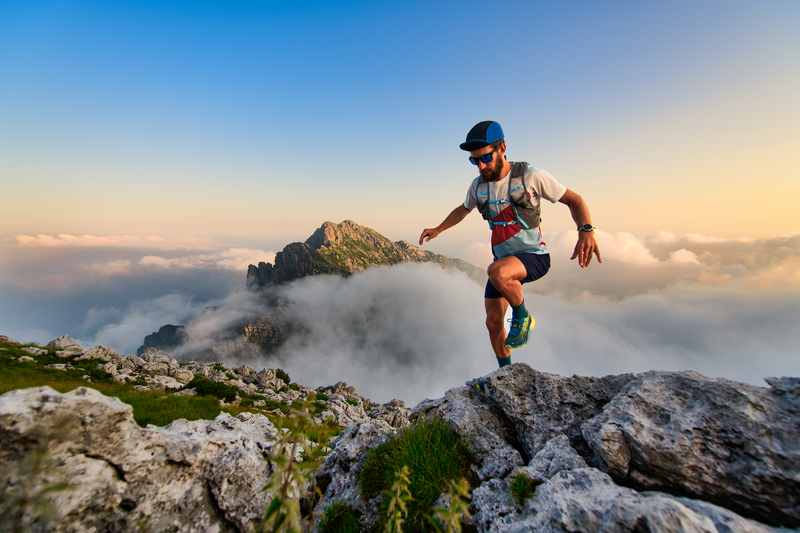
95% of researchers rate our articles as excellent or good
Learn more about the work of our research integrity team to safeguard the quality of each article we publish.
Find out more
ORIGINAL RESEARCH article
Front. Microbiol. , 03 December 2020
Sec. Extreme Microbiology
Volume 11 - 2020 | https://doi.org/10.3389/fmicb.2020.614227
This article is part of the Research Topic Archaea in the Environment: Views on Archaeal Distribution, Activity, and Biogeography View all 10 articles
A cement-based geological disposal facility (GDF) is one potential option for the disposal of intermediate level radioactive wastes. The presence of both organic and metallic materials within a GDF provides the opportunity for both acetoclastic and hydrogenotrophic methanogenesis. However, for these processes to proceed, they need to adapt to the alkaline environment generated by the cementitious materials employed in backfilling and construction. Within the present study, a range of alkaline and neutral pH sediments were investigated to determine the upper pH limit and the preferred route of methane generation. In all cases, the acetoclastic route did not proceed above pH 9.0, and the hydrogenotrophic route dominated methane generation under alkaline conditions. In some alkaline sediments, acetate metabolism was coupled to hydrogenotrophic methanogenesis via syntrophic acetate oxidation, which was confirmed through inhibition studies employing fluoromethane. The absence of acetoclastic methanogenesis at alkaline pH values (>pH 9.0) is attributed to the dominance of the acetate anion over the uncharged, undissociated acid. Under these conditions, acetoclastic methanogens require an active transport system to access their substrate. The data indicate that hydrogenotrophic methanogenesis is the dominant methanogenic pathway under alkaline conditions (>pH 9.0).
Globally, geological disposal is largely the preferred choice for the management of intermediate level radioactive wastes (ILWs; IAEA, 2011) with a number of countries pursuing the establishment of geological disposal facilities (GDFs; Faybishenko et al., 2016). The exact design of these facilities is dependent on the host geology; however, there are often a number of common features, including the use of cementitious construction and backfill materials, the presence of metal in the construction, container and waste components, and organic, primarily cellulose, containing wastes.
The presence of metallic and organic materials means that abiotic and biotic gas generating processes (NEA, 2014) are likely to proceed within a GDF resulting in gas generation being evaluated in a number of international waste management programs (Felicione et al., 2003; NDA, 2010; Avis et al., 2011; Summerling, 2013; Poller et al., 2016). The anaerobic corrosion of metals will generate molecular hydrogen (NDA, 2010; Libert et al., 2011), which can in turn, act as an electron donor for hydrogenotrophic methanogenesis (Libert et al., 2011; Bagnoud et al., 2016). The alkaline conditions generated by the cementitious materials will promote the alkaline hydrolysis of cellulosic wastes (Humphreys et al., 2010). This abiotic process generates a range of cellulose degradation products (CDPs; Rout et al., 2015b; Charles et al., 2019) collectively which are dominated (>70%) by isosaccharinic acids (ISAs; Almond et al., 2012).
Recent research has focused on the use of lime kiln waste sites to provide an insight into the biodegradation of CDP components under alkaline conditions (Milodowski et al., 2013; Charles et al., 2019). Despite the harsh geochemical environment (pH > 10.0), these sites support extensive and diverse bacterial and archaeal populations (Burke et al., 2012; Kyeremeh et al., 2016) capable of a wide range of metabolic and energy generating processes (Bassil et al., 2014; Rout et al., 2014, 2015a,b), including methanogenesis (Rout et al., 2015b). The information that is available for these sites suggests that the hydrogenotrophs dominate the methanogenic populations despite the availability of acetic acid and the absence of competition by other acetate consuming pathways (Charles et al., 2015; Rout et al., 2015b; Kyeremeh et al., 2016).
Given the importance of methanogenesis to gas generation within a GDF, a range of anthropogenic alkaline environments of various ages were investigated to determine the impact of environmental pH on the nature and extent of methanogenic activity.
The investigation involved sediments from three lime kiln waste sites (≈25 to ≈150 years old; New Lime sites, designated B, H, and T; Charles et al., 2019), five field kiln sites (Johnson, 2008; 200–300 years old; Old Lime sites, designated LK1, LK2, LK3, LK4, and LK5), and four steel industry waste sites (5 to ≈30 years old; Mayes et al., 2008; Steel sites, designated CW, CS, RC, and SC; see Supporting Information). In addition, a neutral pH, freshwater anaerobic sediment was employed as a control (Control). In the case of the New Lime, Steel, and Control sites, hand cored samples (dia. 30 mm) were taken at depth (~1 m). In the case of the Old Lime sites, samples were taken from a shallow depth of 10–30 cm using a stainless steel trowel. All sampling equipment was surface sterilize using a commercial hypochlorous acid spray (Redditch Medical Ltd., Stockton on Tees, UK). Sterile plastic sample containers were filled to maximum capacity with sediments and where possible with the associated pore waters in order to minimize the presence of oxygen. The pH values of the associated pore waters were determined in situ with a hand-held pH probe (Mettler Toledo, Columbus, OH, Unites States) and the pH of the soil/sediment determined using standard methods (B.S.I, 2005).
All microcosms employed in the study were incubated in the dark at 25°C, and the incubation time was dependent on the time taken for the microcosm to generate detectable methane and ranged from 2 to 8 weeks. Initial microcosms employing New Lime and Control sediments were established by mixing a soil/sediment sample (5 g) from each location with 5 ml of sterile, nitrogen purged mineral media (MM; Supplementary Tables S1, S2; B.S.I, 2005) adjusted to pH 10.0 with 4 M NaOH for New Lime microcosms and pH 7.0 for the Control microcosms. Slurries were then transferred to 100 ml crimp top bottles containing 100 ml of MM supplemented with 10% v/v CDP and a hydrogen:nitrogen (10:90) headspace (BOC Ltd., Guildford, UK). A CDP/hydrogen enrichment was chosen since this had previously been shown (Rout et al., 2014, 2015a; Kyeremeh et al., 2016) to support a diverse range of microbial processes and provided a source of fermentable substrates, acetate, and hydrogen. CDP was prepared as previously described (Rout et al., 2014, 2015a) All microcosm experiments were carried out in duplicate.
On the detection of methane initial enrichments were sub-cultured twice at the starting pH by inoculating sterile microcosms with 5 ml of the previous microcosm suspension (Supplementary Figure S1). This was carried out to reduce the impact of residual fermentable substrates, mineral components, and other electron acceptors [e.g., Iron (III) and sulphate] present within the inoculating sediment.
Once methane generation was detected at the starting pH, each sediment was sequentially exposed to a range of pH values ranging from 7.0 to 12.0 (Supplementary Figure S1) as described below. Every microcosm that was methane positive was sub-cultured at the next pH in the series and, hydrogen and acetate enrichments were created at the original pH (Supplementary Figure S1). The last pH in the series that failed to generate methane was still sub-cultured into hydrogen and acetate enrichments. These enrichments were performed by transferring 5 ml of the microcosm suspension to a 100 ml crimp top bottle containing 100 ml of MM with either a hydrogen: carbon dioxide: nitrogen (10:10:80; BOC Ltd., Guildford, United Kingdom) headspace or MM supplemented with Na-acetate (30 mM) with a 100% nitrogen headspace. A set of blank microcosms were also established as described above with MM, soil, and a 100% nitrogen atmosphere.
Headspace hydrogen and methane was determined via an Agilent GC6850 equipped with HP-PLOT/Q column with particle traps ((35 m × 0.32 mm × 20 μm), Agilent Technologies, Santa Clara, CA, United States) employing thermal conductivity detection. Liquid microcosm samples (1 ml) were also removed over the course of incubation using degassed syringes and needles. Acetic acid concentrations were determined using gas chromatography (Hewlett Packard Ltd., London, United Kingdom) with a flame Ionization detector (GC-FID) as described previously (Rout et al., 2015a). To determine the presence of acetoclastic methanogenesis the metabolic inhibitor methylflouride was employed at an initial concentration of 1% as described previously (Daebeler et al., 2013). Duplicate reactors without inhibitor addition were also prepared and served as a control. Methane produced from CO2 (mCO2) was calculated from inhibited microcosms002C and acetate-derived methane was calculated from methane production in uninhibited microcosms (macetate = mtotal − mCO2).
Total genomic DNA was extracted from both soil/sediment samples and methanogenic enrichment cultures using the PowerSoil DNA Isolation Kit (Qiagen, Manchester, UK). The enrichment cultures were sampled after 2 weeks of incubation by the centrifugation (10,000 × g) of the microcosm fluid to pellet the cells prior to DNA extraction. The V4 region of the 16S rRNA gene was then amplified by PCR using primers 341f and 805r (Takahashi et al., 2014). Next generation sequencing of PCR products was carried out by ChunLab (South Korea) using the Illumina MiSeq platform. Sequences were then paired and clustered into OTUs using DADA2 employing R3.2.5 (Callahan et al., 2016), before being identified using the MegaBLAST search strategies (Altschul et al., 1997).
Within microcosms utilizing CDP as a carbon source, the Control enrichments demonstrated a linear reduction in methane production between pH 7.0 and 11.0 (Figure 1), while the New Lime enrichments demonstrated an optimum pH of 9.0 for methane generation and a linear reduction down to pH 12.0. This optimum is below the in-situ pH of the New Lime sites (pH 11.5–13.0, Supplementary Table S3), suggesting that the in-situ populations have alternative strategies to manage these harsh pH values (Charles et al., 2017).
Figure 1. Methane production rates in CDP-fed microcosms employing Control and New Lime sediments operating between pH 7.0 and 12.0. Control microcosms (n = 2 at all pH values) had a pH optimum of 7.0 (n = 3), in contrast to the New Lime microcosms (n = 2 pH 7.0, n = 4 pH 8.0, n = 6 pH 9.0, n = 6 pH 10.0, and n = 2 pH 11.0) were optimal at pH 9.0. The upper pH limit for methanogenesis was pH 10.0 for the Control sediment, and this increased to pH 11.0 in the case of the New Lime sediments. No methane generation was detected at a pH >11.0 (“n” denotes the number of replicates analyzed).
Mean methane production values for those microcosms producing methane are presented (Figure 1) in order to emphasize the overall trends observed. Across the pH range investigated the microbial populations enriched from the three New Lime sites demonstrated different responses to changing pH with the oldest site (site B; see Supplementary Material) generating methane across the whole range and the other two sites generating methane across narrower pH ranges (Supplementary Figure S2).
In the Control sediment enrichments, the archaeal community composition was composed of almost equal proportions of acetoclastic to hydrogenotrophic methanogens (46:54%; Figure 2) at pH 7.0 and 8.0. As the enrichment pH became more alkaline (pH ≥ 9.0), the community composition shifted toward a more hydrogenotrophic population Figure 2 even though acetate was present in these cultures. The New Lime sediment enrichments were dominated by hydrogenotrophic methanogens (Figure 2), suggesting that the long-term exposure to alkaline pH had selected against acetoclastic methanogens in these sediments.
Figure 2. Methanogen communities in neutral and alkaline soil enrichments. When the neutral pH Control sediment was enriched with CDP the acetoclastic and hydrogenotrophic communities were present in roughly equal proportions at pH 7.0 and 8.0, respectively. As the pH increased to pH 10.0, the acetoclastic compostion fell as pH increased, with no methanogen community detectable at pH 11.0. In contrast the alkaline New Lime enrichments were dominated by hydrogenotrophic methanogens irrespective of enrichment pH (ND, none determined).
The trend toward hydrogenotrophic methanogenesis at alkaline pH was confirmed by the establishement of hydrogen and acetate enrichments from the CDP fed Control and New Lime enrichments at each pH. Within the H2:CO2 Control sediment enrichments, hydrogen consumption was at its highest at pH 7.0 at 243.3 μmol day−1, and became undetectable as pH increased to pH 11.0 (Figure 3A). The associated methanogenic communities were most diverse at pH 7.0 with 12 different methanogenic genera detected (Figure 3B). Methanobacterium spp. were the most dominant at this pH, comprising 60.7% of the community; with Methanoregula spp. (14.7%) and Methanosphaerula spp. (10.2%) also being prevalent within the community. As pH was increased through to pH 10.0, the loss of a number of the genera observed at pH 7.0 coincided with the further dominance of Methanobacterium spp., rising to 97.1% of the community at pH 10.0.
Figure 3. Hydrogen consumption rates (A) and methanogen populations (B) in H2/CO2 enriched microcosms from Control (n = 2) and New Lime (n = 6) soils. An increasing pH negatively impacted upon hydrogen consumption in Control soil enrichments (n = 2 at all pH values), while New Lime sediments (n = 4 pH 7.0, n = 4 pH 8.0, n = 6 pH 9.0, n = 6 pH 10.0, n = 4 pH 11.0) demonstrated an optimum pH of 9.0. Across all enrichments, Methanobacterium sp. were the dominant genus. ND, none detected (“n” denotes the number of replicates analyzed).
These findings were reinforced by the H2:CO2 New Lime enrichments in which Methanobacterium spp. were the dominant taxa detected across all pH (7.0–11.0) enrichments (Figure 3B). A negative relationship between hydrogen consumption and increasing pH was seen in these enrichments as pH decreased for pH 9.0–11.0, however hydrogen consumption was still detected at pH 11.0 (77.0 μmol day−1). The optimum pH for hydrogenotrophic methanogenesis was pH 9.0 with a hydrogen consumption of 167.5 μmol day−1. The alkaliphilic nature of the methanogenic population in the New Lime sediments was indicated by the fact that the rates of hydrogen metabolism decreased as the pH fell below pH 9.0 (Figure 3A). The data here reiterate that the optimum pH for hydrogenotrophic methanogenesis is pH 9.0 for communities of this type, and that it is not the availability of substrate from fermentation (such as CDP) that is a limiting factor. As was the case with the CDP reactors, not all the New Lime enrichments were active across the whole pH range (Supplementary Figure S3).
Acetate fed control enrichments contained both acetoclastic and hydrogenotrophic methanogens (Figure 4B). Hydrogenotrophic methanogens are able to generate methane from acetate via syntrophic acetate oxidation (SAO). SAO is an endergonic reaction with a Gibbs free energy of +104.6 kJ mol−1 and is, therefore, energetically less favorable than acetoclastic methanogenesis; however, when the oxidation of acetate is coupled to hydrogenotrophic methanogenesis, the total combined reaction is exergonic (ΔG0' = −31 kJ mol−1; Hattori, 2008). Few SAO bacteria have been described, but SAO is associated with the members of the phylum Firmicutes (Hattori et al., 2000; Balk et al., 2002; Westerholm et al., 2010; Manzoor et al., 2018). In the acetate fed Control enrichments (Figure 4A), a linear reduction (R2 = 0.97) in the rate of acetate removal was observed between pH 7.0 and 9.0 from 182.7 to 33.7 μmoles day−1; with a cessation of activity at pH 10.0. Methanosarcina spp. contributed 65.1% of the pH 7.0 and 79.3% of the pH 8.0 Control enrichment populations (Figure 4B). This fell to 7.1% within the pH 9.0 enrichment. The fall in acetate consumption between pH 7.0 and 8.0 coincided with the loss of both Methanospirillium and Methanosaeta spp. and a reduced proportion of Methanosarcina between pH 8.0 and 9.0. The loss of Methanospirillium spp. may suggest that SAO was active at pH 7.0 in these systems but was not able to adjust to the increase in pH.
Figure 4. Acetate consumption rates (A) and methanogen populations (B) in sodium acetate enriched microcosms from Control and New lime soils. Increasing pH negatively impacted upon acetate consumption with no acetate consumption observed above pH 9.0 in either the Control (n = 2 at all pH values) enrichments or the New Lime enrichments (n = 2 pH 7.0, n = 2 pH 8.0). Methanosarcina spp. and Methanobacterium spp. were the dominant genera in Control enrichments while Methanobacterium sp. were dominant in the New Lime enrichments. ND, none detected (“n” denotes the number of replicates analyzed).
Low levels of acetate consumption were only observed at both pH 8.0 (36.4 μmoles day−1) and pH 7.0 (23.6 μmoles day−1) in acetate enrichments from two of the three New Lime sites. However, the methanogenic populations in these enrichments were dominated by the hydrogenotrophic (Liu and Whitman, 2008; Dridi et al., 2012) Methanobacterium, Methanoculleus, and Methanomassiliicossus spp., with the potentially acetoclastic Methanosarcina spp. representing only 1.2% of the total archaeal populations at these pH values (Figure 4B). However, the members of the phylum Firmicutes which comprised 30–40% of the total populations of the pH 8.0 and 7.0 New Lime acetate enrichments, suggesting that SAO may be responsible for the low levels of acetate removal in these enrichments.
To investigate the role of in the acetate fed enrichments, the pH 7.0 acetate fed New Lime enrichments were sub-cultured in the presence of acetate and fluoromethane, an inhibitor of acetoclastic methanogenesis (Agneessens et al., 2018). Within these enrichments (Supplementary Figure S4), acetate removal was observed in both the presence and absence of inhibitor (removal rates: presence: 4.1 × 10−2 ± 4 × 10−3 mmol day−1; absence: 3.5 × 10−2 ± 4 × 10−3 mmol day−1), suggesting that acetate removal in these sediments was SAO linked. The addition of fluoromethane to pH 7.0 acetate fed Control enrichment saw a cessation of acetate removal, indicating that in this case, acetoclastic methanogens was the dominant acetate removal process even though the presence of hydrogenotrophs such as Methanospirillium spp. may suggest some SAO activities (Supplementary Figure S5). These results suggest that the role of SAO in methanogen generation in alkaline environments warrants further study.
In order to determine the extent to which alkaline pH impacts upon acetoclastic methanogenesis across a wider selection of anthropogenic alkaline environments with more diverse methanogenic populations, sediments from the Steel and Old Lime sites were used to establish microcosms at pH 7.0 and 10.0. The Old Lime sediments (n = 5) had in-situ pH values closer to circum-neutral pH values, suggesting that the bulk environment had recovered from exposure to alkaline contamination. However, lime fragments are likely to be present within these sediments, suggesting that alkaline microsites will persist. The enrichments from these sediments were similar to the Control sediments, with both acetate and hydrogen consumptions being negatively impacted by an increase in pH from pH 7.0 to 10.0 (Figure 5). In particular, no acetate consumption or methane production was observed at pH 10.0. The acetate consumption rates at pH 7.0 were 2.0 × 10−2 (±7.7 × 10−3) day−1 and this coincided with the detection of Methanoculleus sp. (70.5%), Methanosarcina sp. (16.6%), and Methanomasiliicoccus sp. (11.6%). Hydrogen consumption could still be detected within the pH 10.0 enrichments; however, the rate fell from that observed at pH 7.0 [43.1 (±6.4) μmol day−1] to 17.4 (±4.6) μmol day−1. At both pH 7.0 and 10.0, Methanoculleus sp. dominated the community composition, representing 61.6 and 89.8% of the communities, respectively.
Figure 5. Acetate and hydrogen consumption from microcosms prepared using Old Lime and Steel site sediments as an enrichment inoculum. The pattern observed in the Old Lime (n = 10) and Steel (n = 8) sediments mirrored that seen with the New Lime and Control sediments with acetoclastic methanogenesis absent at alkaline pH (“n” denotes the number of replicates analyzed).
The pH of the Steel sediments ranged from pH 9.5 to 12.9. The enrichment of these sediments with either acetate or H2:CO2 demonstrated similar behavior to that observed with the New Lime sediments, again no acetate consumption was detected at pH 10.0 but was observed at pH 7.0 with a rate of 36.5 (±2.0) μmol day−1. At pH 7.0, acetoclastic communities from the Steel sites were dominated by Methanosarcina sp. (78.8%) with Methanobacterium sp. comprising 20.6% of the community. In contrast to the New Lime enrichments (Figure 3A), the Steel site sediments (Figure 5) demonstrated a greater rate of hydrogen consumption at pH 7.0 (90.8 ± 1.9 μmol day−1) than at pH 10.0 (85.1 ± 3.2 μmol day−1), although these were not significantly different (p = 0.105). The hydrogenotrophic methanogenic microcosms were again dominated by Methanobacterium sp.
Overall the results indicate that hydrogenotrophic methanogenesis is favored over acetoclastic methanogenesis at alkaline pH (>9.0). This observation is consistent across a wide range of calcium based anthropogenic sites of different origins and ages. Under the prevailing geochemical conditions created at these pH values the acetoclastic methanogenic pathway is unable to access its substrate due to the absence of undissociated acetic acid at these pH values.
The data presented here suggest that an alkaline pH >9.0 results in a methanogen community that is dominated by hydrogenotrophs, with populations enhanced by the activity of SAO bacteria. The methanogen populations from high pH and neutral sediments exposed to alkaline pH were both dominated by Methanobacterium sp., and in the case of the New Lime sediment communities, were capable of hydrogenotrophic methanogenesis at pH 11.0. Members of the Methanobacteriales have also been detected within a range of hyperalkaline environments resulting from serpentinisation processes (Brazelton et al., 2017; Crespo-Medina et al., 2017). A number of alkaliphilic Methanobacterium sp. have been isolated to date (Boone et al., 1986; Mathrani et al., 1988; Kotelnikova et al., 1998), the observations made here suggest that this genus is adaptable to aggressive pH changes as observed with Control sediment enrichments, but is also capable of surviving high pH after long-term exposure as residents of the initial New Lime sediments. This is in contrast to the Methanomicrobiales such as Methanocalculus sp. which are more prevalent in the hypersaline-hyperalkaline Soda lakes (Surakasi et al., 2007; Antony et al., 2013; Sorokin et al., 2015), due to the low salt tolerance of Methanobacterium sp. (Boone et al., 1986). In this study, Methanosarcina sp. were most impacted in the control sediments exposed to high pH in acetate enrichments. Pure culture studies of Lonar lake Methanosarcina indicated a maximum pH of 9.5 for growth upon acetate (Thakker and Ranade, 2002), which suggests that this genus may require a saline rather than a calcium dominated environment to sustain methane generation above pH 9.0.
These observations indicate that the generation of methane through the acetoclastic pathway, although energetically favorable with respect to bicarbonate (Jin and Kirk, 2018) does not proceed under alkaline conditions (>pH 9.0). High pH favors the dissociation of acetic acid to its anion (CH3COO−), preventing transmembrane diffusion (Kröninger et al., 2014). Under alkaline conditions, acetate transport into the acetoclastic methanogen cell is therefore reliant on an acetate transporter, which is likely to be less energetically favorable than hydrogenotrophic methanogenesis (Kröninger et al., 2014). Much of the current understanding of methanogen ecology under alkaline conditions has been focused upon the microbiology of hypersaline, hyperalkaline Soda lakes, where methanogenesis is most commonly associated with the metabolism of C-1 compounds being released upon the biodegradation of Cyanobacterial mats (Jones et al., 1998; Sorokin et al., 2017). The current study indicates that methanogenesis in non-saline, calcium dominated alkaline environments should be considered as a different ecological niche. Within these environments, hydrogenotrophic methanogenesis is the most prevalent methanogenic pathway above pH 9.0. This observation is supported by the investigations of sediments from 13 individual sites ranging in age, origin and chemical composition. These observations suggest that under the alkaline conditions generated within a cement-based GDF, it is the hydrogenotrophic methane generation pathway that will be active rather than acetoclastic methanogenesis.
The data is deposited with the NCBI under Bioproject accession number PRJNA525260.
PH, SR, WM, and HG contributed to conception and design of the study. RW carried out the experimental work. PH, WM, HG, RW, and SR participated in the field work. SR, PH, and RW wrote the first draft. All authors contributed to manuscript revision, read, and approved the submitted version.
Richard Wormald’s PhD was supported by a Radioactive Waste Management Ltd. (RWM) research bursary. RWM Ltd. is a wholly own subsidiary of the UK Nuclear Decommissioning Authority, a non-departmental public body which reports to the UK Department for Business, Energy, and Industrial Strategy. WM and HG were supported by the NERC R3AW grant (NE/L014211/1).
The authors declare that the research was conducted in the absence of any commercial or financial relationships that could be construed as a potential conflict of interest. RWM Ltd. had no influence on the design or execution of this study or the interpretation and reporting of the data reported in this paper.
We would like to thank the following individuals and organizations. David Johnson for his assistance regarding the field kiln sites investigated. The Canal and River Boat Trust, Hanson Aggregates, Tarmac plc, Tee Valley Wildlife Trust and British Steel.
The Supplementary Material for this article can be found online at: https://www.frontiersin.org/articless/10.3389/fmicb.2020.614227/full#supplementary-material
Agneessens, L. M., Ottosen, L. D. M., Andersen, M., Olesen, C. B., Feilberg, A., and Kofoed, M. V. W. (2018). Parameters affecting acetate concentrations during in-situ biological hydrogen methanation. Bioresour. Technol. 258, 33–40. doi: 10.1016/j.biortech.2018.02.102
Almond, M., Shaw, P. B., Humphreys, P. N., Chadha, M. J., Niemelä, K., and Laws, A. P. (2012). Behaviour of xyloisosaccharinic acid and xyloisosaccharino-1, 4-lactone in aqueous solutions at varying pHs. Carbohydr. Res. 363, 51–57. doi: 10.1016/j.carres.2012.09.019
Altschul, S. F., Madden, T. L., Schäffer, A. A., Zhang, J., Zhang, Z., Miller, W., et al. (1997). Gapped BLAST and PSI-BLAST: a new generation of protein database search programs. Nucleic Acids Res. 25, 3389–3402. doi: 10.1093/nar/25.17.3389
Antony, C. P., Kumaresan, D., Hunger, S., Drake, H. L., Murrell, J. C., and Shouche, Y. S. (2013). Microbiology of Lonar Lake and other soda lakes. ISME 7, 468–476. doi: 10.1038/ismej.2012.137
Avis, J., Calder, N., Humphreys, P., King, F., Suckling, P., and Walsh, R. (2011). Postclosure Safety Assessment: Gas Modelling. Ontario, Canada: Nuclear Waste Management Organization.
B.S.I (2005). BS ISO 14853:2005 Plastics-Determination of the ultimate anaerobic biodegradation of plastic materials in an aqueous system‐ Method by measurement of biogas production. British Standards Institute, London, UK.
Bagnoud, A., Chourey, K., Hettich, R. L., de Bruijn, I., Andersson, A. F., Leupin, O. X., et al. (2016). Reconstructing a hydrogen-driven microbial metabolic network in Opalinus Clay rock. Nat. Commun. 7:12770. doi: 10.1038/ncomms12770
Balk, M., Weijma, J., and Stams, A. J. (2002). Thermotoga lettingae sp. nov., a novel thermophilic, methanol-degrading bacterium isolated from a thermophilic anaerobic reactor. Int. J. Syst. Evol. Microbiol. 52, 1361–1368. doi: 10.1099/00207713-52-4-1361
Bassil, N. M., Bryan, N., and Lloyd, J. R. (2014). Microbial degradation of isosaccharinic acid at high pH. ISME 9, 310–320. doi: 10.1038/ismej.2014.125
Boone, D. R., Worakit, S., Mathrani, I. M., and Mah, R. A. (1986). Alkaliphilic methanogens from high-pH lake sediments. Syst. Appl. Microbiol. 7, 230–234. doi: 10.1016/S0723-2020(86)80011-X
Brazelton, W. J., Thornton, C. N., Hyer, A., Twing, K. I., Longino, A. A., Lang, S. Q., et al. (2017). Metagenomic identification of active methanogens and methanotrophs in serpentinite springs of the Voltri Massif Italy. PeerJ 5:e2945. doi: 10.7717/peerj.2945
Burke, I., Mortimer, R., Palani, S., Whittleston, R., Lockwood, C., Ashley, D., et al. (2012). Biogeochemical reduction processes in a hyper-alkaline affected leachate soil profile. Geomicrobiol J. 29, 769–779. doi: 10.1080/01490451.2011.619638
Callahan, B. J., McMurdie, P. J., Rosen, M. J., Han, A. W., Johnson, A. J. A., and Holmes, S. P. (2016). DADA2: High resolution sample inference from Illumina amplicon data. Nat. Methods 13, 581–583. doi: 10.1038/nmeth.3869
Charles, C. J., Rout, S. P., Garratt, E. J., Patel, K., Laws, A. P., and Humphreys, P. N. (2015). The enrichment of an alkaliphilic biofilm consortia capable of the anaerobic degradation of isosaccharinic acid from cellulosic materials incubated within an anthropogenic, hyperalkaline environment. FEMS Microbiol. Ecol. 91:fiv085. doi: 10.1093/femsec/fiv085
Charles, C., Rout, S., Patel, K., Akbar, S., Laws, A., Jackson, B., et al. (2017). Floc formation reduces the pH stress experienced by microorganisms living in alkaline environments. Appl. Environ. Microbiol. 83, e02985–e02916. doi: 10.1128/AEM.02985-16
Charles, C. J., Rout, S. P., Wormald, R., Laws, A. P., Jackson, B. R., Boxall, S. A., et al. (2019). In-Situ Biofilm Formation in Hyper Alkaline Environments. Geomicrobiol J. 36, 404–411. doi: 10.1080/01490451.2018.1564803
Crespo-Medina, M., Twing, K. I., Sánchez-Murillo, R., Brazelton, W. J., McCollom, T. M., and Schrenk, M. O. (2017). Methane dynamics in a tropical serpentinizing environment: the Santa Elena Ophiolite, Costa Rica. Front. Microbiol. 8:916. doi: 10.3389/fmicb.2017.00916
Daebeler, A., Gansen, M., and Frenzel, P. (2013). Methyl fluoride affects methanogenesis rather than community composition of methanogenic archaea in a rice field soil. PLoS One 8:e53656. doi: 10.1371/journal.pone.0053656
Dridi, B., Fardeau, M. -L., Ollivier, B., Raoult, D., and Drancourt, M. J. (2012). Methanomassiliicoccus luminyensis gen. nov., sp. nov., a methanogenic archaeon isolated from human faeces. Int. J. Syst. Evol. Microbiol. 62, 1902–1907. doi: 10.1099/ijs.0.033712-0
Faybishenko, B., Birkholzer, J., Sassani, D., and Swift, P. (2016). International Approaches for Nuclear Waste Disposal in Geological Formations: Geological Challenges in Radioactive Waste Isolation—Fifth Worldwide Review. Lawrence Berkeley National Lab, Berkeley, CA United States.
Felicione, F. S., Carney, K. P., Dwight, C. C., Cummings, D. G., and Foulkrod, L. E. (2003). “Gas-generation experiments for long-term storage of TRU wastes at WIPP” in Proceedings of WM03; February 23–27, 2003; Tucson, Arizona, United States.
Hattori, S. (2008). Syntrophic acetate-oxidizing microbes in methanogenic environments. Microbes Environ. 23, 118–127. doi: 10.1264/jsme2.23.118
Hattori, S., Kamagata, Y., Hanada, S., and Shoun, H. (2000). Thermacetogenium phaeum gen. nov., sp. nov., a strictly anaerobic, thermophilic, syntrophic acetate-oxidizing bacterium. Int. J. Syst. Evol. Microbiol. 50, 1601–1609. doi: 10.1099/00207713-50-4-1601
Humphreys, P. N., Laws, A., and Dawson, J. (2010). A Review of Cellulose Degradation and the Fate of Degradation Products Under Repository Conditions. Serco Contractors Report for the Nuclear Decommissioning authority, Harwell, UK.
IAEA (2011). Geological Disopsal of Radioactive Waste. Vienna, Austria: International Atomic Energy Agency.
Jin, Q., and Kirk, M. F. (2018). pH as a primary control in environmental microbiology: 1 thermodynamic perspective. Front. Environ. Sci. 6:21. doi: 10.3389/fenvs.2018.00021
Johnson, D. (2008). The archaeology and technology of early-modern lime burning in the yorkshire dales: developing a clamp kiln model. Ind. Archaeol. Rev. 30, 127–143. doi: 10.1179/174581908X347346
Jones, B. E., Grant, W. D., Duckworth, A. W., and Owenson, G. G. (1998). Microbial diversity of soda lakes. Extremophiles 2, 191–200. doi: 10.1007/s007920050060
Kotelnikova, S., Macario, A. J., and Pedersen, K. (1998). Methanobacterium subterraneum sp. nov., a new alkaliphilic, eurythermic and halotolerant methanogen isolated from deep granitic groundwater. Int. J. Syst. Bacteriol. 48, 357–367. doi: 10.1099/00207713-48-2-357
Kröninger, L., Deppenmeier, U., and Welte, C. (2014). Experimental evidence of an acetate transporter protein and characterization of acetate activation in aceticlastic methanogenesis of Methanosarcina mazei. FEMS Microbiol. Lett. 359, 147–153. doi: 10.1111/1574-6968.12550
Kyeremeh, I. A., Charles, C. J., Rout, S. P., Laws, A. P., and Humphreys, P. N. (2016). Microbial community evolution is significantly impacted by the use of calcium isosaccharinic acid as an analogue for the products of alkaline cellulose degradation. PLoS One 11:e0165832. doi: 10.1371/journal.pone.0165832
Libert, M., Bildstein, O., Esnault, L., Jullien, M., and Sellier, R. (2011). Molecular hydrogen: an abundant energy source for bacterial activity in nuclear waste repositories. Phys. Chem. Earth 36, 1616–1623. doi: 10.1016/j.pce.2011.10.010
Liu, Y., and Whitman, W. B. (2008). Metabolic, phylogenetic, and ecological diversity of the methanogenic archaea. Ann. N. Y. Acad. Sci. 1125, 171–189. doi: 10.1196/annals.1419.019
Manzoor, S., Schnürer, A., Bongcam-Rudloff, E., and Müller, B. (2018). Genome-guided analysis of Clostridium ultunense and comparative genomics reveal different strategies for acetate oxidation and energy conservation in syntrophic acetate-oxidising bacteria. Genes 9:225. doi: 10.3390/genes9040225
Mathrani, I. M., Boone, D. R., Mah, R. A., Fox, G. E., and Lau, P. P. (1988). Methanohalophilus zhilinae sp. nov., an alkaliphilic, halophilic, methylotrophic methanogen. Int. J. Syst. Bacteriol. 38, 139–142. doi: 10.1099/00207713-38-2-139
Mayes, W. M., Younger, P. L., and Aumônier, J. (2008). Hydrogeochemistry of alkaline steel slag leachates in the UK. Water Air Soil Pollut. 195, 35–50. doi: 10.1007/s11270-008-9725-9
Milodowski, A. E., Shaw, R. P., and Stewart, D. I. (2013). Harpur Hill Site: its geology, evolutionary history and a catalogue of materials present. British Geological Survey, Keyworth, Nottingham, UK.
NEA (2014). Updating the NEA International FEP List: Technical Note 1: Identification and Review of Recent Project specific FEP Lists. Paris, France: Nuclear Energy Agency.
Poller, A., Mayer, G., Darcis, M., and Smith, P. (2016). Modelling of Gas Generation in Deep Geological Repositories after Closure. Wettingen, Switzerland: NAGRA.
Rout, S. P., Charles, C. J., Doulgeris, C., McCarthy, A. J., Rooks, D. J., Loughnane, J. P., et al. (2015a). Anoxic biodegradation of isosaccharinic acids at alkaline pH by Natural Microbial Communities. PLoS One 10:e0137682. doi: 10.1371/journal.pone.0137682
Rout, S. P., Charles, C. J., Garratt, E. J., Laws, A. P., Gunn, J., and Humphreys, P. N. (2015b). Evidence of the generation of isosaccharinic acids and their subsequent degradation by local microbial consortia within hyper-alkaline contaminated soils, with relevance to intermediate level radioactive waste disposal. PLoS One 10:e0119164. doi: 10.1371/journal.pone.0119164
Rout, S. P., Radford, J., Laws, A. P., Sweeney, F., Elmekawy, A., Gillie, L. J., et al. (2014). Biodegradation of the alkaline cellulose degradation products generated during radioactive waste disposal. PLoS One 9:e107433. doi: 10.1371/journal.pone.0107433
Sorokin, D. Y., Abbas, B., Geleijnse, M., Pimenov, N. V., Sukhacheva, M. V., and van Loosdrecht, M. (2015). Methanogenesis at extremely haloalkaline conditions in the soda lakes of Kulunda Steppe (Altai, Russia). FEMS Microbiol. Ecol. 91:fiv016. doi: 10.1093/femsec/fiv016
Sorokin, D. Y., Makarova, K. S., Abbas, B., Ferrer, M., Golyshin, P. N., Galinski, E. A., et al. (2017). Discovery of extremely halophilic, methyl-reducing euryarchaea provides insights into the evolutionary origin of methanogenesis. Nat. Microbiol. 2:17081. doi: 10.1038/nmicrobiol.2017.81
Surakasi, V. P., Wani, A. A., Shouche, Y. S., and Ranade, D. R. (2007). Phylogenetic analysis of methanogenic enrichment cultures obtained from Lonar Lake in India: isolation of Methanocalculus sp. and Methanoculleus sp. Microb. Ecol. 54, 697–704. doi: 10.1007/s00248-007-9228-z
Takahashi, S., Tomita, J., Nishioka, K., Hisada, T., and Nishijima, M. (2014). Development of a prokaryotic universal primer for simultaneous analysis of bacteria and archaea using next-generation sequencing. PLoS One 9:e105592. doi: 10.1371/journal.pone.0105592
Thakker, C. D., and Ranade, D. R. (2002). An alkalophilic Methanosarcina isolated from Lonar crater. Curr. Sci. 82, 455–458.
Keywords: alkaliphiles, radioactive waste, hydrogenotrophic methanogens, acetoclastic methanogens, alkaline
Citation: Wormald RM, Rout SP, Mayes W, Gomes H and Humphreys PN (2020) Hydrogenotrophic Methanogenesis Under Alkaline Conditions. Front. Microbiol. 11:614227. doi: 10.3389/fmicb.2020.614227
Received: 05 October 2020; Accepted: 11 November 2020;
Published: 03 December 2020.
Edited by:
Ricardo Amils, Autonomous University of Madrid, SpainReviewed by:
Jose Luis, Autonomous University of Madrid, SpainCopyright © 2020 Wormald, Rout, Mayes, Gomes and Humphreys. This is an open-access article distributed under the terms of the Creative Commons Attribution License (CC BY). The use, distribution or reproduction in other forums is permitted, provided the original author(s) and the copyright owner(s) are credited and that the original publication in this journal is cited, in accordance with accepted academic practice. No use, distribution or reproduction is permitted which does not comply with these terms.
*Correspondence: Paul N. Humphreys, cC5uLmh1bXBocmV5c0BodWQuYWMudWs=
Disclaimer: All claims expressed in this article are solely those of the authors and do not necessarily represent those of their affiliated organizations, or those of the publisher, the editors and the reviewers. Any product that may be evaluated in this article or claim that may be made by its manufacturer is not guaranteed or endorsed by the publisher.
Research integrity at Frontiers
Learn more about the work of our research integrity team to safeguard the quality of each article we publish.