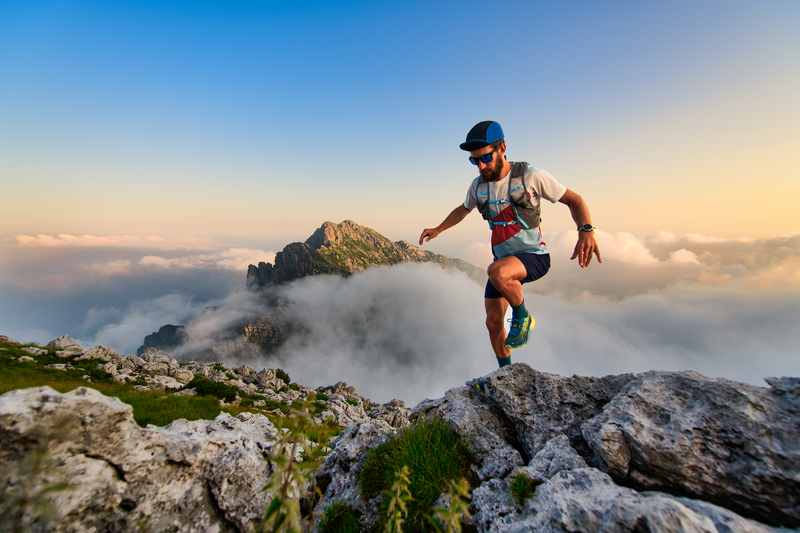
95% of researchers rate our articles as excellent or good
Learn more about the work of our research integrity team to safeguard the quality of each article we publish.
Find out more
ORIGINAL RESEARCH article
Front. Microbiol. , 11 January 2021
Sec. Antimicrobials, Resistance and Chemotherapy
Volume 11 - 2020 | https://doi.org/10.3389/fmicb.2020.610296
This article is part of the Research Topic Antimicrobial Resistance in Aquatic Environments View all 15 articles
In this paper we describe the transmission of a multi-drug resistant Klebsiella pneumoniae ST101 clone from hospital to wastewater and its persistence after chlorine treatment. Water samples from influents and effluents of the sewage tank of an infectious diseases hospital and clinical strains collected from the intra-hospital infections, during a period of 10 days prior to wastewater sampling were analyzed. Antibiotic resistant K. pneumoniae strains from wastewaters were recovered on selective media. Based on antibiotic susceptibility profiles and PCR analyses of antibiotic resistance (AR) genetic background, as well as whole-genome sequencing (Illumina MiSeq) and subsequent bioinformatic analyses, 11 ST101 K. pneumoniae strains isolated from hospital wastewater influent, wastewater effluent and clinical sector were identified as clonally related. The SNP and core genome analyses pointed out that five strains were found to be closely related (with ≤18 SNPs and identical cgMLST profile). The strains belonging to this clone harbored multiple acquired AR genes [blaCTX–M–15, blaOXA–48, blaOXA–1, blaSHV–106, blaTEM–150, aac(3)-IIa, aac(6′)-Ib-cr, oqxA10, oqxB17, fosA, catB3, dfrA14, tet(D)] and chromosomal mutations involved in AR (ΔmgrB, ΔompK35, amino acid substitutions in GyrA Ser83Tyr, Asp87Asn, ParC Ser80Tyr). Twenty-nine virulence genes involved in iron acquisition, biofilm and pili formation, adherence, and the type six secretion system – T6SS-III were identified. Our study proves the transmission of MDR K. pneumoniae from hospital to the hospital effluent and its persistence after the chlorine treatment, raising the risk of surface water contamination and further dissemination to different components of the trophic chain, including humans.
Due to the worldwide use of antibiotics in the treatment of human and animal infectious diseases, but also in livestock and agriculture, a large amount of antibiotics of pharmaceutical origin are found in anthropic environments, such as sewage and wastewater treatment plants (WWTPs) and ends up being discharged in the natural environment (Kraemer et al., 2019; Zhang T. et al., 2019; Zhang Z. et al., 2019). The extensive use of antibiotics leads to the spread of antibiotic resistance (AR), which currently represents a major public health concern. AR rates are particularly high in acute care hospitals driven by selective pressure of antibiotic usage (Hocquet et al., 2016), hospitals being main ecological niches for the selection, accumulation, and spread of antibiotic resistant bacteria (ARB).
Water plays a crucial role in the spreading of AR, through inappropriate release of human and animal effluents in the surface waters through hospital wastewaters, WWTPs, aquaculture farms, surface, and groundwater. Hospital wastewaters are highly complex effluents, carrying a wide range of micro- and macropollutants, including antibiotic compounds, metabolized drugs, disinfectants, patient excrements, and microorganisms. The presence of ARB as well as of antibiotic residues, which could inhibit the growth of susceptible bacteria, are thereby increasing the population of resistant bacteria in the receiving water (Kaur et al., 2020; Rozman et al., 2020).
Hospitals generate a large amount of wastewater per day. The hospital effluents are loaded with pathogenic microorganisms, antibiotics, and other pharmaceutical or toxic substances, which are only partially removed during wastewater treatments, driving the pollution of the natural environments, including selection, and dissemination of AR (Kummerer et al., 2000; Kim and Aga, 2007; Alrhmoun et al., 2014; Laffite et al., 2016). Antibiotic pollutants, as well as heavy metals and even chlorination, could increase the general rates of mutation, recombination, and lateral gene transfer, thus recruiting more genes into the resistome and mobilome, and simultaneously providing the selective force to fix such changes, acting as drivers of bacterial evolution, with potentially adverse consequences for human welfare (Gillings, 2013). To minimize the risk of superbugs selection, the World Health Organization (WHO, 2014) recommends that hospitals have onsite facilities for the pre-treatment of hospital effluent prior to discharge into the general wastewater streaming1; the purpose is to eliminate different contaminants, such as bacterial pathogens, antibiotics, disinfectants, radioactive substances, toxic chemicals, etc.
Considering the increasing risk of microbial infections by reclaiming water through processing it in WWTPs, advanced treatment technologies and disinfection process are considered a major tool to control the spread of ARB into the environment (Rizzo et al., 2013), one of the most widely used and accepted ways to achieve it being through chlorine treatment (Huang et al., 2011). Chlorine efficiency depends on the concentration, exposure time and the formulation used. It has been shown that chlorine may also contribute to the selection of bacteria highly resistant to tetracycline, chloramphenicol, trimethoprim, and to the accumulation of various ARGs (such as ampC, aphA2, blaTEM–1, tetA/G, ermA/B, plasmids, insertion sequences, and integrons) (Shi et al., 2013). In addition, metagenomic analyses performed on wastewater samples after chlorine treatment, shows that up to 40% of erythromycin resistance genes and 80% of tetracycline resistance genes cannot be removed (Yuan et al., 2015). Furthermore, chlorine treatment may promote conjugation and the ARGs transmission through horizontal gene transfer of mobile genetic elements and also plasmid over-replication and the emergence of multi-drug resistance (MDR) through activation of multi-drug efflux pumps (Shi et al., 2013; Popa et al., 2018; Sanganyado and Gwenzi, 2019; Vrancianu et al., 2020).
Multi-drug resistant Klebsiella pneumoniae is a major nosocomial pathogen, causing infections with high morbidity and mortality rates (up to 50%) (Bassetti et al., 2018), caused by limited treatment options. This pathogen harbors a wide resistome that could evolve under antibiotic and biocide selective pressure, leading to the occurrence of extremely drug resistant (XDR) or high-risk (HiR) clones, with great epidemic potential (Navon-Venezia et al., 2017).
As many as 90,000 infections and more than 7,000 deaths in Europe are attributable to K. pneumoniae resistant to carbapenems, to colistin or producing extended spectrum β-lactamase (ESBL) (Cassini et al., 2015). In 2018, resistance to carbapenems (last resort antibiotics) in K. pneumoniae ranged in various countries from 0 to 63.9%, the highest prevalence being encountered in Greece, followed by Romania (29.5%) and Italy (26.8%) (EARSS-Net), Europe being considered “epidemic” for carbapenemase- producing K. pneumoniae (Bassetti et al., 2018). The dissemination of MDR K. pneumoniae strains from hospitals to the environment was previously demonstrated (Mahon et al., 2017; Khan et al., 2018; Lepuschitz et al., 2019), highlighting the ability of these strains to survive and persists in environmental conditions. Despite this evidence, primary treatment of hospital wastewaters before their discharge in the urban sewage is not mandatory in many countries (Hocquet et al., 2016; Rozman et al., 2020), these wastewaters ending up being treated in urban wastewater treatment plants. One of the primary treatments of hospital wastewaters used in Romania is represented by chlorine treatment, but the knowledge regarding the effects of chlorination on ARB is scarce. The current literature reports conflicting results, since some studies describe the removal of some ARB by chlorine treatment (Zhang et al., 2015; Lin et al., 2016), while other data suggest that this treatment is ineffective (Yuan et al., 2015).
Previous data from our research team (Surleac et al., 2020) showed that MDR, carbapenemase and ESBL-producing K. pneumoniae isolated from clinics, hospital wastewater, and urban WWTPs in different regions of the country exhibit multiple antibiotic and antiseptic resistance, as well as virulence genes, the ST101 clone being the most frequently encountered in all sampling sites. The K. pneumoniae ST101 clone seems to be well established in Romanian hospitals (Dortet et al., 2015; Czobor et al., 2016) and wastewaters (Surleac et al., 2020), this determining us to investigate its possible transmission from hospital to wastewater, aiming to comparatively characterize the K. pneumoniae ST101 isolated from an infectious diseases hospital and its wastewaters.
Grab water samples were collected in November (21st and 23rd) 2018, and March (20) 2019 from the influent and the effluent of the hospital collecting sewage tank (Figure 1), in which active chlorine solution (0.06 g/L) is intermittently pulverized, according to the hospital standard operating procedure. Water samples were processed following the recommendations of SR EN ISO 9308-2/2014 (coliform bacteria). Briefly, different water volumes and dilutions (undiluted 1 ml, 10 ml, and 30 ml as well as 1 ml and 3 ml out of ten-fold dilutions – 1/10 and 1/100, respectively) were filtered through 0.45 μm pore size membranes (Millipore, France), subsequently inoculated on antibiotic-enriched, chromogenic media (BioMérieux, France), namely ChromID ESBL (for ESBL-producing enterobacteria), ChromID OXA-48 agar and ChromID CARBA agar for carbapenemase (CRE)-producing strains. Up to ten colonies with KESC (Klebsiella – Enterobacter – Serratia – Citrobacter) carbapenem-resistance phenotype were randomly selected from each culture media per sample. The isolates were confirmed by subsequent cultivation on the same type of chromogenic media used for their isolation, identified using the MALDI-TOF-MS Bruker system and subsequently introduced in the microbial collection of the Research Institute of the University of Bucharest. In order to evaluate the occurrence of particular clones of MDR K. pneumoniae in hospital wastewater, 10 days prior to water sampling, all K. pneumoniae strains isolated from positive clinical specimens, were collected by the hospital microbiology laboratory and provided for comparative analysis (Supplementary Table 1). The clinical strains were sampled from inpatients, cultured and subsequently isolated on blood agar, and Cystine Lactose Electrolyte Deficient (CLED) agar (Rafila et al., 2015), identified using MALDI-TOF-MS Bruker system, and included in the microbial collection of the Research Institute of the University of Bucharest without any link to personal data regarding the patients.
The two colonies of K. pneumoniae selected from each chromogenic media (ChromID ESBL, OXA-48, and CARBA) and each volume/dilution used for isolation, as well as the clinical K. pneumoniae strains were further studied using the disk diffusion method (CLSI, 2019) and the following 16 antibiotics: amikacin (AK), amoxicillin-clavulanic acid (AMC), ampicillin (AMP), aztreonam (ATM), cefepime (FEP), cefoxitin (FOX), ceftriaxone (CRO), cefuroxime (CXM), ciprofloxacin (CIP), ertapenem (ETP), gentamicin (CN), imipenem (IMP), meropenem (MEM), piperacillin (PRL), tetracycline (TET), trimethoprim-sulfamethoxazole (SXT). Escherichia coli ATCC 25922 strain was used for quality control.
In order to investigate the genetic support of β-lactam enzymatic resistance (carbapenemases, ESBLs), bacterial DNA was extracted using an adapted alkaline extraction method (Almahdawy et al., 2019). The genetic background of AR was investigated by PCR (PCR Master Mix 2X, Thermo Scientific), using 1 μl of DNA and specific primers for blaTEM (Quinteros et al., 2003), blaSHV (Kim and Lee, 2000), blaCTX–M (Israil et al., 2013), blaOXA–48 (Poirel et al., 2011), blaVIM (Shirani et al., 2016), blaIMP (Shirani et al., 2016), blaNDM (Nordmann et al., 2011), blaSIM (Qi et al., 2008), blaSPM (Ellington et al., 2007), and blaKPC (Poirel et al., 2011) genes (Supplementary Table 2). All reactions were carried out using the temperature of 55°C for primers annealing, except for blaTEM and blaIMP, for which the annealing was performed at 52°C.
The aquatic strains from the three sampling points (n = 23) showing matching AR profiles with the clinical strains (n = 8) (Supplementary Table 3) were selected for whole genome sequencing. Total DNA was extracted (DNeasy UltraClean Microbial Kit Qiagen optimized with an additional mechanical and chemical bacterial lysis step and ethanol precipitation) and subjected to Illumina (Nextera DNA Flex Library Prep Kit) sequencing. Both quality (2100 Bioanalyzer, Agilent) and quantity (Qubit 4 Fluorometer, Thermo Fisher Scientific, United States) checks were performed on the DNA pool libraries before starting the paired-end shotgun sequencing on the MiSeq platform (Illumina, United States). MiSeq reagent kit v.3 (600 cycles) was chosen for the high quality of the generated output.
Raw reads were quality-checked using FastQC (Andrews, 2010), assembled using Shovill pipeline (Seemann, 2018), and primarily annotated using Prokka (Seemann, 2014). Specific gene profiling was assessed using ABRicate (Seemann, 2020b) software, with specific databases for AR (Feldgarden et al., 2019), virulence (Chen et al., 2016), and plasmid replicons (Carattoli et al., 2014). ARIBA was also used for predicting the resistance genes for each sample (Hunt et al., 2017). Moreover, we also interrogated the database provided by the online CGE platform – http://www.genomicepidemiology.org/- (CGE Platform, 2020), regarding the resistance (Zankari et al., 2012), virulence (Joensen et al., 2014) and the pathogenic potential using Pathogen Finder predictor software (Cosentino et al., 2013). Capsular and LPS antigens (K and O loci) and chromosomal mutations involved in AR were annotated using Kleborate (Wyres et al., 2016; Wick et al., 2018). Strain relatedness was investigated using MLST (Seemann, 2020a), cgMLST (Jolley and Maiden, 2010) Snippy (Seemann, 2015) and kSNP3 (Gardner et al., 2015). Comparative gene analyses were performed using Roary (Page et al., 2015) and the output was used to infer phylogenies using RAxML (Stamatakis, 2014) and visualized using iTOL (Letunic and Bork, 2019).
Out of a total of 101 carbapenem resistant K. pneumoniae strains, 78 isolated from wastewater and 23 from inpatients, some of them previously characterized for their antibiotic susceptibility profiles (Surleac et al., 2020), 31 were initially selected for the present study, based on their sampling location and MDR phenotype, as defined by Magiorakos et al., 2012 (Supplementary Table 3). For all the 31 strains, the MLST profile was inferred from WGS data (Surleac et al., 2020). The MLST profiles revealed by WGS data analyses highlighted that the K. pneumoniae ST101 subtype was the most prevalent (n = 11, 36%) in all three sampling points (Supplementary Figure 2). Thus, these isolated were further selected for characterization.
For the 31 strains selected, the antibiotic susceptibility profiles, the genetic background of β-lactam resistance and ST-types were compared, (Supplementary Table 3) revealing the presence of matching patterns of AR profiles (i.e., resistance to same classes of antibiotics, or to a single antibiotic from an antibiotic class), the presence/absence of ARGs and the abundance of K. pneumoniae ST101 The PCR for detection of β-lactam resistance genes revealed the presence of carbapenemases genes, which were, in decreasing frequency order, blaOXA–48, blaNDM, and blaKPC as well as of the blaCTX–M encoding for ESBLs. The blaSHV gene was detected in all isolates (Supplementary Table 3).
All these strains were further characterized by whole genome sequencing. The ARGs identified by gene prediction were diverse and encoded resistance to β-lactams, aminoglycosides, quinolones, folate inhibitors, tetracyclines and others; no significant decrease of ARG distribution in the chlorine-treated effluent of vs. untreated influent of the hospital chlorination tank was observed, the majority of the ARGs being present in the aquatic strains isolated from all three sampling points. The most frequent genes encoding for β-lactam resistance were blaCTX–M–15, blaOXA–1, blaOXA–48, blaSHV–106, blaTEM–1, and blaSHV–158, for aminoglycosides were aac(3)II-a, aph(6)-Id, aph(3″)-Ib, and aadA2, while for quinolone resistance the transferable qnrS1 gene was the most abundant (Supplementary Figure 1).
The main characteristics of the selected K. pneumoniae ST101 strains were: the presence of the MDR phenotype, three wastewater-sourced strains being resistant to all tested antibiotics (22 bac, 23 bac, 34bac), while the other two were susceptible only to aztreonam (29 bac, 82 bac). All six clinical strains were resistant to all tested antibiotics except for amikacin to which four strains were susceptible (Supplementary Table 3).
All K. pneumoniae ST101 strains selected for WGS presented the KL17/O1v1 serotype (as predicted by Kleborate software). All of them harbored multiple common ARGs (Table 1) encoding for β-lactam (mainly carbapenemase blaOXA–48), quinolone (oqxA10, oqxB17) and trimethoprim (dfrA14) resistance, as well as chromosomal mutations involved in resistance to quinolones (mutations of gyrA and parC), colistin (truncation of mgrB gene) and linked with the decreased susceptibility to cephalosporins and carbapenems (truncation of porin encoding gene OmpK35).
Virulence determinants harbored by the analyzed strains were represented by siderophores, such as enterobactin (entABCDEF, fepABCDG) and yersiniabactin (irp1, irp2, ybtSXQPA, fyuA), as well as the operon ecpRABCDE, involved in adherence and biofilm formation. The biocides resistance gene mdfA encodes resistance to quaternary-ammonium compounds, sodium hydroxide and other biocides, while merA encodes resistance to mercury (Table 1).
Additionally, the majority of the strains harbored ESBL (blaTEM–150, blaCTX–M–15), tetracyclines – tet(D), aminoglycoside- mainly aac(3)-IIa, chloramphenicol -catB3 genes and presented an amino acid substitution in OmpK36 porin, which is known to be associated with carbapenem resistance (Table 1).
The pathogenic potential was estimated to 90%, according to Pathogen Finder predictor software (Cosentino et al., 2013). All strains harbored the same plasmid replicons: IncFIA, IncL/M, IncR_1, and Col, respectively.
The mobile genetic platform harboring aac(6′)-Ib-cr – blaOXA–1 – catB3 was encountered in all isolates, in three of them, respectively, the clinical strain 36bac and the wastewater isolates 34bac and 29bac being flanked by IS26 at both ends. In the other tested strains the aac(6′)-Ib-cr gene was truncated (this might be probably due to sequencing limitations) (Figure 2).
Figure 2. Antibiotic resistance (AR) platform carrying three different resistance genes: aac (6′) lb-cr, blaOXA–1, and catB3. (A) The whole fragment flanked by two copies of IS26. (B) Truncated aac(6′) Ib-cr gene.
Core SNPs analyses revealed that three clinical strains isolated in November 2018 (36bac, 41bac, and 43 bac) are closely related (≤18 SNPs) with two strains isolated on the 23rd November 2018 from influent (22bac) and effluent (23bac), respectively; the wastewater sourced strains presented no SNPs in their core site (Table 2). Moreover, cgMLST profiling revealed the same allelic content. Additionally, 99% of the total genes detected in these strains were common (core-genes). This low variability allowed us to hypothesize that the three selected strains belong to the same clone, more as they were tracked in the same temporal sequence, in three spatial points of the hospital-wastewater transmission chain.
Table 2. Matrix representation of calculated SNPs distances between the closely related strains (≤18 SNPs highlighted in gray).
These strains, isolated in November 2018, were related with those isolated in March 2019 (Table 2), where one clinical strain (89 bac) was found to be closely related with a strain isolated from the hospital effluent (82 bac) and having the same cgMLST profile. All strains presented in Table 1 are clustered together in the Maximum likelihood phylogeny (Figure 3). Two strains from influent (29 bac, 34 bac) and one clinical strain (37 bac) isolated in November 2018, although belonging to ST101 clone, were more distantly related (55–799 SNPs) and belong to a different cluster.
Figure 3. Maximum likelihood phylogeny of all ST101 K. pneumoniae isolated from hospital sewage: influent (IN), effluent (EF) of chlorination tank, and from clinical samples (CL) with isolation date.
Although clinically relevant clones, such as carbapenem producing K. pneumoniae have been isolated in different environments, data on the occurrence and characteristics of K. pneumoniae resistant strains in environmental sources are still scarce (Chi et al., 2019). Our study describes the presence of the same clone of K. pneumoniae MDR in the hospital wastewater, in the untreated influent as well in the effluent of the chlorination tank, highlighting the inefficiency of the chlorine treatment in removing MDR K. pneumoniae from hospital wastewater before being released to urban wastewater collecting system.
In order to prove the clonal dissemination of K. pneumoniae from the clinical compartment to the hospital wastewater, a detailed characterization of the ST101 MDR K. pneumoniae strains recovered from hospital wastewater before and after chlorination and inpatients was performed. We have demonstrated that five MDR K. pneumoniae ST101 strains isolated from intra-hospital infections and two strains isolated from hospital wastewater (hospital WWTP influent and effluent) in November 2018 belong to the same clone, harboring common AR, virulence, and biocides resistance genes, two strains belonging to the same clone being isolated also in March 2019 (isolated from inpatients and from the hospital WWTP effluent, respectively).
The most important feature of the successful K. pneumoniae ST101 clone isolated in November 2018 was its resistance to multiple antibiotics, encoded by chromosomal mutations, as well as by resistance genes acquired through horizontal gene transfer, located within mobile genetic elements which could potentially disseminate to commensal bacterial strains. The virulence determinants harbored by this successful clone were represented by siderophores such as enterobactin (entABCDEF, fepABCDG) and yersiniabactin (irp1, irp2, ybtSXQPA, fyuA), their co-presence being associated with an increased risk of respiratory tract infections (Bachman et al., 2011), as well as the operon ecpRABCDE, involved in adherence and biofilm formation. The variants of capsular and somatic antigens KL17 and O1v1 were described as strongly associated with K. pneumoniae ST101 (Roe et al., 2019). In addition, the O1 antigen has been described as a major contributor to the virulence of pyogenic liver abscess causing K. pneumoniae (Hsieh et al., 2012). Additionally, the biocides resistance gene mdfA encodes resistance to quaternary-ammonium compounds, sodium hydroxide and other biocides, while merA encodes resistance to mercury. Although genes associated with resistance and virulence are usually identified in separate subpopulations of K. pneumoniae there are strains harboring both high resistance and virulence (Lam et al., 2019; Wyres et al., 2020b) making them highly pathogenic and almost impossible to treat (Wyres et al., 2020b).
Our data point that the K. pneumoniae clone presented here may have the ability to survive in the urban wastewater after being released in the hospital sewage. The persistence of this clone in the hospital effluent after chlorination indicates its dissemination potential in surface and recreational waters, as previously suggested by other authors (Lepuschitz et al., 2019; Suzuki et al., 2020).
Therefore, multi-level studies are needed for increasing knowledge on ecology, population structure and pathogenicity of resistant K. pneumoniae strains and to elucidate the possible transmission of clinical strains into the environment and the subsequent potential risk posed to human and environmental health (Wyres et al., 2020a).
Extended spectrum β-lactamase and carbapenem producing K. pneumoniae were previously isolated from different components of the hospital sewage treatment facilities, demonstrating the dissemination of ESBL producers between intra-hospital infections and the final effluent after the treatment process (Prado et al., 2008). The identification of VIM and KPC-producing Klebsiella spp. in the treated wastewater of a hospital (Gomi et al., 2018), of OXA-48 producing K. pneumoniae from hospital sewage (Zurfluh et al., 2017), or of the presence of the same clone in the hospital sewage and receiving river (Suzuki et al., 2020) raises concerns. Since its first detection in Székely et al. (2013), OXA-48 was the most frequently encountered carbapenemase in Romania (Lixandru et al., 2015; Grundmann et al., 2017; Popescu et al., 2017; Baicus et al., 2018; Surleac et al., 2020).
The IncFII, IncN, IncR, and IncX3 incompatibility groups are most often associated with the horizontal gene transfer of AR genes in K. pneumoniae determined mainly by the presence of the following replicons: FIBK, FIIK, R, Col, FII, FIA (all identified in this study) FIB, X, N, HI1B, AC/2 (Wyres et al., 2020a). Beside these replicons, we have also identified the IncL/M replicon in all isolates. The acquired ARGs in K. pneumoniae ST101 isolates are associated with particular mobile elements, e.g., the association of blaCTX–M–15 with IncFII plasmids while blaOXA–48 was often identified in IncL/M plasmids (Wyres and Holt, 2016), as also revealed by our results.
One might consider a limitation of this study the low number of the selected MDR K. pneumoniae strains belonging to the same clone traveling from hospital to WWTP effluents. Further studies, with more sampling campaigns and more isolates are required to provide the epidemiological link from the clinical compartment to the water bodies through hospital wastewater as well as the correlation between the persistence of the clones and different exposure times to chlorination treatment.
The survival of ARB in treated hospital wastewater is very alarming and highlights the necessity of an improved surveillance and the need to elucidate the role of the environment in the transmission and dissemination of MDR K. pneumoniae strains. The isolation of the same clone from both hospital and WWTP influent and effluent after chlorination suggests the highly adaptive potential of the clone and highlights the need for further studies designed to track the fate of these clones after release from hospital in the aquatic environment. In addition, disinfection strategies for hospital wastewaters should be reconsidered, in the light of such novel epidemiological data.
The datasets presented in this study can be found in online repositories. The names of the repository/repositories and accession number(s) can be found below: https://www.ncbi.nlm.nih.gov/, BioProject PRJNA579879.
LP: conceptualization, data curation, methodology, software, formal analysis, and writing – original draft. IG: conceptualization, data curation, methodology, formal analysis, and writing – original draft. IB: conceptualization, data curation, formal analysis, software, and writing – original draft. MS: data curation, formal analysis, software, and writing – review and editing. SP: conceptualization, methodology, and writing – review and editing. LM, MP, GP, DT, MN, and AnS-C: methodology. AdS-C: conceptualization. DO: conceptualization and writing – review and editing. MC: conceptualization, data curation, formal analysis, funding acquisition project administration, supervision, and writing – review and editing. All authors contributed to the article and approved the submitted version.
The authors declare that the research was conducted in the absence of any commercial or financial relationships that could be construed as a potential conflict of interest.
This study was supported by the Romanian Executive Agency for Higher Education, Research, Development and Innovation (https://uefiscdi.gov.ro/) research projects PN-III-P4-ID-PCCF-2016-0114 POSCCE (RADAR) and PN-III-P1-1.1-PD-2016-2137, National Council for the Financing of Higher Education (CNFIS) research project FDI-2020-0834, the ERANET-JPI-EC-AMR – AWARE-WWTP (AWARE-WWTP), and by Research Institute of the University of Bucharest (ICUB) grant no. 20964/30.10.2020. The funding had no role in study design, data collection and analysis, decision to publish, or preparation of the manuscript.
The Supplementary Material for this article can be found online at: https://www.frontiersin.org/articles/10.3389/fmicb.2020.610296/full#supplementary-material
Supplementary Figure 1 | The distribution of antibiotic resistance (AR) genes to β-lactam antibiotics. (A) aminoglycosides and quinolones, (B) and other classes of antibiotics, (C) predicted from WGS data in the analyzed K. pneumoniae strains.
Supplementary Figure 2 | MLST profiles of the K. pneumoniae strains isolated from the clinical compartment, influent, and effluent of the chlorination tank.
Almahdawy, O. T., Pricop, R., Sadik, O., Najee, H., Pircalabioru, G., Marutescu, L., et al. (2019). Description of vancomycin resistance genes in Enterococcus sp. clinical strains isolated from Bucharest, Romania. Rom. Biotechnol. Lett. 24, 395–399. doi: 10.25083/rbl/24.3/395.399
Alrhmoun, M., Carrion, C., Casellas, M., and Dagot, C. (2014). “Hospital wastewater treatment by membrane bioreactor: performance and impact on the biomasses,” in Proceedings of the International Conference on Biological, Civil and Environmental Engineering, (BCEE-2014) March 17-18, Dubai (UAE).
Andrews, S. (2010). FastQC: A Quality Control Tool for High Throughput Sequence Data. Available online at: http://www.bioinformatics.babraham.ac.uk/projects/fastqc (accessed December 18, 2020).
Bachman, M. A., Oyler, J. E., Burns, S. H., Caza, M., Lepine, F., Dozois, C. M., et al. (2011). Klebsiella pneumoniae yersiniabactin promotes respiratory tract infection through evasion of lipocalin 2. Infect. Immun. 79, 3309–3316. doi: 10.1128/IAI.05114-11
Baicus, A., Lixandru, B., Stroia, M., Cirstoiu, M., Constantin, A., Usein, C. R., et al. (2018). Antimicrobial susceptibility and molecular epidemiology of carbapenem-resistant Klebsiella pneumoniae strains isolated in an emergency university hospital. Rom. Biotechnol. Lett. 23, 13525–13529.
Bassetti, M., Righi, E., Carnelutti, A., Graziano, E., and Russo, A. (2018). Multidrug-resistant Klebsiella pneumoniae: challenges for treatment, prevention and infection control. Expert Rev. Anti Infect. Ther. 16, 749–761. doi: 10.1080/14787210.2018.1522249
Carattoli, A., Zankari, E., Garcia-Fernandez, A., Larsen, M. V., Lund, O., Villa, L., et al. (2014). In silico detection and typing of plasmids using PlasmidFinder and plasmid multilocus sequence typing. Antimicrob. Agents Chemother. 58, 3895–3903. doi: 10.1128/AAC.02412-14
Cassini, A., Hogberg, L. D., Plachouras, D., Quattorocchi, A., Hoxha, A., Simones, G. S., et al. (2015). Attributable deaths and disability-adjusted life-years caused by infections with antibiotic-resistant bacteria in the EU and the European Economic Area in 2015: a population-level modelling analysis. Lancet Infect. Dis. 19, 56–66. doi: 10.1016/S1473-3099(18)30605-4
CGE Platform (2020). Available online at: http://www.genomicepidemiology.org/ (accessed June 24, 2020).
Chen, L., Zheng, D., Liu, B., Yang, J., and Jin, Q. (2016). VFDB 2016: hierarchical and refined dataset for big data analysis-10 years on. Nucleic Acids Res. 44, D694–D697. doi: 10.1093/nar/gkv1239
Chi, X., Berglund, B., Zou, H., Zheng, B., Borjesson, S., Ji, X., et al. (2019). Characterization of clinically relevant strains of extended-spectrum β-lactamase-producing Klebsiella pneumoniae occurring in environmental sources in a rural area of China by using whole-genome sequencing. Front. Microbiol. 10:211. doi: 10.3389/fmicb.2019.00211
CLSI (2019). Performance Standards for Antimicrobial Susceptibility Testing, CLSI Supplement M100, 29th Edn. Wayne, PA: Clinical and Laboratory Standards Institute.
Cosentino, S., Larsen, M. V., Aarestrup, F. M., and Lund, O. (2013). PathogenFinder – distinguishing friend from foe using bacterial whole genome sequence data. PLoS One 8:e77302. doi: 10.1371/journal.pone.0077302
Czobor, I., Novais, A., Chifiriuc, M. C., Mihaescu, G., Lazar, V., and Peixe, L. (2016). Efficient transmission of IncFIIY and IncL plasmids and Klebsiella pneumoniae ST101 clone producing OXA-48, NDM-1 or OXA-181 in Bucharest hospitals. Int. J. Antimicrob. Agents 48, 223–224. doi: 10.1016/j.ijantimicag.2016.05.004
Dortet, L., Flonta, M., Boudehen, Y.-M., Creton, E., Bernabeu, S., Vogel, A., et al. (2015). Dissemination of carbapenemase-producing Enterobacteriaceae and Pseudomonas aeruginosa in Romania. Antimicrob. Agents Chemother. 59, 7100–7103. doi: 10.1128/AAC.01512-15
Ellington, M. J., Kistler, J., Livermore, D. M., and Woodford, N. (2007). Multiplex PCR for rapid detection of genes encoding acquired metallo-β-lactamases. J. Antimicrob. Chemother. 59, 321–322. doi: 10.1093/jac/dkl481
European Centre for Disease Prevention and Control (2020). Available online at: https://www.ecdc.europa.eu/sites/default/files/documents/surveillance-antimicrobial-resistance-Europe-2018.pdf (accessed April 14, 2020).
Feldgarden, M., Brover, V., Haft, D. H., Prasad, A. B., Slotta, D. J., Tolstoy, I., et al. (2019). Validating the AMRFinder tool and resistance gene database by using antimicrobial resistance genotype-phenotype correlations in a collection of isolates. Antimicrob. Agents Chemother. 63:e00483-19. doi: 10.1128/AAC.00483-19
Gardner, S. N., Slezak, T., and Hall, B. G. (2015). kSNP3.0: SNP detection and phylogenetic analysis of genomes without genome alignment or reference genome. Bioinformatics 31, 2877–2878. doi: 10.1093/bioinformatics/btv271
Gomi, R., Matsuda, T., Yamamoto, M., Chou, P.-H., Tanaka, M., Ichiyama, S., et al. (2018). Characteristics of carbapenemase-producing Enterobacteriaceae in wastewater revealed by genomic analysis. Antimicrob. Agents Chemother. 62:e02501-17. doi: 10.1128/AAC.02501-17
Gillings, M. R. (2013). Evolutionary consequences of antibiotic use for the resistome, mobilome, and microbial pangenome. Front. Microbiol. 4:4. doi: 10.3389/fmicb.2013.00004
Grundmann, H., Glasner, C., Albiger, B., Aanensen, D. M., Tomlinson, C. T., Andrasević, A. T., et al. (2017). Occurrence of carbapenemase-producing Klebsiella pneumoniae and Escherichia coli in the European survey of carbapenemase-producing Enterobacteriaceae (EuSCAPE): a prospective, multinational study. Lancet Infect. Dis. 17, 153–163. doi: 10.1016/s1473-3099(16)30257-2
Hocquet, D., Muller, A., and Bertrand, X. (2016). What happens in hospitals does not stay in hospitals: antibiotic-resistant bacteria in hospital wastewater systems. J. Hosp. Infection. 93, 395–402. doi: 10.1016/j.jhin.2016.01.010
Hsieh, P. F., Lin, T. L., Yang, F. L., Wu, M.-C., Pan, Y.-J., Wu, S.-H., et al. (2012). Lipopolysaccharide O1 antigen contributes to the virulence in Klebsiella pneumoniae causing pyogenic liver abscess. PLoS One 7:e33155. doi: 10.1371/journal.pone.0033155
Huang, J.-J., Hu, H.-Y., Tang, F., Li, Y., Lu, S.-Q., and Lu, T. (2011). Inactivation and reactivation of antibiotic-resistant bacteria by chlorination in secondary effluents of a municipal wastewater treatment plant. Water Res. 45, 2775–2781. doi: 10.1016/j.watres.2011.02.026
Hunt, M., Mather, A. E., Sánchez-Busó, L., Page, A. J., Parkhill, J., Keane, J. A., et al. (2017). ARIBA: rapid antimicrobial resistance genotyping directly from sequencing reads. Microb. Genomics 3:e000131. doi: 10.1099/mgen.0.000131
Israil, A., Chifiriuc, C., Palade, G., and Cotar, A. (2013). Clinical and bacteriological aspects of bacterial infections associated to abdominaş surgical emergencies. Bucharest: Ars Docendi Publ. House. 150.
Joensen, K. G., Scheutz, F., Lund, O., Hasman, H., Kaas, R. S., Neilsen, E. M., et al. (2014). Real-time whole-genome sequencing for routine typing, surveillance, and outbreak detection of verotoxigenic Escherichia coli. J. Clin. Micobiol. 52, 1501–1510. doi: 10.1128/JCM.03617-13
Jolley, K. A., and Maiden, M. C. (2010). BIGSdb: scalable analysis of bacterial genome variation at the population level. BMC Bioinformatics 11:595. doi: 10.1186/1471-2105-11-595
Kaur, R., Yadav, B., and Tyagi, R. D. (2020). “Microbiology of hospital wastewater,” in Current Developments in Biotechnology and Bioengineering, eds G. Mannina, A. Pandey, C. Larroche, H. Y. Ng, and H. H. Ngo (Amsterdam: Elsevier), 103–148. doi: 10.1016/B978-0-12-819722-6.00004-3
Khan, F. A., Hellmark, B., Ehricht, R., Söderquist, B., and Jass, J. (2018). Related carbapenemase-producing Klebsiella isolates detected in both a hospital and associated aquatic environment in Sweden. Eur. J. Clin. Microbiol. Infect Dis. 37, 2241–2251. doi: 10.1007/s10096-018-3365-9
Kim, J., and Lee, H.-J. (2000). Rapid discriminatory detection of genes coding for SHV b-lactamases by ligase chain reaction. Antimicrob. Agents Chemother. 44, 1860–1864. doi: 10.1128/aac.44.7.1860-1864.2000
Kim, S., and Aga, D. S. (2007). Potential ecological and human health impacts of antibiotics and antibiotic-resistant bacteria from wastewater treatment plants. J. Toxicol. Environ. Health B Crit. Rev. 10, 559–573. doi: 10.1080/15287390600975137
Kraemer, S. A., Ramachandran, A., and Perron, G. G. (2019). Antibiotic pollution in the environment: from microbial ecology to public policy. Microorganisms 7:180. doi: 10.3390/microorganisms7060180
Kummerer, K., Al-Ahmad, A., and Mersch-Sundermann, V. (2000). Biodegradability of some antibiotics, elimination of the genotoxicity and affection of wastewater bacteria in a simple test. Chemosphere 40, 701–710.
Laffite, A., Kilunga, P. I., Kayembe, J. M., Devarajan, N., Mulaji, C. K., Giuliani, G., et al. (2016). Hospital effluents are one of several sources of metal, antibiotic resistance genes, and bacterial markers disseminated in sub-Saharan urban rivers. Front. Microbiol. 7:1128. doi: 10.3389/fmicb.2016.01128
Lam, M. M. C., Wyres, K. L., Wick, R. R., Judd, L. M., Fostervold, A., Holt, K. E., et al. (2019). Convergence of virulence and MDR in a single plasmid vector in MDR Klebsiella pneumoniae ST15. J. Antimicrob. Chemother. 74, 1218–1222.
Lepuschitz, S., Schill, S., Stoeger, A., Pekard-Amenitsch, S., Hululescu, S., Inreiter, N., et al. (2019). Whole genome sequencing reveals resemblance between ESBL-producing and carbapenem resistant Klebsiella pneumoniae isolates from Austrian rivers and clinical isolates from hospitals. Sci. Total Environ. 662, 227–235. doi: 10.1016/j.scitotenv.2019.01.179
Letunic, I., and Bork, P. (2019). Interactive tree of life (iTOL) v4: recent updates and new development. Nucleic Acids Res. 47, W256–W259. doi: 10.1093/nar/gkz239
Lin, W., Zhang, M., Zhang, S., and Yu, X. (2016). Can chlorination co-select antibiotic-resistance genes? Chemosphere 156, 412–419. doi: 10.1016/j.chemosphere.2016.04.139
Lixandru, B. E., Cotar, A. I., Straut, M., Usein, C. R., Cristea, D., Ciontea, S., et al. (2015). Carbapenemase-producing Klebsiella pneumoniae in Romania: a six-month survey. PLoS One 10:e0143214. doi: 10.1371/journal.pone.0143214
Magiorakos, A. P., Srinivasan, A., Carey, R. B., Carmeli, Y., Falagas, M. E., Giske, C. G., et al. (2012). Multidrug-resistant, extensively drug-resistant and pandrug-resistant bacteria: an international expert proposal for interim standard definitions for acquired resistance. Clin. Microbiol. Infect. 18, 268–281. doi: 10.1111/j.1469-0691.2011.03570.x
Mahon, B. M., Brehony, C., McGrath, E., Killeen, J., Cormican, M., Hickey, P., et al. (2017). Indistinguishable NDM-producing Escherichia coli isolated from recreational waters, sewage, and a clinical specimen in Ireland, 2016 to 2017. Eurosurveillance 22:30513. doi: 10.2807/1560-7917.ES.2017.22.15.30513
Navon-Venezia, S., Kondratyeva, K., and Carattoli, A. (2017). Klebsiella pneumoniae: a major worldwide source and shuttle for antibiotic resistance. FEMS Microbiol. Rev. 41, 252–275. doi: 10.1093/femsre/fux013
Nordmann, P., Poirel, L., Carrer, A., Toleman, M. A., and Walsh, T. R. (2011). How to detect NDM-1 producers. J. Clin. Microbiol. 49, 718–721. doi: 10.1128/JCM.01773-10
Page, A. J., Cummins, C. A., Hunt, M., Wong, V. K., Reuter, S., Holden, M. T. G., et al. (2015). Roary: rapid large-scale prokaryote pan genome analysis. Bioinformatics 31, 3691–3693. doi: 10.1093/bioinformatics/btv421
Poirel, L., Walsh, T. R., Cuvillier, V., and Nordmann, P. (2011). Multiplex PCR for detection of acquired carbapenemase genes. Diagn. Microbiol. Infect. Dis. 70, 119–123. doi: 10.1016/j.diagmicrobio.2010.12.002
Popa, L. I., Barbu, I. C., and Chifiriuc, M. C. (2018). Mobile genetic elements involved in the horizontal transfer of antibiotic resistance genes. Rom. Arch. Microbiol. 77, 263–276.
Popescu, C., Popescu, G. A., Dorobat, O., Rafila, A., Tanase, D., Mikula, C., et al. (2017). OXA-48-carbapenemase-producing Klebsiella pneumoniae infections – the first cases diagnosed in Romanian National Institute of Infectious Disease. Rev. Rom. Med. Lab. 25, 55–61. doi: 10.1515/rrlm-2017-0006
Prado, T., Pereira, W., Silva, D. M., Seki, L. M., Carvalho, A. P. D. ’A., et al. (2008). Detection of extended-spectrum β- lacramase-producing Klebsiella pneumoniae in effluents and sludge of hospital sewage treatment plant. Lett. Appl. Microbiol. 46, 136–141.
Qi, C., Malczynski, M., Parker, M., and Scheetz, M. H. (2008). Characterization of genetic diversity of carbapenem-resistant Acinetobacter baumannii clinical strains collected from 2004 to 2007. J. Clin. Microbiol. 46, 1106–1109. doi: 10.1128/JCM.01877-07
Quinteros, M., Radice, M., Gardella, N., Rodriguez, M. M., Costa, N., Korbenfeld, D., et al. (2003). Extended-spectrum B-lactamases in Enterobacteriaceae in Buenos Aires, Argentina, public hospitals. Antimicrob. Agents Chemother. 47, 2864–2867. doi: 10.1128/AAC.47.9.2864-2867.2003
Rafila, A., Talapan, D., Dorobăţ, O. M., Popescu, G. A., Piţigoi, D., Florea, D., et al. (2015). Emergence of carbapenemase-producing Enterobacteriaceae, a public health threat: a Romanian infectious disease hospital based study. Rev. Rom. Med. Lab. 23, 295–301. doi: 10.1515/rrlm-2015-0024
Rizzo, L., Manaia, C., Merlin, C., Schwartz, T., Dagot, C., Ploy, M. C., et al. (2013). Urban wastewater treatment plants as hotspots for antibiotic resistant bacteria and genes spread into the environment: a review. Sci. Total Environ. 447, 345–360. doi: 10.1016/j.scitotenv.2013.01.032
Roe, C. C., Vazquez, A. J., Esposito, E. P., Zarrilli, R., and Sahl, J. W. (2019). Diversity, virulence, and antimicrobial resistance in isolates from the newly emerging Klebsiella pneumoniae ST101 lineage. Front. Microbiol. 10:542. doi: 10.3389/fmicb.2019.00542
Rozman, U., Duh, D., Cimerman, M., and Turk, S. S. (2020). Hospital wastewater effluent: hot spot for antibiotic resistant bacteria. J. Water Sanit. Hyg. Dev. 10, 171–178. doi: 10.2166/washdev.2020.086
Sanganyado, E., and Gwenzi, W. (2019). Antibiotic resistance in drinking water systems: occurrence, removal, and human health risks. Sci. Total Environ. 669, 785–797. doi: 10.1016/j.scitotenv.2019.03.162
Seemann, T. (2015). Snippy: Fast Bacterial Variant Calling from NGS Reads (v4.6.0). Available online at: https://github.com/tseemann/snippy (accessed May 12, 2020).
Seemann, T. (2018). Shovill: Faster SPAdes Assembly of Illumina Reads (v1.1.0). Available online at: https://github.com/tseemann/shovill (accessed May 12, 2020).
Seemann, T. (2020a). mlst (v2.19.0) Github. Available online at: https://github.com/tseemann/mlst (accessed May 12, 2020).
Seemann, T. (2020b). Abricate, Github (v1.0.1). Available online at: https://github.com/tseemann/abricate (accessed May 12, 2020).
Shi, P., Jia, S., Zhang, X.-X., Zhang, T., Cheng, S., and Li, A. (2013). Metagenomic insights into chlorination effects on microbial antibiotic resistance in drinking water. Water Res. 47, 111–120. doi: 10.1016/j.watres.2012.09.046
Shirani, K., Ataei, B., and Roshandel, F. (2016). Antibiotic resistance pattern and evaluation of metallo-beta lactamase genes (VIM and IMP) in Pseudomonas aeruginosa strains producing MBL enzyme, isolated from patients with secondary immunodeficiency. Adv. Biomed. Res. 5:124. doi: 10.4103/2277-9175.186986
Stamatakis, A. (2014). RAxML version 8: a tool for phylogenetic analysis and post-analysis of large phylogenies. Bioinformatics 30, 1312–1313. doi: 10.1093/bioinformatics/btu033
Surleac, M., Czobor Barbu, I., Paraschiv, S., Popa, L. I., Gheorghe, I., Marutescu, L., et al. (2020). Whole genome sequencing snapshot of multi-drug resistant Klebsiella pneumoniae strains from hospitals and receiving wastewater treatment plants in Southern Romania. PLoS One 15:e0228079. doi: 10.1371/journal.pone.0228079
Suzuki, Y., Nazareno, P. J., Nakano, R., Mondoy, M., Nakano, A., Bugayong, M. P., et al. (2020). Environmental presence and genetic characteristics of carbapenemase-producing Enterobacteriaceae from hospital sewage and river water in the Philippines. Appl. Environ. Microbiol. 86:e01906-19. doi: 10.1128/AEM.01906-19
Székely, E., Damjanova, I., Jánvári, L., Vas, K. E., Molnar, S., Bilca, D. V., et al. (2013). First description of bla(NDM-1), bla(OXA-48), bla(OXA-181) producing Enterobacteriaceae strains in Romania. Int. J. Med. Microbiol. 303, 697–700. doi: 10.1016/j.ijmm.2013.10.001
Vrancianu, C. O., Popa, L. I., Bleotu, C., and Chifiriuc, M. C. (2020). Targeting plasmids to limit acquisition and transmission of antimicrobial resistance. Front. Microbiol. 11:761. doi: 10.3389/fmicb.2020.00761
Wick, R. R., Heinz, E., Holt, K. E., and Wyres, K. L. (2018). Kaptive web: user-friendly capsule and lipopolysaccharide serotype prediction for Klebsiella genomes. J. Clin. Microbiol. 56:e00197-18. doi: 10.1128/JCM.00197-18
WHO, in Chartier, Y., Emmanuel, J., Pieper, U., Prüss, A., Rushbrook, P., Stringer, R., et al. (Eds.) (2014). Safe Management of Wastes From Health-care Activities, 2nd Edn.
Wyres, K. L., and Holt, K. E. (2016). Klebsiella pneumoniae population genomics and antimicrobial-resistant clones. Trends Microbiol. 24, 944–956. doi: 10.1016/j.tim.2016.09.007
Wyres, K. L., Lam, M. M. C., and Holt, K. E. (2020a). Population genomics of Klebsiella pneumoniae. Nat. Rev. Microbiol. 18, 344–359. doi: 10.1038/s41579-019-0315-1
Wyres, K. L., Nguyer, T. N. T., Lam, M. M. C., Judd, L. M., van Winh Chau, N., Dance, D. A. B., et al. (2020b). Genomic surveillance for hypervirulence and multi-drug resistance in invasive Klebsiella pneumoniae from South and Southeast Asia. Genome Med. 12, 11. doi: 10.1186/s13073-019-0706-y
Wyres, K. L., Wick, R. R., Gorrie, C., Jenney, A., Follador, R., Thomson, N. R., et al. (2016). Identification of Klebsiella capsule synthesis loci from whole genome data. Microb. Genom. 2:e000102. doi: 10.1099/mgen.0.000102
Yuan, Q. B., Guo, M. T., and Yang, J. (2015). Fate of antibiotic resistant bacteria and genes during wastewater chlorination: implication for antibiotic resistance control. PLoS One 10:e0119403. doi: 10.1371/journal.pone.0119403
Zankari, E., Hasman, H., Cosentino, S., Vestergaard, M., Rasmyssen, S., Lund, O., et al. (2012). Identification of acquired antimicrobial resistance genes. J. Antimicrob. Chemother. 67, 2640–2644. doi: 10.1093/jac/dks261
Zhang, T., Hu, Y., Jiang, L., Yao, S., Lin, K., Zhou, Y., et al. (2019). Removal of antibiotic resistance genes and control of horizontal transfer risk by UV, chlorination and UV/chlorination treatments of drinking water. Chem. Eng. J. 358, 589–597. doi: 10.1016/j.cej.2018.09.218
Zhang, Y., Zhuang, Y., Geng, J., Ren, H., Zhang, Y., Ding, L., et al. (2015). Inactivation of antibiotic resistance genes in municipal wastewater effluent by chlorination and sequential UV/chlorination disinfection. Sci. Total Environ. 51, 125–132. doi: 10.1016/j.scitotenv.2015.01.028
Zhang, Z., Li, B., Li, N., Sardar, M. F., Song, T., Zhu, C., et al. (2019). Effects of UV disinfection on phenotypes and genotypes of antibiotic-resistant bacteria in secondary effluent from a municipal wastewater treatment plant. Water Res. 157, 545–554. doi: 10.1016/j.watres.2019.03.079
Keywords: wastewater treatment plant, MDR Klebsiella pneumoniae, whole-genome sequencing, hospital wastewater chlorine treatment, hospital sewage
Citation: Popa LI, Gheorghe I, Barbu IC, Surleac M, Paraschiv S, Măruţescu L, Popa M, Pîrcălăbioru GG, Talapan D, Niţă M, Streinu-Cercel A, Streinu-Cercel A, Oţelea D and Chifiriuc MC (2021) Multidrug Resistant Klebsiella pneumoniae ST101 Clone Survival Chain From Inpatients to Hospital Effluent After Chlorine Treatment. Front. Microbiol. 11:610296. doi: 10.3389/fmicb.2020.610296
Received: 25 September 2020; Accepted: 09 December 2020;
Published: 11 January 2021.
Edited by:
William Calero-Cáceres, Technical University of Ambato, EcuadorReviewed by:
Laura Sala-Comorera, University College Dublin, IrelandCopyright © 2021 Popa, Gheorghe, Barbu, Surleac, Paraschiv, Măruţescu, Popa, Pîrcălăbioru, Talapan, Niţă, Streinu-Cercel, Streinu-Cercel, Oţelea and Chifiriuc. This is an open-access article distributed under the terms of the Creative Commons Attribution License (CC BY). The use, distribution or reproduction in other forums is permitted, provided the original author(s) and the copyright owner(s) are credited and that the original publication in this journal is cited, in accordance with accepted academic practice. No use, distribution or reproduction is permitted which does not comply with these terms.
*Correspondence: Ilda Czobor Barbu, aWxkYS5iYXJidUBiaW8udW5pYnVjLnJv
†These authors have contributed equally to this work
Disclaimer: All claims expressed in this article are solely those of the authors and do not necessarily represent those of their affiliated organizations, or those of the publisher, the editors and the reviewers. Any product that may be evaluated in this article or claim that may be made by its manufacturer is not guaranteed or endorsed by the publisher.
Research integrity at Frontiers
Learn more about the work of our research integrity team to safeguard the quality of each article we publish.