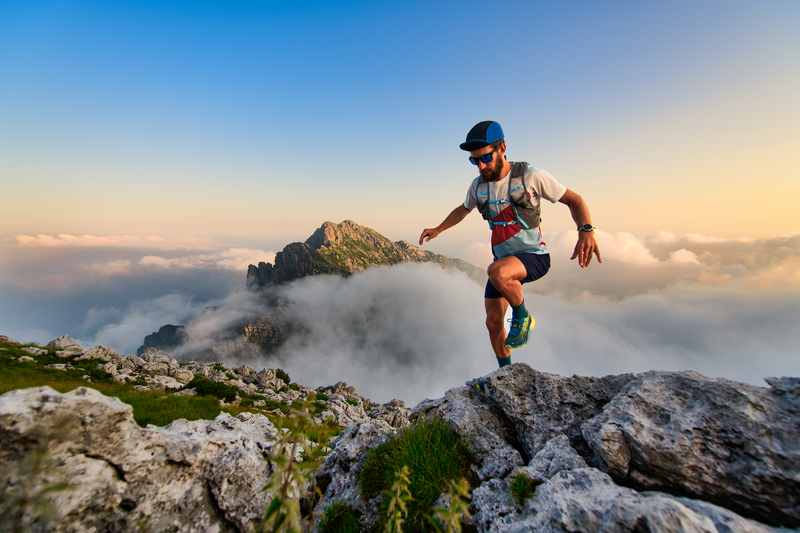
95% of researchers rate our articles as excellent or good
Learn more about the work of our research integrity team to safeguard the quality of each article we publish.
Find out more
REVIEW article
Front. Microbiol. , 28 January 2021
Sec. Microbiotechnology
Volume 11 - 2020 | https://doi.org/10.3389/fmicb.2020.619766
This article is part of the Research Topic Trace Elements in the Environment: Biogeochemical Cycles and Bioremediation View all 6 articles
Chromium (Cr) (VI) is a well-known toxin to all types of biological organisms. Over the past few decades, many investigators have employed numerous bioprocesses to neutralize the toxic effects of Cr(VI). One of the main process for its treatment is bioreduction into Cr(III). Key to this process is the ability of microbial enzymes, which facilitate the transfer of electrons into the high valence state of the metal that acts as an electron acceptor. Many underlying previous efforts have stressed on the use of different external organic and inorganic substances as electron donors to promote Cr(VI) reduction process by different microorganisms. The use of various redox mediators enabled electron transport facility for extracellular Cr(VI) reduction and accelerated the reaction. Also, many chemicals have employed diverse roles to improve the Cr(VI) reduction process in different microorganisms. The application of aforementioned materials at the contaminated systems has offered a variety of influence on Cr(VI) bioremediation by altering microbial community structures and functions and redox environment. The collective insights suggest that the knowledge of appropriate implementation of suitable nutrients can strongly inspire the Cr(VI) reduction rate and efficiency. However, a comprehensive information on such substances and their roles and biochemical pathways in different microorganisms remains elusive. In this regard, our review sheds light on the contributions of various chemicals as electron donors, redox mediators, cofactors, etc., on microbial Cr(VI) reduction for enhanced treatment practices.
Chromium (Cr) is a pervasive toxin that inhabits almost every component of the environment including aerial, terrestrial, aquatic, and biological systems (Rahman and Singh, 2019). The nearly ubiquitous existence of this element is detrimental to natural establishments of Earth. Various environment and health protection agencies have considered Cr as a priority pollutant (DesMarias and Costa, 2019; Laxmi and Kaushik, 2020). The high magnitude of Cr contamination across the globe is estimated to pour risk on approximately 16 million people (Pure Earth, 2015). Both anthropogenic and natural events can contribute to the global Cr reservoir (Jeřábková et al., 2018; Coetzee et al., 2020; Tumolo et al., 2020). For example, industrial activities such as discharge of effluents and solid wastes from industrial energy production, manufacturing of refractories, stainless steel and chemical dye pigment production, chrome plating, treatment of wood, use of organic fertilizers and chemicals, waste and wastewater management, tanning, mining, etc., have created a widespread accumulation of Cr in their surroundings (Barnhart, 1997; Wilbur et al., 2012; Huang et al., 2019; Rahman and Singh, 2019). Cr is also used as catalyst, oxidizing agent and cooling agent with water (Saha et al., 2013). In another way, hastened dissolution of chromite and other minerals from natural reserves (i.e., serpentine soil and ultramafic rocks) as a natural event instigates the release of Cr into groundwaters upon suitable conditions (Gonzalez et al., 2005; Islam et al., 2020). Moreover, volcanic eruptions and forest fires also account for some Cr contamination in the environment (Viti et al., 2014).
The toxicity of Cr relies upon its oxidation states. Among commonly occurring states of Cr(0), Cr(III), and Cr(VI), the last species is viewed as more toxic than any other species (RoyChowdhury et al., 2018; Pechancová et al., 2019). Cr(VI) usually exists in the form of oxyanions of HCrO4–, CrO42–, or Cr2O72– after reacting with oxygen (Rahman and Singh, 2019). The bioavailability and mobility of Cr(VI) are highly dependent on pH regulation, molecular oxygen (O2) availability, and the presence of organic matter and manganese oxides (MnO2) (Reijonen and Hartikainen, 2016; Shahid et al., 2017; Choppala et al., 2018). Cr speciation is also sensitive to soil redox potential (Eh), as the reduction and oxidation processes prevail at low and high Eh values, respectively (Xiao et al., 2015). Cr(VI) compounds are more toxic than Cr(III) compounds because of their high water solubility and mobility, whereas Cr(III) forms precipitation at body pH and does not exist in mobile species. Because of the structural similarity of chromate with sulfate, Cr(VI) can pass the cell membrane, but trivalent Cr fails to do so (Mukherjee et al., 2013). Further, the high oxidizing potential of Cr(VI) is also liable for its toxic effects in biological organisms (Sobol and Schiestl, 2012).
Cr(VI) displays genotoxic, carcinogenic, teratogenic, and mutagenic effects along with epigenetic profile silencing (Hu et al., 2016; DesMarias and Costa, 2019; Rager et al., 2019). As per the United States Environmental Protection Agency (US EPA), Cr(VI) is categorized as a “Group A” human carcinogen by the inhalation route of exposure (US EPA, 1998). Cr can induce phytotoxicity in plants by interfering with nutrient uptake and photosynthesis, generation of reactive oxygen species (ROS), lipid peroxidation, and altering the antioxidant activities (Shahid et al., 2017). Even elevated Cr(VI) concentrations can reduce the abundance of microbial communities by electron competing ability, inhibition of extracellular polymeric substances (EPSs) synthesis, and other effects including overproduction of ROS, protein and enzyme dysfunction, destruction of thiol and iron-sulfide cluster, inhibition of functional genes, nutrient assimilation and metabolic pathways, lipid peroxidation, DNA damage, etc. (Bhakta, 2017; Liu et al., 2017; Sun et al., 2019).
Removal of Cr(VI) from the environment is challenging. There are many methods such as ion exchange, electrochemical precipitation, solvent extraction, membrane separation, evaporation, foam separation, ultrafiltration, electrodialysis, cementation, biosorption, and reduction (Mukherjee et al., 2013). However, the conventional reduction and precipitation methods of Cr(VI) removal utilize large amounts of chemicals and generate enormous toxic sludge (Mukherjee et al., 2013). The recent possible removal measures rely on the implementation of biotechnological approaches, considering ecofriendly and cost-effective approaches (Fernández et al., 2018; Jobby et al., 2018). Especially, Cr(VI) reduction by microorganisms is being recognized as an eventual treatment. Despite successful outcomes, certain disadvantages such as low efficiency and poor compatibility due to limited availability of electron donors and other inducers often hamper the prominence of Cr(VI) bioremediation in large-scale implementation (Malaviya and Singh, 2016; Beretta et al., 2019; Wang et al., 2020). These limitations can be subdued by introducing low concentrations of suitable chemicals in the medium (Figure 1). But such chemical integration demands a detailed understanding of additive-influenced mechanisms in credible microorganisms. Considering this significance, this review recognizes and emphasizes the experimental studies of influences of electron donors, electron mediators, and other chemical additives on microbial Cr(VI) reduction for the enhanced Cr(VI) removal approaches.
Figure 1. Role of various chemicals other than electron donors and mediators on enhanced Cr(VI) reduction.
Several different microorganisms have adopted various strategies to counter-effect the toxicity of Cr(VI). Among different methods, enzymatic reduction of Cr(VI) into Cr(III) by microorganisms is the best characterized mechanism for its bioremediation (Singh et al., 2008). All Cr(VI)-resistant microbes cannot reduce Cr(VI). However, Cr(VI) resistance is a common phenomenon in all Cr(VI) bioreducers, which delivers proficiency in detoxification process (Singh et al., 2008; Thatoi et al., 2014). The catalysis of Cr(VI) reduction can be demonstrated using chromosome or plasmid-encoded non-specific enzymes (Cervantes and Campos-García, 2007; Pradhan et al., 2016; Baldiris et al., 2018). These enzymes are mainly oxidoreductases such as chromate reductases (ChrA and YieF), NADH-dependent nitroreductase, iron reductase, quinone reductases, hydrogenases, NADH/NADPH-dependent flavin reductases, and NADPH-dependent reductases (Puzon et al., 2002; Kwak et al., 2003; Ackerley et al., 2004). Even many microorganisms exhibited Cr(VI) reduction using reductases with multiple substrate specificity (Table 1). Cr(VI) reduction enzymes have shown inductive or constitutive expression in different microorganisms (Ibrahim et al., 2012; Chai et al., 2018).
Table 1. Cr(VI) reduction in different microorganisms using reductases with multiple substrate specificity.
Biological Cr(VI) reduction activities can occur at extracellular, cell membrane, and intracellular locations in aerobic and anaerobic conditions (Cervantes et al., 2001; Cervantes and Campos-García, 2007). Extracellular Cr(VI) reduction is regulated by soluble (cytoplasmic) proteins exported to the extracellular medium by an energy-intensive process (Cheung and Gu, 2007; Elangovan et al., 2010; Das et al., 2014). This mechanism is accustomed to protect microbes from the damaging effects of Cr(VI) by minimizing its active intracellular transport (Wani et al., 2018). In anaerobic reduction, microorganisms may use Cr(VI) as the terminal electron acceptor in electron transport system associated with membrane enclosed regions (Ramírez-Díaz et al., 2008). Consecutively, the transformation of Cr(VI) into Cr(III) can also occur spontaneously in microorganisms by chemical reactions using different intracellular or extracellular compounds, metabolic end products, and intracellular reductants of ascorbate glutathione, cysteine, and hydrogen peroxide (H2O2) (Donati et al., 2003; Viti and Giovannetti, 2007; Poljsak et al., 2010; Thatheyus and Ramya, 2016).
The enzymatic reduction of Cr(VI) to Cr(III) can also yield variable concentrations of ROS, which may or may not involve in the formation of reactive intermediates (Cheng et al., 2009; McNeill et al., 2012; Baldiris et al., 2018). However, different reductase enzymatic activities possess some capacity to alleviate the effects of ROS. Accordingly, homologous enzymes involved in catalyzing the electron movement from electron donors to reduce Cr(VI) are classified into class I (tight) and class II (semitight) reductases (Thatoi et al., 2014; Baldiris et al., 2018). The class I reductases catalyze one-step electron reduction of Cr(VI) to form the highly unstable Cr(V) intermediate. The tendency of this reactive intermediate to oxidize into Cr(VI) by donating electrons to molecular oxygen generates ample ROS. However, class II chromate reductases are two electron reducers of Cr(VI), in which the formation of Cr(III) proceeds without forming a Cr(V) intermediate. This results in much lesser generation of ROS during the reduction process (Thatoi et al., 2014). Moreover, quinone reductase activity can further neutralize the effects of ROS to some extents (Cheung and Gu, 2007; Thatoi et al., 2014). Apart from this, chromate-resistant microorganisms also tend to stimulate the efflux system, SOS response of DNA damage repair, ROS scavenging enzymes (catalase, superoxide dismutase, etc.), and non-enzymatic antioxidants (vitamin C and E, carotenoids, thiol antioxidants, and flavonoids) for inactivating ROS-mediated oxidative stress (Ackerley et al., 2006; Cervantes and Campos-García, 2007; Flora, 2009).
In general, electron donors release electrons during cellular respiration accompanied by the release of energy. But such cellular reaction in microorganisms also enables biological treatment by providing electrons to Cr(VI) (Ceci et al., 2019; Truskewycz et al., 2019). Even suitable electron donors can facilitate the enhancement of reduction activity (Poopal and Laxman, 2009; Alam and Ahmad, 2012; Ibrahim et al., 2012; Shi et al., 2012). The requirement of electron donor is indispensable for both microbial growth and Cr(VI) reduction (Poopal and Laxman, 2009). Most microbial species capable of Cr(VI) reduction are heterotrophic and need such additional nutrition from external sources during the reduction process (Ancona et al., 2020). Certainly, some endogenous reserves can also serve electrons to Cr(VI) during its bioreduction (McLean and Beveridge, 2001; Ray et al., 2018).
The most common electron donors for Cr(VI) reduction are organic molecules, although reports on inorganic matters as reducing equivalents also exist (Figure 2). Usually, the abundant oxygen-containing functional groups (C–O, CO–OH, C–OH, and C–O–R) in organic matters present the utility as electron donors (π electrons) (Choppala et al., 2016; Shaheen et al., 2019; Xu et al., 2019). Glucose, fructose, lactose, pyruvate, lactate, citrate, glycerol, acetate, formate, NADH/NADPH, reduced glutathione, etc., are the various popular electron donors for Cr(VI) reduction (Garbisu et al., 1998; Poopal and Laxman, 2009; Murugavelh and Mohanty, 2013; Salamanca et al., 2013; Ahemad, 2014; Mala et al., 2015). Many such examples for the treatment of Cr(VI) can be further studied from Table 2. Among them, the widespread electron donor implicated in Cr(VI) reduction process is NAD(P)H, which is usually associated with intracellular reduction (Robins et al., 2013; Tan et al., 2020). Even many chromate reductase enzymes exhibited NAD(P)H-dependent properties, where the attachment of dehydrogenase molecule to enzyme is crucial for a feasible reaction. The reductase enzyme in Alishewanella sp. possessed Arg13 and Gly113 residues for the cobinding of NAD(P)H and Cr(VI) (Xia et al., 2018). Another Flavin mononucleotide (FMN)-containing chromate reductase of Gluconacetobacter hansenii (Gh-ChrR) induced structural rearrangement of active site during the binding of chromate anion and NADH. With this rearrangement, both species could bind simultaneously for efficient enzyme cycling, of which binding site otherwise overlapped with the electron donor (Jin et al., 2012). Also, microbial Cr(VI) reduction using glucose as the donor source is very prominent (Camargo et al., 2003;
Table 2. Enhancement of Cr(VI) reduction using different electron donors by different microorganisms.
Megharaj et al., 2003; Satarupa and Paul, 2013; Ziagova et al., 2014). Glucose, being the most easily metabolized carbon source, can deliver maximum electrons for Cr(VI) reduction. The synthesis of volatile fatty acids (VFAs) during glucose fermentation provides additional sources of electron donors, which can also be attributed for the improved performance of Cr(VI) reduction (Zheng et al., 2019). Furthermore, glucose exhibits indirect action on Cr(VI) reduction by promoting microbial growth (Leita et al., 2011). However, the role of glucose as the most suitable electron donor is not consistent for all Cr(VI) reducer. Anaerobic process by Pannonibacter phragmitetus LSSE-09 promoted Cr(VI) reduction more in presence of acetate, lactate, and pyruvate rather than glucose (Xu et al., 2011). Another anaerobic strain, P. phragmitetus BB also exhibited more reduction of Cr(VI) in presence of lactate than many other electron donors. Metabolic analyses of strain BB elucidated that the presence of Cr(VI) upregulated pyruvate dehydrogenase and lactate dehydrogenase, and these dehydrogenases preferentially transferred the cellular electrons to extracellular Cr(VI) over other electron acceptors (Chai et al., 2019). In another study, anaerobic suspensions of Pelosinus sp. HCF1 reduced chromate in the presence of lactate as a sole electron donor (Beller et al., 2013). Lactate fermentation to acetate and propionate through methylmalonyl-CoA pathway also broadened the availability of electron donors in the system (Beller et al., 2013). Bill et al. (2019) also corroborated the evidence of lactate fermentation into simpler metabolites and further degradation into CO2. In this process, Cr(VI) removal was basically associated with the breakdown of carboxylic acids. However, lactate as an electron donor also causes a significant abiotic Cr(VI) reduction due to the formation of a lactate–Cr(VI) complex or sodium lactate syrup that functions as a non-specific reductant (Brodie et al., 2011). Fungal metabolites such as salicylate, tartrate, and citrate produced by a metalliferous Aspergillus tubingensis Ed8 were also stimulators for Cr(VI) reduction (Coreño-Alonso et al., 2009). However, non-fermentable substrates such as citrate, butyrate, and acetate as electron donors have shown usually lower effects for enhanced Cr(VI) removal rates (Orozco et al., 2010). Nevertheless, the contribution of acetate as the most suitable electron donor for Cr(VI) reduction in certain microorganisms is also not deniable. The accumulation of acetate as one of the fermentation product has greatly influenced Cr(VI) reduction in the anaerobic system (Bai et al., 2018; Zheng et al., 2019; Zhang et al., 2020). In an investigation, addition of Cr(VI) shifted the amounts of fermentation products toward more oxidative form, i.e., acetate (∼2.5 times) (Sharma, 2002). The plausible reason of this shift toward acetate formation after Cr(VI) exposure is likely to secure more NADH molecule that can be channeled toward bioreduction process. Mass balance reaction also supported the reason, which derived more loss of NADH molecules by butyrate and lactate formation than acetate synthesis (Sharma, 2002). Occasionally, acetoclastic pathway by Archaea instigated the release of electrons from acetate for Cr(VI) reduction (Hu et al., 2018). Aerobic bacterial strains, Lysinibacillus fusiformis ZC1 and Intrasporangium sp. strain Q5-1 also consumed acetate as the most suitable electron donor for Cr(VI) reduction in reports of He et al. (2011) and Yang et al. (2009), respectively.
The bacterium Pseudomonas aeruginosa CCTCC AB91095 offered simultaneous removal of phenol and Cr(VI), where the degradation of the former compound supported Cr(VI) reduction (Song et al., 2009). Supposedly, meta-pathways maintained the requirement of electrons from phenol biodegradation for Cr(VI) removal (Chen et al., 2003; Song et al., 2009). The bacterium, Brevibacterium casei employed azo dye acid orange 7 (AO7) as an electron donor for Cr(VI) reduction by coupling with dye decolorization under nutrient-limiting conditions (Ng et al., 2010). Another reactive black-5 azo dye was also proposed to serve electron for Cr(VI) reduction by bacterial strains (Mahmood et al., 2013). Chen et al. (2014) identified a new pathway for Cr(VI) reduction by Streptomyces violaceoruber LZ-26-1, which involved thioredoxin as an electron donor following its reduction using thioredoxin reductase. However, the main supply of electrons to thioredoxin was originated from NADPH molecules.
The utilization of electron donors for Cr(VI) reduction is species-dependent, and the reduction rate in specific microorganisms also varies for different sources (Das et al., 2014; Zhang et al., 2018). The electron donor that is effective in catalyzing Cr(VI) reduction for one microorganism might not be as useful for other species. It is also highly probable that multiple reduction pathways of variable efficiencies can be operated for different electron donors in different species. The capacity of electron donation of different donor compounds also relies on a mobile cytochrome electron carrier, quinone pool, or a dehydrogenase activity, where the measures of decreased potential differences between donor and acceptor compound states matter (Kracke et al., 2015). However, if the available electron donors are not suitable for any microorganism, adverse impacts on both growth and reduction process are noticeable. For example, Cr(VI) can be more toxic to microbes, when ethanol or butyrate is the sole electron donor as compared to more favorable glucose or lactate (Field et al., 2018). The cells in aerobic systems cannot gain enough energy from butyrate or ethanol for growth. Therefore, the availability of electrons for Cr(VI) reduction remains insufficient. Moreover, the presence of ethanol decreases the cell viability by shearing biological membrane, and metabolically active cells become more susceptible to alcohol and Cr(VI) uptake and their toxicity. Nevertheless, some anaerobic conditions have utilized the route of oxidation of ethanol by sulfate reducing bacteria for Cr(VI) removal (Pagnanelli et al., 2012; Cirik et al., 2013).
In recent years, microbial synergy has been used to couple various electron donors for effective Cr(VI) reduction process (Lu et al., 2020; Shi et al., 2020a). The outcomes have demonstrated obvious improvement in Cr(VI) reduction. The utilization of both hydrogen and methane (CH4) as dual electron donors involving microbial consortia of autohydrogenotrophic bacteria (e.g., Hydrogenophaga, Thiobacillus, and Acetoanaerobium), CH4–metabolizing microorganisms (e.g., Methanobacterium and Methanosaeta), and heterotrophic Cr(VI) reducers (e.g., Geobacter, Spirochaetaceae, Delftia, and Anaerolineaceae) promoted Cr(VI) reduction in different bioreactors (He et al., 2020, 2021). However, microbial anaerobic Cr(VI) reduction using hydrogen (H2) or CH4 as an individual electron donor also persists, but the capacity of removal is limited in different aspects (Chung et al., 2006, 2007; Lai et al., 2016; Luo et al., 2019). In a CH4-based membrane biofilm reactor (MBfR), metagenomics analysis showed that oxidation of CH4 by a sole anaerobic methanotroph, Candidatus “Methanoperedens,” in the biofilm was responsible for Cr(VI) reduction (Luo et al., 2019). Another MBfR exhibited relative abundance of three genera Methylocystis, Meiothermus, and Ferruginibacter, which was coupled with CH4 oxidation, and Cr(VI), Se(VI), and SO42– reduction (Lv et al., 2018). In other ways, S(0) or Fe(0) can also be used as inorganic electron donors for biologically Cr(VI) reduction employing microbial synergism. Shi et al. (2019) found that VFAs produced by Fe(0) or S(0) oxidizing bacteria (e.g., Thiobacillus or Ferrovibrio) could be utilized by Cr(VI) reducer (e.g., Geobacter or Desulfovibrio). Another sulfur-based mixotrophic Cr(VI) reduction process involved autotrophic sulfur oxidation and heterotrophic chromate reducing bacteria such as Desulfovibrio and Desulfuromonas (Zhang et al., 2020). The coupling of different processes such as microbial sulfur cycle, Fe(III)/Fe(II) transformation, phenol degradation, and Cr(VI) reduction by microbial aggregates involving bacteria such as Desulfovibrio, Comamonas, Ochrobactrum, and Thiobacillus offered effective and simultaneous removal of multiple contaminants including Cr(VI) and also facilitated the reduction of the reoxidized Cr(III) (Zhao et al., 2020b). Recently, the mixed biogas forms, CH4 and hydrogen, regulated by bacteria such as Geobacter and Methanobacterium in a microbial fuel cell (MFC) promoted Cr(VI) reduction by Cr(VI) reducers (e.g., Hydrogenophaga, Thiobacillus, Geobacter, and Anaerolineaceae) coupled with hydrogen-oxidizing bacteria (e.g., Hydrogenophaga and Thiobacillus) and CH4-oxidizing bacteria (e.g., Methanobacterium and Methanosaeta) (He et al., 2021).
Microbes are further known to utilize composite matters or combinations of donor compounds for Cr(VI) reduction (Figure 2; Shi et al., 2012; Mala et al., 2015; Huang et al., 2019). Abundant natural substances such as cellulose, hemicellulose, pectin, starch, and xylose can be the bulk sources of reducing equivalents like NADH and reduced ferredoxin during the metabolic route of glucose fermentation (Bai et al., 2018). Cellulosic waste that can be biodegraded to other carbon sources such as sugars, organic acids, and alcohols can readily stimulate bacterial growth and the subsequent reduction of Cr(VI) (Thomas et al., 2016; Field et al., 2018). This was also supported by an observation where Cr stress situation enhanced production of cellulase enzyme for the hydrolysis of cellulose (Aslam et al., 2019). Another study measured the coupling of cellulose and cellulose-degrading bacterium, Cellulomonas strain Lsc-8 in an MFC to reduce Cr(VI), and generated electricity simultaneously (Cao et al., 2020). Emulsified vegetable oil (EVO) and molasses as carbon sources have also influenced the biological metabolism to promote Cr(VI) reduction (Michailides et al., 2015; Wen et al., 2017). EVO being an oil-in-water emulsion operated as a slowly released electron donor by fermenting into acetate and hydrogen (Wen et al., 2017). EVO has advantages over other soluble substrates for Cr(VI) reduction, as it can provide long-term electrons for the biological process by inhibiting rapid biodegradation, and downgradient migration (Wen et al., 2017). Likewise, another solid substrate, cellulose-rich materials as a biodegradable meal box (BMB) also exhibited property of slowly releasing electrons for Cr(VI) reduction (Li et al., 2016). Molasses as an economical and readily available carbon source have offered more efficient Cr(VI) reduction than by many other sugars. The superiority of molasses over other carbon sources in Cr(VI) reduction can be attributed to its diverse constituents such as nitrogenous substances, vitamins, trace elements, and a large amount of different sugars (Smith et al., 2002; Field et al., 2013). Also, the presence of more easily utilizable sugars in molasses can be presumably more effective than pure sucrose for electron donating capability (Field et al., 2013). The phenolic hydroxyl and carbonyl groups of reducing substances such as flavonoids in molasses might also supply the electrons to the Cr(VI) (Okello et al., 2012; Chen et al., 2015). Complex nutrient media such as yeast extract and peptone can act as energy substances to support natural microbial communities, which rapidly increase the biological Cr(VI) reduction (Ancona et al., 2020; Dey and Paul, 2020). Possibly, such constituents in different culture media have always influenced the Cr(VI) reduction process by electron donating property as well. In contrary, some organic constituents in complex matters can interfere with the bioavailability of Cr(VI) for its reduction (Dey and Paul, 2020). Also, the competition of electron donors for the reduction of other co-contaminants such as SO42–, NO3–, and Se(VI) might often impede with enhanced Cr(VI) reduction (Zhong et al., 2017; Lv et al., 2018). But the advantage lies as the oxidation of organic compounds preferentially donates electrons to Cr(VI) over many other acceptor molecules considering its high reducing potential (Cirik et al., 2013).
Extracellular reduction may require some passage capacity for intracellular reducing equivalents to approach exterior Cr(VI). Such pathway raises the value of electron mediators (or electron shuttle) for reduction outside the cell (Bai et al., 2018). Mediators can reversibly reduce and oxidize to shuttle electrons from cellular boundary to terminal electron acceptor [i.e., Cr(VI)] (Han et al., 2016; Tanaka et al., 2018; Cheng et al., 2019). In this process, complexes such as porin-cytochrome located on the bacterial membrane can provide an interface for the transfer of intracellular electrons using redox mediators (Richardson et al., 2013). Mediators have a role in accelerating the reducing activity rather than promotion effect on the Cr(VI) reduction (Huang et al., 2019). Because such additives can prominently speed up the electron transfer rate (Cheng et al., 2019). Such chemical reaction is feasible when the standard redox potential of an electron mediator lies between the standard redox potentials of two half-reactions (Kavita and Keharia, 2012). In this conversion, electrons can readily shuttle from low-potential electron donors to mediators and then from low-potential mediators to the terminal electron acceptor, i.e., Cr(VI). Consequently, mere contacts among such components are sufficient for the catalysis of the Cr(VI) reduction (Voordeckers et al., 2010). The most suitable range of redox potential for mediators to succeed Cr(VI) reduction lies between −0.320 and 1.28 V, where the previous value belongs to cofactor NADPH, and the latter for chromate (Kavita and Keharia, 2012). Some redox mediators are also reported to overcome thermodynamic barriers of redox conversions and steric hindrance of the reactants (Sun et al., 2015; Dai et al., 2016). Appropriate concentrations of electron donors are also a crucial factor for the regeneration of mediators, whereas the absence of suitable electron donors may substantially hamper the activity of mediators (Chen et al., 2011; Xafenias et al., 2013; Bai et al., 2018). Many investigations identified that electron mediators could exhibit a concentration-dependent effect on Cr(VI) reduction (Rahman and Singh, 2014; Mahmood et al., 2015; Huang et al., 2016), because high concentrations of the mediator compounds could arouse inhibitory effects on microbial growth and its metabolic activity (Wolf et al., 2009; He et al., 2020).
Electron mediators for Cr(VI) reduction belong to a diverse range of organic compounds of foreign origins and endogenous metabolism and can involve some heavy metals (Figure 3; Smutok et al., 2011; Xafenias et al., 2013; Huang et al., 2019; Cao et al., 2020). Often organic mediators are heterocyclic aromatic rings possessing conjugated bonds that enable reduction and rearrangement at biologically accessible reduction potentials (Chai et al., 2019). Various humic substances and their quinoid analogs such as lawsone, menadione, anthraquinone (AQ), anthraquinone-1-sulfonate (α-AQS), anthraquinone 2-sulfonate (AQS), anthraquinone-1,5-disulfonate (1,5-AQDS), anthraquinone-2,6-disulfonate (AQDS), anthraquinone-2,7-disulfonate (2,7-AQDS), 1-chloroanthraquinone (1-CAQ), 1,5-dichloroanthraquinone (1,5-DCAQ), 1,4,5,8-tetrachloroanthraquinone (1,4,5,8-TCAQ), etc., have been popularly used to increase the electron transfer rate for microbial Cr(VI) reduction (Guo et al., 2012; Hong et al., 2012; Huang et al., 2019). Mainly, the carboxyl and phenolic hydroxyl groups present in quinoid compounds of mediators take accountability for the redox conversions between electron donors and the high valence state of the metal (Xu et al., 2020). The position and the presence of chloride ion(s) and other substituents in the aforementioned quinoid compounds also influence electron transfer limitations in Cr(VI) reduction (Hong et al., 2012; Zhang et al., 2014; Dai et al., 2016; Lian et al., 2016). In some investigations, the synergistic associations of Fe(III) minerals and AQDS were involved to deliver an alternative and attractive strategy of enhanced Cr(VI) reduction for co-contaminated sites using microorganisms like Cellulomonas sp., Shewanella oneidensis, and Geobacter sulfurreducens (Field et al., 2013; Meng et al., 2018; He et al., 2019; Liu et al., 2019b). This association employed Fe(III) reduction, and the biogenic product of this reaction, Fe(II), made possible catalysis of Cr(VI) reduction, where AQDS served as an electron carrier between the electron donor and Fe(III). However, the unaccompanied employment of Fe(III) produced lower effect on Cr(VI) reduction because of insoluble nature of the metal ion (Sharma, 2002). In another study, the biomass of henna plant showed a dual role as electron donor and redox mediator for Cr(VI) reduction. A compound, lawsone, present in the biomass displayed rate-enhancing effect on Cr(VI) reduction, whereas the VFAs and H2 produced from hydrolysis and fermentation of carbohydrate and protein supplied electrons for reduction (Liu et al., 2010; Huang et al., 2016). Various other organic compounds such as riboflavin, uric acid, dissolved organic matter, etc., have also enhanced the performance of Cr(VI) reduction by shuttling electrons from different microbial metabolisms (Xafenias et al., 2013; Mahmood et al., 2015; He et al., 2020). Another cellular metabolite, pyrrole-2-carboxylic acid (C5H5NO2), also accelerated the bioreduction process in presence of high Cr(VI) concentration by P. phragmitetus BB (Chai et al., 2019). Interestingly, the EPSs in a sulfur-reducing bacterium, Enterococcus avium strain BY7, were also reported to support as an electron carrier for Cr(VI) reduction, where different EPS components such as polysaccharides, proteins, and humic substances exhibited dissimilar electron transfer rates (Yan et al., 2020). Further, the self-assembly of strain BY7 on reduced graphene oxide (rGO) showed enhanced EPS production on bacterial surface for increased removal of Cr(VI). However, the crucial role of rGO in this association involved in reduction of Cr(III) into elemental Cr (a rare species) by lowering electronic potential or activation energy. Several dyes such as methylene blue, neutral dye, dichlorophenolindophenol (DCPIP), meldola blue, and Nile blue have also possessed electron transfer efficiency for Cr(VI) reduction in different fungal and bacterial cells (Smutok et al., 2011; Bai et al., 2018). Simultaneous removal of dyes along with Cr(VI) sets another reputation for their involvement in this reaction. However, such prominence is more suitable, when dyes act as electron donors. Two herbicides, ethyl and methyl viologens, which have been commonly used as mediators for biotransformation of different chemical pollutants, were also employed in Cr(VI) reduction. But the very low redox potential of viologen compounds weakened their roles for Cr(VI) reduction and procured only limited accelerations (Kavita and Keharia, 2012). Some heavy metals such as Cu, Fe(III), and As(III) acting as mediators have also accelerated the enzyme activity for Cr(VI) reduction (details in the following subsection). The role of Cu as a mediator in Cr(VI) transformation is very prominent in various microorganisms. Several Cr(VI) reducers such as Enterobacter sp. DU17, Bacillus spp., Pseudomonas putida KI, Ochrobactrum sp. strain CSCr-3, Brevibacterium sp. K1, and Stenotrophomonas sp. D6, etc., have shown positive effects of Cu on reduction process using different approaches (Camargo et al., 2003; Desai et al., 2008; He et al., 2009; Rahman and Singh, 2014; Ge et al., 2015; Mahmood et al., 2015). Wang et al. (2017b) showed that Fe(III) as an electron shuttle stimulated 1.6-fold more reduction of Cr(VI) in an MFC.
Figure 3. The utilization of various electron donors for Cr(VI) reduction. EVO and ETS mean emulsified vegetable oil and electron transport system, respectively. Sign represents assimilation.
Different mediator compounds have shown different electron transfer competence for Cr(VI) reduction (Wang and Jia, 2007; Liu et al., 2010; Cheng et al., 2019). For example, the addition of AQS greatly improved electron transfer efficiency in Escherichia coli BL21, complying 98.5% Cr(VI) reduction, whereas the presence of α-AQS, 1,5-AQDS, AQDS, and 2,7-AQDS could enable only 21–34% metal reduction (Guo et al., 2012). Another investigation reported increased rate of Cr(VI) reduction by E. coli K12 using different shuttles following an order of lawsone > menadione > AQS > AQDS (Liu et al., 2010). Even many microorganisms exhibited the ability to utilize multiple electron mediators for the acceleration of Cr(VI) reduction rate (Field et al., 2013; Huang et al., 2019; He et al., 2020). In different studies, redox mediators were also reported to alter the electron transfer pathway of some microorganisms for Cr(VI) reduction (Yan et al., 2014; Dai et al., 2016). The suitable example is the supply of 2-amino-3-chloro-1,4-naphthoquinone-GO (NQ-GO) in Acinetobacter sp. HK-1, which shifted and promoted electron transfer from cytoplasmic fraction to membrane counterpart for extracellular Cr(VI) reduction (Zhang et al., 2014). The electron transfer competence of different redox mediators also varies in different microorganisms, where several other factors may administer different stimulation. In many anaerobic conditions, electrons can be shuttled by series of membrane-associated proteins, where the adsorbed Cr(VI) onto cell surface can be subsequently reduced using various membrane-bound reductases (Komori et al., 1989; Wang et al., 1990; Zhu et al., 2008; Belchik et al., 2011; Cao et al., 2020). In S. oneidensis MR-1, the quinone/quinol pool in the cytoplasmic membrane transferred the electron from inner membrane across outer membrane through periplasm using network formed by cytochrome c (cyt c), where the outer membrane decaheme cyt c molecules, MtrC and OmcA, played major roles in the transfer of electrons to Cr(VI) on the cell surface using electron shuttles (Belchik et al., 2011).
Immobilization of redox mediators onto different substances for Cr(VI) reduction has also received attention for their better stability, reusability, and persistence. Moreover, lessening the toxic effects of mediator compounds on microbes by their immobilization is also evident (He et al., 2020). Lian et al. (2016) observed nearly 4.5-fold increase in Cr(VI) reduction rate using shuttle facility from 1-CAQ cellulose acetate beads involving bacterium, Mangrovibacter plantisponsor. Additionally, many previous MFCs have developed synthetic conductive polymers such as polypyrrole/9, 10-anthraquinone-2-sulfonic acid sodium salt, Si-carbide derived carbon cathode, rutile-modified polished graphite rod, etc., as insoluble redox mediators for improved concurrent Cr(VI) removal and bioelectricity production (Li et al., 2009; Pang et al., 2013; Gupta et al., 2017; Wang et al., 2017b; Li and Zhou, 2019). Table 3 provides information on the measured capacity of different redox mediators for Cr(VI) reduction by different microorganisms.
Table 3. Acceleration of Cr(VI) reduction by various electron mediators (electron shuttles) using microorganisms.
The role of chemicals beyond electron donors and mediators for improved Cr(VI) reduction also exists. A variety of chemical affluences acquires diverse mechanisms to advance Cr(VI) reduction process. Even some electron donors and mediators have enhanced Cr(VI) reduction involving other procedures. For examples, heavy metals such as Cu(II), As(III), and Fe(III) that acted as shuttles to accelerate Cr(VI) reduction activity have also upgraded reduction performance by some other effects. Few bacteria recognized Cu as a protective agent for oxygen-sensitive chromate reductase in Cr(VI) reduction (Ibrahim et al., 2012). This metal was also reported to trigger a Cr(VI) reducing enzyme, NAD(P)H-favin oxidoreductase (NfoR), by acting as ligand and changing the enzyme conformation (Han et al., 2017). In a different study, employment of CuO nanoparticles on a biological system activated multiple redox enzymes, and sulfur- and nitrogen-containing proteins for the positive effects on Cr(VI) bioreduction (Yan et al., 2019). A Cu-dependent Cr(VI) reductase is also evident in a bacterium, Amphibacillus sp. KSUCr3 (Ibrahim et al., 2012). However, Cu does not necessarily produce encouraging results in all Cr(VI) reducers. Many microorganisms have also experienced the inhibitory effects on Cr(VI) reduction for the effect of Cu (Wang et al., 1990; Park et al., 2000; Pal et al., 2005). A membrane-bound chromate reductase of Enterobacter cloacae displayed negative effects after exposure to Cu (Wang et al., 1990). Also, the addition of Cu altered electron transfer pathway and Cr(VI) reduction ability by inhibiting Ni-Fe hydrogenase activity in Caldicellulosiruptor saccharolyticus (Bai et al., 2018). Likewise, As(III) also showed varied roles for enhancing Cr(VI) reduction in different microorganisms. A study identified the surplus concentration of As(III) increased the Cr(VI) reduction rate involving Bacillus firmus TE7, and complete reduction was observed within 48 h, which was otherwise 60 h (Bachate et al., 2013). This instance sets an example of As(III) as an electron shuttle. In another study, Enterobacter sp. Z1 could positively influence the efficiency of Cr(VI) reduction from 64.5 to 92.8% after addition of As(III) within 7 days of incubation (Shi et al., 2020b). In this process, two different mechanisms were proposed for the role of As(III) in enhanced Cr(VI) reduction. In one aspect, As(III) could act as an inducer for the synthesis of cysteine and other sulfur-containing molecules to improve Cr(VI) reduction. Another scheme advised that As(III) as an electron donor might enable redox conversion of Cr(VI). Many other divalent metal ions such as Co(II), Mn(II), Ni(II), Mg(II), Zn(II), and Pb(II) and a monovalent metal ion Ag(I), which usually inhibit chromate reductase activity or produce no significant effect on reduction process, have also promoted Cr(VI) reduction by different bacterial species in various studies (Camargo et al., 2003; Elangovan et al., 2006; Sultan and Hasnain, 2007; He et al., 2009; Liu et al., 2010; Dey and Paul, 2015; Ge et al., 2015; Han et al., 2017; Bansal et al., 2019). However, the exact mechanisms of Cr(VI) bioreduction under the influence of many such metals are yet to be identified. In recent years, the co-presence of another heavy metal, vanadium V(V), has received popularity for their simultaneous removal (Zhang et al., 2012; Wang et al., 2017a, 2018; Shi et al., 2020a). Both Cr(VI) and V(V) have high reducing potential and can be reduced utilizing the same electron sources. But Cr(VI) reduction is preferential and can be enhanced by suppression of V(VI) reduction (Wang et al., 2018; Shi et al., 2020a). Also, this simultaneous removal process involved inhabited approach for V(V) reduction (Wang et al., 2017a). Addition of Ca(II) in the medium weakened the damage of Cr(VI) and promoted Cr(VI) reduction by Penicillium oxalicum SL2. The main role of this divalent cation was in stimulating the synthesis of calcium oxalate crystals for maintaining the integrity of cell wall (Luo et al., 2020). Amendment of Na(I) contributed to an increase in the activity of Cr(VI) reduction by the yeast, Pichia jadinii M9, where the role of monovalent cation in the bioprocess remained unidentified (Martorell et al., 2012). Even a metabolic inhibitor, 2,4-di nitrophenol (DNP), has also promoted Cr(VI) reduction activity in some Cr(VI) bioreducers (Wani et al., 2007; Alam and Ahmad, 2012; Dey and Paul, 2015). The stimulation of Cr(VI) reduction by DNP is attributed to the fact that its involvement as a decoupling agent could enhance the electron flow in electron transport system, thereby using Cr(VI) as a terminal electron acceptor for maximum reduction under oxygen-limiting conditions (Wani et al., 2007; Alam and Ahmad, 2012). The anionic surfactant, sodium dodecyl sulfate has also influenced the removal efficiency by partitioning of protons due to electrostatic attraction (Nandi et al., 2017). Another anion, SO4–2 also ensured increased Cr(VI) reduction by improving cell growth in some species (Bonilla et al., 2016; He et al., 2019). In a different way, the synthesis of thiol compounds from SO4–2 alleviated the oxidative stress of Cr(VI), which accelerated Cr(VI) reduction (Luo et al., 2020). NO3–-N as the sole nitrogen source also promoted Cr(VI) reduction by Pseudomonas brassicacearum LZ-4 (Yu et al., 2016). In another study, the supplement of phosphorus mineral enriched genes related to metal reduction; denitrification; carbon, nitrogen, and phosphorus cycles for the absorption of nutrient synthesis; and electron shuttles for improved Cr(VI) reduction (Ma et al., 2020). Adenylate cyclase expressing S. oneidensis MR−1 exhibited enhanced bidirectional EET and Cr(VI) reduction capacities. This was attributed to increased production of cyclic adenosine 3′,5′−monophosphate (cAMP) and cAMP-receptor protein system, which enhanced the gene expression of c−type cytochromes and flavins synthetic pathways for Cr(VI) reduction (Cheng et al., 2020). Similarly, the outer membranes of Geobacter spp. with sulfate transporter proteins and cytochromes were critical to metal reduction (Shi et al., 2009; Santos et al., 2015). The enhanced Cr(VI) reduction by microorganisms for the various roles of different chemical additives beyond electron donors and mediators is illustrated in Figure 4.
Development of bioreduction technology for large-scale applications involved a multitude of interactions and biomolecular engineering to multiscale integration. Several key factors are critical to the successful implementation of chemical additives in bioreduction of Cr(VI). Often local physicochemical characteristics present different challenges for the effectiveness of the reduction reaction. For example, reduction of Cr(VI) in contaminated environment can be influenced by the presence of different electron acceptors including O2, Fe, Mn, nitrate, etc., which can variably shift the movement of electrons (Sharma, 2002).
Lu et al. (2020) found that natural material, mackinawite, could be effectively operated as electron donors by a neutrophilic chemoautotroph, Acidovorax in a batch bioreactor, and the intermediate microbial physiological constituents, VFAs, released from mineral bio-oxidation, could enable the shuttle of electrons to Cr(VI) involving a reducer like Geobacter. In an up-flow anaerobic sludge bed reactor, the population of genus Trichococcus greatly increased toward the operation period of Cr(VI) reduction using anaerobic sludge, which mainly attributed to fermentation of organic complexes to be available as electron donors, where genera like Desulfovibrio, Ochrobactrum, and Anaerovorax were the main Cr(VI) reducers (Qian et al., 2017). Anaerobic digestion using various electron donors have shown high efficiency of Cr(VI) reduction in different bioreactors (Wang et al., 2018; Zheng et al., 2019). A large laboratory-scale experiment of a 512-day period identified that injection of EVO in Cr(VI)-contaminated aquifer created an acidic and reducing environment, which facilitated Cr(VI) bioreduction by bacteria. Amendment of EVO also greatly influenced microbial community structure and diversity toward the abundance of EVO biodegradation capabilities, which aided supply of reducing equivalents for Cr(VI) reduction (Dong et al., 2018). Additionally, the utilization of bioelectrochemical systems in the form of microbial electrolysis cell (MEC) and MFC is also an innovative route to sustainable bioremediation of Cr(VI)-polluted groundwater (Li et al., 2019b; He et al., 2021). The biogas hydrogen (H2) and CH4 produced in MEC can serve as electron donors for Cr(VI) bioreduction involving synergism of autohydrogenotrophic genus, CH4-metabolizing microorganisms and Cr(VI) reducers (He et al., 2020). Elevated Cr(VI) reduction is greatly convenient in MFC, and simultaneous power generation and removal of co-contaminants were achieved using different variants (Pophali et al., 2020; Zhao et al., 2020a). Yang et al. (2020) showed that compost-derived humic acid along with hematite could promote Cr(VI) reduction by strain MR-1, where the quinonoid and acid groups in organic substances exhibited role as electron shuttle and electron donor, respectively. In a microcosm assay, biostimulation of acetate was effective for anaerobic Cr(VI) treatment in highly alkaline and saline soil of long-term contaminated landfill of León (Guanajuato), Mexico, where a haloalkaliphilic isolate, Halomonas, was expected to lead the catalysis of Cr(VI) reduction (Lara et al., 2017). Treatment of Cr(VI)-contaminated soil from tannery site with Pseudomonas sp. (RPT) revealed enhancement of Cr(VI) reduction after biostimulation of neem (Azadirachta indica) oil cake (NOC), and the application of NOC further improved soil enzyme properties (Govarthanan et al., 2019). Habitually, indigenous microbial communities of different sites greatly influence the reduction process (Lara et al., 2017).
In situ amendment of AQSD shifted dynamic state of non-equilibrium transformation of Cr toward accelerated Cr(VI) reduction and inhibited Cr(III) oxidation in contaminated soil (Brose and James, 2010). In a field-scale investigation at Hanford’s 100 area of the United States Department of Energy facility, researchers employed a stable carbon isotope of sodium lactate (13C-labeled) to monitor biostimulation and electron donor fate for Cr(VI) reduction into the high-permeability aquifer comprising gravel and coarse sand sediments (Bill et al., 2019). Several lines of evidence in this study suggested that original 13C-lactate underwent some microbial metabolic pathways through total organic carbon (TOC), acetate, and propionate to complete mineralization by serving as electron donors for Cr(VI) reduction. In another study, amendment of organic matter followed by bioaugmentation with a consortium of actinobacteria greatly influenced the Cr(VI) reduction in soil (Lacalle et al., 2020).
The perplexing task of removing Cr(VI) contamination from the environment has led to the development of various bioremediation approaches, particularly the proficient reduction strategies by microorganisms. However, the high efficiency of large-scale microbial Cr(VI) reduction can be limited by the availability of various chemical factors, mainly electron donors, mediators, and cofactors. The oxidation of many suitable chemicals (as electron donors) enables supply of electrons to Cr(VI) to facilitate enhanced reduction. The use of composite matters or combinations of chemical compounds as electron donors grants additional benefits for large- scale application, considering economic aspects. Presence of mediator molecules takes part in improvement of the dynamics of bioreaction. Moreover, several chemicals achieve merits for Cr(VI) bioreduction involving diverse roles other than electron donors and electron mediators. Very often the information on aforementioned chemicals supports their employment for in situ and ex situ Cr(VI) bioremediation.
Chemical-assisted microbially mediated Cr(VI) reduction is sustainable only when the chemical dosages are low. Otherwise, their high concentrations may cause secondary pollution, impede the ecofriendly approach, and lose prospect of preserving the natural resources particularly for in situ applications. Although aforementioned facilities for Cr(VI) bioreduction also exist in environment through natural attenuation at limited efficiencies, in-depth understanding of the governing procedures can device commercial exploitation of the processes for enhanced removal approaches. Therefore, there is a necessity for developing eco-biotechnological schemes that could use unexploited potential of the natural ecosystems for remediation of Cr(VI) contaminated environment. Several key points important to successful application of chemical additives for improved Cr(VI) bioreduction are as follows:
• Identification of novel microbial isolates with better reduction capacity from different environmental samples and their enhanced reducing activity under various chemical affluences are foreseen.
• New knowledge of diverse and specific reduction mechanisms involving chemical consolidation can be constructive in devising effective Cr(VI) treatment.
• The choices of appropriate chemical sources as electron donors, mediators, and cofactors for different microorganisms are important to be recognized.
• Identification of green chemicals for the stimulation of Cr(VI) bioreduction is highly desirable considering the sustainability of ecofunctioning.
• Employment of indigenous chemicals as the substitute for foreign substances may provide better compatibility for the treatment of the contaminated system.
• Employment of wastes generated from other industries may contribute as the cheap alternatives for the Cr(VI) reduction process.
• The electron-donating abilities of different electron donors from composite matters are subject too difficult to analyze, but such integration to biological Cr(VI) reduction for large-scale application and economical virtue states matter.
• The capacity of utilizing endogenous microbial metabolites for reduction process is also rational.
• Chemical integration of donor and mediator compounds often implicates a complex system for Cr(VI) reduction, involving various pathways and processes and multilevel interactions. Such phenomena are also needed to be addressed in-depth.
• The involvement and interaction of numerous compounds by microbial synergy in different intricate pathways have received little attention and therefore warrant further investigations.
• The regular monitoring of microbial growth and Cr(VI) reduction for the treatment of specific chemical utilized for the process is crucial. Moreover, the parameter of soil enzyme activities of the native microbial flora should also be analyzed to identify the various effects of chemical additives.
• The role of many chemicals on Cr(VI) bioreduction is poorly understood, and their characterization relies mainly on past studies. For the same, advanced “omics” technology can add more sight on their specific involvements.
• Variously, genetic manipulation of certain genes of different bioreducers can also empower the microbial ability to exploit various chemical affluences for enhanced Cr(VI) removal.
Both authors of this manuscript have made equal contributions to writing, formation of tables and diagrams, editing, and approved it for publication.
The authors declare that the research was conducted in the absence of any commercial or financial relationships that could be construed as a potential conflict of interest.
Ackerley, D. F., Barak, Y., Lynch, S. V., Curtin, J., and Matin, A. (2006). Effect of chromate stress on Escherichia coli K-12. J. Bacteriol. 188, 3371–3381. doi: 10.1128/JB.188.9.3371-3381.2006
Ackerley, D. F., Gonzalez, C. F., Keyhan, M., Blake, R., and Matin, A. (2004). Mechanism of chromate reduction by the Escherichia coli protein, NfsA, and the role of different chromate reductases in minimizing oxidative stress during chromate reduction. Environ. Microbiol. 6, 851–860. doi: 10.1111/j.1462-2920.2004.00639.x
Ahemad, M. (2014). Bacterial mechanisms for Cr(VI) resistance and reduction: an overview and recent advances. Folia Microbiol. 59, 321–332. doi: 10.1007/s12223-014-0304-8
Alam, M. Z., and Ahmad, S. (2012). Toxic chromate reduction by resistant and sensitive bacteria isolated from tannery effluent contaminated soil. Ann. Microbiol. 62, 113–121. doi: 10.1007/s13213-011-0235-4
Ancona, V., Campanale, C., Tumolo, M., De Paola, D., Ardito, C., and Volpe, A. (2020). Enhancement of chromium (VI) reduction in microcosms amended with lactate or yeast extract: a laboratory-scale Study. Int. J. Environ. Res. Public Health 17:704. doi: 10.3390/ijerph17030704
Aslam, S., Hussain, A., and Qazi, J. I. (2019). Production of cellulase by Bacillus amyloliquefaciens-ASK11 under high chromium stress. Waste Biomass Valor. 10, 53–61. doi: 10.1007/s12649-017-0046-3
Bachate, S. P., Nandre, V. S., Ghatpande, N. S., and Kodam, K. M. (2013). Simultaneous reduction of Cr(VI) and oxidation of As (III) by Bacillus firmus TE7 isolated from tannery effluent. Chemosphere 90, 2273–2278. doi: 10.1016/j.chemosphere.2012.10.081
Bai, Y. N., Lu, Y. Z., Shen, N., Lau, T. C., and Zeng, R. J. (2018). Investigation of Cr(VI) reduction potential and mechanism by Caldicellulosiruptor saccharolyticus under glucose fermentation condition. J. Hazard. Mater. 344, 585–592. doi: 10.1016/j.jhazmat.2017.10.059
Baldiris, R., Acosta-Tapia, N., Montes, A., Hernández, J., and Vivas-Reyes, R. (2018). Reduction of hexavalent chromium and detection of chromate reductase (ChrR) in Stenotrophomonas maltophilia. Molecules 23:406. doi: 10.3390/molecules23020406
Bansal, N., Coetzee, J. J., and Chirwa, E. M. (2019). In situ bioremediation of hexavalent chromium in presence of iron by dried sludge bacteria exposed to high chromium concentration. Ecotoxicol. Environ. Saf. 172, 281–289. doi: 10.1016/j.ecoenv.2019.01.094
Barnhart, J. (1997). Occurrences, uses, and properties of chromium. Regulatory Toxicol. Pharmacol. 26, S3–S7. doi: 10.1006/rtph.1997.1132
Batool, R., Yrjala, K., and Hasnain, S. (2012). Hexavalent chromium reduction by bacteria from tannery effluent. J. Microbiol. Biotechnol. 22, 547–554. doi: 10.4014/jmb.1108.08029
Belchik, S. M., Kennedy, D. W., Dohnalkova, A. C., Wang, Y., Sevinc, P. C., Wu, H., et al. (2011). Extracellular reduction of hexavalent chromium by cytochromes MtrC and OmcA of Shewanella oneidensis MR-1. Appl. Environ. Microbiol. 77, 4035–4041. doi: 10.1128/AEM.02463-10
Beller, H. R., Han, R., Karaoz, U., Lim, H., and Brodie, E. L. (2013). Genomic and physiological characterization of the chromate-reducing, aquifer-derived firmicute Pelosinus sp. strain HCF1. Appl. Environ. Microbiol. 79, 63–73. doi: 10.1128/AEM.02496-12
Beretta, G., Daghio, M., Espinoza Tofalos, A., Franzetti, A., Mastorgio, A. F., Saponaro, S., et al. (2019). Progress towards bioelectrochemical remediation of hexavalent chromium. Water 11:2336. doi: 10.3390/w11112336
Bhakta, J. N. (2017). Metal toxicity in microorganism. in Handbook of Research on Inventive Bioremediation Techniques, ed. J. N. Bhakta Hershey, PA: IGI Global, 1–23.
Bill, M., Conrad, M. E., Faybishenko, B., Larsen, J. T., Geller, J. T., Borglin, S. E., et al. (2019). Use of carbon stable isotopes to monitor biostimulation and electron donor fate in chromium-contaminated groundwater. Chemosphere 235, 440–446. doi: 10.1016/j.chemosphere.2019.06.056
Biswas, J., Bose, P., Mandal, S., and Paul, A. K. (2018). Reduction of hexavalent chromium by a moderately halophilic bacterium, Halomonas smyrnensis KS802 under saline environment. Environ. Sustain. 1, 411–423. doi: 10.1007/s42398-018-00037-x
Bonilla, J. O., Callegari, E. A., Delfini, C. D., Estevez, M. C., and Villegas, L. B. (2016). Simultaneous chromate and sulfate removal by Streptomyces sp. MC1. Changes in intracellular protein profile induced by Cr(VI). J. Basic Microbiol. 56, 1212–1221. doi: 10.1002/jobm.201600170
Brodie, E. L., Joyner, D. C., Faybishenko, B., Conrad, M. E., Rios-Velazquez, C., Malave, J., et al. (2011). Microbial community response to addition of polylactate compounds to stimulate hexavalent chromium reduction in groundwater. Chemosphere 85, 660–665. doi: 10.1016/j.chemosphere.2011.07.021
Brose, D. A., and James, B. R. (2010). Oxidation- reduction transformations of chromium in aerobic soils and the role of electron-shuttling quinones. Environ. Sci. Technol. 44, 9438–9444. doi: 10.1021/es101859b
Camargo, F. A. O., Bento, F. M., Okeke, B. C., and Frankenberger, W. T. (2003). Chromate reduction by chromium−resistant bacteria isolated from soils contaminated with dichromate. J. Environ. Q. 32, 1228–1233. doi: 10.2134/jeq2003.1228
Cao, L., Ma, Y., Deng, D., Jiang, H., Wang, J., and Liu, Y. (2020). Electricity production of microbial fuel cells by degrading cellulose coupling with Cr(VI) removal. J. Hazard. Mater. 391:122184. doi: 10.1016/j.jhazmat.2020.122184
Ceci, A., Pinzari, F., Russo, F., Persiani, A. M., and Gadd, G. M. (2019). Roles of saprotrophic fungi in biodegradation or transformation of organic and inorganic pollutants in co-contaminated sites. Appl. Microbiol. Biotechnol. 103, 53–68. doi: 10.1007/s00253-018-9451-1
Cervantes, C., and Campos-García, J. (2007). “Reduction and efflux of chromate by bacteria,”. in Molecular Microbiology of Heavy Metals, Eds D. H. Nies and S. Silver (Berlin: Springer), 407–419.
Cervantes, C., Campos-García, J., Devars, S., Gutiérrez-Corona, F., Loza-Tavera, H., Torres-Guzmán, J. C., et al. (2001). Interactions of chromium with microorganisms and plants. FEMS Microbiol. Rev. 25, 335–347. doi: 10.1111/j.1574-6976.2001.tb00581.x
Chai, L., Ding, C., Li, J., Yang, Z., and Shi, Y. (2019). Multi-omics response of Pannonibacter phragmitetus BB to hexavalent chromium. Environ. Pollut. 249, 63–73. doi: 10.1016/j.envpol.2019.03.005
Chai, L., Ding, C., Tang, C., Yang, W., Yang, Z., Wang, Y., et al. (2018). Discerning three novel chromate reduce and transport genes of highly efficient Pannonibacter phragmitetus BB: from genome to gene and protein. Ecotoxicol. Environ. Saf. 162, 139–146. doi: 10.1016/j.ecoenv.2018.06.090
Chen, H., Li, X., and Xu, Z. (2011). Cr(VI) remediation by enriched sediment with anthraquinone-2, 6-disulfonate as electron shuttles. Phys. Chem. Earth A/B/C 36, 451–454. doi: 10.1016/j.pce.2010.05.002
Chen, Y. X., Liu, H. E., and Chen, H. L. (2003). Characterization of phenol biodegradation by Comamonas testosteroni ZD4-1 and Pseudomonas aeruginosa ZD4-3. Biomed. Environ. Sci. 16, 163–172.
Chen, Z. F., Zhao, Y. S., Zhang, J. W., and Bai, J. (2015). Mechanism and kinetics of hexavalent chromium chemical reduction with sugarcane molasses. Water Air Soil Pollut. 226:363. doi: 10.1007/s11270-015-2629-6
Chen, Z., Zou, L., Zhang, H., Chen, Y., Liu, P., and Li, X. (2014). Thioredoxin is involved in hexavalent chromium reduction in Streptomyces violaceoruber strain LZ-26-1 isolated from the Lanzhou reaches of the Yellow River. Int. Biodeterior. Biodegrad. 94, 146–151. doi: 10.1016/j.ibiod.2014.07.013
Cheng, K. Y., Karthikeyan, R., and Wong, J. W. (2019). “Microbial electrochemical remediation of organic contaminants: possibilities and perspective,” in Microbial Electrochemical Technology, eds D. Pant and S. M. Tiquia-Arashiro (Amsterdam: Elsevier), 613–640.
Cheng, Y., Yongming, X. I. E., Zheng, J., Zhaoxian, W. U., Zhi, C., Xiaoyan, M. A., et al. (2009). Identification and characterization of the chromium (VI) responding protein from a newly isolated Ochrobactrum anthropi CTS-325. J. Environ. Sci. 21, 1673–1678. doi: 10.1016/S1001-0742(08)62472-9
Cheng, Z. H., Xiong, J. R., Min, D., Cheng, L., Liu, D. F., Li, W. W., et al. (2020). Promoting bidirectional extracellular electron transfer of Shewanella oneidensis MR−1 for hexavalent chromium reduction via elevating intracellular cAMP level. Biotechnol. Bioeng. 117, 1294–1303. doi: 10.1002/bit.27305
Cheung, K. H., and Gu, J. D. (2007). Mechanism of hexavalent chromium detoxification by microorganisms and bioremediation application potential: a review. Int. Biodeterior. Biodegrad. 59, 8–15. doi: 10.1016/j.ibiod.2006.05.002
Choppala, G., Bolan, N., Kunhikrishnan, A., and Bush, R. (2016). Differential effect of biochar upon reduction-induced mobility and bioavailability of arsenate and chromate. Chemosphere 144, 374–381. doi: 10.1016/j.chemosphere.2015.08.043
Choppala, G., Kunhikrishnan, A., Seshadri, B., Park, J. H., Bush, R., and Bolan, N. (2018). Comparative sorption of chromium species as influenced by pH, surface charge and organic matter content in contaminated soils. J. Geochemical Explor. 184, 255–260. doi: 10.1016/j.gexplo.2016.07.012
Chung, J., Nerenberg, R., and Rittmann, B. E. (2006). Bio-reduction of soluble chromate using a hydrogen-based membrane biofilm reactor. Water Res. 40, 1634–1642. doi: 10.1016/j.watres.2006.01.049
Chung, J., Rittmann, B. E., Wright, W. F., and Bowman, R. H. (2007). Simultaneous bio-reduction of nitrate, perchlorate, selenate, chromate, arsenate, and dibromochloropropane using a hydrogen-based membrane biofilm reactor. Biodegradation 18:199. doi: 10.1007/s10532-006-9055-9
Cirik, K., Dursun, N., Sahinkaya, E., and Çinar, Ö (2013). Effect of electron donor source on the treatment of Cr(VI)-containing textile wastewater using sulfate-reducing fluidized bed reactors (FBRs). Biores. Technol. 133, 414–420. doi: 10.1016/j.biortech.2013.01.064
Coetzee, J. J., Bansal, N., and Chirwa, E. M. (2020). Chromium in environment, its toxic effect from chromite-mining and ferrochrome industries, and its possible bioremediation. Exposure Health 12, 51–62. doi: 10.1007/s12403-018-0284-z
Coreño-Alonso, A., Acevedo-Aguilar, F. J., Reyna-López, G. E., Tomasini, A., Fernández, F. J., Wrobel, K., et al. (2009). Cr(VI) reduction by an Aspergillus tubingensis strain: role of carboxylic acids and implications for natural attenuation and biotreatment of Cr(VI) contamination. Chemosphere 76, 43–47. doi: 10.1016/j.chemosphere.2009.02.031
Dai, R., Chen, X., Ma, C., Xiang, X., and Li, G. (2016). Insoluble/immobilized redox mediators for catalyzing anaerobic bio-reduction of contaminants. Rev. Environ. Sci. Bio Technol. 15, 379–409. doi: 10.1007/s11157-016-9404-z
Das, S., Mishra, J., Das, S. K., Pandey, S., Rao, D. S., Chakraborty, A., et al. (2014). Investigation on mechanism of Cr(VI) reduction and removal by Bacillus amyloliquefaciens, a novel chromate tolerant bacterium isolated from chromite mine soil. Chemosphere 96, 112–121. doi: 10.1016/j.chemosphere.2013.08.080
Desai, C., Jain, K., and Madamwar, D. (2008). Evaluation of in vitro Cr(VI) reduction potential in cytosolic extracts of three indigenous Bacillus sp. isolated from Cr(VI) polluted industrial landfill. Biores. Technol. 99, 6059–6069. doi: 10.1016/j.biortech.2007.12.046
DesMarias, T. L., and Costa, M. (2019). Mechanisms of chromium-induced toxicity. Curr. Opin. Toxicol. 14, 1–7. doi: 10.1016/j.cotox.2019.05.003
Dey, A. K., and Paul, S. (2015). Hexavalent chromate reduction during growth and by immobilized cells of Arthrobacter sp. SUK 1205. Science 34, 158–168. doi: 10.3923/std.2015.158.168
Dey, S., and Paul, A. K. (2020). In-vitro bioreduction of hexavalent chromium by viable whole cells of Arthrobacter sp. SUK 1201. J. Microbiol. Biotechnol. Food Sci. 9, 19–23.
Donati, E., Oliver, C., and Curutchet, G. (2003). Reduction of chromium (VI) by the indirect action of Thiobacillus thioparus. Braz. J. Chem. Eng. 20, 69–73. doi: 10.1590/S0104-66322003000100013
Dong, J., Yu, J., and Bao, Q. (2018). Simulated reactive zone with emulsified vegetable oil for the long-term remediation of Cr(VI)-contaminated aquifer: dynamic evolution of geological parameters and groundwater microbial community. Environ. Sci. Pollut. Res. 25, 34392–34402. doi: 10.1007/s11356-018-3386-z
Elangovan, R., Abhipsa, S., Rohit, B., Ligy, P., and Chandraraj, K. (2006). Reduction of Cr(VI) by a Bacillus sp. Biotechnol. Lett. 28, 247–252. doi: 10.1007/s10529-005-5526-z
Elangovan, R., Philip, L., and Chandraraj, K. (2010). Hexavalent chromium reduction by free and immobilized cell-free extract of Arthrobacter rhombi-RE. Appl. Biochem. Biotechnol. 160:81. doi: 10.1007/s12010-008-8515-6
Fernández, P. M., Viñarta, S. C., Bernal, A. R., Cruz, E. L., and Figueroa, L. I. (2018). Bioremediation strategies for chromium removal: current research, scale-up approach and future perspectives. Chemosphere 208, 139–148. doi: 10.1016/j.chemosphere.2018.05.166
Field, E. K., Blaskovich, J. P., Peyton, B. M., and Gerlach, R. (2018). Carbon-dependent chromate toxicity mechanism in an environmental Arthrobacter isolate. J. Hazard. Mater. 355, 162–169. doi: 10.1016/j.jhazmat.2018.05.020
Field, E. K., Gerlach, R., Viamajala, S., Jennings, L. K., Peyton, B. M., and Apel, W. A. (2013). Hexavalent chromium reduction by Cellulomonas sp. strain ES6: the influence of carbon source, iron minerals, and electron shuttling compounds. Biodegradation 24, 437–450. doi: 10.1007/s10532-012-9600-7
Flora, S. J. (2009). Structural, chemical and biological aspects of antioxidants for strategies against metal and metalloid exposure. Oxid. Med. Cell. Longev. 2, 191–206. doi: 10.4161/oxim.2.4.9112
Garbisu, C., Alkorta, I., Llama, M. J., and Serra, J. L. (1998). Aerobic chromate reduction by Bacillus subtilis. Biodegradation 9, 133–141. doi: 10.1023/A:1008358816529
Ge, S., Ge, S., Zhou, M., and Dong, X. (2015). Bioremediation of hexavalent chromate using permeabilized Brevibacterium sp. and Stenotrophomonas sp. cells. J. Environ. Manag. 157, 54–59. doi: 10.1016/j.jenvman.2015.04.011
Gonzalez, C. F., Ackerley, D. F., Lynch, S. V., and Matin, A. (2005). ChrR, a soluble quinone reductase of Pseudomonas putida that defends against H2O2. J. Biol. Chem. 280, 22590–22595. doi: 10.1074/jbc.M501654200
Govarthanan, M., Selvankumar, T., Mythili, R., Srinivasan, P., Ameen, F., AlYahya, S. A., et al. (2019). Biogreen remediation of chromium-contaminated soil using Pseudomonas sp. (RPT) and neem (Azadirachta indica) oil cake. Int. J. Environ. Sci. Technol. 16, 4595–4600. doi: 10.1007/s13762-018-2136-6
Guo, J., Lian, J., Xu, Z., Xi, Z., Yang, J., Jefferson, W., et al. (2012). Reduction of Cr(VI) by Escherichia coli BL21 in the presence of redox mediators. Biores. Technol. 123, 713–716. doi: 10.1016/j.biortech.2012.07.090
Gupta, S., Yadav, A., Singh, S., and Verma, N. (2017). Synthesis of silicon carbide-derived carbon as an electrode of a microbial fuel cell and an adsorbent of aqueous Cr(VI). Ind. Eng. Chem. Res. 56, 1233–1244. doi: 10.1021/acs.iecr.6b03832
Han, H., Ling, Z., Zhou, T., Xu, R., He, Y., Liu, P., et al. (2017). Copper (II) binding of NAD (P) H-flavin oxidoreductase (NfoR) enhances its Cr(VI)-reducing ability. Sci. Rep. 7, 1–12. doi: 10.1038/s41598-017-15588-y
Han, R., Li, F., Liu, T., Li, X., Wu, Y., Wang, Y., et al. (2016). Effects of incubation conditions on Cr(VI) reduction by c-type cytochromes in intact Shewanella oneidensis MR-1 cells. Front. Microbiol. 7:746. doi: 10.3389/fmicb.2016.00746
He, C., Zhang, B., Jiang, Y., Liu, H., and Zhao, H. P. (2021). Microbial electrolysis cell produced biogas as sustainable electron donor for microbial chromate reduction. Chem. Eng. J. 403:126429. doi: 10.1016/j.cej.2020.126429
He, C., Zhang, B., Yan, W., Ding, D., and Guo, J. (2020). Enhanced microbial chromate reduction using hydrogen and methane as joint electron donors. J. Hazard. Mater 395:122684. doi: 10.1016/j.jhazmat.2020.122684
He, M., Li, X., Liu, H., Miller, S. J., Wang, G., and Rensing, C. (2011). Characterization and genomic analysis of a highly chromate resistant and reducing bacterial strain Lysinibacillus fusiformis ZC1. J. Hazard. Mater. 185, 682–688. doi: 10.1016/j.jhazmat.2010.09.072
He, Y., Gong, Y., Su, Y., Zhang, Y., and Zhou, X. (2019). Bioremediation of Cr(VI) contaminated groundwater by Geobacter sulfurreducens: environmental factors and electron transfer flow studies. Chemosphere 221, 793–801. doi: 10.1016/j.chemosphere.2019.01.039
He, Z., Gao, F., Sha, T., Hu, Y., and He, C. (2009). Isolation and characterization of a Cr(VI)-reduction Ochrobactrum sp. strain CSCr-3 from chromium landfill. J. Hazard. Mater. 163, 869–873. doi: 10.1016/j.jhazmat.2008.07.041
Hong, Y., Wu, P., Li, W., Gu, J., and Duan, S. (2012). Humic analog AQDS and AQS as an electron mediator can enhance chromate reduction by Bacillus sp. strain 3C 3. Appl. Microbiol. Biotechnol. 93, 2661–2668. doi: 10.1007/s00253-011-3577-8
Hu, G., Liu, J., Zhang, Y., Zheng, P., Wang, L., Zhao, L., et al. (2016). Gene expression profiling and bioinformatics analysis in 16HBE cells treated by chromium (VI). Toxicol. Lett. 264, 71–78. doi: 10.1016/j.toxlet.2016.10.015
Hu, Q., Sun, J., Sun, D., Tian, L., Ji, Y., and Qiu, B. (2018). Simultaneous Cr(VI) bio-reduction and methane production by anaerobic granular sludge. Biores. Technol. 262, 15–21. doi: 10.1016/j.biortech.2018.04.060
Huang, J., Wu, M., Tang, J., Zhou, R., Chen, J., Han, W., et al. (2016). Enhanced bio-reduction of hexavalent chromium by an anaerobic consortium using henna plant biomass as electron donor and redox mediator. Desalination Water Treat. 57, 15125–15132. doi: 10.1080/19443994.2015.1070292
Huang, X. N., Min, D., Liu, D. F., Cheng, L., Qian, C., Li, W. W., et al. (2019). Formation mechanism of organo-chromium (III) complexes from bioreduction of chromium (VI) by Aeromonas hydrophila. Environ. Int. 129, 86–94. doi: 10.1016/j.envint.2019.05.016
Ibrahim, A. S., El-Tayeb, M. A., Elbadawi, Y. B., Al-Salamah, A. A., and Antranikian, G. (2012). Hexavalent chromate reduction by alkaliphilic Amphibacillus sp. KSUCr3 is mediated by copper-dependent membrane-associated Cr(VI) reductase. Extremophiles 16, 659–668. doi: 10.1007/s00792-012-0464-x
Ikegami, K., Hirose, Y., Sakashita, H., Maruyama, R., and Sugiyama, T. (2020). Role of polyphenol in sugarcane molasses as a nutrient for hexavalent chromium bioremediation using bacteria. Chemosphere 250;126267. doi: 10.1016/j.chemosphere.2020.126267
Islam, M. A., Angove, M. J., Morton, D. W., Pramanik, B. K., and Awual, M. R. (2020). A mechanistic approach of chromium (VI) adsorption onto manganese oxides and boehmite. J. Environ. Chem. Eng. 8:103515. doi: 10.1016/j.jece.2019.103515
Jeřábková, J., Tejnecký, V., Borůvka, L., and Drábek, O. (2018). Chromium in anthropogenically polluted and naturally enriched soils: a review. Sci. Agric. Bohem. 49, 297–312. doi: 10.2478/sab-2018-0037
Jin, H., Zhang, Y., Buchko, G. W., Varnum, S. M., Robinson, H., Squier, T. C., et al. (2012). Structure determination and functional analysis of a chromate reductase from Gluconacetobacter hansenii. PLoS One 7:e42432. doi: 10.1371/journal.pone.0042432
Jobby, R., Jha, P., Yadav, A. K., and Desai, N. (2018). Biosorption and biotransformation of hexavalent chromium [Cr(VI)]: a comprehensive review. Chemosphere 207, 255–266. doi: 10.1016/j.chemosphere.2018.05.050
Kavita, B., and Keharia, H. (2012). Reduction of hexavalent chromium by Ochrobactrum intermedium BCR400 isolated from a chromium-contaminated soil. 3 Biotech 2, 79–87. doi: 10.1007/s13205-011-0038-0
Komori, K., Wang, P. C., Toda, K., and Ohtake, H. (1989). Factors affecting chromate reduction in Enterobacter cloacae strain HO1. Appl. Microbiol. Biotechnol. 31, 567–570. doi: 10.1007/BF00270796
Kracke, F., Vassilev, I., and Krömer, J. O. (2015). Microbial electron transport and energy conservation–the foundation for optimizing bioelectrochemical systems. Front. Microbiol. 6:575. doi: 10.3389/fmicb.2015.00575
Kwak, Y. H., Lee, D. S., and Kim, H. B. (2003). Vibrio harveyi nitroreductase is also a chromate reductase. Appl. Environ. Microbiol. 69, 4390–4395. doi: 10.1128/AEM.69.8.4390-4395.2003
Lacalle, R. G., Aparicio, J. D., Artetxe, U., Urionabarrenetxea, E., Polti, M. A., Soto, M., et al. (2020). Gentle remediation options for soil with mixed chromium (VI) and lindane pollution: biostimulation, bioaugmentation, phytoremediation and vermiremediation. Heliyon 6:e04550. doi: 10.1016/j.heliyon.2020.e04550
Lai, C. Y., Zhong, L., Zhang, Y., Chen, J. X., Wen, L. L., Shi, L. D., et al. (2016). Bioreduction of chromate in a methane-based membrane biofilm reactor. Environ. Sci. Technol. 50, 5832–5839. doi: 10.1021/acs.est.5b06177
Lara, P., Morett, E., and Juárez, K. (2017). Acetate biostimulation as an effective treatment for cleaning up alkaline soil highly contaminated with Cr(VI). Environ. Sci. Pollut. Res. 24, 25513–25521. doi: 10.1007/s11356-016-7191-2
Laxmi, V., and Kaushik, G. (2020). “Toxicity of hexavalent chromium in environment, health threats, and its bioremediation and detoxification from tannery wastewater for environmental safety,” in Bioremediation of Industrial Waste for Environmental Safety, eds G. Saxena and R. N. Bharagava (Singapore: Springer), 223–243.
Leita, L., Margon, A., Sinicco, T., and Mondini, C. (2011). Glucose promotes the reduction of hexavalent chromium in soil. Geoderma 164, 122–127. doi: 10.1016/j.geoderma.2011.05.013
Li, J., Jin, R., Liu, G., Tian, T., Wang, J., and Zhou, J. (2016). Simultaneous removal of chromate and nitrate in a packed-bed bioreactor using biodegradable meal box as carbon source and biofilm carriers. Biores. Technol. 207, 308–314. doi: 10.1016/j.biortech.2016.02.005
Li, M., and Zhou, S. (2019). Efficacy of Cu(II) as an electron-shuttle mediator for improved bioelectricity generation and Cr(VI) reduction in microbial fuel cells. Biores. Technol. 273, 122–129. doi: 10.1016/j.biortech.2018.10.074
Li, M., He, Z., Hu, Y., Hu, L., and Zhong, H. (2019a). Both cell envelope and cytoplasm were the locations for chromium (VI) reduction by Bacillus sp. M6. Biores. Technol. 273, 130–135. doi: 10.1016/j.biortech.2018.11.006
Li, M., Zhou, S., and Xu, Y. (2019b). Performance of Pb (II) reduction on different cathodes of microbial electrolysis cell driven by Cr(VI)-reduced microbial fuel cell. J. Power Sources 418, 1–10. doi: 10.1016/j.jpowsour.2019.02.032
Li, Y., Lu, A., Ding, H., Jin, S., Yan, Y., Wang, C., et al. (2009). Cr(VI) reduction at rutile-catalyzed cathode in microbial fuel cells. Electrochem. Comm. 11, 1496–1499. doi: 10.1016/j.elecom.2009.05.039
Lian, J., Hu, Z., Li, Z., Guo, J., Xu, Z., Guo, Y., et al. (2016). Effects of non-dissolved redox mediators on a hexavalent chromium bioreduction process. Biotechnol. Biotechnol. Equip. 30, 292–298. doi: 10.1080/13102818.2015.1134277
Liu, G., Yang, H., Wang, J., Jin, R., Zhou, J., and Lv, H. (2010). Enhanced chromate reduction by resting Escherichia coli cells in the presence of quinone redox mediators. Biores. Technol. 101, 8127–8131. doi: 10.1016/j.biortech.2010.06.050
Liu, H., Wang, Y., Zhang, H., Huang, G., Yang, Q., and Wang, Y. (2019a). Synchronous detoxification and reduction treatment of tannery sludge using Cr(VI) resistant bacterial strains. Sci. Tot. Environ. 687, 34–40. doi: 10.1016/j.scitotenv.2019.06.093
Liu, X., Chu, G., Du, Y., Li, J., and Si, Y. (2019b). The role of electron shuttle enhances Fe (III)-mediated reduction of Cr(VI) by Shewanella oneidensis MR-1. World J. Microbiol. Biotechnol. 35:64. doi: 10.1007/s11274-019-2634-9
Liu, Y., Jin, R., Liu, G., Tian, T., and Zhou, J. (2017). Effects of hexavalent chromium on performance, extracellular polymeric substances and microbial community structure of anaerobic activated sludge in a sequencing batch reactor. J. Chem. Technol. Biotechnol. 92, 2719–2730. doi: 10.1002/jctb.5294
Lu, J., Zhang, B., He, C., and Borthwick, A. G. (2020). The role of natural Fe (II)-bearing minerals in chemoautotrophic chromium (VI) bio-reduction in groundwater. J. Hazard. Mater. 389:121911. doi: 10.1016/j.jhazmat.2019.121911
Luo, J. H., Wu, M., Liu, J., Qian, G., Yuan, Z., and Guo, J. (2019). Microbial chromate reduction coupled with anaerobic oxidation of methane in a membrane biofilm reactor. Environ. Int. 130:104926. doi: 10.1016/j.envint.2019.104926
Luo, Y., Ye, B., Ye, J., Pang, J., Xu, Q., Shi, J., et al. (2020). Ca2+ and SO42– accelerate the reduction of Cr(VI) by Penicillium oxalicum SL2. J. Hazard. Mater. 382:121072. doi: 10.1016/j.jhazmat.2019.121072
Lv, P. L., Zhong, L., Dong, Q. Y., Yang, S. L., Shen, W. W., Zhu, Q. S., et al. (2018). The effect of electron competition on chromate reduction using methane as electron donor. Environ. Sci. Pollut. Res. 25, 6609–6618. doi: 10.1007/s11356-017-0937-7
Ma, L., Chen, N., Feng, C., Li, M., Gao, Y., and Hu, Y. (2020). Coupling enhancement of Chromium (VI) bioreduction in groundwater by phosphorus minerals. Chemosphere 240:124896. doi: 10.1016/j.chemosphere.2019.124896
Mahmood, S., Khalid, A., Arshad, M., and Ahmad, R. (2015). Effect of trace metals and electron shuttle on simultaneous reduction of reactive black-5 azo dye and hexavalent chromium in liquid medium by Pseudomonas sp. Chemosphere 138, 895–900. doi: 10.1016/j.chemosphere.2014.10.084
Mahmood, S., Khalid, A., Mahmood, T., Arshad, M., and Ahmad, R. (2013). Potential of newly isolated bacterial strains for simultaneous removal of hexavalent chromium and reactive black−5 azo dye from tannery effluent. J. Chem. Technol. Biotechnol. 88, 1506–1513. doi: 10.1002/jctb.3994
Mala, J. G. S., Sujatha, D., and Rose, C. (2015). Inducible chromate reductase exhibiting extracellular activity in Bacillus methylotrophicus for chromium bioremediation. Microbiol. Res. 170, 235–241. doi: 10.1016/j.micres.2014.06.001
Malaviya, P., and Singh, A. (2016). Bioremediation of chromium solutions and chromium containing wastewaters. Crit. Rev. Microbiol. 42, 607–633. doi: 10.3109/1040841X.2014.974501
Martorell, M. M., Fernández, P. M., Fariña, J. I., and Figueroa, L. I. (2012). Cr(VI) reduction by cell-free extracts of Pichia jadinii and Pichia anomala isolated from textile-dye factory effluents. Int. Biodeterior. Biodegrad. 71, 80–85. doi: 10.1016/j.ibiod.2012.04.007
McLean, J., and Beveridge, T. J. (2001). Chromate reduction by a pseudomonad isolated from a site contaminated with chromated copper arsenate. Appl. Environ. Microbiol. 67, 1076–1084. doi: 10.1128/AEM.67.3.1076-1084.2001
McNeill, L., McLean, J., Edwards, M., and Parks, J. (2012). State of the Science of Hexavalent Chromium in Drinking Water. Denver, CO: Water Research Foundation, 36.
Megharaj, M., Avudainayagam, S., and Naidu, R. (2003). Toxicity of hexavalent chromium and its reduction by bacteria isolated from soil contaminated with tannery waste. Curr. Microbiol. 47, 0051–0054. doi: 10.1007/s00284-002-3889-0
Meng, Y., Zhao, Z., Burgos, W. D., Li, Y., Zhang, B., Wang, Y., et al. (2018). Iron (III) minerals and anthraquinone-2, 6-disulfonate (AQDS) synergistically enhance bioreduction of hexavalent chromium by Shewanella oneidensis MR-1. Sci. Tot. Environ. 640, 591–598. doi: 10.1016/j.scitotenv.2018.05.331
Michailides, M. K., Tekerlekopoulou, A. G., Akratos, C. S., Coles, S., Pavlou, S., and Vayenas, D. V. (2015). Molasses as an efficient low-cost carbon source for biological Cr(VI) removal. J. Hazard. Mater. 281, 95–105. doi: 10.1016/j.jhazmat.2014.08.004
Mukherjee, K., Saha, R., Ghosh, A., and Saha, B. (2013). Chromium removal technologies. Res. Chem. Intermediates 39, 2267–2286.
Murugavelh, S., and Mohanty, K. (2013). Isolation, identification and characterization of Cr(VI) reducing Bacillus cereus from chromium contaminated soil. Chem. Eng. J. 230, 1–9. doi: 10.1016/j.cej.2013.06.049
Nandi, R., Laskar, S., and Saha, B. (2017). Surfactant-promoted enhancement in bioremediation of hexavalent chromium to trivalent chromium by naturally occurring wall algae. Res. Chem. Int. 43, 1619–1634.
Ng, T. W., Cai, Q., Wong, C. K., Chow, A. T., and Wong, P. K. (2010). Simultaneous chromate reduction and azo dye decolourization by Brevibacterium casei: azo dye as electron donor for chromate reduction. J. Hazard. Mater. 182, 792–800. doi: 10.1016/j.jhazmat.2010.06.106
Okello, V. A., Mwilu, S., Noah, N., Zhou, A., Chong, J., Knipfing, M. T., et al. (2012). Reduction of hexavalent chromium using naturally-derived flavonoids. Environ. Sci. Technol. 46, 10743–10751. doi: 10.1021/es301060q
O’Neill, A. G., Beaupre, B. A., Zheng, Y., Liu, D., and Moran, G. R. (2020). NfoR, Chromate Reductase or FMN reductase? Appl. Environ. Microbiol 86:e01758-20. doi: 10.1128/AEM.01758-20
Orozco, A. F., Contreras, E. M., and Zaritzky, N. E. (2010). Cr(VI) reduction capacity of activated sludge as affected by nitrogen and carbon sources, microbial acclimation and cell multiplication. J. Hazard. Mater. 176, 657–665. doi: 10.1016/j.jhazmat.2009.11.082
Pagnanelli, F., Viggi, C. C., Cibati, A., Uccelletti, D., Toro, L., and Palleschi, C. (2012). Biotreatment of Cr(VI) contaminated waters by sulphate reducing bacteria fed with ethanol. J. Hazard. Mater. 199, 186–192. doi: 10.1016/j.jhazmat.2011.10.082
Pal, A., Dutta, S., and Paul, A. K. (2005). Reduction of hexavalent chromium by cell-free extract of Bacillus sphaericus AND 303 isolated from serpentine soil. Curr. Microbiol. 51, 327–330. doi: 10.1007/s00284-005-0048-4
Pang, Y., Xie, D., Wu, B., Lv, Z., Zeng, X., Wei, C., et al. (2013). Conductive polymer-mediated Cr(VI) reduction in a dual-chamber microbial fuel cell under neutral conditions. Synth. Metals 183, 57–62. doi: 10.1016/j.synthmet.2013.09.019
Park, C. H., Keyhan, M., Wielinga, B., Fendorf, S., and Matin, A. (2000). Purification to homogeneity and characterization of a novel Pseudomonas putida chromate reductase. Appl. Environ. Microbiol. 66, 1788–1795. doi: 10.1128/AEM.66.5.1788-1795.2000
Pechancová, R., Pluháček, T., and Milde, D. (2019). Recent advances in chromium speciation in biological samples. Spectrochim. Acta B At. Spectrosc. 152, 109–122. doi: 10.1016/j.sab.2018.12.008
Poljsak, B., Pócsi, I., Raspor, P., and Pesti, M. (2010). Interference of chromium with biological systems in yeasts and fungi: a review. J. Basic Microbiol. 50, 21–36. doi: 10.1002/jobm.200900170
Poopal, A. C., and Laxman, R. S. (2009). Studies on biological reduction of chromate by Streptomyces griseus. J. Hazard. Mater. 169, 539–545. doi: 10.1016/j.jhazmat.2009.03.126
Pophali, A., Singh, S., and Verma, N. (2020). A dual photoelectrode-based double-chambered microbial fuel cell applied for simultaneous COD and Cr(VI) reduction in wastewater. Int. J. Hydrogen Energy 46, 3160–3170. doi: 10.1016/j.ijhydene.2020.06.162
Pradhan, S. K., Singh, N. R., Rath, B. P., and Thatoi, H. (2016). Bacterial chromate reduction: a review of important genomic, proteomic, and bioinformatic analysis. Crit. Rev. Environ. Sci. Technol. 46, 1659–1703. doi: 10.1080/10643389.2016.1258912
Pure Earth (2015). The New Top Six Toxic Threats: A Priority List For Remediation, World’s Worst Pollution Problems Report. Available online at: http://www.worstpolluted.org/docs/WWPP_2015_Final.pdf
Puzon, G. J., Petersen, J. N., Roberts, A. G., Kramer, D. M., and Xun, L. (2002). A bacterial flavin reductase system reduces chromate to a soluble chromium (III)–NAD+ complex. Biochem. Biophys. Res. Communic. 294, 76–81. doi: 10.1016/S0006-291X(02)00438-2
Qian, J., Zhou, J., Wang, L., Wei, L., Li, Q., Wang, D., et al. (2017). Direct Cr(VI) bio-reduction with organics as electron donor by anaerobic sludge. Chem. Eng. J. 309, 330–338. doi: 10.1016/j.cej.2016.10.077
Rager, J. E., Suh, M., Chappell, G. A., Thompson, C. M., and Proctor, D. M. (2019). Review of transcriptomic responses to hexavalent chromium exposure in lung cells supports a role of epigenetic mediators in carcinogenesis. Toxicol. Lett. 305, 40–50. doi: 10.1016/j.toxlet.2019.01.011
Rahman, Z., and Singh, V. P. (2014). Cr(VI) reduction by Enterobacter sp. DU17 isolated from the tannery waste dump site and characterization of the bacterium and the Cr(VI) reductase. Int. Biodeterior. Biodegrad. 91, 97–103. doi: 10.1016/j.ibiod.2014.03.015
Rahman, Z., and Singh, V. P. (2019). The relative impact of toxic heavy metals (THMs)(arsenic (As), cadmium (Cd), chromium (Cr)(VI), mercury (Hg), and lead (Pb)) on the total environment: an overview. Environ. Monit. Assess. 191:419. doi: 10.1007/s10661-019-7528-7
Ramírez-Díaz, M. I., Díaz-Pérez, C., Vargas, E., Riveros-Rosas, H., Campos-García, J., and Cervantes, C. (2008). Mechanisms of bacterial resistance to chromium compounds. Biometals 21, 321–332. doi: 10.1007/s10534-007-9121-8
Ray, A. E., Connon, S. A., Neal, A. L., Fujita, Y., Cummings, D. E., Ingram, J. C., et al. (2018). Metal transformation by a novel Pelosinus isolate from a subsurface environment. Front. Microbiol. 9:1689. doi: 10.3389/fmicb.2018.01689
Reijonen, I., and Hartikainen, H. (2016). Oxidation mechanisms and chemical bioavailability of chromium in agricultural soil–pH as the master variable. Appl. Geochem. 74, 84–93.
Richardson, D. J., Butt, J. N., and Clarke, T. A. (2013). Controlling electron transfer at the microbe–mineral interface. Proc. Natl. Acad. Sci. U.S.A. 110, 7537–7538. doi: 10.1073/pnas.1305244110
Robins, K. J., Hooks, D. O., Rehm, B. H., and Ackerley, D. F. (2013). Escherichia coli NemA is an efficient chromate reductase that can be biologically immobilized to provide a cell free system for remediation of hexavalent chromium. PLoS One 8:e59200. doi: 10.1371/journal.pone.0059200
RoyChowdhury, A., Datta, R., and Sarkar, D. (2018). “Heavy metal pollution and remediation,” in Green Chemistry, eds B. Török and T. Dransfield (Amsterdam: Elsevier), 359–373.
Saha, R., Saha, I., Nandi, R., Ghosh, A., Basu, A., Ghosh, S. K., et al. (2013). Application of Chattim tree (devil tree, Alstonia scholaris) saw dust as a biosorbent for removal of hexavalent chromium from contaminated water. Can. J. Chem. Eng. 91, 814–821.
Salamanca, D., Strunk, N., and Engesser, K. H. (2013). Chromate reduction in anaerobic systems by bacterial strain Pseudomonas aeruginosa CRM100. Chem. Ing. Tech. 85, 1575–1580. doi: 10.1002/cite.201200144
Santos, T. C., Silva, M. A., Morgado, L., Dantas, J. M., and Salgueiro, C. A. (2015). Diving into the redox properties of Geobacter sulfurreducens cytochromes: a model for extracellular electron transfer. Dalton Trans. 44, 9335–9344. doi: 10.1039/C5DT00556F
Sarangi, A., and Krishnan, C. (2016). Detoxification of hexavalent chromium by Leucobacter sp. uses a reductase with specificity for dihydrolipoamide. J. Basic Microbiol. 56, 175–183. doi: 10.1002/jobm.201500285
Satarupa, D., and Paul, A. K. (2013). Hexavalent chromium reduction by aerobic heterotrophic bacteria indigenous to chromite mine overburden. Braz. J. Microbiol. 44, 307–315. doi: 10.1590/S1517-83822013000100045
Shaheen, S. M., Niazi, N. K., Hassan, N. E., Bibi, I., Wang, H., Tsang, D. C., et al. (2019). Wood-based biochar for the removal of potentially toxic elements in water and wastewater: a critical review. Int. Mater. Rev. 64, 216–247. doi: 10.1080/09506608.2018.1473096
Shahid, M., Shamshad, S., Rafiq, M., Khalid, S., Bibi, I., Niazi, N. K., et al. (2017). Chromium speciation, bioavailability, uptake, toxicity and detoxification in soil-plant system: a review. Chemosphere 178, 513–533. doi: 10.1016/j.chemosphere.2017.03.074
Shapovalova, A. A., Khijniak, T. V., Tourova, T. P., and Sorokin, D. Y. (2009). Halomonas chromatireducens sp. nov., a new denitrifying facultatively haloalkaliphilic bacterium from solonchak soil capable of aerobic chromate reduction. Microbiol. 78, 102–111.
Sharma, K. (2002). Microbial Cr(VI) Reduction: Role of Electron Donors, Acceptors, and Mechanisms, With Special Emphasis on Clostridium spp. Doctoral dissertation, University of Florida, Gainesville, FL.
Shi, C., Cui, Y., Lu, J., and Zhang, B. (2020a). Sulfur-based autotrophic biosystem for efficient Vanadium (V) and Chromium (VI) reductions in groundwater. Chem. Eng. J. 395:124972. doi: 10.1016/j.cej.2020.124972
Shi, J., Zhang, B., Qiu, R., Lai, C., Jiang, Y., He, C., et al. (2019). Microbial chromate reduction coupled to anaerobic oxidation of elemental sulfur or zerovalent iron. Environ. Sci. Technol. 53, 3198–3207. doi: 10.1021/acs.est.8b05053
Shi, K., Dai, X., Fan, X., Zhang, Y., Chen, Z., and Wang, G. (2020b). Simultaneous removal of chromate and arsenite by the immobilized Enterobacter bacterium in combination with chemical reagents. Chemosphere 259:127428. doi: 10.1016/j.chemosphere.2020.127428
Shi, L., Richardson, D. J., Wang, Z., Kerisit, S. N., Rosso, K. M., Zachara, J. M., et al. (2009). The roles of outer membrane cytochromes of Shewanella and Geobacter in extracellular electron transfer. Environ. Microbiol. Rep. 1, 220–227. doi: 10.1111/j.1758-2229.2009.00035.x
Shi, Y., Chai, L., Yang, Z., Jing, Q., Chen, R., and Chen, Y. (2012). Identification and hexavalent chromium reduction characteristics of Pannonibacter phragmitetus. Bioproc. Biosyst. Eng. 35, 843–850. doi: 10.1007/s00449-011-0668-y
Singh, S., Kang, S. H., Mulchandani, A., and Chenm, W. (2008). Bioremediation: environmental clean-up through pathway engineering. Curr. Opin. Biotechnol. 19, 437–444. doi: 10.1016/j.copbio.2008.07.012
Smith, W. A., Apel, W. A., Petersen, J. N., and Peyton, B. M. (2002). Effect of carbon and energy source on bacterial chromate reduction. Bioremed. J. 6, 205–215. doi: 10.1080/10889860290777567
Smutok, O., Broda, D., Smutok, H., Dmytruk, K., and Gonchar, M. (2011). Chromate-reducing activity of Hansenula polymorpha recombinant cells over-producing flavocytochrome b2. Chemosphere 83, 449–454. doi: 10.1016/j.chemosphere.2010.12.078
Sobol, Z., and Schiestl, R. H. (2012). Intracellular and extracellular factors influencing Cr (VI and Cr (III) genotoxicity. Environ. Mol. Mutagen. 53, 94–100. doi: 10.1002/em.20679
Song, H., Liu, Y., Xu, W., Zeng, G., Aibibu, N., Xu, L., et al. (2009). Simultaneous Cr(VI) reduction and phenol degradation in pure cultures of Pseudomonas aeruginosa CCTCC AB91095. Biores. Technol. 100, 5079–5084. doi: 10.1016/j.biortech.2009.05.060
Sultan, S., and Hasnain, S. (2007). Reduction of toxic hexavalent chromium by Ochrobactrum intermedium strain SDCr-5 stimulated by heavy metals. Biores. Technol. 98, 340–344. doi: 10.1016/j.biortech.2005.12.025
Sun, F. L., Fan, L. L., Wang, Y. S., and Huang, L. Y. (2019). Metagenomic analysis of the inhibitory effect of chromium on microbial communities and removal efficiency in A2O sludge. J. Hazard. Mater. 368, 523–529. doi: 10.1016/j.jhazmat.2019.01.076
Sun, M., Zhang, G., Qin, Y., Cao, M., Liu, Y., Li, J., et al. (2015). Redox conversion of chromium (VI) and arsenic (III) with the intermediates of chromium (V) and arsenic (IV) via AuPd/CNTs electrocatalysis in acid aqueous solution. Environ. Sci. Technol. 49, 9289–9297. doi: 10.1021/acs.est.5b01759
Tan, H., Wang, C., Zeng, G., Luo, Y., Li, H., and Xu, H. (2020). Bioreduction and biosorption of Cr(VI) by a novel Bacillus sp. CRB-B1 strain. J. Hazard. Mater. 386:121628. doi: 10.1016/j.jhazmat.2019.121628
Tanaka, K., Yokoe, S., Igarashi, K., Takashino, M., Ishikawa, M., Hori, K., et al. (2018). Extracellular electron transfer via outer membrane cytochromes in a methanotrophic bacterium Methylococcus capsulatus (Bath). Front. Microbiol. 9:2905. doi: 10.3389/fmicb.2018.02905
Thatheyus, A. J., and Ramya, D. (2016). Biosorption of chromium using bacteria: an overview. Sci. Int. 4, 74–79.
Thatoi, H., Das, S., Mishra, J., Rath, B. P., and Das, N. (2014). Bacterial chromate reductase, a potential enzyme for bioremediation of hexavalent chromium: a review. J. Environ. Manag. 146, 383–399. doi: 10.1016/j.jenvman.2014.07.014
Thomas, L., Ram, H., Kumar, A., and Singh, V. P. (2016). Production, optimization, and characterization of organic solvent tolerant cellulases from a lignocellulosic waste-degrading actinobacterium, Promicromonospora sp. VP111. Appl. Biochem. Biotechnol. 179, 863–879. doi: 10.1007/s12010-016-2036-5
Truskewycz, A., Gundry, T. D., Khudur, L. S., Kolobaric, A., Taha, M., Aburto-Medina, A., et al. (2019). Petroleum hydrocarbon contamination in terrestrial ecosystems—fate and microbial responses. Molecules 24:3400. doi: 10.3390/molecules24183400
Tumolo, M., Ancona, V., De Paola, D., Losacco, D., Campanale, C., Massarelli, C., et al. (2020). Chromium pollution in European water, sources, health risk, and remediation strategies: an overview. Int. J. Environ. Res. Public Health 17:5438. doi: 10.3390/ijerph17155438
US EPA (1998). “Toxicological review of hexavalent chromium (CAS No. 18540-29-9),” in Support of Summary Information on the Integrated Risk Information System (IRIS), (Washington, DC: U.S. Environmental Protection Agency).
Viti, C., and Giovannetti, L. (2007). “Bioremediation of soils polluted with hexavalent chromium using bacteria: a challenge,” in Environmental Bioremediation Technologies, eds S. N. Singh and R. D. Tripathi (Berlin: Springer), 57–76.
Viti, C., Marchi, E., Decorosi, F., and Giovannetti, L. (2014). Molecular mechanisms of Cr(VI) resistance in bacteria and fungi. FEMS Microbiol. Rev. 38, 633–659. doi: 10.1111/1574-6976.12051
Voordeckers, J. W., Kim, B. C., Izallalen, M., and Lovley, D. R. (2010). Role of Geobacter sulfurreducens outer surface c-type cytochromes in reduction of soil humic acid and anthraquinone-2, 6-disulfonate. Appl. Environ. Microbiol. 76, 2371–2375. doi: 10.1128/AEM.02250-09
Wang, G., Zhang, B., Li, S., Yang, M., and Yin, C. (2017a). Simultaneous microbial reduction of vanadium (V) and chromium (VI) by Shewanella loihica PV-4. Biores. Technol. 227, 353–358. doi: 10.1016/j.biortech.2016.12.070
Wang, P. C., Mori, T., Toda, K., and Ohtake, H. (1990). Membrane-associated chromate reductase activity from Enterobacter cloacae. J. Bacteriol. 172, 1670–1672. doi: 10.1128/jb.172.3.1670-1672.1990
Wang, P., and Jia, H. (2007). “Power-generation from biorenewable resources: biocatalysis in biofuel cells,” in Bioprocessing for Value-Added Products from Renewable Resources, ed. S.-T. Yang (Amsterdam: Elsevier), 507–525.
Wang, Q., Huang, L., Pan, Y., Quan, X., and Puma, G. L. (2017b). Impact of Fe (III) as an effective electron-shuttle mediator for enhanced Cr(VI) reduction in microbial fuel cells: reduction of diffusional resistances and cathode overpotentials. J. Hazard. Mater. 321, 896–906. doi: 10.1016/j.jhazmat.2016.10.011
Wang, S., Zhang, B., Diao, M., Shi, J., Jiang, Y., Cheng, Y., et al. (2018). Enhancement of synchronous bio-reductions of vanadium (V) and chromium (VI) by mixed anaerobic culture. Environ. Pollut. 242, 249–256.
Wang, X., Aulenta, F., Puig, S., Esteve-Núñez, A., He, Y., Mu, Y., et al. (2020). Microbial electrochemistry for bioremediation. Environ. Sci. Ecotechnol. 1:100013.
Wani, P. A., Wani, J. A., and Wahid, S. (2018). Recent advances in the mechanism of detoxification of genotoxic and cytotoxic Cr(VI) by microbes. J. Environ. Chem. Eng. 6, 3798–3807. doi: 10.1016/j.jece.2018.05.042
Wani, R., Kodam, K. M., Gawai, K. R., and Dhakephalkar, P. K. (2007). Chromate reduction by Burkholderia cepacia MCMB-821, isolated from the pristine habitat of alkaline crater lake. Appl. Microbiol. Biotechnol. 75, 627–632. doi: 10.1007/s00253-007-0862-7
Wen, C., Sheng, H., Ren, L., Dong, Y., and Dong, J. (2017). Study on the removal of hexavalent chromium from contaminated groundwater using emulsified vegetable oil. Proc. Saf. Environ. Protect. 109, 599–608. doi: 10.1016/j.psep.2017.05.004
Wilbur, S., Abadin, H., Fay, M., Yu, D., Tencza, B., Ingerman, L., et al. (2012). Toxicological Profile for Chromium, (Atlanta, GA: US Department of Health and Human Services, Public Health Service, Agency for Toxic Substances and Disease Registry).
Wolf, M., Kappler, A., Jiang, J., and Meckenstock, R. U. (2009). Effects of humic substances and quinones at low concentrations on ferrihydrite reduction by Geobacter metallireducens. Environ. Sci. Technol. 43, 5679–5685. doi: 10.1021/es803647r
Xafenias, N., Zhang, Y., and Banks, C. J. (2013). Enhanced performance of hexavalent chromium reducing cathodes in the presence of Shewanella oneidensis MR-1 and lactate. Environ. Sci. Technol. 47, 4512–4520. doi: 10.1021/es304606u
Xia, X., Wu, S., Li, N., Wang, D., Zheng, S., and Wang, G. (2018). Novel bacterial selenite reductase CsrF responsible for Se (IV) and Cr(VI) reduction that produces nanoparticles in Alishewanella sp. WH16-1. J. Hazard. Mater. 342, 499–509. doi: 10.1016/j.jhazmat.2017.08.051
Xiao, W., Ye, X., Yang, X., Li, T., Zhao, S., and Zhang, Q. (2015). Effects of alternating wetting and drying versus continuous flooding on chromium fate in paddy soils. Ecotoxicol. Environ. Saf. 113, 439–445. doi: 10.1016/j.ecoenv.2014.12.030
Xu, J., Dai, Y., Shi, Y., Zhao, S., Tian, H., Zhu, K., et al. (2020). Mechanism of Cr(VI) reduction by humin: role of environmentally persistent free radicals and reactive oxygen species. Sci. Tot. Environ. 725, 138413. doi: 10.1016/j.scitotenv.2020.138413
Xu, L., Luo, M., Li, W., Wei, X., Xie, K., Liu, L., et al. (2011). Reduction of hexavalent chromium by Pannonibacter phragmitetus LSSE-09 stimulated with external electron donors under alkaline conditions. J. Hazard. Mater. 185, 1169–1176. doi: 10.1016/j.jhazmat.2010.10.028
Xu, X., Huang, H., Zhang, Y., Xu, Z., and Cao, X. (2019). Biochar as both electron donor and electron shuttle for the reduction transformation of Cr(VI) during its sorption. Environ. Pollut. 244, 423–430. doi: 10.1016/j.envpol.2018.10.068
Yan, F. F., He, Y. R., Wu, C., Cheng, Y. Y., Li, W. W., and Yu, H. Q. (2014). Carbon nanotubes alter the electron flow route and enhance nitrobenzene reduction by Shewanella oneidensis MR-1. Environ. Sci. Technol. Lett. 1, 128–132. doi: 10.1021/ez4000093
Yan, J., Ye, W., Liang, X., Wang, S., Xie, J., Zhong, K., et al. (2020). Enhanced reduction of sulfate and chromium under sulfate-reducing condition by synergism between extracellular polymeric substances and graphene oxide. Environ. Res. 183:109157. doi: 10.1016/j.envres.2020.109157
Yan, X., Song, M., Zhou, M., Ding, C., Wang, Z., Wang, Y., et al. (2019). Response of Cupriavidus basilensis B-8 to CuO nanoparticles enhances Cr(VI) reduction. Sci. Tot. Environ. 688, 46–55. doi: 10.1016/j.scitotenv.2019.05.438
Yang, C., Zheng, M. X., Zhang, Y., Xi, B. D., Tian, Z. F., and He, X. S. (2020). Bioreduction of hexavalent chromium: effect of compost-derived humic acids and hematite. Chin. Chem. Lett. 31, 2693–2697. doi: 10.1016/j.cclet.2020.04.001
Yang, J., He, M., and Wang, G. (2009). Removal of toxic chromate using free and immobilized Cr(VI)-reducing bacterial cells of Intrasporangium sp. Q5-1. World J. Microbiol. Biotechnol. 25, 1579–1587. doi: 10.1016/j.biortech.2016.09.037
Yu, X., Jiang, Y., Huang, H., Shi, J., Wu, K., Zhang, P., et al. (2016). Simultaneous aerobic denitrification and Cr(VI) reduction by Pseudomonas brassicacearum LZ-4 in wastewater. Biores. Technol. 221, 121–129. doi: 10.1016/j.biortech.2016.09.037
Zhang, B., Feng, C., Ni, J., Zhang, J., and Huang, W. (2012). Simultaneous reduction of vanadium (V) and chromium (VI) with enhanced energy recovery based on microbial fuel cell technology. J. Power Sourc. 204, 34–39. doi: 10.1016/j.jpowsour.2012.01.013
Zhang, B., Wang, Z., Shi, J., and Dong, H. (2020). Sulfur-based mixotrophic bio-reduction for efficient removal of chromium (VI) in groundwater. Geochim. Cosmochim. Acta 268, 296–309. doi: 10.1016/j.gca.2019.10.011
Zhang, H. K., Lu, H., Wang, J., Zhou, J. T., and Sui, M. (2014). Cr(VI) reduction and Cr (III) immobilization by Acinetobacter sp. HK-1 with the assistance of a novel quinone/graphene oxide composite. Environ. Sci. Technol. 48, 12876–12885. doi: 10.1021/es5039084
Zhang, Q., Amor, K., Galer, S. J., Thompson, I., and Porcelli, D. (2018). Variations of stable isotope fractionation during bacterial chromium reduction processes and their implications. Chem. Geol. 481, 155–164. doi: 10.1016/j.chemgeo.2018.02.004
Zhao, S., Chen, Z., Khan, A., Wang, J., Kakade, A., Kulshrestha, S., et al. (2020a). Elevated Cr(VI) reduction in a biocathode microbial fuel cell without acclimatization inversion based on strain Corynebacterium vitaeruminis LZU47-1. Int. J. Hydrogen Energy 46, 3193–3203. doi: 10.1016/j.ijhydene.2020.05.254
Zhao, Z., Sun, C., Li, Y., Yu, Q., Jin, Z., Wang, M., et al. (2020b). Driving microbial sulfur cycle for phenol degradation coupled with Cr(VI) reduction via Fe (III)/Fe (II) transformation. Chem. Eng. J. 393, 124801. doi: 10.1016/j.cej.2020.124801
Zheng, X., Yuan, D., Li, Y., and Liu, C. (2019). Exploration of the reduction mechanism of Cr(VI) in anaerobic hydrogen fermenter. Environ. Pollut. 254:113042. doi: 10.1016/j.envpol.2019.113042
Zhong, L., Lai, C. Y., Shi, L. D., Wang, K. D., Dai, Y. J., Liu, Y. W., et al. (2017). Nitrate effects on chromate reduction in a methane-based biofilm. Water Res. 115, 130–137. doi: 10.1016/j.watres.2017.03.003
Zhu, W., Chai, L., Ma, Z., Wang, Y., Xiao, H., and Zhao, K. (2008). Anaerobic reduction of hexavalent chromium by bacterial cells of Achromobacter sp. strain Ch1. Microbiol. Res. 163, 616–623. doi: 10.1016/j.micres.2006.09.008
Keywords: Cr(VI) reduction, chemical-assisted, electron donors, electron mediators, microbially mediated process
Citation: Rahman Z and Thomas L (2021) Chemical-Assisted Microbially Mediated Chromium (Cr) (VI) Reduction Under the Influence of Various Electron Donors, Redox Mediators, and Other Additives: An Outlook on Enhanced Cr(VI) Removal. Front. Microbiol. 11:619766. doi: 10.3389/fmicb.2020.619766
Received: 21 October 2020; Accepted: 16 December 2020;
Published: 28 January 2021.
Edited by:
Jian Chen, Florida International University, United StatesCopyright © 2021 Rahman and Thomas. This is an open-access article distributed under the terms of the Creative Commons Attribution License (CC BY). The use, distribution or reproduction in other forums is permitted, provided the original author(s) and the copyright owner(s) are credited and that the original publication in this journal is cited, in accordance with accepted academic practice. No use, distribution or reproduction is permitted which does not comply with these terms.
*Correspondence: Zeeshanur Rahman, emVlc2hhbjg4aW5kQGdtYWlsLmNvbQ==
†These authors have contributed equally to this work
Disclaimer: All claims expressed in this article are solely those of the authors and do not necessarily represent those of their affiliated organizations, or those of the publisher, the editors and the reviewers. Any product that may be evaluated in this article or claim that may be made by its manufacturer is not guaranteed or endorsed by the publisher.
Research integrity at Frontiers
Learn more about the work of our research integrity team to safeguard the quality of each article we publish.