- Shanghai Veterinary Research Institute, Chinese Academy of Agricultural Sciences, Shanghai, China
Toxoplasma gondii is a ubiquitous apicomplexan protozoan parasite that can infect all warm-blooded animals, causing toxoplasmosis. Thus, efficient diagnosis methods for acute T. gondii infection are essential for its management. Circulating antigens (CAgs) are reliable diagnostic indicators of acute infection. In this study, we established a mouse model of acute T. gondii infection and explored new potential diagnostic factors. CAgs levels peaked 60 h after T. gondii inoculation and 31 CAgs were identified by immunoprecipitation-liquid chromatography-tandem mass spectrometry, among which RuvB-like helicase (TgRuvBL1), ribonuclease (TgRNaseH1), and ribosomal protein RPS2 (TgRPS2) were selected for prokaryotic expression. Polyclonal antibodies against these three proteins were prepared. Results from indirect enzyme-linked immunosorbent assay indicated that anti-rTgRuvBL1, anti-rTgRNase H1, and anti-rTgRPS2 mouse sera were recognized by natural excretory-secretory antigens from T. gondii tachyzoites. Moreover, immunofluorescence assays revealed that TgRuvBL1 was localized in the nucleus, while TgRNase H1 and TgRPS2 were in the apical end. Western blotting data confirmed the presence of the three proteins in the sera of the infected mice. Moreover, mice immunized with rTgRuvBL1 (10.0 ± 0.30 days), TgRNaseH1 (9.67 ± 0.14 days), or rTgRPS2 (11.5 ± 0.34 days) had slightly longer lifespan when challenged with a virulent T. gondii RH strain. Altogether, these findings indicate that these three proteins can potentially be diagnostic candidates for acute toxoplasmosis. However, they hold poor protective potential against highly virulent T. gondii infection.
Introduction
Toxoplasma gondii is an obligatory intracellular protozoan parasite that infects virtually any warm-blooded animal, including a third of the human population (Montoya and Liesenfeld, 2004). Infection is mainly acquired by ingestion of undercooked meats containing T. gondii cysts (Hide et al., 2009). After ingestion, the parasite causes lifelong infections by converting from rapidly dividing tachyzoites into encysted slow-growing bradyzoites, mainly localizing to the brain, and muscle tissues (Hunter and Sibley, 2012; Wohlfert et al., 2017). Moreover, a latent infection may be reactivated via immune suppression (Dupont et al., 2020). In general, toxoplasmosis is usually asymptomatic in immunocompetent persons, but it can cause life-threatening infections in immunocompromised individuals and developing fetuses (Nissapatorn, 2009; Csep and Drãghici, 2013; Lima and Lodoen, 2019). Thus, timely and effective diagnosis of acute T. gondii infection is imperative.
Convenient serological tests have been widely applied to diagnose toxoplasmosis, which are mainly based on the detection of specific antibodies (IgM and IgG; Robert-Gangneux and Dardé, 2012). IgG can only be detected 13 days after infection, whereas IgM can persist in some patients with toxoplasmosis for over a year (Elsheikha et al., 2020). Thus, the presence of IgG and IgM antibodies does not necessarily indicate an acute infection. The lack of effective serological diagnostic methods for acute toxoplasmosis can lead to delayed treatment and even therapy failure, since available strategies can control acute infections, but not treat chronic toxoplasmosis.
Toxoplasma gondii excretory-secretory antigens (ESA) represent the majority of circulating antigens (CAgs) in the sera of hosts with acute toxoplasmosis (Daryani et al., 2003; Abdollahi et al., 2009). Previous studies have shown that T. gondii CAgs can be used as accurate markers for acute toxoplasmosis diagnosis (Hafid et al., 1995; Meira et al., 2008; Abdollahi et al., 2009; Darcy et al., 2010; Xue et al., 2016). Therefore, analysis of CAg profiles and screening of candidate diagnostic molecules should improve the specificity and sensitivity of detection methods for acute infection. Moreover, CAgs modulate the host immune response (Hafid et al., 2005; Abdollahi et al., 2013; Daryani et al., 2014; Carlos et al., 2019; Xue et al., 2019) and the identification of CAg components may be conducive to the discovery of potential candidate antigens for vaccines against toxoplasmosis. To date, CAgs have not been investigated in detail, and only a few CAgs components have been identified. The present study aimed to determine the spectrum of T. gondii CAgs in sera of acute infected mice using immunoprecipitation-liquid chromatography (LC)-tandem mass spectrometry (MS/MS). We also aimed to verify three novel CAgs in the sera of infected model mice and to evaluate their protective ability.
Materials and Methods
Parasite Strains and Culture
The T. gondii strain RH and African green monkey kidney (VERO) cells were stored at our facility. T. gondii tachyzoites were grown in VERO cells maintained in Dulbecco’s Modified Eagle’s Medium (DMEM; Gibco Laboratories, Gaithersburg, MD, United States) supplemented with 2% fetal bovine serum, 100 kU/L streptomycin, and 400 kU/L penicillin at 37°C under a 5% CO2 atmosphere.
Animals
Eight-week old Kunming mice and New Zealand white female rabbits weighing ∼25 g and ∼2 kg, respectively (Shanghai Laboratory Animal Centre, Chinese Academy of Sciences, Shanghai, China) were raised in a sterilized room and fed with sterilized food and water at the Animal Laboratory Centre at the Shanghai Veterinary Research Institute. The Animal Care and Use Committee of the Shanghai Veterinary Research Institute approved the study and the protocols complied with approved guidelines.
ESA and Antisera Preparation
Excretory-secretory antigens were obtained as previously described (Zhou et al., 2005). Briefly, purified tachyzoites (5 × 108/mL) suspended in phosphate-buffered saline (PBS) were incubated at 37°C for 2 h. After centrifugation for 15 min at 1,000 × g at 4°C, the supernatant was collected, and the protein density was determined using DC protein assay kits (Bio-Rad Laboratories Inc., Hercules, CA, United States). Thereafter, 1 mg of protein per tube (1.5 mL) was lyophilized. At 0, 2, 4, and 6 weeks, the mice and rabbits were immunized with 100 μg per mouse and 500 μg/kg proteins, respectively, or PBS (the control). The 206 adjuvant was applied as described by the manufacturer (Seppic, Paris, France). Blood was sampled from the tails of mice at the end of each interval for immunoblotting and enzyme-linked immunosorbent assay (ELISA).
Establishment of Double-PcAb Sandwich ELISA
A double-PcAb sandwich ELISA was established to detect CAgs using anti-ESA rabbit and mouse sera as coating and detection antibodies, respectively. Nonspecific protein binding was blocked with PBS containing 0.05% Tween-20 (PBST) and 5% non-fat powdered milk. Briefly, Costar microtiter plates (Corning Inc., Corning, NY, United States) were coated with anti-ESA rabbit serum in 50 mM carbonate buffer overnight at 4°C, then washed with PBST. Nonspecific binding was blocked for 2 h at 37°C. The plates were washed, then 500 ng/mL ESA or samples were added and the plates were incubated for 1 h at 37°C, and washed. Sample dilution buffer was the negative control. Anti-ESA mouse sera were added to the plates and incubated for 1 h at 37°C, followed by three washes with PBST. Secondary HRP-conjugated goat anti-mouse IgG antibody (Cat. No. SE131; Solarbio, Beijing, China) was added, and the plates were incubated for 1 h at 37°C. After five washes with PBST, immune complexes were visualized by incubation with tetramethylbenzidine (TMB) for 20 min. The reaction was stopped by adding 2 M H2SO4, and absorbance was measured at 450 nm using an automated MULTISKAN GO microplate reader (Thermo Fisher Scientific Inc., Waltham, MA, United States). All samples were analyzed in triplicate. The optimal coating and detection concentrations were determined using checkerboard titration. The sensitivity of the method was assessed using positive canine sera at 3 days post-challenge with T. gondii (Xue et al., 2016).
Development of Acute Infection Models
Control and experimental (n = 3 each) mice were injected with 500 μL of PBS without and with 1 × 107 purified tachyzoites, respectively. Blood (100 μL) samples were collected at 0, 3, 9, 12, 24, 36, 48, and 60 h. Portions of these samples (20 μL) were mixed with sodium citrate anticoagulant for genomic DNA extraction and T. gondii detection, and serum CAgs was detected and purified by immunoprecipitation in 80 μL of samples.
Evaluation of Infection Models Using ELISA and Nested PCR
We analyzed CAgs in mouse sera using the double-PcAb sandwich ELISA. To detect T. gondii, DNA was obtained from anticoagulated blood using a genomic DNA extraction kit (Cat. No. DP308; Tiangen, Beijing, China). Nested PCR was conducted as we previously described (Wang et al., 2012).
Immunoprecipitation
Proteins of interest were immunoprecipitated as described (Xue et al., 2016), mixed with sera at 60 h after challenge (20 μL per mouse), and diluted (1:10) in PBS. Protein G agarose beads (200 μL, 25%; Cat. No. P2009; Beyotime, Nantong, China) were added, and stirred at 4°C for 3 h to remove serum antibodies. The supernatant was collected after centrifugation (260 × g, 4°C, 5 min), then 10 μL of anti-ESA mouse serum was added, and stirred slowly overnight at 4°C. Protein G agarose beads (200 μL) were added, and the mixture was stirred at 4°C for 3 h. The beads were collected by centrifugation (260 × g, 4°C, 5 min), washed three times with PBS, and boiled in 5× sample buffer (60 μL) for 5 min. The supernatant collected after centrifugation (260 × g, 4°C, 5 min) was resolved by 10% sodium dodecyl sulfate-polyacrylamide gel electrophoresis (SDS-PAGE), then analyzed by LC-tandem mass spectrometry (LC-MS/MS; Shanghai Applied Protein Technology Co., Ltd., Shanghai, China).
LC-Electrospray Lonization MS/MS Analysis
Samples were analyzed using a Q Exactive mass spectrometer coupled with an Easy-nLC (Thermo Fisher Scientific Inc.) liquid chromatograph. Briefly, samples were reduced, alkylated, and trypsinized (mass ratio 1:50) at 37°C for 20 h. The enzymolysis product was desalted, lyophilized, dissolved in 0.1% formic acid, and stored at -20°C. For MS analysis, solution A comprised 0.1% formic acid in high performance liquid chromatography (HPLC)-grade water, and solution B comprised 0.1% formic acid in 84% acetonitrile. After the chromatographic column was equilibrated with 95% solution A, samples were loaded from the autosampler to the trap column (0.5 H gradient). To collect mass spectra, the mass-to-charge ratios of peptides and peptide fragments were determined by collecting 20 fragment maps (MS2 scan) after each full scan. The original raw files from the MS test were used to search the UniProt database for proteins using Mascot 2.2 software. The search parameters were as follows: enzyme, trypsin; fixed modification, carbamidomethyl (C); variable modification, oxidation (M); missed cleavage, 2; peptide mass tolerance, 20 ppm; MS/MS tolerance, 0.1 Da; and filter by score ≥ 20.
Expression of TgRuvBL1, TgRNase H1, and TgRPS2 in vitro and Antibody Production
To further verify the CAgs components in serum, RuvB-like helicase (TgRuvBL1), ribonuclease (TgRNase H1), and ribosomal protein RPS2 (TgRPS2) were expressed in an Escherichia coli (BL 21) system with the pET-28a-c(+) vector. Table 1 shows the expressed regions, and primers, etc. The sequence of the recombinant plasmid was confirmed by dideoxy chain termination sequencing (Invitrogen, Carlsbad, CA, United States). The recombinant plasmid was transformed into E. coli (BL21) competent cells, and cultured in LB medium containing kanamycin (70 μg/mL) at 37°C. Expression was induced with 1 mM isopropyl β-D-thiogalactopyranoside (IPTG) for 6 h at 37°C. Cells were sonicated, then recombinant proteins isolated from inclusion bodies were refolded using TB234 kits (Cat. No: 70123-3; Novagen, San Diego, CA, United States), and purified using His-tag protein purification kits (Cat. No. P2226; Beyotime, Nantong, China). Recombinant proteins were concentrated using 3-kDa centrifugal filters (Merck KGaA, Darmstadt, Germany), and quantified using DC protein assay kits (Bio-Rad Laboratories Inc.). At 0, 2, and 4 weeks, the mice were injected with each protein (100 μg per mouse) or PBS (control). Blood samples were collected from the tail vein of mice at the end of each interval for indirect ELISA and immunofluorescence assay analysis. For indirect ELISA, plates were coated with recombinant proteins (500 ng/mL) or ESA (1 μg/mL). Horseradish peroxidase-conjugated goat anti-mouse IgG were diluted 1:5,000.
Immunofluorescence Assays
For Immunofluorescence assays (IFA), infected confluent VERO monolayers were fixed with 4% paraformaldehyde in PBS, permeabilized with 0.5% Triton X-100, and blocked with 1% bovine serum albumin in PBS (BSA-PBS). The primary antibodies used were mouse anti-TgRuvBL1 (1:500), mouse anti-TgRNase H1 (1:500), mouse anti-TgRPS2 (1:500), and rabbit anti-SAG1 (1:4,000). The secondary antibodies used were Alexa Fluor 488-conjugated goat anti-mouse IgG (1:2,000; Thermo Fisher Scientific Inc.) and Alexa Fluor 594-conjugated goat anti-rabbit IgG (1:2,000; Jackson ImmunoResearch, West Grove, PA, United States). Images were acquired with a LSM880 confocal laser scanning microscope (Zeiss, Germany) after the samples were stained with DAPI.
Verification of TgRuvBL1, TgRNase H1, and TgRPS2 in Sera of Acute Infection Model
To verify the presence of TgRuvBL1, TgRNase H1, and TgRPS2 in the sera of infected model. Protein G agarose beads were used to eliminate serum antibodies, then the pooled sera (60 h) from the test and control (negative) group were analyzed by immunoblotting. The positive control was ESA. Primary TgRuvBL1, TgRNase H1, and TgRPS2 antisera were diluted 1:1000, and HRP-conjugated goat anti-mouse IgG secondary antibody was diluted 1:5 000.
Mouse Vaccination and Challenge
To further explore the protective ability of TgRuvBL1, TgRNase H1, and TgRPS2, 48 mice were randomly divided into four groups and were subcutaneously injected at weeks 0, 2, and 4 with 100 μg of rTgRuvBL1, rTgRNase H1, rTgRPS2, or PBS (control group), all with 206 adjuvant. Blood was sampled from all mice on week 6. Anti-ESA IgG was determined by ELISA in mice from each group. Afterward, 12 mice from each group were intraperitoneally challenged with 1 × 103 tachyzoites of the T. gondii RH strain.
Statistical Analysis
All data were statistically analyzed using IBM SPSS 20.0 Data Editor. Differences in data among all groups were compared using one-way ANOVA. Survival of the mice was compared using Kaplan–Meier curves. Differences between groups were considered significant at P < 0.05.
Results
Establishment of Double-PcAb Sandwich ELISA
The results of indirect ELISA and immunoblotting assays indicated that anti-ESA mouse and rabbit sera could identify numerous ESA components with good immunogenicity (Figures 1A,B). According to the checkerboard titration results (Figure 2A), the P/N value was highest (6.42) at dilutions of 1:1 000 for both anti-ESA rabbit serum coating and mouse serum detection antibodies. The dilution gradient of positive canine sera was detected using the double antibody sandwich ELISA with a P/N cutoff of 2.1. Figure 2B shows that the maximum dilution of positive serum was 1:160. These results showed that the double-PcAb sandwich ELISA was sufficiently sensitive.
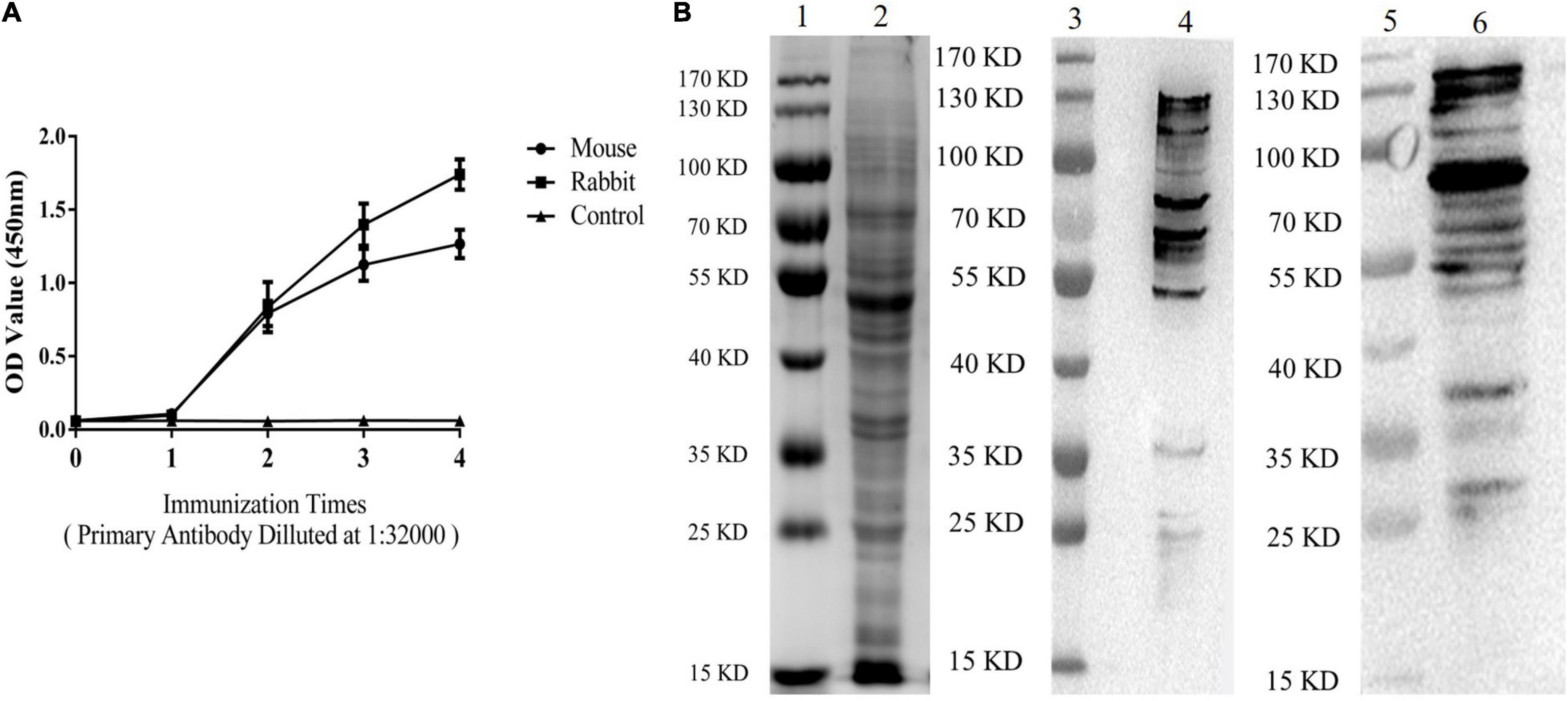
Figure 1. ESA antibodies were tested by ELISA and immunoblotting analyses. (A) Levels of ESA antibodies after each immunization (Mouse and Rabbit). Blood sera were sampled from the tails of mice and the ear vein of rabbit 10 days after immunization. (B) Western blotting analysis of ESA. Lane 1: marker on gel; Lane 2: ESA on gel; Lane 3: marker on NC membrane; and Lane 4: ESA on NC membrane (anti-ESA mice serum). Lane 5: marker on NC membrane; Lane 6: ESA on NC membrane (anti-ESA rabbit serum).
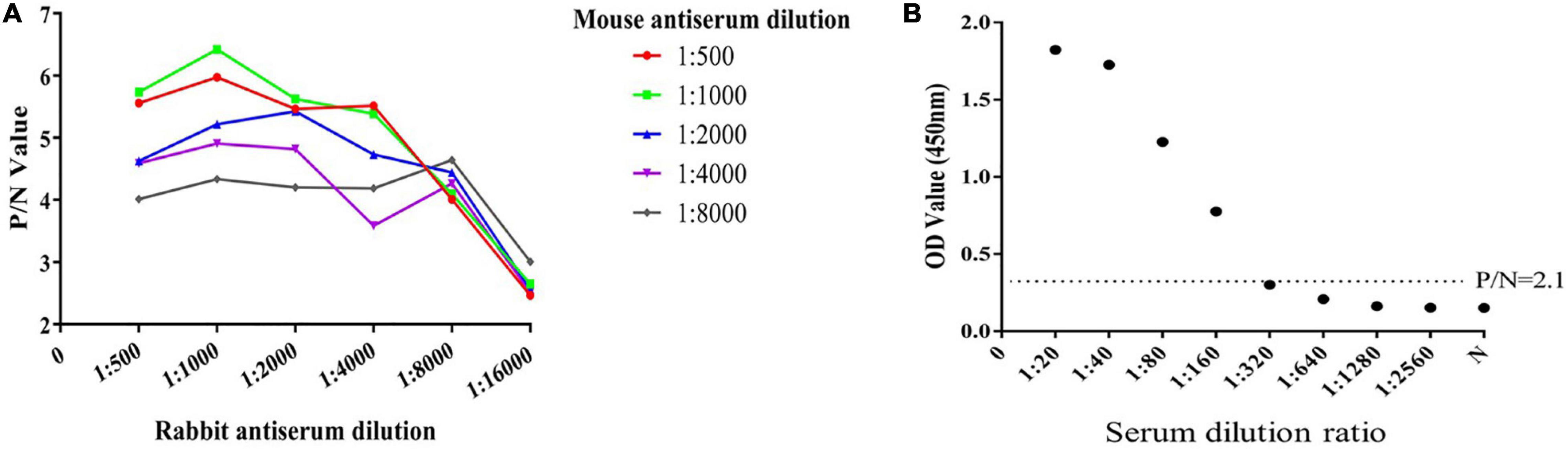
Figure 2. Determination of the optimum working concentration of mouse and rabbit antiserum and the maximum dilution of positive serum. (A) The optimal working coating concentration (anti-ESA rabbit serum) and detection concentration (anti-ESA mice serum) were determined using the checkerboard titration method. The P/N value was the criterion standard. (B) Sensitivity test using double-PcAb sandwich ELISA. The average optical density (OD) value for negative samples was multiplied by 2.1 to obtain the cut-off value. The horizontal lines represent cut-off values.
Establishment of Murine T. gondii Infection Model Using Inoculation With Tachyzoites
The T. gondii strain RH is lethal in mice. In this study, the mice survived for 65 ± 2 h after inoculation with 1 × 107 tachyzoites. Both T. gondii and CAgs were detected in the blood at 3 h (Figures 3A,B). The CAgs values initially first increased from 0 to 3 h, decreased from 3 to 24 h, increased from (24 to 60 h), then peaked at 60 h. The decrease in CAgs from 3 to 24 h might have been due to T. gondii in the bloodstream invading various organs. These data showed that the T. gondii infection model was effective.
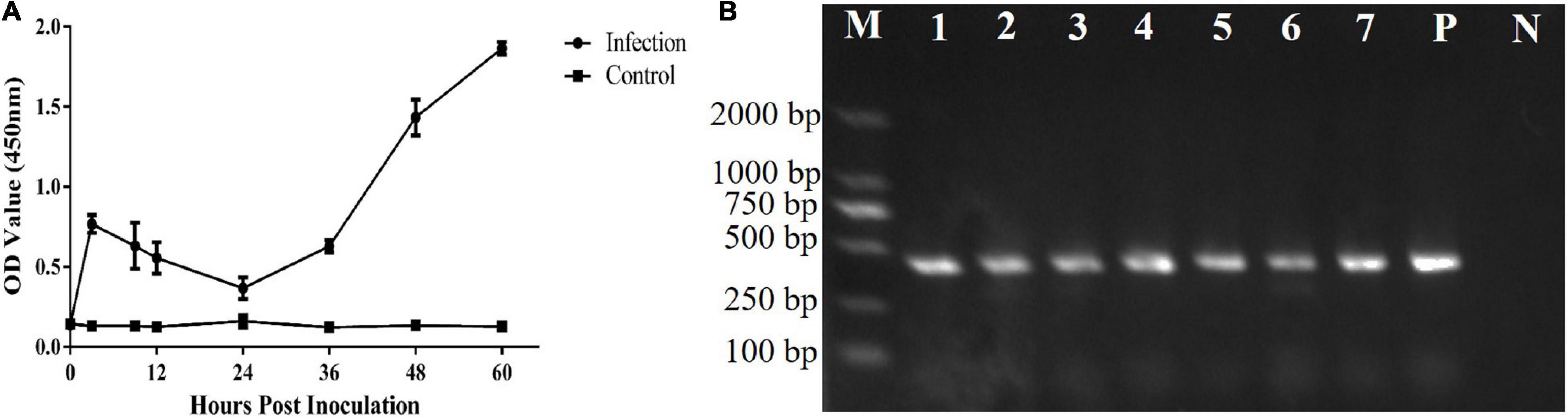
Figure 3. Analyses of CAgs and nested-PCR. (A) Sera of 0, 3, 9, 12, 24, 36, 48, and 60 h were tested for CAgs levels. (B) Nested-PCR results. Lane M: marker; Lane P: positive control; Lane N: negative control; and Lanes 1–7: Nested-PCR results of 3, 9, 12, 24, 36, 48, and 60 h, The nested-PCR results from one mouse in the test group are shown.
Identification of Circulating Antigens in the Sera of Acute T. gondii Infected Mice
Sodium dodecyl sulfate-polyacrylamide gel electrophoresis analysis showed that CAgs weighted more than 15 kD (Figure 4A). Among 250 protein groups identified by LC-MS/MS (Supplementary File 1), the unique peptide counts for 31 proteins were ≥2. Table 2 shows that the proteins identified by immunoprecipitation-shotgun analysis comprised micronemal proteins (PLP1 and M2AP), surface antigens (SAG1), rhoptry protein (ROP28), dense granule proteins (PI-1), and novel CAgs proteins (RuvB-like helicase, ribonuclease, and ribosomal protein RPS2) and others (Table 2).
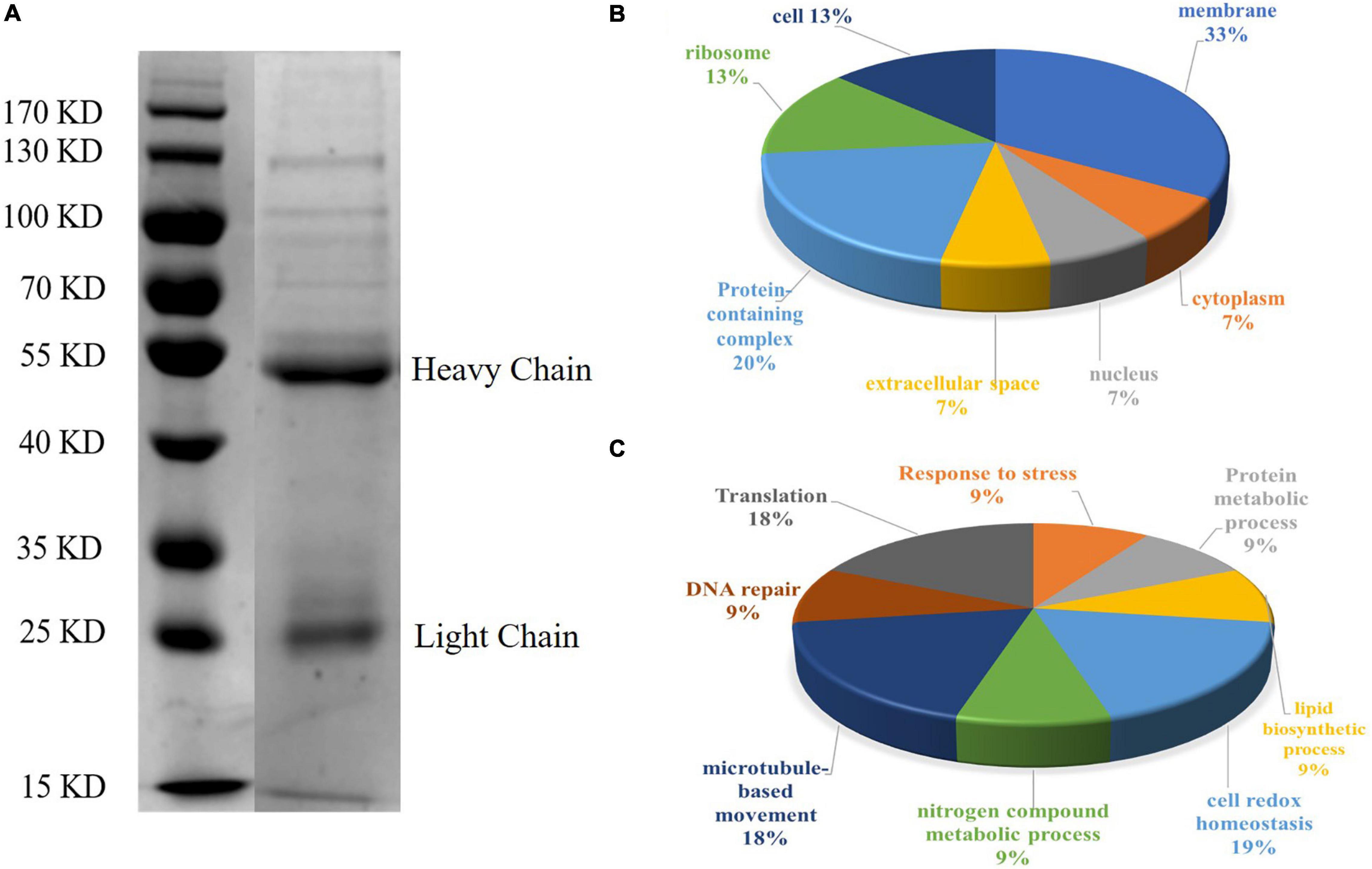
Figure 4. SDS-PAGE analysis and GO annotation of CAgs enriched and purified by immunoprecipitation. (A) Circulating antigens enriched and purified by immunoprecipitation were analyzed by SDS-PAGE (10%). Lane 1: marker; Lane 2: immunoprecipitation supernatant (In the original image, there is a crack in a lane near the marker, so it is cut off in the manuscript. Please refer to the original images in the Supplementary Figure 1). (B) Pie charts showing the GO distributions for the identified circulating antigens based on the cell component. (C) Pie charts showing the GO distributions for the identified circulating antigens based on the major biological process categories.
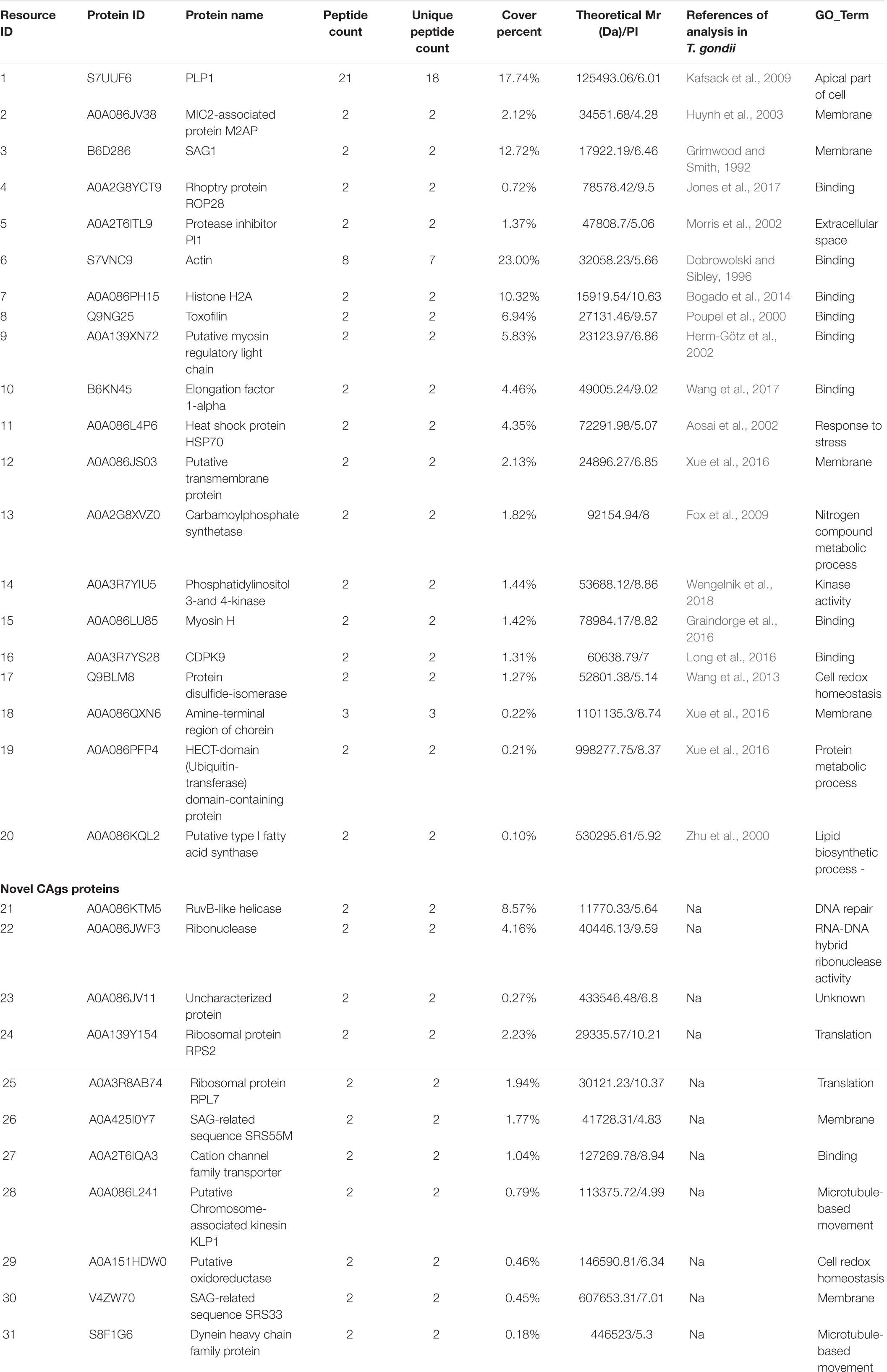
Table 2. CAgs proteins identified by LC-MS/MS after IP enrichment and purification with ESA mouse antibodies.
Gene Ontology Analysis
To further understand the functions of the CAgs herein identified, their gene ontology (GO) was evaluated. Figures 4B,C shows the GO annotations of the 31 high-confidence proteins, among which 15 were annotated in cell composition and were related to membrane components (33%), protein-containing complexes (20%), ribosomes (13%), cells (13%), extracellular regions (7%), the nucleus (7%), and the cytoplasm (7%). Only 11 proteins were annotated within biological process, being related to cell redox homeostasis (19%), translation (18%), microtubule-based movement (18%), response to stress (9%), protein metabolic processes (9%), lipid biosynthetic processes (9%), nitrogen compound metabolic processes (9%), and DNA repair (9%).
Expression, Purification, and Antibody Production of Recombinant TgRuvBL1, TgRNase H1, and TgRPS2
The genes encoding TgRuvBL1, TgRNase H1, and TgRPS2 were amplified from the T. gondii RH strain. The recombinant proteins were expressed in E. coli as inclusion body His-tagged fusion proteins when bacterial growth occurred at 37°C. The inclusion body recombinant proteins were refolded, purified, and concentrated. SDS-PAGE analysis indicated that the recombinant proteins (rTgRuvBL1, rTgRNase H1, and rTgRPS2) were successfully expressed and purified (Figure 5A). Figure 5B shows that the titer of the antibodies in mice serum reached more than 1:32,000 after three immunizations.
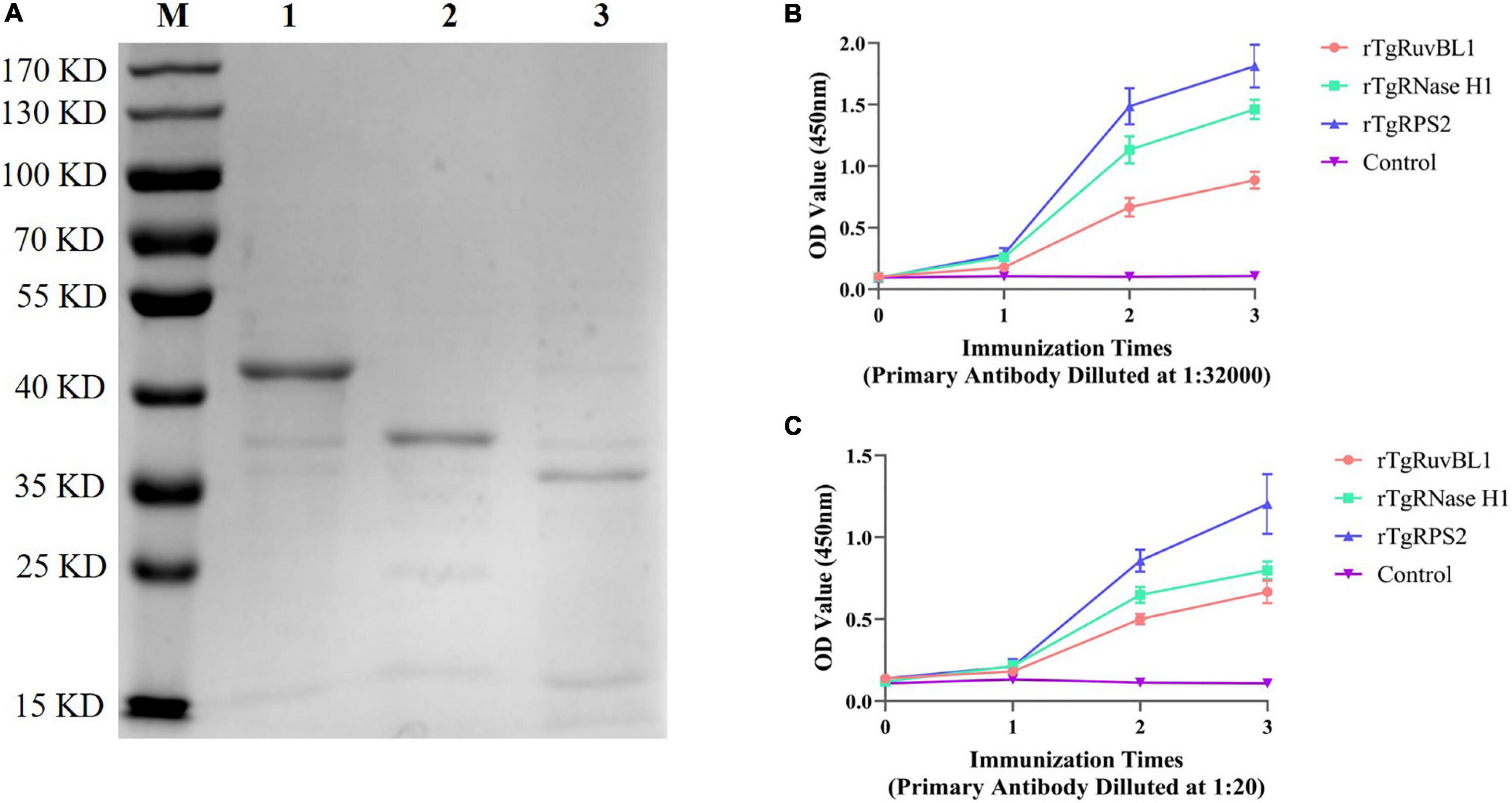
Figure 5. Expression of recombinant proteins and the dynamics of specific antibody amounts in Kunming mice induced by recombinant proteins. (A) SDS-PAGE analysis of purified recombinant proteins. M, Marker; 1, rTgRuvBL1; 2, rTgRNase H1; and 3, rTgRPS2. (B,C) Plates were coated with recombinant proteins (500 ng/mL) or ESA (1 μg/mL) to detect the dynamics of specific IgG antibody amounts.
Reactivity of Polyclonal Antibodies Toward T. gondii-Derived ESA and Tachyzoites
To characterize the reactivity of anti-recombinant proteins mouse sera with the ESA and T. gondii tachyzoites, antisera against rTgRuvBL1, rTgRNase H1, and rTgRPS2 were used as the primary antibody for indirect ELISA and IFA. The ELISA results showed that TgRuvBL1, TgRNase H1, and TgRPS2 antisera were recognized by T. gondii tachyzoites-derived natural ESA (Figure 5C), indicating that these proteins were present in T. gondii ESA. Moreover, IFA using anti-TgRuvBL1, anti-TgRNase H1, or anti-TgRPS2 antibodies revealed that the three proteins were localized in different subcellular compartments in T. gondii tachyzoites, including the nucleus (TgRuvBL1) and the apical end (TgRNase H1 and TgRPS2; Figure 6).
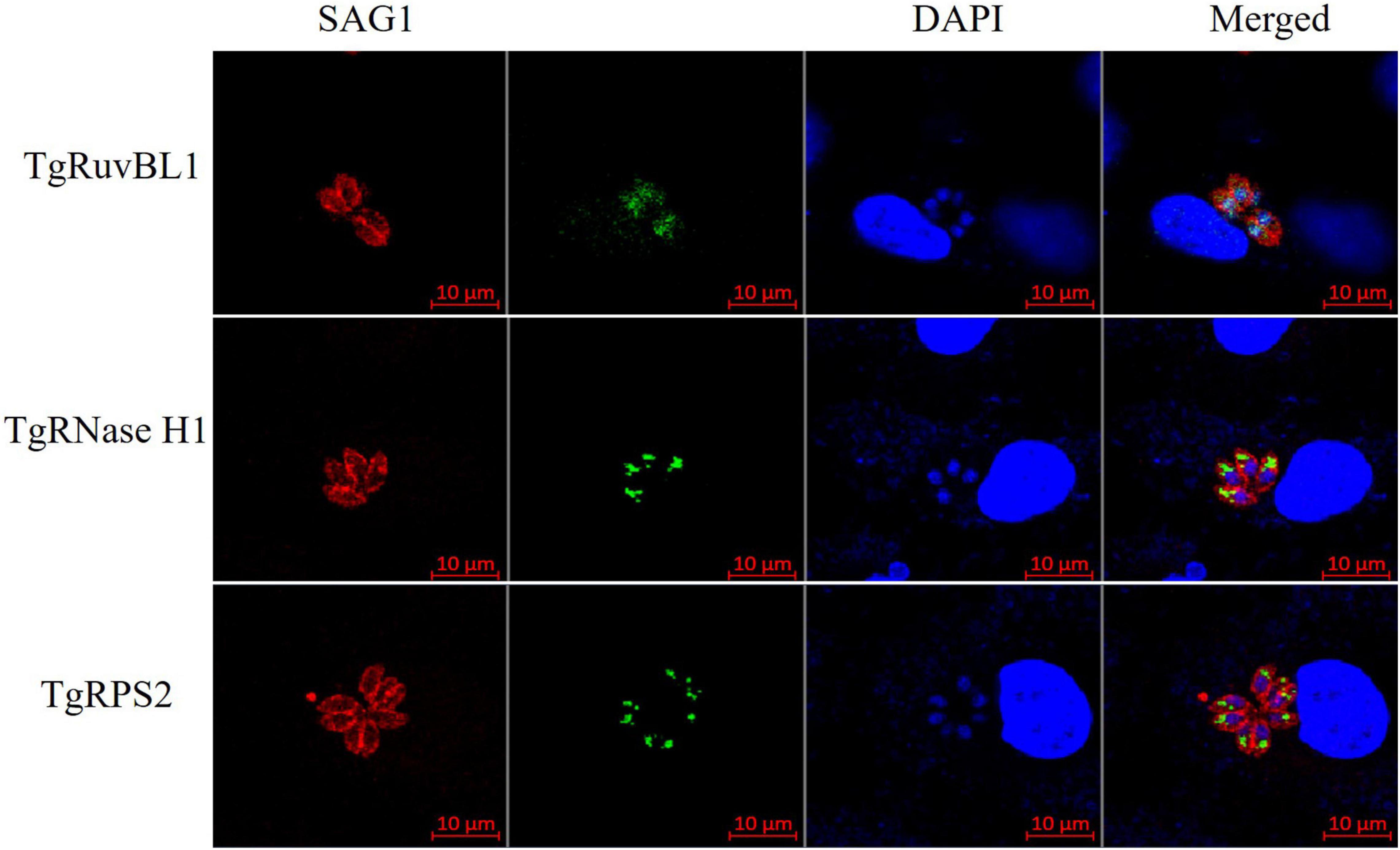
Figure 6. TgRuvBL1, TgRNase H1, and TgRPS2 localize to two subcellular regions. Immunofluorescence analysis showing the localization of the three CAgs, performed in T. gondii stained with anti-TgRuvBL1, anti-TgRNase H1, or anti-TgRPS2 antibodies. Surface antigen 1 (SAG1) was used as a marker of the surface.
Detection of Three CAgs in the Sera of Infection Murine Models
Among the newly identified CAgs, the presence of TgRuvBL1, TgRNase H1, and TgRPS2 was verified in mouse sera. Pooled sera from the test and control groups were analyzed by immunoblotting, with ESA being used as positive control. The three CAgs were detected in the sera of mice with acute T. gondii infections (Figure 7).
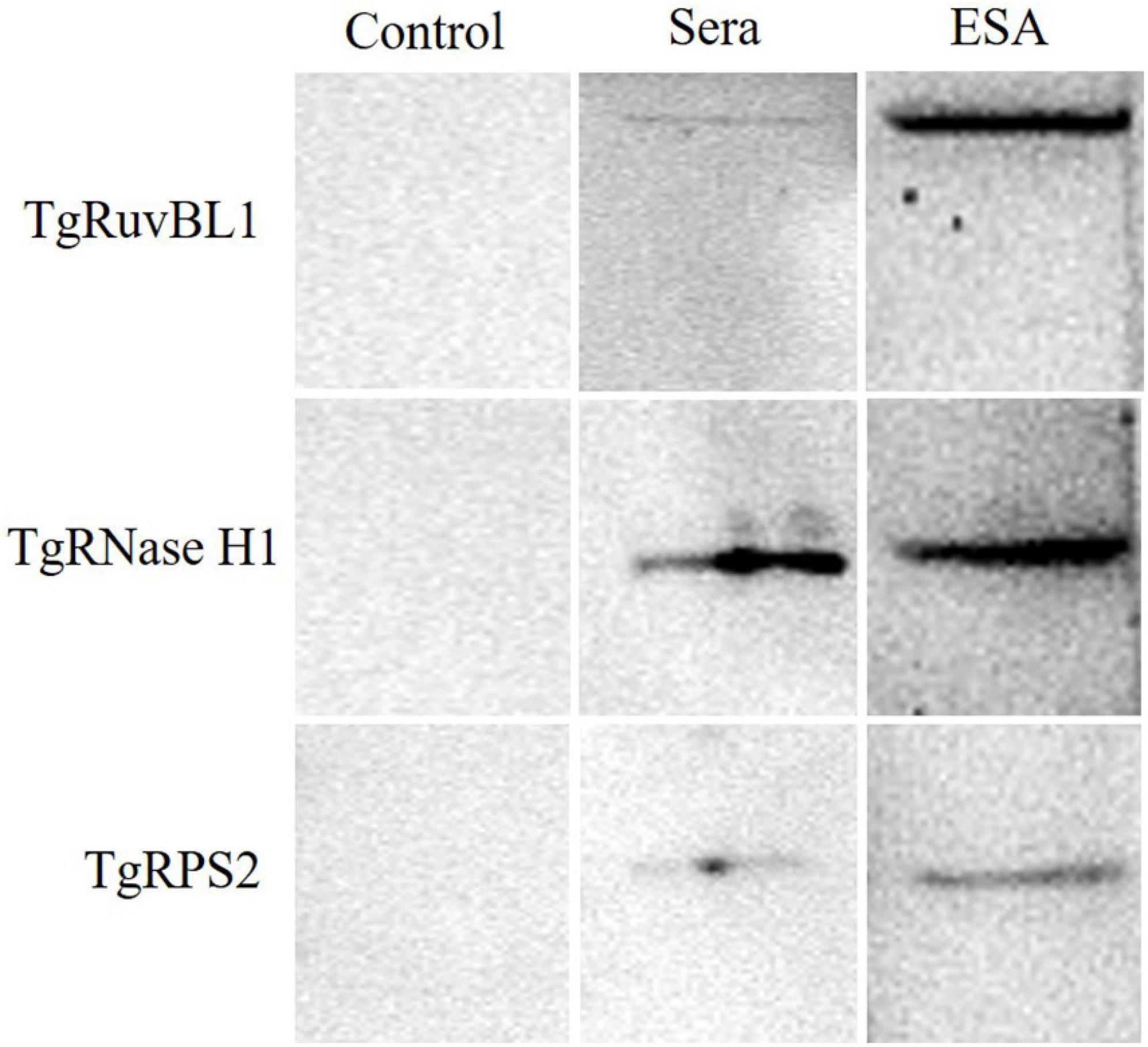
Figure 7. Western blotting of the recombinant proteins. Pooled serum samples from the control group were used as negative control. Pooled serum samples from the test group at 60 h were analyzed by western blotting at a dilution of 1:10. ESA was used as positive control.
Protective Ability of Recombinant Proteins in Kunming Mice
Significantly more IgG antibodies were detected in the sera of mice immunized with rTgRuvBL1, rTgRNase H1, or rTgRPS2 compared with control mice (Figure 8A). Figure 8B shows survival curves for the four groups of mice. Mice immunized with rTgRuvBL1, rTgRNase H1, or rTgRPS2 survived longer than control mice, all of which died within 8 days of challenge (10.0 ± 0.30, 9.67 ± 0.14, and 11.50 ± 0.34 vs. 7.25 ± 1.31 days; P < 0.05).
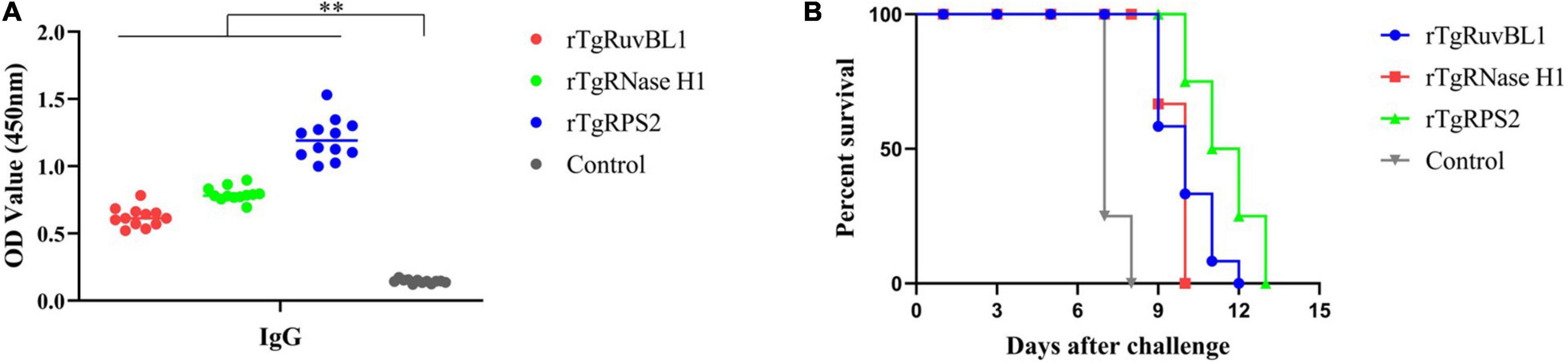
Figure 8. Survival curve of mice after challenge infection with T. gondii RH strain. (A) Levels of IgG in the sera of mice at week 2 after the final booster immunization. (B) Mice were challenged with 103 tachyozoites of the RH strain injected intraperitoneally at week 2 after the third immunization. *p < 0.05 and **p < 0.01, compared with the control groups.
Discussion
Detection of T. gondii-specific IgM and IgG antibodies is the main serological diagnostic method for acute toxoplasmosis. However, detection of IgG antibodies is not reliable for the diagnosis of acute infections, as they induce the production of low levels of IgG antibodies in acute infection and IgG positivity may be indicative of a previous infection. Moreover, IgM antibodies remain detectable for over a year. Therefore, even the presence of IgM antibodies does not necessarily indicate an acute infection (Elsheikha et al., 2020; Mcleod et al., 2020). ESA are the majority of the CAgs in sera from hosts with acute toxoplasmosis and they were proven to be a serologic marker for the diagnosis of acute toxoplasmosis and the infection route not affecting the detection of CAgs (Hafid et al., 1995; Abdollahi et al., 2009; Darcy et al., 2010; Xue et al., 2016). Particularly, they were shown to be diagnostic markers for cerebral toxoplasmosis in immunocompromised individuals (Meira et al., 2008; Meira et al., 2011). Thus, the identification of CAg components and screening of candidate diagnostic molecules can help improve the specificity and sensitivity of acute infection detection. However, only few studies have focused on the identification of CAgs.
In the current study, a murine model of acute T. gondii infection was established, which further demonstrated that CAgs could serve as markers of acute infection in mice in agreement with previous studies (Asai et al., 1987; Hassl et al., 1988; Chen et al., 2008; Azami et al., 2011; Wang et al., 2012; Xue et al., 2016). Moreover, CAgs were detected 3 h post-inoculation, which was consistent with the results of nested PCR. The levels of CAgs decreased during the following 3 to 24 h, and increased in the subsequent 24 to 60 h. The initial reduction might have been due to the host immune response and T. gondii in the bloodstream invading various organs.
Herein, 31 CAgs were identified in this study, among which, the diagnostic applications of SAG1, and ubiquitin-transferase domain-containing protein have been investigated (Felgner et al., 2015; Jiang et al., 2015). Moreover, some of the proteins identified, such as PLP1 (Kafsack et al., 2009), M2AP (Huynh et al., 2003), PI1 (Morris et al., 2002), Toxofilin (Poupel et al., 2000), Carbamoylphosphate synthetase (Fox et al., 2009), Myosin H (Graindorge et al., 2016), and CDPK9 (Long et al., 2016), have been shown to impair parasite progression through the lytic cycle, resulting in a loss of or weaker virulence when knocked-down. In addition, although most of the proteins have low reliability, some of them play important roles, such as ROP13 (Turetzky et al., 2010; Kang et al., 2019). Therefore, it is reasonable to believe that the CAgs identified in this study may contribute to the discovery of new virulence factors.
Furthermore, three CAgs – TgRuvBL1, TgRNaseH1, and TgRPS2 – were selected for prokaryotic expression to prepare murine polyclonal antibodies. Polyclonal anti-rTgRuvBL1, anti-rTgRNase H1, and anti-rTgRPS2 antibodies were recognized by natural ESA from T. gondii tachyzoites. Moreover, IFA data showed that they localize to two subcellular compartments, including the nucleus (TgRuvBL1) and the apical end (TgRNase H1 and TgRPS2). Of note, as both TgRNase H1 and TgRPS2 localize to the apical end, they may be involved in the invasion or egress of T. gondii tachyzoites. However, further research is warranted to explore this hypothesis. Moreover, these three proteins were found in the sera from mice with acute infection, which suggests that they may hold diagnostic potential for acute T. gondii infection and that their antibodies may be used in serological detection of CAgs. However, a large number of clinical trials are needed to verify whether they can be used in the diagnosis of acute infections. The present results also provide a theoretical basis for the continued development of rapid, highly specific, and accurate immunological diagnostic strategies.
Most CAgs are ESA that play important roles in inducing appropriate humoral and cellular immune responses against T. gondii (Hafid et al., 2005; Jongert et al., 2009; Costa-Silva et al., 2012; Chen et al., 2013; Daryani et al., 2014). Moreover, some proteins identified herein such as PLP1 (Yan et al., 2011), M2AP (Dautu et al., 2007), SAG1 (Mohamed et al., 2003), protease inhibitor PI1 (Cuppari et al., 2008), Histone H2A (Yu et al., 2020), Elongation factor 1-alpha (EF-1α; Wang et al., 2017), CDPK9 (Wang H. L. et al., 2016; Wang J. L. et al., 2016), HSP70 (Mohamed et al., 2003), protein disulfide-isomerase (Wang et al., 2013), and toxofilin (Song et al., 2017) elicit powerful specific immune responses, providing partial protection against acute or chronic T. gondii infection. Thus, we evaluated the protective ability of TgRuvBL1, TgRNase H1, and TgRPS2.
We showed that survival was prolonged in mice immunized with rTgRuvBL1, rTgRNase H1, or rTgRPS2, and then lethally challenged with T. gondii RH. However, all mice in our study died after challenge with T. gondii RH. The protective efficacy of these three recombinant proteins was weaker than that of other proteins, such as rTgEF-1 (14.53 ± 1.72 days; Wang et al., 2017), suggesting that they only weakly protected against infection by a highly virulent T. gondii strain. Although the demonstrated protection is not good enough for a vaccine, perhaps other proteins among the newly identified CAgs have better protective efficacy, similar to some other proteins identified in this study [e.g., PLP1 (Yan et al., 2011), EF-1α (Wang et al., 2017), among others].
Conclusion
In summary, we identified 31 CAgs in the sera of mice infected with T. gondii. Among these CAg molecules, three novel proteins (TgRuvBL1, TgRNase H1, and TgRPS2) were successfully confirmed to be present in the sera of the infection mouse models. Although these proteins only showed a weak protection potential against a highly virulent T. gondii strain, they may represent potential diagnostic candidates for acute T. gondii infection.
Data Availability Statement
The datasets presented in this study can be found in online repositories. The names of the repository/repositories and accession number(s) can be found below: ProteomeXchange Consortium via the PRIDE partner repository with the dataset identifier PXD021938 (https://www.ebi.ac.uk/pride/archive/projects/PXD021938).
Ethics Statement
The animal study was reviewed and approved by The Animal Care and Use Committee of the Shanghai Veterinary Research Institute approved the study and the protocols complied with approved guidelines.
Author Contributions
QL and QW participated in the experiment design and wrote the manuscript. QL and WJ analyzed the data. QL, QW, YC, MZ, and XG carried out the experiments. QL carried out bioinformatics analysis. All authors read and approved the final version of the manuscript.
Funding
This work was supported by the Shanghai Science and Technology Promotion Agriculture Innovation Program (2019No.3-3) and the National Key Research and Development Programs of China (No. 2016YFD0 501101).
Conflict of Interest
The authors declare that the research was conducted in the absence of any commercial or financial relationships that could be construed as a potential conflict of interest.
Supplementary Material
The Supplementary Material for this article can be found online at: https://www.frontiersin.org/articles/10.3389/fmicb.2020.612252/full#supplementary-material
Supplementary Figure 1 | SDS-PAGE analysis of CAg enriched and purified by immunoprecipitation. Circulating antigens enriched and purified by immunoprecipitation were analysed by SDS-PAGE (10 %). Lanes 1, 2, 3, and 4: immunoprecipitation supernatant. Due to the cracks in Lane 1 and Lane 2 is intercepted in the manuscript.
Supplementary File 1 | List of CAgs proteins identified by LC-MS/MS after IP enrichment and purification with ESA antibodies.
References
Abdollahi, S. H., Ayoobi, F., Khorramdelazad, H., Hassanshahi, G., Ahmadabadi, B. N., Rezayati, M., et al. (2013). Interleukin-10 serum levels after vaccination with in vivo prepared Toxoplasma gondii excreted/secreted antigens. Oman. Med. J. 28, 112–115. doi: 10.5001/omj.2013.29
Abdollahi, S. O., Arababadi, M. K., and Hassanshahi, G. (2009). Evaluation of excreted/secreted antigens derived from peritoneal of toxoplasma infected small mice to detect IgG against toxoplasma. Pak. J. Biol. Sci. 12, 530–533. doi: 10.3923/pjbs.2009.530.533
Aosai, F., Chen, M., Kang, H. K., Mun, H. S., and Yano, A. (2002). Toxoplasma gondii-derived heat shock protein HSP70 functions as a B cell mitogen. Cell Stress Chaperones 7, 357–364. doi: 10.1379/146612682002007<0357:tgdhsp >2.0.co;2
Asai, T., Kim, T. J., Kobayashi, M., and Kojima, S. (1987). Detection of nucleoside triphosphate hydrolase as a circulating antigen in sera of mice infected with Toxoplasma gondii. Infect. Immun. 55, 1332–1335. doi: 10.1128/IAI.55.5.1332-1335
Azami, S. J. P., Keshavarz, H., Rezaian, M., Mohebali, M., and Shojaee, S. (2011). Rapid detection of Toxoplasma gondii antigen in experimentally infected mice by Dot- ELISA. Iran. J. Parasitol. 6, 28–33.
Bogado, S. S., Dalmasso, C., Ganuza, A., Kim, K., Sullivan, W. J., Angel, S. O., et al. (2014). Canonical histone H2Ba and H2A.X dimerize in an opposite genomic localization to H2A.Z/H2B.Z dimers in Toxoplasma gondii. Mol. Biochem. Parasitol. 197, 36–42. doi: 10.1016/j.molbiopara.2014.09.009
Carlos, J. R. F., Rosalba, C. M., Mónica, E. M. C., Sirenia, G. P., Emmanuel, R. C., and Ricardo, M. F. (2019). Proteomic and structural characterization of self-assembled vesicles from excretion/secretion products of Toxoplasma gondii. Proteomics 208:103490. doi: 10.1016/j.jprot.2019.103490
Chen, J. L., Yi-yue, G., Jie, Z., Xiao-yan, Q., Jing-fan, Q., Jiang-ping, W., et al. (2013). The dysfunction of CD4+CD25+ regulatory T cells contributes to the abortion of mice Caused by Toxoplasma gondii Excreted-secreted antigens in early pregnancy. PLoS One 8:e69012. doi: 10.1371/journal.pone.0069012
Chen, R., Lu, S., Lou, D., Lin, A., and Fu, C. (2008). Evaluation of a rapid ELISA technique for detection of circulating antigens of Toxoplasma gondii. Microbiol. Immunol. 52, 180–187. doi: 10.1111/j.1348-0421.2008.00020.x
Costa-Silva, T. A., Borges, M. M., Galhardo, C. S., and Pereira-Chioccola, V. L. (2012). Immunization with excreted/secreted proteins in AS/n mice activating cellular and humoral response against Toxoplasma gondii infection. Acta Trop. 124, 203–209. doi: 10.1016/j.actatropica.2012.08.013
Csep, A., and Drãghici, S. (2013). Toxoplasma gondii infection in immunocompromised individuals. BMC Infect. Dis. 13, (Suppl. 1):P4. doi: 10.1186/1471-2334-13-S1-P4
Cuppari, A. F., Sanchez, V., Ledesma, B., Frank, F. M., Goldman, A., Angel, S. O., et al. (2008). Toxoplasma gondii protease inhibitor-1 (TgPI-1) is a novel vaccine candidate against toxoplasmosis. Vaccine. 26, 5040–5045. doi: 10.1016/j.vaccine.2008.07.031
Darcy, F., Deslee, D., Santoro, F., Charif, H., Auriault, C., Decoster, A., et al. (2010). Induction of a protective antibody-dependent response against toxoplasmosis by in vitro excreted/secreted antigens from tachyzoites of Toxoplasma gondii. Parasite Immunol. 10, 553–567. doi: 10.1111/j.1365-3024.1988.tb00242.x
Daryani, A., Hosseini, A. Z., and Dalimi, A. (2003). Immune responses against excreted/secreted antigens of Toxoplasma gondii tachyzoites in the murine model. Vet. Parasitol. 113, 123–134. doi: 10.1016/s0304-4017(03)00044-x
Daryani, A., Sharif, M., Kalani, H., Rafiei, A., and Ahmadpour, E. (2014). Electrophoretic patterns of Toxoplasma gondii excreted/secreted antigens and their role in induction of the humoral immune response. Jundishapur J. Microbiol. 7:e9525. doi: 10.5812/jjm.9525
Dautu, G., Munyaka, B., Carmen, G., Zhang, G., Omata, Y., Xuenan, X., et al. (2007). Toxoplasma gondii: DNA vaccination with genes encoding antigens MIC2, M2AP, AMA1 and BAG1 and evaluation of their immunogenic potential. Exp. Parasitol. 116, 273–282. doi: 10.1016/j.exppara.2007.01.017
Dobrowolski, J. M., and Sibley, L. D. (1996). Toxoplasma invasion of mammalian cells is powered by the actin cytoskeleton of the parasite. Cell 84, 933–939. doi: 10.1016/S0092-8674(00)81071-5
Dupont, D., Fricker-Hidalgo, H., Brenier-Pinchart, M. P., Garnaud, C., Wallon, M., Pelloux, H., et al. (2020). Serology for toxoplasma in immunocompromised patients: still useful? Trends Parasitol. S1471-4922, 30248–30248. doi: 10.1016/j.pt.2020.09.006
Elsheikha, H. M., Marra, C. M., and Zhu, X. Q. (2020). Epidemiology, pathophysiology, diagnosis, and management of cerebral toxoplasmosis. Clin. Microbiol. Rev. 34, e115–e119. doi: 10.1128/CMR.00115-19
Felgner, J., Juarez, S., Hung, C., Liang, L. I., Jain, A., Döşkaya, M., et al. (2015). Identification of Toxoplasma gondii antigens associated with different types of infection by serum antibody profiling. Parasitology 142, 827–838. doi: 10.1017/S0031182014001978
Fox, B. A., Ristuccia, J. G., and Bzik, D. J. (2009). Genetic identification of essential indels and domains in carbamoyl phosphate synthetase II of Toxoplasma gondii. Int. J. Parasitol. 39, 533–539. doi: 10.1016/j.ijpara.2008.09.011
Graindorge, A., Frénal, K., Jacot, D., Salamun, J., Marq, J. B., and Soldati-Favre, D. (2016). The conoid associated motor myoh is indispensable for Toxoplasma gondii entry and exit from host cells. PLoS Pathog. 12:e1005388. doi: 10.1371/journal.ppat.1005388
Grimwood, J., and Smith, J. E. (1992). Toxoplasma gondii: the role of a 30-kDa surface protein in host cell invasion. Exp. Parasitol. 74, 106–111. doi: 10.1016/0014-4894(92)90144-y
Hafid, J., Tran Manh Sung, R., Raberin, H., Akono, Z. Y., Pozzetto, B., and Jana, M. (1995). Detection of circulating antigens of Toxoplasma gondii in human infection. Am. J. Trop. Med. Hyg. 52, 336–339. doi: 10.4269/ajtmh.1995.52.336
Hafid, J., Vincent, N., Flori, P., Bellete, B., Raberin, H., and Sung, R. T. (2005). Production of antibodies in murine mucosal immunization with Toxoplasma gondii excreted/secreted antigens. Vet. Parasitol. 128, 23–28. doi: 10.1016/j.vetpar.2004.11.002
Hassl, A., Aspöck, H., and Flamm, H. (1988). Circulating antigen of Toxoplasma gondii in patients with AIDS: significance of detection and structural properties. Zentralbl Bakteriol Mikrobiol Hyg A 270, 302–309. doi: 10.1016/s0176-6724(88)80167-6
Herm-Götz, A., Weiss, S., Stratmann, R., Fujita-Becker, S., Ruff, C., Meyhöfer, E., et al. (2002). Toxoplasma gondii myosin A and its light chain: a fast, single-headed, plus-end-directed motor. EMBO J. 21, 2149–2158. doi: 10.1093/emboj/21.9.2149
Hide, G., Morley, E. K., Hughes, J. M., Gerwash, O., Elmahaishi, M. S., Elmahaishi, K. H., et al. (2009). Evidence for high levels of vertical transmission in Toxoplasma gondii. Parasitology 136, 1877–1885. doi: 10.1017/S0031182009990941
Hunter, C. A., and Sibley, L. D. (2012). Modulation of innate immunity by Toxoplasma gondii virulence effectors. Nat. Rev. Microbiol. 10, 766–778. doi: 10.1038/nrmicro2858
Huynh, M. H., Rabenau, K. E., Harper, J. M., Beatty, W. L., Sibley, L. D., and Carruthers, V. B. (2003). Rapid invasion of host cells by Toxoplasma requires secretion of the MIC2-M2AP adhesive protein complex. EMBO J. 22, 2082–2090. doi: 10.1093/emboj/cdg217
Jiang, W., Liu, Y., Chen, Y., Yang, Q., and Chun, P. (2015). A novel dynamic flow immunochromatographic test (DFICT) using gold nanoparticles for the serological detection of Toxoplasma gondii infection in dogs and cats. Biosens. Bioelectron. 72, 133–139. doi: 10.1016/j.bios.2015.04.035
Jones, N. G., Wang, Q., and Sibley, L. D. (2017). Secreted protein kinases regulate cyst burden during chronic toxoplasmosis. Cell Microbiol. 19:10.1111/cmi.12651. doi: 10.1111/cmi.12651
Jongert, E., Roberts, C. W., Gargano, N., Förster-Waldl, E., and Petersen, E. (2009). Vaccines against Toxoplasma gondii: challenges and opportunities. Mem. Inst. Oswaldo. Cruz. 104, 252–266. doi: 10.1590/s0074-02762009000200019
Kafsack, B. F. C., Pena, J. D. O., Coppens, I., Ravindran, S., Boothroyd, J. C., and Carruthers, V. B. (2009). Rapid membrane disruption by a perforin-like protein facilitates parasite exit from host cells. Science 323, 530–533. doi: 10.1126/science.1165740
Kang, H. J., Lee, S. H., Kim, M. J., Chu, K. B., Lee, D. H., Chopra, M., et al. (2019). Influenza virus-like particles presenting both Toxoplasma gondii ROP4 and ROP13 Enhance Protection against T. gondii infection. Pharmaceutics 11:342. doi: 10.3390/pharmaceutics11070342
Lima, T. S., and Lodoen, M. B. (2019). Mechanisms of human innate immune evasion by Toxoplasma gondii. Front. Cell Infect. Microbiol. 9:103. doi: 10.3389/fcimb.2019.00103
Long, S., Wang, Q., and Sibley, L. D. (2016). Analysis of noncanonical calcium-dependent protein kinases in Toxoplasma gondii by targeted gene deletion using CRISPR/Cas9. Infect. Immun. 84, 1262–1273. doi: 10.1128/IAI.01173-15
Mcleod, R., Cohen, W., Dovgin, S., Finkelstein, L., and Boyer, K. M. (2020). “Human Toxoplasma infection,” in Toxoplasma gondii, eds L. M. Weiss and K. Kim (Cambridge, MA: Academic), 143–148.
Meira, C. S., Costa-Silva, T. A., Vidal, J. E., Ferreira, I. M. R., and Hiramoto, R. M. (2008). Use of the serum reactivity against Toxoplasma gondii excreted-secreted antigens in cerebral toxoplasmosis diagnosis in human immunodeficiency virus-infected patients. J. Med. Microbiol. 57(Pt 7), 845–850. doi: 10.1099/jmm.0.47687-0
Meira, C. S., Vidal, J. E., Costa-Silva, T. A., Frazatti-Gallina, N., and Pereira-Chioccola, V. L. (2011). Immunodiagnosis in cerebrospinal fluid of cerebral toxoplasmosis and HIV-infected patients using Toxoplasma gondii excreted/secreted antigens. Diagn. Microbiol. Infect. Dis. 71, 279–285. doi: 10.1016/j.diagmicrobio.2011.07.008
Mohamed, R. M., Aosai, F., Mei, C., Mun, H. S., Norose, K., Belal, U. S., et al. (2003). Induction of protective immunity by DNA vaccination with Toxoplasma gondii HSP70, HSP30 and SAG1 genes. Vaccine 21, 2852–2861. doi: 10.1016/s0264-410x(03)00157-9
Montoya, J. G., and Liesenfeld, O. (2004). Toxoplasmosis. Lancet 363, 1965–1976. doi: 10.1016/S0140-6736(04)16412-X
Morris, M. T., Coppin, A., Tomavo, S., and Carruthers, V. B. (2002). Functional analysis of Toxoplasma gondii protease inhibitor 1. J. Biol. Chem. 277, 45259–45266. doi: 10.1074/jbc.M205517200
Nissapatorn, V. (2009). Toxoplasmosis in HIV/AIDS: a living legacy. Southeast Asian. J. Trop. Med. Public Health 40, 1158–1178.
Poupel, O., Boleti, H., Axisa, S., Couture-Tosi, E., and Tardieux, I. (2000). Toxofilin, a novel actin-binding protein from Toxoplasma gondii, sequesters actin monomers and caps actin filaments. Mol. Biol. Cell 11, 355–368. doi: 10.1091/mbc.11.1.355
Robert-Gangneux, F., and Dardé, M. L. (2012). Epidemiology of and diagnostic strategies for toxoplasmosis. Clin. Microbiol. Rev. 25, 264–296. doi: 10.1128/CMR.05013-11
Song, P., He, S., Zhou, A., Lv, G., Guo, J., Zhou, J., et al. (2017). Vaccination with toxofilin DNA in combination with an alum-monophosphoryl lipid A mixed adjuvant induces significant protective immunity against Toxoplasma gondii. BMC Infect. Dis. 17:19. doi: 10.1186/s12879-016-2147-1
Turetzky, J. M., Chu, D. K., Hajagos, B. E., and Bradley, P. J. (2010). Processing and secretion of ROP13: a unique Toxoplasma effector protein. Int. J. Parasitol. 40, 1037–1044. doi: 10.1016/j.ijpara.2010.02.014
Wang, H. L., Wang, Y. J., Pei, Y. J., Bai, J. Z., Yin, L. T., Guo, R., et al. (2016). DNA vaccination with a gene encoding Toxoplasma gondii Rhoptry Protein 17 induces partial protective immunity against lethal challenge in mice. Parasite 23:4. doi: 10.1051/parasite/2016004
Wang, J. L., Huang, S. Y., Li, T. T., Chen, K., Ning, H. R., and Zhu, X. Q. (2016). Evaluation of the basic functions of six calcium-dependent protein kinases in Toxoplasma gondii using CRISPR-Cas9 system. Parasitol. Res. 115, 697–702. doi: 10.1007/s00436-015-4791-6
Wang, H. L., Ya-Qing, L., Li-Tian, Y., Xiao-Li, M., Min, G., Jian-Hong, Z., et al. (2013). Toxoplasma gondii protein disulfide isomerase (TgPDI) is a novel vaccine candidate against toxoplasmosis. PLoS One 8:e70884. doi: 10.1371/journal.pone.0070884
Wang, Q., Jiang, W., Chen, Y. J., Liu, C. Y., Shi, J. L., and Li, X. T. (2012). Prevalence of Toxoplasma gondii antibodies, circulating antigens and DNA in stray cats in Shanghai, China. Parasit Vectors 5:190. doi: 10.1186/1756-3305-5-190
Wang, S., Zhang, Z., Wang, Y., Gadahi, J. A., Xu, L., Yan, R., et al. (2017). Toxoplasma gondii elongation Factor 1-Alpha (TgEF-1α) is a novel vaccine candidate antigen against toxoplasmosis. Front. Microbiol. 8:168. doi: 10.3389/fmicb.2017.00168
Wengelnik, K., Daher, W., and Lebrun, M. (2018). Phosphoinositides and their functions in apicomplexan parasites. Int. J. Parasitol. 48, 493–504. doi: 10.1016/j.ijpara.2018.01.009
Wohlfert, E. A., Blader, I. J., and Wilson, E. H. (2017). Brains and brawn: toxoplasma infections of the central nervous system and skeletal muscle. Trends Parasitol. 33, 519–531. doi: 10.1016/j.pt.2017.04.001
Xue, J., Jiang, W., Chen, Y., Liu, Y., Zhang, H., Xiao, Y., et al. (2016). Twenty-six circulating antigens and two novel diagnostic candidate molecules identified in the serum of canines with experimental acute toxoplasmosis. Parasites Vectors 9:374. doi: 10.1186/s13071-016-1643-x
Xue, J., Jiang, W., Li, J., Xiong, W., Tian, Z., Zhang, Q., et al. (2019). Toxoplasma gondii RPL40 is a circulating antigen with immune protection effect. Folia Parasitol. 66:2019.013. doi: 10.14411/fp.2019.013
Yan, H. K., Yuan, Z.-G., Petersen, E., Zhang, X.-X., Zhou, D.-H., Liu, Q., et al. (2011). Toxoplasma gondii: protective immunity against experimental toxoplasmosis induced by a DNA vaccine encoding the perforin-like protein 1. Exp Parasitol. 128, 38–43. doi: 10.1016/j.exppara.2011.02.005
Yu, Z., Zhou, T., Luo, Y., Dong, L., Li, C., Chen, M., et al. (2020). Modulation effects of Toxoplasma gondii histone H2A1 on murine macrophages and encapsulation with polymer as a vaccine candidate. Vaccines 8:E731. doi: 10.3390/vaccines8040731
Zhou, X. W., Kafsack, B. F. C., Cole, R. N., Beckett, P., Shen, R. F., and Carruthers, V. B. (2005). The opportunistic pathogen Toxoplasma gondii deploys a diverse legion of invasion and survival proteins. J. Biol. Chem. 280, 34233–34244. doi: 10.1074/jbc.M504160200
Keywords: Toxoplasma gondii, acute infection, circulating antigens, proteomics, diagnostic candidates
Citation: Liu Q, Jiang W, Chen Y, Zhang M, Geng X and Wang Q (2021) Study on Circulating Antigens in Serum of Mice With Experimental Acute Toxoplasmosis. Front. Microbiol. 11:612252. doi: 10.3389/fmicb.2020.612252
Received: 30 September 2020; Accepted: 23 December 2020;
Published: 18 January 2021.
Edited by:
Hong-Juan Peng, Southern Medical University, ChinaReviewed by:
Shuai Wang, Xinxiang Medical University, ChinaSi-Yang Huang, Yangzhou University, China
Copyright © 2021 Liu, Jiang, Chen, Zhang, Geng and Wang. This is an open-access article distributed under the terms of the Creative Commons Attribution License (CC BY). The use, distribution or reproduction in other forums is permitted, provided the original author(s) and the copyright owner(s) are credited and that the original publication in this journal is cited, in accordance with accepted academic practice. No use, distribution or reproduction is permitted which does not comply with these terms.
*Correspondence: Quan Wang, d2FuZ3F1YW5Ac2h2cmkuYWMuY24=