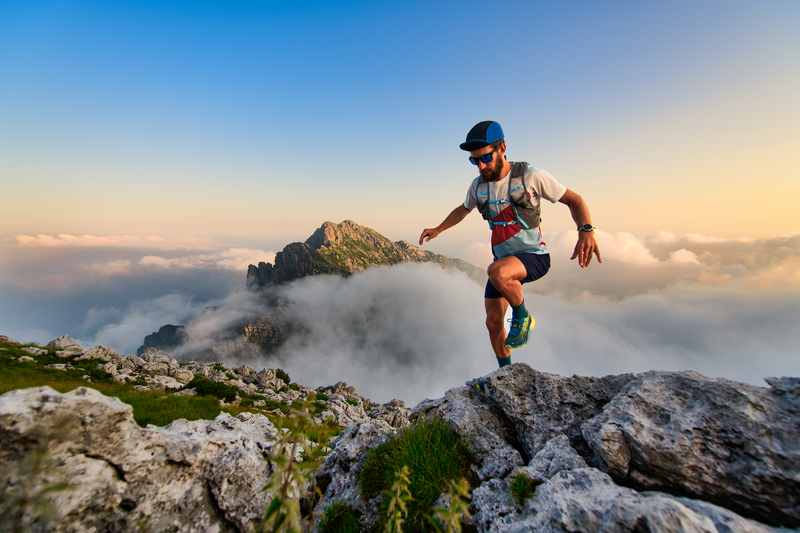
95% of researchers rate our articles as excellent or good
Learn more about the work of our research integrity team to safeguard the quality of each article we publish.
Find out more
ORIGINAL RESEARCH article
Front. Microbiol. , 26 February 2021
Sec. Evolutionary and Genomic Microbiology
Volume 12 - 2021 | https://doi.org/10.3389/fmicb.2021.566908
This article is part of the Research Topic New Insights on Botulism, Botulinum Neurotoxins, and Botulinum Toxin-producing Clostridia View all 10 articles
Of the seven currently known botulinum neurotoxin-producing species of Clostridium, C. parabotulinum, or C. botulinum Group I, is the species associated with the majority of human botulism cases worldwide. Phylogenetic analysis of these bacteria reveals a diverse species with multiple genomic clades. The neurotoxins they produce are also diverse, with over 20 subtypes currently represented. The existence of different bont genes within very similar genomes and of the same bont genes/gene clusters within different bacterial variants/species indicates that they have evolved independently. The neurotoxin genes are associated with one of two toxin gene cluster types containing either hemagglutinin (ha) genes or orfX genes. These genes may be located within the chromosome or extrachromosomal elements such as large plasmids. Although BoNT-producing C parabotulinum bacteria are distributed globally, they are more ubiquitous in certain specific geographic regions. Notably, northern hemisphere strains primarily contain ha gene clusters while southern hemisphere strains have a preponderance of orfX gene clusters. OrfX C. parabotulinum strains constitute a subset of this species that contain highly conserved bont gene clusters having a diverse range of bont genes. While much has been written about strains with ha gene clusters, less attention has been devoted to those with orfX gene clusters. The recent sequencing of 28 orfX C. parabotulinum strains and the availability of an additional 91 strains for analysis provides an opportunity to compare genomic relationships and identify unique toxin gene cluster characteristics and locations within this species subset in depth. The mechanisms behind the independent processes of bacteria evolution and generation of toxin diversity are explored through the examination of bacterial relationships relating to source locations and evidence of horizontal transfer of genetic material among different bacterial variants, particularly concerning bont gene clusters. Analysis of the content and locations of the bont gene clusters offers insights into common mechanisms of genetic transfer, chromosomal integration, and development of diversity among these genes.
Botulinum neurotoxins (BoNTs) are a worldwide public health issue and are listed as Tier 1 Select Agents due to their potential to pose a severe threat to human and animal health (Federal Select Agent Program Select Agents and Toxins List)1. The recent availability of genomes from over 250 BoNT-producing clostridial strains has provided new opportunities to gain perspective about the toxins and their toxin gene clusters, and the relationships of the strains that produce these toxins (Giordani et al., 2015; Mazuet et al., 2016; Williamson et al., 2016).
The neurotoxins are extremely diverse. They have historically been categorized according to the neutralizing ability of serotype-specific antisera (Peck et al., 2017). Seven toxin types (A-G) have been determined using these methods. Four of these serotypes, BoNT/A, BoNT/B, BoNT/E, and BoNT/F, have been definitively linked with human botulism. While these toxins show commonalities in protein structure and activity, genetic sequencing of the toxin serotypes has revealed that they differ by 35–70% in amino acid sequence. A second level of diversity is seen within these toxins; over 40 toxin subtypes have been currently identified having amino acid sequence differences of 2–36% (Peck et al., 2017). The subtypes are labeled with a number following the toxin type, such as BoNT/A1 or BoNT/F5. This diversity among BoNT proteins contrasts with tetanus toxin, (TeNT), which is closely related to the BoNTs in structure and mechanism of action but differs in both its singularity and a lack of non-toxic complex proteins.
The BoNT-producing bacteria are also diverse in their composition. Historically any bacteria that produced BoNTs was considered to be the species “Clostridium botulinum” based on the single characteristic of their neurotoxicity, and various species were instead differentiated into four Groups (I-IV) based on their biochemical and metabolic properties. In 1988, Group IV organisms were genetically confirmed to be the species Clostridium argentinense (Suen et al., 1988a) and additional BoNT-producing strains were identified and genetically confirmed to be members of Clostridium baratii and Clostridium butyricum (Suen et al., 1988b). This created a confusing hybrid nomenclature system involving both Group and species designations. However, genomic studies have now confirmed that BoNT-producing strains are represented in seven distinct species (Smith et al., 2018). Within this manuscript the bacteria will be designated by Latin binomials: C. parabotulinum (proteolytic C. botulinum Group I) (Seddon, 1922; Meyer and Gunnison, 1929); C. botulinum (non-proteolytic Group II) (Smith, 1977); C. novyi sensu lato (C. botulinum Group III) (Skarin et al., 2011); BoNT/G-producing C. argentinense; BoNT/F-producing C. baratii; BoNT/E-producing C. butyricum; and BoNT/B-producing C. sporogenes (Weigand et al., 2015; Williamson et al., 2016). Examples of both BoNT-producing and non-neurotoxigenic bacteria are found within each species. Table 1 lists information on the species, including the toxin types that they produce.
The discovery and study of BoNT-producing clostridia has a history spanning more than 100 years (van Ermengem, 1897; Landmann, 1904; Leuchs, 1910; Burke, 1919). C. parabotulinum bacteria are the most studied BoNT-producing species, as they produce toxins that are responsible for the vast majority of human botulism cases worldwide. They are most closely related to C. sporogenes (Hatheway, 1993; Collins and East, 1998; Stackebrandt et al., 1999), prompting some to consider them as variants of the same species. However, recent studies have indicated that they should be considered separate species (Weigand et al., 2015; Williamson et al., 2016).
The neurotoxins are naturally found in association with several non-toxic accessory proteins, known collectively as the toxin complex. The genes encoding these proteins are arranged in a cluster adjacent to the neurotoxin gene. There are two types of bont gene clusters, known as ha or orfX. The toxin gene clusters encode the neurotoxin protein and a variety of non-toxic proteins. Non-toxin/non-hemagglutinin (NTNH) proteins, expressed by the ntnh gene, are universally present in both types of toxin complexes. The ha gene clusters produce hemagglutinin proteins (HA70, HA17, and HA33) which form a complex that is directly linked to the NTNH protein. Within the orfX gene clusters are three open reading frames (orfX1, orfX2, orfX3) that have been shown to produce proteins that apparently form somewhat fragile toxin complexes (Mazuet et al., 2012; Lin et al., 2015; Kalb et al., 2017). The functions of these proteins are not presently known. Other genes that are found within the bont gene cluster include botR, a transcriptional regulator, and p47, whose protein was recently shown to share a structural domain with the OrfX2 and OrfX3 proteins and that is related to the TULIP family of lipid-binding proteins (Gustafsson et al., 2017; Lam et al., 2018).
Each botulinum neurotoxin gene (bont) is part of one or the other of the above gene clusters. For example, bont/B genes are always found within ha clusters, while bont/E genes are always associated with orfX gene clusters. The bont genes within BoNT/A1 strains are unique in that they can be located within either of the two gene clusters. The bont genes in BoNT/A5 strains, most BoNT/A1 strains, and all BoNT/B strains are located within the smaller (11.7 kb) ha toxin gene cluster (Collins and East, 1998; Carter et al., 2011), while a few bont/A1 genes, as well as bont/A2-A4 and bont/A6-A8 genes, and all bont/F genes are located within the larger (∼17 kb) orfX gene cluster (Luquez et al., 2005; Hill et al., 2009; Kull et al., 2015; Mazuet et al., 2016). In bivalent strains that express two toxins, such as BoNT/A2B5, BoNT/A1(B), and BoNT/B5F2 strains, both toxin gene clusters may be found within a single bacterial isolate. In those cases, the bont/A or bont/F genes are always within orfX gene clusters and the bont/B genes are within ha gene clusters (Hill et al., 2009; Kalb et al., 2017). The orfX gene clusters show a high degree of relatedness which contrasts with the variability of the associated toxin type or subtype. For example, gene clusters with identical or nearly identical orfX, botR, p47, and ntnh genes may contain bont genes with only ∼60% nucleotide identity.
C. parabotulinum strains are commonly isolated from environmental and clinical samples, particularly in association with human botulism cases. While C. parabotulinum strains are globally distributed, the distinct predominance of C. parabotulinum strains with orfX gene clusters in the southern hemisphere and, conversely, the predominance of C. parabotulinum having ha gene clusters in the northern hemisphere suggests a differential evolution of these bacteria and their toxin genes which may have been influenced by environmental factors.
Currently the genomes of over 150 C. parabotulinum strains have been publicly posted in the NCBI database, which provides an opportunity to examine genomic relationships and toxin gene cluster commonalities on a broad scale. An additional 28 orfX C. parabotulinum isolates that are mainly located in the southern hemisphere were sequenced and analyzed as part of this study. These genomes have provided an opportunity for in depth study of the relationships of this particular species subset that varies in its genomic characteristics, toxin genes and gene clusters, and geographic locations from their previously studied northern hemisphere counterparts.
A comprehensive approach was used that integrates molecular information and phylogeography in order to provide an understanding of these organisms from multiple perspectives, including an examination of the unique components, arrangements, and locations of their orfX bont gene clusters.
Genomic relationships among these orfX C. parabotulinum strains were analyzed and new insights associated with the components of their toxin gene clusters and associated co-located genes were revealed in this study. The plasmid and chromosomal locations of the bont gene clusters in these strains are dependent on both the species (C. parabotulinum) and the gene cluster type (orfX), and investigation of unique features within these bont gene clusters and surrounding genes suggests connections between the locations of these genes and the processes involved in their acquisition and chromosomal integration.
Source attributions of BoNT-producing clostridia have become more important as we seek to understand links between these bacteria and their effects on humans, domestic animals, and the environment. Toxin subtypes differ in their sensitivities to treatments, and a knowledge of the prevalent toxin types in various geographical regions is key to prevention and treatment strategies. An extensive review of the literature, to include over 60 journal articles and book chapters, was undertaken to provide information relating to the geographic diversity of BoNT/A and BoNT/F producing strains, toxin subtypes, and locations. NCBI records associated with BoNT-producing clostridia and unpublished records from various collections were also examined for sources related to additional strains.
Whole genome sequencing of the 28 newly sequenced isolates was conducted on Illumina MiSeq or GAIIx platforms. Briefly, genomes were assembled with a pipeline that incorporates Trimmomatic (v0.30) (Bolger et al., 2014), BayesHammer (Nikolenko et al., 2013), SPAdes (v3.7.1) (Bankevich et al., 2012), and Pilon 1.17 (Walker et al., 2014). Contaminating contigs were identified with BLAST (Altschul et al., 1990) alignments against known contaminants in the NCBI nucleotide database. Any contig associated with contamination or containing anomalously low average coverage was removed. Closely-related, publicly-available genome assemblies and sequencing read data were downloaded from the National Center for Biotechnology Information (NCBI). If only sequencing read data were available for a genome, an assembly was generated with the approach described above or with SPAdes only. Genome assemblies were annotated with Prokka v1.11 (Seemann, 2014). Genomic data has been deposited in the appropriate NCBI databases. Supplementary Table 1 provides information on all genomes in the study, including NCBI accession information, genome assembly statistics, and average nucleotide identity estimated with MASH (v2.2) (Ondov et al., 2016) compared to a reference genome (C. parabotulinum Kyoto-F – GCF_000022765.1).
Core-genome single nucleotide polymorphisms (SNPs) were called as described in Williamson et al. (2017). Sequencing reads were aligned to a reference genome, C. parabotulinum Kyoto-F (GCF_000022765.1), with BWA-MEM (v0.7.7) (Li, 2013) and SNPs were called with the Unified Genotyper method in GATK (v3.3) (McKenna et al., 2010; DePristo et al., 2011) within NASP (Sahl et al., 2016). If sequence reads were not available for a genome, reads were simulated from publicly-available genome assemblies with ART (MountRainier) (-ss MSv3 -l 250 -f 75 -m 300 -s 30) (Huang et al., 2012) in order to compare a uniform data type. SNPs were removed from the analysis if the depth of coverage was less than ten or if the allele proportion was less than 0.9. Duplicated regions of the reference genome were identified by a reference self-alignment with NUCmer (Delcher et al., 2002; Kurtz et al., 2004) and SNPs falling in these regions were filtered from all downstream analysis. Maximum likelihood phylogenies were generated from the resulting SNP matrices (bestsnps) with IQ-TREE (v1.4.4) (Nguyen et al., 2015) using the K3Pu F ASC G4 model. The consistency index and retention index were calculated with Phangorn. Trees were viewed in FigTree2.
Gene sequences and associated regions of orfX toxin gene clusters were extracted from Prokka output files for further analyses. BLASTn (Altschul et al., 1990) was used to identify and compare individual bont genes, toxin cluster genes and surrounding genes and intergenic sequences. The lycA genes and arsC genes (and pseudogenes) were aligned with MUSCLE (v3.8.31) (Edgar, 2004) and phylogenies were generated with IQ-TREE (v1.5.5) (Nguyen et al., 2015) using the following best-fit models (Kalyaanamoorthy et al., 2017): lycA gene sequences – TIM3 F G4, arsC gene sequences – TPM2u F R2.
Complete orfX BoNT/A and BoNT/F genomes were searched for evidence of bacteriophage DNA sequences using PHASTER (Zhou et al., 2011; Arndt et al., 2016). Complete, incomplete, and questionable phage sequences were located and compared in order to gain a better understanding of their movements and integration into chromosomes.
The occurrence of human and animal botulism is directly linked to exposure to BoNTs or BoNT-producing bacteria that are resident in the environment or have been introduced via exported foods or other materials. A knowledge of the predominant BoNT serotypes and subtypes among clostridia resident within specific geographic locations is critical to providing effective treatment options.
Bacteria that contain bont/A genes and produce BoNT/A are the most commonly isolated BoNT-producing strains. While the geographic distribution of BoNT/A strains is known to be worldwide (Fernandez, 1994; Williamson et al., 2016), environmental studies, literature reviews, and examinations of records in public databases and private collections have determined that BoNT/A subtypes exhibit specific geographic ranges.
The major BoNT/A subtype in the northern hemisphere is BoNT/A1. BoNT/A1 strains with ha gene clusters are widespread, but BoNT/A1 within orfX gene clusters are quite rare. Exceptions to this would be BoNT/A1(B) strains that contain the bont/A1 gene within an orfX gene cluster and a truncated bont/B gene within a complete ha gene cluster. These strains are commonly found within the United States (Dabritz et al., 2014; Raphael et al., 2014), and appear to be emerging pathogens in Japan (Kenri et al., 2014) and France (Mazuet et al., 2016).
In contrast, the predominant BoNT/A subtype in the southern hemisphere is BoNT/A2. BoNT/A2-producing C. parabotulinum are commonly found in the soils in Argentina (Luquez et al., 2005) and BoNT/A2 is the major causative agent in infant and foodborne botulism there (Sagua et al., 2009). Bacteria producing this toxin type are also found in Australia (McCallum et al., 2015) and they been associated with botulism cases in Africa (Mackay-Scollay, 1958; Smith et al., 1979; Frean et al., 2004; Luquez et al., 2012; Viray et al., 2014). Some C. parabotulinum strains are bivalent and contain bont/B6, bont/F4, or bont/F5 genes in addition to bont/A2 genes.
The first BoNT/A3-producing strain, known as the Loch Maree strain, was identified in 1922 in association with a foodborne botulism outbreak in Scotland (Leighton, 1922) and was considered to be unique. However, additional BoNT/A3 strains were isolated decades later in Argentina (Luquez et al., 2012) and recently there was an isolation of a BoNT/A3 strain in connection with a foodborne case in Slovakia (Mad’arova et al., 2017). C. parabotulinum strains containing bont/A4, bont/A5, bont/A6, bont/A7, and bont/A8 genes are all quite rare and have the commonality of source locations in the northern hemisphere.
The first BoNT/F-producing strain was isolated in Denmark in 1960 (Moller and Scheibel, 1960) and since then eight distinct BoNT/F subtypes have been identified. BoNT/F strains are comparatively rare and are associated with a variety of clostridial strains, including C. parabotulinum (BoNT/F1-F5 and BoNT/F8), C. botulinum (BoNT/F6), and C. baratii bacteria (BoNT/F7). They have a global distribution, with occasional strains being isolated in North and South America, and across Eurasia from Denmark and Italy to China. C. parabotulinum strains producing BoNT/F3, BoNT/F4, and BoNT/F5 have only been isolated from Argentina, while BoNT/F1, BoNT/F2 and BoNT/F8 producers are confined in location to the northern hemisphere.
Phylogenies based on multiple genomic analysis methods support earlier findings that several clostridial species are capable of harboring bont genes and producing BoNTs (Popoff, 1995; Collins and East, 1998; Hill et al., 2009; Weigand et al., 2015; Williamson et al., 2016). One of these species, C. parabotulinum, includes a range of genomic variants. Strains containing ha and orfX bont gene clusters are dispersed throughout the C. parabotulinum phylogeny (Williamson et al., 2016). The genomes of 28 strains containing bont/A and/or bont/F genes within orfX gene clusters were sequenced as part of this study, and compared with 91 additional OrfX C. parabotulinum genomes using a core genome SNP phylogeny (consistency index with only parsimony informative SNPs – 0.43, retention index – 0.90) that was generated from an alignment of 119,955 SNP positions called from a core alignment of 1,714,645 positions. The core genome SNP analysis did not include SNPs within the toxin cluster genes or within plasmids, as they are not conserved across all genomes.
Examination of the core genome SNP phylogeny identified several defined clades within the orfX C. parabotulinum strains (Figure 1). One of these is a conserved clade composed of subclades containing closely related genomes from strains that were isolated from soils or foodborne botulism cases in northwest Argentina, and a few European strains. Within this major clade are three subclades: one subclade containing 37 genomes having bont/A2 gene clusters, one subclade with six genomes having bont/A2 and bont/F4 gene clusters, and one subclade with five genomes containing bont/A3 gene clusters. Notably, while BoNT/A2F4 strains form a highly conserved clade that is closely related to Argentinean BoNT/A2 and BoNT/A3 strains, the bacteria that contain only bont/F4 genes are in a distinct, unrelated cluster of clades. Among these 48 strains, 41 were isolated from a specific region in Argentina. The close relationship of these lineages coupled with their common geographic location is an indication that these strains may have evolved from a single ancestral strain and subsequently acquired different toxin genes. The bont genes in these BoNT/A2 strains and several of the BoNT/A3 strains are located within the chromosome, which provides for a greater genetic stability than genes that are within extrachromosomal elements.
Figure 1. Core genome SNP phylogeny of C. parabotulinum strains with orfX toxin clusters. The bont gene types, gene cluster locations, and geographic locations of the strains are color coded according to the legend. Additional information is listed in Supplementary Table 1 with the genomes in the order they appear in this figure.
While one of the BoNT/A2-producing strains represented in this clade (Kyoto-F) was isolated from an infant botulism case in Japan, it is known that this case was associated with the ingestion of honey and that the honey was likely imported from Argentina (personal communication, Dr. Shunji Kozaki), providing a geographic link between these strains.
Two separate conserved clades that contain genomes of BoNT/A1(B) strains also exhibit localized geographic ranges. For example, in the largest clade of BoNT/A1(B) isolates 19 of 24 strains were located in the United States, four were from Japan and one was from Ecuador, while the smaller clade contained genomes from one Italian and three Japanese strains. With one exception, the strains within the two BoNT/A1(B) clades were isolated in the northern hemisphere.
A more variable clade contains Australian BoNT/A2 strains and one BoNT/A2B6 strain. While the bont/A2 genes in these strains are located within the chromosome, bont/B6 genes are universally located within large conjugative plasmids in both C. parabotulinum and C. sporogenes strains. The existence of bont/B6 genes within plasmids in multiple clostridial species illustrates cross-species transfer of toxin genes by introduction via their plasmids.
Two African BoNT/A2 strains are part of a second variable genomic clade that includes Italian BoNT/A2 strains and BoNT/F8 It 357. Two subclades containing BoNT/F1 isolates, and BoNT/F4 strains plus the lone BoNT/F3 isolate indicate a relationship with the African strains. The Mexican isolate containing bont/A6 and bont/B1 genes is an outlier within this clade that is somewhat related to the BoNT/F3 and BoNT/F4 strains.
Rare BoNT/A1 strains having orfX bont gene clusters are found within one of two clades. One isolate from Australia is part of a clade that contains seven Australian BoNT/A2 strains, while two OrfX BoNT/A1 strains that are part of the “bivalent toxin” clade are from the United States and Argentina. Genomes from BoNT/A3 strains also sort into two clades according to location (Argentina and Scotland). While the Argentinian isolates show a definite relationship with geographically related Argentinean BoNT/A2 strains, the Scottish BoNT/A3 strain shows a relationship with the unusual Italian BoNT/A2B7 It 92 strain.
Genomes containing bont/A2 genes form part of a final variable clade that also contains genomes of bivalent BoNT/A2B5, BoNT/B5A4, and BoNT/B5F2 strains, as well as several BoNT/F5 strains and the two OrfX BoNT/A1 strains previously mentioned. These strains have a global geographic range; many of its members are sourced from Argentina, but locations also include Sweden, Italy, and the United States. With the exception of the BoNT/A2 and OrfX BoNT/A1 strains in this clade, the toxin genes within these genomes are located within large, highly conserved plasmids.
Within this phylogeny are examples of conserved clades containing geographically co-located strains having the same bont genes and gene clusters; conserved clades containing different bont genes; and variable clades containing unrelated strains having same bont genes. While the conserved clades emphasize an ability for a particular strain to become established in a certain region, the variable clades are indications that global movements of these strains do occur, and that transported strains are capable of exchanging their toxin genes with a variety of strain variants and species. While bont gene diversity is facilitated by the movement of extrachromosomal plasmids from strain to strain, stability of the genes may be ensured by subsequent integration into the chromosome. Novel toxins have been formed through homologous recombination events that place new toxin genes or gene fragments into existing bont gene clusters and, on a lesser scale, through single nucleotide mutations. A study of the composition and location of orfX bont genes and gene clusters aids in the understanding of the processes involved in gene cluster movements and integrations.
Toxin gene clusters in C. parabotulinum strains containing ha bont/A1, bont/B, and bont/A5 genes are arranged in the following order: ha70-ha17-ha33-botR-ntnh-bont. Gene clusters from all other C. parabotulinum strains show the following arrangement: orfX3-orfX2-orfX1-botR-p47-ntnh-bont. Adjacent genes include the lycA gene and, in some cases, arsC genes.
OrfX bont gene clusters may be located within large, highly conserved plasmids or they may be present as pathogenicity islands (PAIs) within the chromosome. PAIs are gene clusters linked to virulence factors that endow the bacteria with pathogenic properties. They are derived from mobile genetic elements, such as conjugative plasmids or bacteriophage, and are subsequently integrated into the chromosome (Davis and Waldor, 2002). Within the chromosome, PAIs are typically present as discreet genetic units of 10–100 kb distinguished by a lower G + C content than the surrounding genomic DNA. They are often flanked by direct repeat sequences and mobility genes, such as IS elements, integrases, and transposases (Hacker et al., 1997). These general PAI characteristics are in agreement with those of bont gene clusters.
It is interesting to note that the non-toxic accessory genes within orfX toxin clusters show remarkable conservation, despite their association with multiple bont/A and bont/F genes. A discontiguous BLASTn comparison of the entire toxin gene cluster sequence (minus the toxin gene) from the BoNT/F1 Langeland strain results in 94–100% identity with gene clusters from over 60 strains located worldwide that contain bont/A2, bont/A3, bont/HA, bont/F1, bont/F3, bont/F4, and bont/F5 genes. This high degree of toxin gene cluster identity ends at the terminal 50 nucleotides of the ntnh gene, where a homologous recombination (HR) event has placed bont/A neurotoxin genes within the bont/F gene cluster, or vice versa (Figure 2A). This is similar to a recombination event described within the ntnh gene that inserted the bont/A1 gene into the ha bont/B toxin gene cluster, producing the ha bont/A1 gene cluster (Hill et al., 2009).
Figure 2. Homologous recombination (HR) events that have exchanged or altered bont genes/gene clusters, resulting in the generation of diverse toxin subtypes. (A) C-terminal sequence of selected orfX ntnh genes showing homologous and disparate sequences that illustrate the recombination event placing bont/A2/A3 genes within bont/F gene clusters, or vice versa. Sequences showing complete identity are in black font, bont/A-specific sequences are in blue font, bont/F sequences are in green font, and single mutational differences are shown in red font. (B) Comparisons of bont/F2:bont/F3 and bont/F1:bont/F8 gene sequences showing areas of identity, possibly gained through HR events, and regions where sequences differ (shaded in gray).
HR events within bont genes have also been responsible for the generation of novel toxin subtypes. It is known that the bont/A2 gene is a mosaic of the bont/A1 and bont/A3 genes (Hill et al., 2007), and comparisons of bont/F genes as part of this study has revealed HR events among these subtypes as well. The bont/F2 and bont/F3 genes show greater than 99% identity until the final 470 nucleotides, where the identity decreases to 97.24%, indicating an HR event has occurred at its 3’ terminus. Similarly, the bont/F1 and bont/F8 genes show >99% identity throughout the 5’ half of the gene and also at final third of its nucleotide sequence; however, between these closely related DNA sections the percent identity decreases to slightly more than 90%, again signaling an HR event has occurred (Figure 2B).
The orfX bont gene clusters are found at four distinct genomic locations – three sites within the chromosome and one within plasmids (Figure 3). The locations are identified by their proximity to specific genes. Chromosomally located orfX bont/A1, bont/A2, bont/F1, and bont/F8 gene clusters are co-located with the ars operon, while bont/F3 and bont/F4 gene clusters are inserted between fragments of a split pulE gene (Dover et al., 2013; Smith et al., 2020). Plasmid-borne orfX bont gene clusters and chromosomal bont/A3 gene clusters are universally adjacent to HepA/SNF2, thermonuclease, and DNA helicase genes.
Figure 3. Chromosomal and plasmid C. parabotulinum orfX bont gene cluster locations. The sites are numbered according to their base pair location within the BoNT/A3 SU1169 genome and plasmid pCBG from BoNT/A2B5 CDC 1436.
The lycA gene, while not historically considered a part of the toxin gene cluster, is universally situated alongside orfX gene clusters. It is found in two locations: 1) following the bont gene with chromosomally located orfX toxin gene clusters that are co-located with the ars operon and 2) prior to the orfX3 gene within plasmid-borne toxin gene clusters and chromosomally located bont/A3, bont/F3 and bont/F4 toxin gene clusters, where the ars operon is remotely located.
The lycA genes are highly conserved, with two basic variants that correspond to their locations relative to the bont and ars gene clusters, and are also generally linked to their chromosomal or plasmid location. A phylogeny comparing lycA gene sequences (Figure 4) illustrates these relationships. Nucleotide differences between the two lycA gene variants are approximately 6%, while differences of less than 2% are seen within the two variants.
Figure 4. Dendrogram showing the relationship between bont/A and bont/F lycA gene sequences located within the chromosome and plasmids. Chromosomally located lycA genes are in black font and plasmid-borne genes are in blue font. Two major lycA variants with nucleotide differences of ∼6% can be discerned. The tree is midpoint rooted.
The lycA gene product is a lysozyme that is homologous to lytic proteins found in Lactobacillus and Streptococcus pneumoniae bacteriophage (Henderson et al., 1977). These bacteriophage proteins lyse the bacterial cell walls, releasing phage during their lytic cycles. In similar fashion, the lycA lysozyme may be responsible for bacterial autolysis and subsequent release of toxin in proteolytic C. parabotulinum bacteria (Bonventre and Kempe, 1960; Dineen et al., 2002).
It should be noted that intact lycA genes are only found among orfX gene clusters from members of C. parabotulinum. The lycA gene’s ubiquitous presence adjacent to this toxin cluster and the role its protein may play in toxin dissemination suggests that it could be considered a component of orfX toxin gene clusters.
Chromosomally located orfX bont gene clusters, with the exception of those containing bont/A3, bont/F3 or bont/F4, are co-located with the ars operon genes. The ars operon encodes proteins that confer resistance against inorganic arsenite that is found in anaerobic environments. The complete ars system (arsR, arsD, arsA, arsB, arsC, arsM) is present in most C. parabotulinum strains and provides the most efficient removal of arsenic, but operons that are devoid of some ars genes appear to provide a minimal level of protection (Lindstrom et al., 2009). The ars genes are found both within the chromosome and within mobile extrachromosomal elements in a wide variety of bacteria and are known to be involved in horizontal gene transfer events (Andres and Bertin, 2016). However, with BoNT-producing clostridia, the ars operon appears to be strictly located within the chromosome.
Up to three copies of arsC genes form part of this operon (Dineen et al., 2004; Hill et al., 2009). The three arsC genes are identified here as arsC1, arsC2, or arsC3 according to their locations relative to the bont and ars gene clusters. The arsC1 gene is adjacent to the orfX3 gene in the bont gene cluster, arsC2 is found at the beginning of the ars gene cluster, and arsC3, when present, is located within the ars gene cluster. These genes vary in size, ranging from 337 to 392 bp with arsC1 and 366–417 bp with arsC2. The arsC genes appear to have deteriorated in some cases, forming pseudogenes. A phylogeny comparing the arsC1, arsC2, and arsC3 genes shows that the arsC1 genes and the arsC2 genes are each located within two distinct clades, while the arsC3 genes form a single clade (Figure 5).
Figure 5. Dendrogram comparing sequences from arsC1, arsC2, and arsC3 genes associated with selected BoNT/A and BoNT/F toxin clusters. The ars genes are numbered 1, 2, or 3 according to their locations in relation to the bont gene cluster. Sequences from strains that contain all three arsC genes are shown in green font, sequences in blue font are from strains with arsC1 and arsC2 sequences, and sequences in black font are from strains with arsC2 only. The arsC3 gene differs by ∼20% in nucleotide residues from arsC1 and arsC2. The tree is midpoint rooted.
All three arsC genes are generally observed in strains containing chromosomally located bont/A2 genes and with orfX bont/A1 strain AM303. Where all three arsC genes are present, each arsC variant is nearly identical in sequence and the arsC1 and arsC2 genes are closely related, having greater than 96% identity. The arsC3 gene is less closely related, with ∼80% identity when compared to arsC1 or arsC2. In these genomes the ars operon follows the bont gene cluster (Figure 6A). However, with orfX bont/A1 toxin gene clusters in BoNT/A1(B) strains and OrfX BoNT/A1 strains CDC 297 and SU0729; bont/F1 gene clusters; the bont/F8 gene cluster; and a few bont/A2 toxin gene clusters the arsC3 gene is missing and the location has been re-arranged so that the ars operon now precedes arsC1 and the bont gene cluster (Figures 6B–G). Genomes that lack arsC3 genes show greater variability within their arsC1 and arsC2 genes and their arsC1 genes are within a distinct clade that differs by 6–7% from the clade in genomes having three arsC variants. In strains that lack both arsC1 and arsC3, such as BoNT/A3, BoNT/F3, and BoNT/F4 strains, bont gene clusters are inserted into the chromosome at sites that are remote from the ars genes.
Figure 6. Arrangements of conserved genes surrounding chromosomally located bont gene clusters that are adjacent to the ars operon. Conserved genes are numbered 1–31 with #9–16 representing the bont gene cluster (colored red with the lycA gene in green) and #17–24 representing the ars operon (colored blue). Supplementary Table 2 lists the genes/encoded proteins corresponding to the numbers shown in Figure 6. Conserved genes that flank this location are colored orange; conserved hypothetical genes are gray; and non-conserved hypothetical genes are black. Panel (A) represents an arrangement where the ars operon is located downstream from the bont gene cluster while panels (B–G) represent arrangements where the ars operon precedes the bont gene cluster. Strains representing these arrangements include: (A) most Argentinean and Australian BoNT/A2 strains; (B) BoNT/F1 strains; (C) OrfX BoNT/A1 strains; (D) BoNT/A1(B) strains; (E) African BoNT/A2 strains; (F) Italian BoNT/A2 trains; and (G) Italian BoNT/F8 It 357.
While the bont genes and gene arrangements immediately surrounding the bont gene clusters vary, the same conserved genes (#1–7 and #27–31 in Figure 6) flank these regions, placing them at a common location within the chromosome. Figures 6E–G show arrangements where arsC1 and arsC2 are present but several other ars genes are absent, an indication of diminished arsenic resistance in these strains. Supplementary Table 2 lists the genes/encoded proteins corresponding to the numbers shown in Figure 6.
The re-arrangement of the bont gene cluster related to the ars operon indicates an evolutionary shift, as does the loss of several ars genes seen with the arrangements of bont gene clusters in African and Italian strains. The arrangement in OrfX BoNT/A1 and BoNT/A1(B) strains suggests their bont/A1 gene clusters may have evolved from a common ancestor, but several distinct genes that are lacking in the OrfX BoNT/A1 strains (Figure 6B) have been inserted upstream from the bont/A1 gene cluster in the BoNT/A1(B) strains (Figure 6C), indicating an evolutionary divergence. Similarly, the arrangements in the African and Italian strains (Figures 6D,E, respectively) show a clear ancestral relationship, but additional genes have been inserted between the ars and bont cluster genes in the Italian strains, again signaling an evolutionary divergence. While these bont gene clusters show relationships within their non-toxin bont cluster genes and chromosomal integration arrangements, the strains that harbor these genes are often unrelated, emphasizing the differential evolution of the bacteria and the bont genes.
As bont/F3 and bont/F4 gene clusters are remotely located (∼250 kb – 1.1 mb) from ars operons that lack both arsC1 and arsC3 genes, the chromosomal integration process differs from strains that contain bont/A2 and bont/F1 gene clusters. The insertion process for the bont/F3 and bont/F4 gene clusters involves excision of a pulE gene with insertion of bont gene cluster DNA within the pulE gene (Figure 7; Dover et al., 2013; Smith et al., 2020). The pulE gene is one of 15 genes that encode the pul secreton, which is type II secretion system for proteases and toxins in gram negative bacteria. This mechanism is similar to the insertion mechanisms for bont/E gene clusters that involve a split rarA gene (Hill et al., 2009) and bont/F6 genes, where the split gene is topB (Carter et al., 2013); however, while a second intact rarA or topB gene is seen in these insertions, there is no duplicate intact pulE gene adjacent to the inserted bont/F3 or bont/F4 gene clusters. In clostridia the pulE gene may be non-essential, as most of the remaining genes encoding proteins that form this secretion system are absent. Figure 7 shows the arrangement of the genes present within and flanking the split pulE gene for BoNT F3 SU0160 and multiple BoNT F4 strains, and the intact pulE gene in BoNT F5 strains, where the bont gene cluster resides within a plasmid. With both the bont/F3 and bont/F4 gene clusters the inserted genetic material between the two pulE gene fragments contains, in addition to the bont gene cluster, a 35 kb DNA fragment that includes a PHP domain protein gene, a KAP family p-loop domain protein gene, a helix-turn-helix protein gene (#5–7 in Figures 7B,C), and several hypothetical proteins. A complete listing of the genes/encoded proteins numbered in Figure 7 is shown in Supplementary Table 3. However, with the bont/F3 gene cluster, an additional 57 kb DNA fragment encoding an intact bacteriophage is present (Smith et al., 2020). The significance of this finding is as yet unknown. An interesting finding is a similar split pulE gene within the BoNT/A3 Loch Maree strain which is devoid of bont cluster genes but instead contains an intact prophage sequence that is distinct from the one associated with the bont/F3 gene cluster (Dover et al., 2013; Smith et al., 2020), marking this as a general site for integration of prophage sequences as well as bont gene clusters.
Figure 7. Illustration of the integration location of chromosomally located bont/F3 and bont/F4 cluster genes, which is distinct from the ars operon location. At this site the bont gene clusters in panels (B,C) (#9–16, shown in red with the lycA gene in green) are inserted within a split pulE gene (#4 and 4’, purple). Supplementary Table 3 lists the genes/encoded proteins corresponding to the numbers shown in Figure 7. Additional conserved known genes are colored orange and conserved hypothetical genes are in black. Transposase genes are in light green. (A) Insertion region in BoNT F5 BrDura showing a complete pulE gene and an absence of the bont gene cluster. (B) The same region in BoNT F4 SU1425, showing an approximately 35 kb region between the truncated segments of the pulE gene. (C) The 92.5 kb DNA sequence in BoNT F3 SU0160 with the identical sequence found in BoNT F4 plus an inserted segment containing an intact bacteriophage.
While most of the bacterial strains analyzed here contain bont genes within the chromosome, several strains contain one or more bont genes within large (∼250–280 kb) conserved conjugative plasmids. Characteristics of the plasmids for which complete sequence information is known are shown in Table 2. The strains that harbor plasmid-borne bont genes are relatively rare, contain a wider variety of orfX bont/A and bont/F genes, and are found in genomes that are within variable phylogenetic clades. Strains containing these plasmids have been isolated in North and South America, Europe, Asia, and Australia, indicating an ease with which these plasmids may spread among globally located strains. The ability of these plasmids to move between different clostridial strains and species has also been confirmed through laboratory experiments (Marshall et al., 2010).
These plasmids may contain one or two toxin gene clusters, located at two distinct sites. One site exclusively contains ha bont/B gene clusters and the second site contains orfX bont/A or bont/F gene clusters (Figure 3). Plasmids that contain only one bont gene cluster (bont/A3, bont/F5, or bont/B gene clusters) are known as well as those with two bont gene clusters (bont/F2 plus bont/B5 genes or bont/A2, bont/A4, or bont/A6 paired with bont/B genes). A search for plasmid gene sequences within C. parabotulinum using BLASTn indicates that, with one exception, all of these related large C. parabotulinum plasmids contain bont genes and, because of their common toxin cluster locations within the plasmid, none contain both bont/A and bont/F gene clusters together.
Identical bont genes and gene clusters have been located either within plasmids or the chromosome of C. parabotulinum strains, which confirms the ability of these plasmid-borne genes to integrate into the chromosome and also affords us the opportunity to study the extent of exchanged genetic material. This duplicate location of bont clusters in the chromosome or plasmids is not specific to orfX C. parabotulinum strains. Gene clusters containing bont/B1 or bont/B2 genes have been identified that are located within either the chromosome or plasmids (Franciosa et al., 2009), as well as bont/E1, bont/E3, and bont/E10 genes (Zhang et al., 2013; Carter et al., 2016) and bont/F7 genes (Halpin et al., 2017; Mazuet et al., 2017).
BLASTn analysis of complete plasmids from twelve of these strains show 95–100% identity over large segments of the plasmid sequence, illustrating the close relationships among them (Table 3). However, they also show evidence of continuing insertion, deletion, and inversion events. For example, the plasmid from strain An436 (LFON01000008) that contains bont/B5 and bont/F2 gene clusters is closely related to the strain 657 plasmid (pCLJ) that contains bont/B5 and bont/A4, but a large (99 kb) deletion can be seen and, in addition, the bont/F2 gene cluster is reversed in orientation compared to the rest of its plasmid DNA (Hill et al., 2009).
In fact, the orientation of the bont gene cluster in relation to the surrounding genes varies from plasmid to plasmid. The bont/A gene cluster (#1–8 in Figure 8) in the BoNT/A2B3 It 87 strain, BoNT/A2B5 CDC 1436, BoNT/A6B1 CDC 41370, and BoNT/B5A4 strains is in the same orientation as the surrounding genes (Figures 8A,B), but with BoNT/A2B7 It 92, BoNT/A2F5 or BoNT/F5 strains, and BoNT/A3 Loch Maree the genes are in the opposite orientation from the surrounding genes (Figures 8C–F). The factors that govern the orientation of bont gene clusters are not understood; however, the presence of transposes adjacent to the lycA gene with most of these gene clusters suggests a possible role for them in gene integration and orientation.
Figure 8. Arrangements of conserved genes surrounding orfX bont gene clusters that are located within plasmids. Conserved genes representing the bont gene cluster are numbered #1–9, colored red with the lycA gene in green. Conserved genes that flank this location are colored orange, including the HepA/SNF, Tnase, and DNA helicase genes (#9–11); conserved hypothetical genes are gray; and non-conserved hypothetical genes are black. Supplementary Table 4 lists the genes/encoded proteins corresponding to the numbers shown in Figure 8. Panels (A,B) illustrate arrangements where the bont gene cluster is in the same orientation as the surrounding genes, while panels (C–F) show arrangements where the bont genes are in opposite orientation. Examples of these arrangements are found with (A) the bont/A2 gene cluster in strain BoNT/A2B3 It 87; (B) the bont/A2 gene cluster in BoNT/A2B5 CDC 1436; (C) the bont/F2 gene cluster in BoNT/B5F2 An436; (D) the bont/A2 gene cluster in BoNT/A2B7 It 92; (E) the bont/F5 gene cluster in BoNT/A2F5 BrDura, BoNT/F5 SU0634F, and BoNT/A2F4F5 AF84; and (F) the bont/A3 gene cluster in BoNT/A3 Loch Maree.
In the plasmid-borne orfX bont cluster regions, the gene sequences that are downstream of the bont gene cluster are well-conserved, showing that all orfX bont clusters are located in the same position within the plasmid, regardless of the bont gene present. The lycA and bont clusters are followed by HepA/SNF2, which encodes a DNA helicase protein, plus thermonuclease (Tnase), and an additional DNA helicase gene (#9–11 in Figure 8). However, the upstream sequences are arranged in multiple patterns.
The upstream genes in the BoNT A2b3 It 87 strain differ from those in other plasmids and contain over a dozen unique hypothetical genes (Figure 8A). Several transposases flank the upstream and downstream genes. A conserved upstream gene sequence arrangement is seen with BoNT/A2b3 CDC 1436, BoNT/B5a4 657, and BoNT/A6b1 CDC 41370 strains that includes AraC family transcriptional regulators, a methionine adenosyltransferase gene, the chaperone ClpB gene, a cytidine deoxyribosyltransferase gene, and a thiamine biosynthesis protein gene (#14–20 in Figures 8B,C). These strains are geographically related, having been isolated in the southwestern U.S. and Mexico. A similar arrangement is seen in the North American BoNT/B5F2 strains.
A different arrangement is seen in the Italian A2b7 It 92 strain and strains containing bont/F5 genes where the upstream genes have been replaced with five unique genes (genes encoding a single strand binding protein gene, a DNA polymerase III subunit gene, and A-type inclusion proteins) (#21–24 in Figures 8D,E). Variable numbers of transposase genes and genes encoding hypothetical proteins are also present within these regions.
The upstream region of the plasmid bont/A3 gene cluster in the Loch Maree strain consists of nine unique hypothetical genes and genes encoding a putative ABC transporter lipoprotein and an adenylate and guanylate cyclase protein (#27 and #28 in Figure 8F). This region is similar to, but not identical with, that of the bont/A3 gene cluster region in strain SU0972. A complete listing of the numbered genes/encoded proteins in Figure 8 is shown in Supplementary Table 4.
The conserved downstream gene sequences identify a common orfX bont gene cluster location within the plasmid but the varied upstream DNA sequences may provide some clues as to why these bont gene clusters have not integrated into the chromosome. The HepA/SNF2, Tnase, and DNA helicase genes are also present in the absence of orfX bont gene clusters in plasmids that contain only ha bont/B clusters. Their presence may be an indication of the past existence of orfX bont gene clusters at that site or an opportunity for possible future acquisitions. Similar to the “chicken or the egg” quandary, it is not definitively known whether these bont gene clusters originated within plasmids followed by integration into the chromosome, whether the opposite is true, or whether movements in both directions is possible.
An understanding of the gene components and arrangements surrounding identical bont/A2 and bont/A3 gene clusters within the chromosome and plasmids presents an opportunity to examine the extent of genetic material that is exchanged within these genetic regions and potentially identify genes or insertion elements that may aid in bont gene cluster integration. When chromosomal and plasmid bont gene cluster regions of bont/A2 gene clusters are compared, only the lycA and bont gene cluster sequences are found to be present in both locations, so that the 17 kb of material transferred between the plasmid and chromosome is strictly composed of the lycA/bont gene cluster (Figure 9). In this figure, the bont gene cluster genes are colored red and the lycA gene is green. Known genes that are unique to each site are colored in purple in Figure 9, while conserved known genes are in orange, conserved hypothetical genes are in gray or black. Individual genes are identified in Supplementary Tables 2,4, as numbered in Figure 6A (BoNT/A2 chromosome) and Figure 8B (BoNT/A2 plasmid).
Figure 9. Illustrations of conserved DNA sequences that are shared between chromosomal and plasmid located bont clusters. With BoNT A2 strains, conserved sequences comprise only the actual bont cluster and lycA genes, while the conserved sequence with BoNT A3 strains includes additional surrounding genes. The bont cluster genes are colored red, the lycA gene is green, and other conserved known genes are orange. Genes that are unique to chromosomal or plasmid-borne bont/A2 gene clusters are shown in purple. Conserved hypothetical genes that are common to both plasmid-borne bont/A2 and plasmid and chromosomally located bont/A3 are in gray and conserved hypothetical genes that are exclusive to bont/A3 are in black. Specific gene identifications are listed in Supplementary Tables 2, 4 and are related to Figure 6A (bont/A2 chromosome), 8B (bont/A2 plasmid), and 8F (bont/A3 chromosome and plasmid).
However, with BoNT/A3 strains the sequence that has been transferred is comprised of a 35 kb highly conserved region surrounding the inverted bont/A3 gene cluster which includes two known genes and nine conserved hypothetical genes that are located upstream of the bont/A3 gene cluster, and the downstream HepA/SNF, Tnase, and DNA helicase genes. Individual genes surrounding the bont/A3 gene clusters are identified in Supplementary Table 4, as numbered in Figure 8F (BoNT/A3 chromosome and plasmid).
SNF family proteins contain helicase-like domains and often reside within large multi-protein complexes that may facilitate various DNA manipulations (Ryan and Owen-Hughes, 2011). Thus, it is possible that the proteins encoded by the HepA/SNF2 and DNA helicase genes may function as facilitators for the transfer of the bont/A3 gene cluster from plasmid to chromosome, or vice versa. While the plasmid-borne bont/A2 and bont/A3 gene clusters are located at the same site, these gene clusters are differentially placed at discrete sites within the chromosome implying interaction processes involving distinct target and facilitating genes.
The bont/A2 gene clusters are commonly found within the chromosome, and only in rare cases are they located within plasmids. As there is an association between chromosomally located bont/A2 gene clusters and arsC genes, an examination of the ars gene cluster regions within the genomes of several strains having plasmid-borne bont/A2 gene clusters (BoNT/A2B3 It87, BoNT/A2B5 CDC 1436, and BoNT/A2B7 It92) was undertaken. The chromosomally located ars gene operons in these strains contained arsC2 genes, however, none contained the arsC1 or arsC3 genes, which provides an indication by omission of the possible involvement of the arsC1 genes in the movement of bont/A2 gene clusters between plasmid and chromosome.
Similarly, the sequence regions surrounding the bont/A3 gene cluster within the chromosome of BoNT/A3 SU1169 were compared with the same region in BoNT/A3 Loch Maree, where the bont/A3 gene cluster is found within a large plasmid. The Loch Maree chromosome is missing a 44.7 kb region found within SU1169 that includes the entire ∼35 kb region that is conserved among chromosomal and plasmid-borne bont/A3 clusters plus additional genes, hinting that the as-yet-unknown facilitators for chromosomal insertion of the bont/A3 gene might be located within the 45 kb region that is absent in the Loch Maree strain. Further study of these DNA sequences is needed to enable a better understanding of how their bont/A3 gene clusters are excised from plasmids and integrated into the chromosome.
Both intact and incomplete bacteriophages are commonly found among the BoNT-producing clostridia. Intact active phages have been recovered from mitomycin C-induced lysed cultures from BoNT/A, BoNT/B, BoNT/E, and BoNT/F strains (Eklund et al., 1969). In addition, it is well known that the genes for type C and D toxins are associated with bacteriophage DNA, that “curing” of these bacteria of its phage results in a reversion from toxic to non-toxic status, and that subsequent infection with isolated phage preparations reactivate its toxicity (Eklund and Poysky, 1974). Bacteriophage are also known to be vehicles for the general transfer of PAIs.
Prophage DNA sequences have also been located within the genomes of numerous orfX C. parabotulinum organisms. A search for phage sequences in 15 complete orfX BoNT/A and BoNT/F genomes using PHASTER (Zhou et al., 2011; Arndt et al., 2016) uncovered more than 19 intact phages within the chromosomes, and numerous incomplete phage sequences were discovered within both the chromosome and the large highly conserved plasmids among these strains. The majority of the identified phages were found within Argentinean BoNT/A2, BoNT/A3, and BoNT/F strains and were related to phages associated with either Clostridium difficile or Clostridium tetani (Table 4). Some phages associated with Clostridium sporogenes and Clostridium tyrobutyricum were also identified. The additional finding of shared DNA sequences with prophage fragments from the phage Clostr_c_st (the phage that contains bont/C and bont/D gene clusters) in the C. parabotulinum bont-containing plasmids is not surprising, as these shared sequences were generally linked to non-toxic genes within bont gene clusters. However, some conserved transposases were noted as well.
Table 4. A sampling of bacteriophages found within orfX + BoNT/A and BoNT/F C. parabotulinum strains.
A bacteriophage associated with Bacillus megaterium, Bacilli_Moonbeam, was found adjacent to the bont/F3 gene cluster in the genome of BoNT/F3 SU0160. This is a unique association of an intact phage sequence with the bont cluster of an orfX C. parabotulinum strain, as in all other cases complete prophage DNA sequences are remotely located from the bont gene cluster. However, it is not known if the bont/F3 gene cluster is actually part of the prophage, or if they are simply co-located.
Individual genes related to incomplete phage sequences have also been identified that are scattered among the conserved regions surrounding many bont clusters within both the chromosome and plasmids. These may represent remnants of ancient phages that previously integrated into the chromosome, possibly in association with bont gene clusters, or they may indicate that the present day conjugative plasmids responsible for the movement of bont gene clusters may have originated as bacteriophages where certain phage-specific genes have deteriorated while elements necessary for conjugation and bont genes have persisted. The significance of these bacteriophages may be underappreciated as contributors to overall genetic diversity in these bacteria through the placement of foreign genes into the chromosome via HGT.
Clostridium parabotulinum is a diverse species in their geographic range, in their genomic properties, and in their neurotoxins. BoNT-producing C. parabotulinum bacteria have a global distribution but are mainly found in temperate and subarctic regions in both the northern and southern hemispheres. It is noteworthy that, while the majority of C. parabotulinum strains isolated in the northern hemisphere harbor bont gene clusters containing ha genes, in the southern hemisphere the bont gene clusters predominantly contain orfX genes. As clostridia are spore-forming bacteria, this discrepancy in distribution may be due to differential movements of their bacterial spores. East-west movements among strains containing similar bont gene clusters, which could primarily be due to transport of bacterial spores on upper level wind currents, may be more common than north-south movements, which are more likely the results of carriage of spores by migrating birds or due to transfers of contaminated soil, plants, or foodstuffs.
There is evidence that certain geographic areas have provided conditions conducive to the widespread colonization of phylogenetically related bacterial strains, or clonal expansions. However, naturally occurring or manmade movements of bacterial spores from these areas have provided opportunities for these bacteria to become established in faraway regions and allowed for exchanges of novel genetic material between different bacterial strains via horizontal gene transfers (HGT). Comparisons of genomes and bont gene cluster sequences reveals that closely related strains have acquired different bont genes, often within related bont gene clusters, while unrelated strains may contain identical bont genes/gene clusters. The closely related strains isolated in northwest Argentina that contain bont/A2, bont/A2F4, or bont/A3 genes might be considered clonal expansions, while the finding of phylogenetically diverse C. parabotulinum strains located in Argentina, Africa, and Australia that contain identical bont/A2 gene clusters is an example of the apparent movements of these strains with subsequent exchanges of genetic material among them. Phylogenetic analysis of the genomes representing a subset of C. parabotulinum strains that contain orfX gene clusters demonstrates these relationships, revealing the presence of several clades that are highly conserved, contrasting with others that are quite variable.
HGT events may be accomplished following active infection by bacteriophage or by movement of genes that are incorporated into mobile genetic elements such as temperate prophage DNA or conjugative plasmids. All three mechanisms may have been utilized in the movement of toxin genes among the BoNT-producing clostridia, but with C. parabotulinum strains the majority of such transfers appear to involve conjugative plasmids. However, bacteriophage gene remnants are frequently found adjacent to bont gene clusters within the chromosomes and extrachromosomal plasmids. These remnants contain DNA sequences that encode various viral components, including recombinases, integrases, and transposases. It is interesting to speculate that perhaps these large plasmids have originated from bacteriophage that subsequently lost DNA sequences encoding vital structural genes, so that ancient bacteriophages may have evolved to become modern conjugative plasmids.
Once the plasmid-borne bont gene clusters have established a presence in a bacterial strain, they may proceed to integrate into their host chromosome and/or exchange genetic material with existing bont genes or gene clusters. This is accomplished through homologous recombination (HR) events that involve alignment of paired gene sequences, excision of a particular gene or intergenic sequence, and insertion of a new or exchanged DNA sequence. HR events within ntnh genes have placed orfX bont/A1 genes within ha bont/B gene clusters, and bont/A2 genes within bont/F1 gene clusters, and they are responsible for some of the toxin subtype diversity seen among C. parabotulinum strains. It is known that the bont/A2 gene resulted from an HR event involving bont/A1 and bont/A3 genes, and there is evidence of HR interactions between bont/F2 and bont/F3 and bont/F1 and bont/F8 that may have shaped their individual genetic identities.
Chromosomal integrations of entire bont gene clusters also utilize HR processes, including the necessity for paired genes or intergenic sequences. For example, integrations of bont/A2 and bont/F1 gene clusters, that occur at a common site within the chromosome, are dependent on paired arsC1 and arsC2 genes. Chromosomal integration provides a more stable location within the bacteria than extrachromosomal locations - it has been shown both experimentally and through natural occurrence that extrachromosomal DNA is subject to deletion through plasmid loss or “curing” of bacteriophage, while loss of chromosomally-located bont genes has yet to be definitively demonstrated.
It is thought that movement of bont gene clusters into non-neurotoxigenic clostridia is a one-way process that occurs via introduction within extrachromosomal plasmids followed by chromosomal integration. Previously published information and results from analysis of bont gene cluster locations in this study provide some evidence for this hypothesis. The ease of movement of plasmids containing bont genes among C. parabotulinum has been demonstrated in the laboratory and plasmid losses, with subsequent reversion to non-neurotoxicity, have also been noted. With the exception of a few very recently published genomes, there is no evidence of the persistence of these large plasmids after loss of their bont genes. While identical bont gene clusters have been located within either plasmids or the chromosome, the majority of C. parabotulinum strains (∼90%) contain chromosomally located bont gene clusters, indicating an ease of integration and/or selective pressure to do so. In strains where bont genes remain within extrachromosomal plasmids, targeting or facilitating genes that are necessary for chromosomal integration appear to be lacking. For example, strains having chromosomally located bont/A2 gene clusters contain paired arsC genes (arsC1 and arsC2) but in strains where bont/A2 genes are extrachromosomal, arsC1 genes are missing.
However, a question arises as to whether chromosomally integrated genes may be excised from the chromosome and re-inserted into the plasmid. In some cases, there is evidence of deterioration of the facilitating genes or IS elements that may have participated in chromosomal integration, so that use of these facilitators to reverse the integration process is not possible. In others, the plasmids have been lost. There are very few examples where bont gene clusters are located within the chromosome but an extracellular plasmid remains. In BoNT/A2F5 strains and the Australian BoNT/A2B6 strain AM526, bont/F5 and bont/B6 gene clusters remain within the plasmids but the bont/A2 gene clusters are within the chromosome. In the former case, the bont/F5 gene cluster inhabits the plasmid site where the bont/A2 gene would be located, presumably blocking re-integration, but in the BoNT/A2B6 strain, the HepA/SNF site is present in the plasmid. It is not possible to determine if the chromosomally located bont/A2 gene cluster in the BoNT/A2B6 strain could be re-integrated into the plasmid or not, as currently only one such strain has been isolated. The rarity of isolates where there is even a possibility for plasmid re-integration suggests chromosomal integration of bont gene clusters is essentially a one-way process, but further investigations are needed to confirm or refute this hypothesis.
While the genomic diversity seen within this species may have evolved partly through individual genetic mutations that over time have resulted in minor changes in genes, the major evolutionary driver behind genomic diversity is likely wholesale movements of individual genes, gene clusters, and large segments of DNA via horizontal gene transfers and recombination events. Analysis of recently sequenced orfX C. parabotulinum genomes from southern hemisphere bacterial collections have added to our overall knowledge about the BoNT-producing clostridia, increased our understanding of the diversity seen among these strains, and provided insights into mechanisms behind the generation of this diversity. Bacterial strains have been transported across oceans and continents using various environmental means and interactions between diverse strains have produced novel genomic variants. Over time, gene transfers and exchanges have shaped bont gene diversity. While these observations are focused on a particular subset of a single bacterial species, insights may be applied to the study of diversity within other clostridial species and possibly other bacteria as well.
NCBI accession information for all genomic data presented in this study is available in the Supplementary Material.
TS performed analyses and wrote the manuscript. CW performed analyses and edited the manuscript. JS edited the manuscript and guided genomic analyses. KH, SJ, FA, BA, RF, PC, and PK contributed strains/DNA/genomic sequencing and source information for strains. All authors reviewed the manuscript.
The work was supported in part by the U.S. Department of Homeland Security Science and Technology Directorate via award HSHQDC-16-C-B0013.
The authors declare that the research was conducted in the absence of any commercial or financial relationships that could be construed as a potential conflict of interest.
The Supplementary Material for this article can be found online at: https://www.frontiersin.org/articles/10.3389/fmicb.2021.566908/full#supplementary-material
Altschul, S. F., Gish, W., Miller, W., Myers, E. W., and Lipman, D. J. (1990). Basic local alignment search tool. J. Mol. Biol. 215, 403–410. doi: 10.1016/S0022-2836(05)80360-2
Andres, J., and Bertin, P. N. (2016). The microbial genomics of arsenic. FEMS Microbiol. Rev. 40, 299–322. doi: 10.1093/femsre/fuv050
Arndt, D., Grant, J. R., Marcu, A., Sajed, T., Pon, A., Liang, Y., et al. (2016). PHASTER: a better, faster version of the PHAST phage search tool. Nucleic Acids Res. 44, W16–W21. doi: 10.1093/nar/gkw387
Bankevich, A., Nurk, S., Antipov, D., Gurevich, A. A., Dvorkin, M., Kulikov, A. S., et al. (2012). SPAdes: a new genome assembly algorithm and its applications to single-cell sequencing. J. Comput. Biol. 19, 455–477. doi: 10.1089/cmb.2012.0021
Bolger, A. M., Lohse, M., and Usadel, B. (2014). Trimmomatic: a flexible trimmer for Illumina sequence data. Bioinformatics 30, 2114–2120. doi: 10.1093/bioinformatics/btu170
Bonventre, P. F., and Kempe, L. L. (1960). Physiology of toxin production by Clostridium botulinum types A and B. I. Growth, autolysis, and toxin production. J. Bacteriol. 79, 18–23.
Carter, A. T., Austin, J. W., Weedmark, K. A., and Peck, M. W. (2016). Evolution of chromosomal Clostridium botulinum type E neurotoxin gene clusters: evidence provided by their rare plasmid-borne counterparts. Genome Biol. Evol. 8, 540–555. doi: 10.1093/gbe/evw017
Carter, A. T., Pearson, B. M., Crossman, L. C., Drou, N., Heavens, D., Baker, D., et al. (2011). Complete genome sequence of the proteolytic Clostridium botulinum type A5(B3’) strain H04402 065. J. Bacteriol. 193, 2351–2352.
Carter, A. T., Stringer, S. C., Webb, M. D., and Peck, M. W. (2013). The type F6 neurotoxin gene cluster locus of group II Clostridium botulinum has evolved by successive disruption of two different ancestral precursors. Genome Biol. Evol. 5, 1032–1037. doi: 10.1093/gbe/evt068
Collins, M. D., and East, A. K. (1998). Phylogeny and taxonomy of the food-borne pathogen Clostridium botulinum and its neurotoxins. J. Appl. Microbiol. 84, 5–17.
Dabritz, H. A., Hill, K. K., Barash, J. R., Ticknor, L. O., Helma, C. H., Dover, N., et al. (2014). Molecular epidemiology of infant botulism in California and elsewhere, 1976-2010. J. Infect. Dis. 210, 1711–1722.
Davis, B. M., and Waldor, M. K. (2002). “Mobile genetic elements and bacterial pathogenesis,” in Mobile DNA II, ed. N. I. Craig (Washington, DC: ASM Press).
Delcher, A. L., Phillippy, A., Carlton, J., and Salzberg, S. L. (2002). Fast algorithms for large-scale genome alignment and comparison. Nucleic Acids Res. 30, 2478–2483. doi: 10.1093/nar/30.11.2478
DePristo, M. A., Banks, E., Poplin, R., Garimella, K. V., Maguire, J. R., Hartl, C., et al. (2011). A framework for variation discovery and genotyping using next-generation DNA sequencing data. Nat. Genet. 43, 491–498. doi: 10.1038/ng.806
Dineen, S. S., Bradshaw, M., and Johnson, E. A. (2002). Neurotoxin gene clusters in Clostridium botulinum type A strains: sequence comparison and evolutionary implications. Curr. Microbiol. 46, 345–352.
Dineen, S. S., Bradshaw, M., Karasek, C. E., and Johnson, E. A. (2004). Nucleotide sequence and transcriptional analysis of the type A2 neurotoxin gene cluster in Clostridium botulinum. FEMS Microbiol. Lett. 235, 9–16. doi: 10.1016/j.femsle.2004.04.002
Dover, N., Barash, J. R., Hill, K. K., Davenport, K. W., Teshima, H., Xie, G., et al. (2013). Clostridium botulinum strain Af84 contains three neurotoxin gene clusters: bont/A2, bont/F4 and bont/F5. PLoS One 8:e61205. doi: 10.1371/journal.pone.0061205
Edgar, R. C. (2004). MUSCLE: multiple sequence alignment with high accuracy and high throughput. Nucleic Acids Res. 32, 1792–1797. doi: 10.1093/nar/gkh340
Eklund, M. W., and Poysky, F. T. (1974). Interconversion of type C and D strains of Clostridium botulinum by specific bacteriophages. Appl. Microbiol. 27, 251–258.
Eklund, M. W., Poysky, F. T., and Boatman, E. S. (1969). Bacteriophages of Clostridium botulinum types A, B, E, and F and nontoxigenic strains resembling type E. J. Virol. 3, 270–274.
Fernandez, R. A. (1994). Distribution and Prevalence of Clostridium botulinum in Argentina. Implication in Human and Animal Pathology. Ph.D. thesis Universidad Nacional de Cuyo, Mendoza.
Franciosa, G., Maugliani, A., Scalfaro, C., and Aureli, P. (2009). Evidence that plasmid-borne botulinum neurotoxin type B genes are widespread among Clostridium botulinum serotype B strains. PLoS One 4:e4829. doi: 10.1371/journal.pone.0004829
Frean, J., Arntzen, L., van den Heever, J., and Perovic, O. (2004). Fatal type A botulism in South Africa, 2002. Trans. R. Soc. Trop. Med. Hyg. 98, 290–295. doi: 10.1016/S0035-9203(03)00069-5
Giordani, F., Fillo, S., Anselmo, A., Palozzi, A. M., Fortunato, A., Gentile, B., et al. (2015). Genomic characterization of Italian Clostridium botulinum group I strains. Infect. Genet. Evol. 36, 62–71. doi: 10.1016/j.meegid.2015.08.042
Gustafsson, R., Berntsson, R. P., Martinez-Carranza, M., El Tekle, G., Odegrip, R., Johnson, E. A., et al. (2017). Crystal structures of OrfX2 and P47 from a Botulinum neurotoxin OrfX-type gene cluster. FEBS Lett. 591, 3781–3792. doi: 10.1002/1873-3468.12889
Hacker, J., Blum-Oehler, G., Muhldorfer, I., and Tschape, H. (1997). Pathogenicity islands of virulent bacteria: structure, function and impact on microbial evolution. Mol. Microbiol. 23, 1089–1097. doi: 10.1046/j.1365-2958.1997.3101672.x
Halpin, J. L., Hill, K., Johnson, S. L., Bruce, D. C., Shirey, T. B., Dykes, J. K., et al. (2017). Finished whole-genome sequences of Clostridium butyricum toxin subtype E4 and Clostridium baratii toxin subtype F7 strains. Genome Announc. 5:e00375-17. doi: 10.1128/genomeA.00375-17
Hatheway, C. L. (1993). “Bacteriology and pathology of neurotoxigenic clostridia,” in Botulinum and Tetanus Neurotoxins: Neurotransmission and Biomedical Aspects, ed. B. R. DasGupta (New York, NY: Plenum Press), 491–503.
Henderson, I., Davis, T., Elmore, M., and Minton, N. P. (1977). “The genetic basis of toxin production in Clostridium botulinum and Clostridium tetani,” in The Clostridia: Molecular Biology and Pathogenesis, ed. J. Rood (New York, NY: Academic Press), 261–294.
Hill, K. K., Smith, T. J., Helma, C. H., Ticknor, L. O., Foley, B. T., Svensson, R. T., et al. (2007). Genetic diversity among Botulinum Neurotoxin-producing clostridial strains. J. Bacteriol. 189, 818–832. doi: 10.1128/JB.01180-06
Hill, K. K., Xie, G., Foley, B. T., Smith, T. J., Munk, A. C., Bruce, D., et al. (2009). Recombination and insertion events involving the botulinum neurotoxin complex genes in Clostridium botulinum types A, B, E and F and Clostridium butyricum type E strains. BMC Biol. 7:66. doi: 10.1186/1741-7007-7-66
Huang, W., Li, L., Myers, J. R., and Marth, G. T. (2012). ART: a next-generation sequencing read simulator. Bioinformatics 28, 593–594. doi: 10.1093/bioinformatics/btr708
Kalb, S. R., Baudys, J., Smith, T. J., Smith, L. A., and Barr, J. R. (2017). Characterization of Hemagglutinin negative Botulinum progenitor toxins. Toxins 9:193. doi: 10.3390/toxins9060193
Kalyaanamoorthy, S., Minh, B. Q., Wong, T. K. F., von Haeseler, A., and Jermiin, L. S. (2017). ModelFinder: fast model selection for accurate phylogenetic estimates. Nat. Methods 14, 587–589. doi: 10.1038/nmeth.4285
Kenri, T., Sekizuka, T., Yamamoto, A., Iwaki, M., Komiya, T., Hatakeyama, T., et al. (2014). Genetic characterization and comparison of Clostridium botulinum isolates from botulism cases in Japan between 2006 and 2011. Appl. Environ. Microbiol. 80, 6954–6964. doi: 10.1128/AEM.02134-14
Kull, S., Schulz, K. M., Weisemann, J., Kirchner, S., Schreiber, T., Bollenbach, A., et al. (2015). Isolation and functional characterization of the novel Clostridium botulinum neurotoxin A8 subtype. PLoS One 10:e0116381. doi: 10.1371/journal.pone.0116381
Kurtz, S., Phillippy, A., Delcher, A. L., Smoot, M., Shumway, M., Antonescu, C., et al. (2004). Versatile and open software for comparing large genomes. Genome Biol. 5:R12. doi: 10.1186/gb-2004-5-2-r12
Lam, K. H., Qi, R., Liu, S., Kroh, A., Yao, G., Perry, K., et al. (2018). The hypothetical protein P47 of Clostridium botulinum E1 strain Beluga has a structural topology similar to bactericidal/permeability-increasing protein. Toxicon 147, 19–26. doi: 10.1016/j.toxicon.2017.10.012
Landmann, G. (1904). Ueber die ursache der Darmstadter bohnenvergiftung. Hyg. Rundschau 14, 449–452.
Leighton, G. (1922). Botulism and Food Preservation (the Loch Maree Tragedy). London: W. Collins Sons & Co.
Leuchs, J. (1910). Beitraege zur kenntnis des toxins und antitoxins des Bacillus botulinus. Z. Hyg. Infektionskr. 76, 55–84.
Li, H. (2013). Aligning sequence reads, clone sequences and assembly contigs with BWA-MEM. arXiv [Preprint]. Available online at: http://arxiv.org/abs/1303.3997 (accessed July 20, 2019).
Lin, G., Tepp, W. H., Bradshaw, M., Fredrick, C. M., and Johnson, E. A. (2015). Immunoprecipitation of native botulinum neurotoxin complexes from Clostridium botulinum subtype A strains. Appl. Environ. Microbiol. 81, 481–491. doi: 10.1128/AEM.02817-14
Lindstrom, M., Hinderink, K., Somervuo, P., Kiviniemi, K., Nevas, M., Chen, Y., et al. (2009). Comparative genomic hybridization analysis of two predominant Nordic group I (proteolytic) Clostridium botulinum type B clusters. Appl. Environ. Microbiol. 75, 2643–2651. doi: 10.1128/AEM.02557-08
Luquez, C., Bianco, M. I., de Jong, L. I., Sagua, M. D., Arenas, G. N., Ciccarelli, A. S., et al. (2005). Distribution of botulinum toxin-producing clostridia in soils of Argentina. Appl. Environ. Microbiol. 71, 4137–4139. doi: 10.1128/AEM.71.7.4137-4139.2005
Luquez, C., Raphael, B. H., Joseph, L. A., Meno, S. R., Fernandez, R. A., and Maslanka, S. E. (2012). Genetic diversity among Clostridium botulinum strains harboring bont/A2 and bont/A3 genes. Appl. Environ. Microbiol. 78, 8712–8718. doi: 10.1128/AEM.02428-12
Mad’arova, L., Dorner, B. G., Schaade, L., Donath, V., Avdicova, M., Fatkulinova, M., et al. (2017). Reoccurrence of botulinum neurotoxin subtype A3 inducing food-borne botulism, Slovakia, 2015. Euro Surveill. 22:30591. doi: 10.2807/1560-7917.ES.2017.22.32.30591
Marshall, K. M., Bradshaw, M., and Johnson, E. A. (2010). Conjugative botulinum neurotoxin-encoding plasmids in Clostridium botulinum. PLoS One 5:e11087. doi: 10.1371/journal.pone.0011087
Mazuet, C., Ezan, E., Volland, H., Popoff, M. R., and Becher, F. (2012). Toxin detection in patients’ sera by mass spectrometry during two outbreaks of type A botulism in France. J. Clin. Microbiol. 50, 4091–4094.
Mazuet, C., Legeay, C., Sautereau, J., Bouchier, C., Criscuolo, A., Bouvet, P., et al. (2017). Characterization of Clostridium baratii type F strains responsible for an outbreak of botulism linked to beef meat consumption in France. PLoS Curr. 9:ecurrents.outbreaks.6ed2fe754b58a5c42d0c33d586ffc606. doi: 10.1371/currents.outbreaks.6ed2fe754b58a5c42d0c33d586ffc606
Mazuet, C., Legeay, C., Sautereau, J., Ma, L., Bouchier, C., Bouvet, P., et al. (2016). Diversity of Group I and II Clostridium botulinum strains from France including recently identified subtypes. Genome Biol. Evol. 8, 1643–1660. doi: 10.1093/gbe/evw101
McCallum, N., Gray, T. J., Wang, Q., Ng, J., Hicks, L., Nguyen, T., et al. (2015). Genomic epidemiology of Clostridium botulinum isolates from temporally related cases of infant botulism in new South Wales, Australia. J. Clin. Microbiol. 53, 2846–2853. doi: 10.1128/JCM.00143-15
McKenna, A., Hanna, M., Banks, E., Sivachenko, A., Cibulskis, K., Kernytsky, A., et al. (2010). The genome analysis toolkit: a MapReduce framework for analyzing next-generation DNA sequencing data. Genome Res. 20, 1297–1303. doi: 10.1101/gr.107524.110
Meyer, K. F., and Gunnison, J. B. (1929). South African cultures of Clostridium botulinum and parabotulinum. XXXVII with a description of Cl. botulinum type D, N. SP. J. Infect. Dis. 45, 106–118.
Moller, V., and Scheibel, I. (1960). Preliminary report on the isolation of an apparently new type of Cl. botulinum. Acta Pathol. Microbiol. Scand. 48:80.
Nguyen, L. T., Schmidt, H. A., von Haeseler, A., and Minh, B. Q. (2015). IQ-TREE: a fast and effective stochastic algorithm for estimating maximum-likelihood phylogenies. Mol. Biol. Evol. 32, 268–274. doi: 10.1093/molbev/msu300
Nikolenko, S. I., Korobeynikov, A. I., and Alekseyev, M. A. (2013). BayesHammer: bayesian clustering for error correction in single-cell sequencing. BMC Genomics 14(Suppl. 1):S7. doi: 10.1186/1471-2164-14-S1-S7
Ondov, B. D., Treangen, T. J., Melsted, P., Mallonee, A. B., Bergman, N. H., Koren, S., et al. (2016). Mash: fast genome and metagenome distance estimation using MinHash. Genome Biol. 17:132. doi: 10.1186/s13059-016-0997-x
Peck, M. W., Smith, T. J., Anniballi, F., Austin, J. W., Bano, L., Bradshaw, M., et al. (2017). Historical perspectives and guidelines for Botulinum neurotoxin subtype nomenclature. Toxins 9:38. doi: 10.3390/toxins9010038
Popoff, M. R. (1995). “Ecology of neurotoxigenic strains of clostridia,” in Clostridial Neurotoxins, The Molecular Pathogenesis of Tetanus and Botulism, ed. C. Montecucco (Berlin: Springer-Verlag).
Raphael, B. H., Shirey, T. B., Luquez, C., and Maslanka, S. E. (2014). Distinguishing highly-related outbreak-associated Clostridium botulinum type A(B) strains. BMC Microbiol. 14:192. doi: 10.1186/1471-2180-14-192
Ryan, D. P., and Owen-Hughes, T. (2011). Snf2-family proteins: chromatin remodellers for any occasion. Curr. Opin. Chem. Biol. 15, 649–656. doi: 10.1016/j.cbpa.2011.07.022
Sagua, M. D., Luquez, C., Barzola, C. P., Bianco, M. I., and Fernandez, R. A. (2009). Phenotypic characterization of Clostridium botulinum strains isolated from infant botulism cases in Argentina. Rev. Argent. Microbiol. 41, 141–147.
Sahl, J. W., Lemmer, D., Travis, J., Schupp, J. M., Gillece, J. D., Aziz, M., et al. (2016). NASP: an accurate, rapid method for the identification of SNPs in WGS datasets that supports flexible input and output formats. Microb. Genom. 2:e000074. doi: 10.1099/mgen.0.000074
Seddon, H. R. (1922). Bulbar paralysis in cattle due to the action of a toxicogenic bacillus, with a discussion on the relationship of the condition to forage poisoning (botulism). J. Comp. Pathol. Ther. 35, 147–190.
Seemann, T. (2014). Prokka: rapid prokaryotic genome annotation. Bioinformatics 30, 2068–2069. doi: 10.1093/bioinformatics/btu153
Skarin, H., Hafstrom, T., Westerberg, J., and Segerman, B. (2011). Clostridium botulinum group III: a group with dual identity shaped by plasmids, phages and mobile elements. BMC Genomics 12:185. doi: 10.1186/1471-2164-12-185
Smith, D. H., Timms, G. L., and Refai, M. (1979). Outbreak of botulism in Kenyan nomads. Ann. Trop. Med. Parasitol. 73, 145–148. doi: 10.1080/00034983.1979.11687241
Smith, L. D. (1977). Botulism: The organism, its Toxins, the Disease. Springfield, IL: Charles C. Thomas.
Smith, T. J., Williamson, C. H., Hill, K., Sahl, J. W., and Keim, P. (2018). Botulinum neurotoxin -producing bacteria - isn’t it time we called a species a species? mBio 9:e01469-18. doi: 10.1128/mBio.01469-18
Smith, T. J., Xie, G., Williamson, C. H. D., Hill, K. K., Sahl, J. W., Keim, P., et al. (2020). Genomic characterization of newly completed genomes of botulinum neurotoxin-producing species from Argentina, Australia and Africa. Genome Biol. Evol. 12, 229–242. doi: 10.1093/gbe/evaa043
Stackebrandt, E., Kramer, I., Swiderski, J., and Hippe, H. (1999). Phylogenetic basis for a taxonomic dissection of the genus Clostridium. FEMS Immunol. Med. Microbiol. 24, 253–258.
Suen, J. C., Hatheway, C. L., Steigerwalt, A. G., and Brenner, D. J. (1988a). Clostridium argentinense sp. nov.: a genetically homogeneous group composed of all strains of Clostridium botulinum toxin type G and some nontoxigenic strains previously identified as Clostridium subterminale or Clostridium hastiforme. Int. J. Syst. Bacteriol. 38, 375–381.
Suen, J. C., Hatheway, C. L., Steigerwalt, A. G., and Brenner, D. J. (1988b). Genetic confirmation of identities of neurotoxigenic Clostridium baratii and Clostridium butyricum implicated as agents of infant botulism. J. Clin. Microbiol. 26, 2191–2192.
van Ermengem, E. (1897). A new anaerobic bacillus and its relation to botulism. E. van Ermengem. Originally published as “Ueber einen neuen anaëroben Bacillus und seine Beziehungen zum Botulismus” in Zeitschrift für Hygiene und Infektionskrankheiten 26: 1-56, 1897. Rev. Infect. Dis. 1, 701–719.
Viray, M. A., Wamala, J., Fagan, R., Luquez, C., Maslanka, S., Downing, R., et al. (2014). Outbreak of type A foodborne botulism at a boarding school, Uganda, 2008. Epidemiol. Infect. 142, 2297–2301. doi: 10.1017/S0950268814000387
Walker, B. J., Abeel, T., Shea, T., Priest, M., Abouelliel, A., Sakthikumar, S., et al. (2014). Pilon: an integrated tool for comprehensive microbial variant detection and genome assembly improvement. PLoS One 9:e112963. doi: 10.1371/journal.pone.0112963
Weigand, M. R., Pena-Gonzalez, A., Shirey, T. B., Broeker, R. G., Ishaq, M. K., Konstantinidis, K. T., et al. (2015). Implications of genome-based discrimination between Clostridium botulinum Group I and Clostridium sporogenes strains for bacterial taxonomy. Appl. Environ. Microbiol. 81, 5420–5429. doi: 10.1128/AEM.01159-15
Williamson, C. H., Sahl, J. W., Smith, T. J., Xie, G., Foley, B. T., Smith, L. A., et al. (2016). Comparative genomic analyses reveal broad diversity in botulinum-toxin-producing Clostridia. BMC Genomics 17:180. doi: 10.1186/s12864-016-2502-z
Williamson, C. H. D., Vazquez, A. J., Hill, K., Smith, T. J., Nottingham, R., Stone, N. E., et al. (2017). Differentiating botulinum-neurotoxin-producing clostridia with a simple, multiplex PCR assay. Appl. Environ. Microbiol. 83:e00806-17. doi: 10.1128/AEM.00806-17
Zhang, Z., Hintsa, H., Chen, Y., Korkeala, H., and Lindstrom, M. (2013). Plasmid-borne type E neurotoxin gene clusters in Clostridium botulinum strains. Appl. Environ. Microbiol. 79, 3856–3859. doi: 10.1128/AEM.00080-13
Keywords: Clostridium parabotulinum, neurotoxin, plasmids, orfX, lycA, arsC, pulE
Citation: Smith TJ, Williamson CHD, Hill KK, Johnson SL, Xie G, Anniballi F, Auricchio B, Fernández RA, Caballero PA, Keim P and Sahl JW (2021) The Distinctive Evolution of orfX Clostridium parabotulinum Strains and Their Botulinum Neurotoxin Type A and F Gene Clusters Is Influenced by Environmental Factors and Gene Interactions via Mobile Genetic Elements. Front. Microbiol. 12:566908. doi: 10.3389/fmicb.2021.566908
Received: 29 May 2020; Accepted: 08 February 2021;
Published: 26 February 2021.
Edited by:
Daniel Yero, Autonomous University of Barcelona, SpainReviewed by:
Paul Stenmark, Stockholm University, SwedenCopyright © 2021 Smith, Williamson, Hill, Johnson, Xie, Anniballi, Auricchio, Fernández, Caballero, Keim and Sahl. This is an open-access article distributed under the terms of the Creative Commons Attribution License (CC BY). The use, distribution or reproduction in other forums is permitted, provided the original author(s) and the copyright owner(s) are credited and that the original publication in this journal is cited, in accordance with accepted academic practice. No use, distribution or reproduction is permitted which does not comply with these terms.
*Correspondence: Theresa J. Smith, VGhlcmVzYS5TbWl0aEBuYXUuZWR1; dGVycnlzMm11Y2hAY29tY2FzdC5uZXQ=
Disclaimer: All claims expressed in this article are solely those of the authors and do not necessarily represent those of their affiliated organizations, or those of the publisher, the editors and the reviewers. Any product that may be evaluated in this article or claim that may be made by its manufacturer is not guaranteed or endorsed by the publisher.
Research integrity at Frontiers
Learn more about the work of our research integrity team to safeguard the quality of each article we publish.