical strain of raphidiopsis brookii (cyanobacteria) able to produce saxitoxin at three nitrate concentrations. J. Phycol. 45, 585–591. doi: 10.1111/j.1529-8817.2009.00673.x
Zauner, S., Greilinger, D., Laatsch, T., Kowallik, K. V., and Maier, U. G. (2004). Substitutional editing of transcripts from genes of cyanobacterial origin in the dinoflagellate Ceratium horridum. FEBS Lett. 577, 535–538. doi: 10.1016/j.febslet.2004.10.060
Zhang, C., and Lin, S. (2019). Initial evidence of functional siRNA machinery in dinoflagellates. Harmful Algae 81, 53–58. doi: 10.1016/j.hal.2018.11.014
Zhang, F., Xu, X., Li, T., and Liu, Z. (2013). Shellfish toxins targeting voltage-gated sodium channels. Mar. Drugs 11, 4698–4723. doi: 10.3390/md11124698
Zhang, H., and Lin, S. (2003). Complex gene structure of the form II Rubisco in the dinoflagellate Prorocentrum Minimum (Dinophyceae). J. Phycol. 1171, 1160–1171.
Zhang, Y., Zhang, S. F., Lin, L., and Wang, D. Z. (2014). Comparative transcriptome analysis of a toxin-producing dinoflagellate Alexandrium catenella and its non-toxic mutant. Mar. Drugs 12, 5698–5718. doi: 10.3390/md12115698
Zhang, Y., Zhang, S. F., Lin, L., and Wang, D. Z. (2017). Whole transcriptomic analysis provides insights into molecular mechanisms for toxin biosynthesis in a toxic dinoflagellate Alexandrium catenella (ACHK-T). Toxins (Basel) 9, 1–15. doi: 10.3390/toxins9070213
Keywords: Alexandrium, saxitoxins, sxtA4, copy number variation, sxtA, expression, isoform
Citation: Geffroy S, Lechat M-M, Le Gac M, Rovillon G-A, Marie D, Bigeard E, Malo F, Amzil Z, Guillou L and Caruana AMN (2021) From the sxtA4 Gene to Saxitoxin Production: What Controls the Variability Among Alexandrium minutum and Alexandrium pacificum Strains? Front. Microbiol. 12:613199. doi: 10.3389/fmicb.2021.613199
Received: 01 October 2020; Accepted: 03 February 2021;
Published: 24 February 2021.
Edited by:
Per Juel Hansen, University of Copenhagen, DenmarkReviewed by:
Brett A. Neilan, The University of Newcastle, AustraliaRussell John Scott Orr, University of Oslo, Norway
Dazhi Wang, Xiamen University, China
Copyright © 2021 Geffroy, Lechat, Le Gac, Rovillon, Marie, Bigeard, Malo, Amzil, Guillou and Caruana. This is an open-access article distributed under the terms of the Creative Commons Attribution License (CC BY). The use, distribution or reproduction in other forums is permitted, provided the original author(s) and the copyright owner(s) are credited and that the original publication in this journal is cited, in accordance with accepted academic practice. No use, distribution or reproduction is permitted which does not comply with these terms.
*Correspondence: Solène Geffroy, solene.geffroy@ifremer.fr