- Department of Agricultural, Food and Nutritional Science, University of Alberta, Edmonton, AB, Canada
Applying probiotics to improve gut health and growth performance of pigs is considered an effective approach to reduce use of antimicrobial growth promoters in swine production. Understanding the properties of these probiotics is a prerequisite for the selection of probiotic strains for pigs. Host-adapted probiotic strains were suggested to exert probiotic effects by different mechanisms when compared to free-living or nomadic probiotic strains. This study assessed the effect of probiotic intervention with Limosilactobacillus reuteri TMW1.656, a host-adapted species producing the antimicrobial compound reutericyclin, its isogenic and reutericyclin-negative L. reuteri TMW1.656ΔrtcN, and with Limosilactobacillus fermentum and Lacticaseibacillus casei, two species with a nomadic lifestyle. Probiotic strains were supplemented to the post weaning diet in piglets by fermented feed or as freeze-dried cultures. The composition of fecal microbiota was determined by high throughput sequencing of 16S rRNA gene sequence tags; Enterotoxigenic Escherichia coli and Clostridium perfringens were quantified by qPCR targeting specific virulence factors. Inclusion of host-adapted L. reuteri effectively reduced ETEC abundance in swine intestine. In contrast, nomadic L. fermentum and L. casei did not show inhibitory effects on ETEC but reduced the abundance of Clostridium spp. In addition, the increasing abundance of Bacteriodetes after weaning was correlated to a reduction of ETEC abundance. Remarkably, the early colonization of piglets with ETEC was impacted by maternal-neonatal transmission; the pattern of virulence factors changed significantly over time after weaning. Probiotic intervention or the production of reutericyclin showed limited effect on the overall composition of commensal gut microbiota. In conclusion, the present study provided evidence that the lifestyle of lactobacilli is a relevant criterion for selection of probiotic cultures while the production of antimicrobial compounds has only minor effects.
Introduction
The stress triggered by weaning and the immature immune system contributes to the susceptibility of weanling pig to gut microbial dysbiosis and pathogen infection (Katsuda et al., 2006; Gresse et al., 2017). Clostridium perfingens Type C infections are common in neonatal piglets within 7 days of farrowing; infections with enterotoxigenic Escherichia coli (ETEC) are the most significant cause of post-weaning diarrhea (Kyriakis et al., 1996; Fairbrother et al., 2005). Vaccination against ETEC infections provides only limited protection because ETEC are non-invasive pathogens that reside in the intestinal lumen (Felder et al., 2001; Katrien et al., 2010). Alternatives for protection against pathogen infection are required particularly for early weaned pigs after the interrupted protection from maternal immunity. Probiotic bacteria are one of those alternatives and the primary trait required for the application of probiotics in weanling pigs is the ability to inhibit colonization of pathogen.
Probiotic bacteria have been shown to reduce the pathogen load, particularly Salmonella enterica and ETEC, in suckling and weaned piglets (Liao and Nyachoti, 2017; Wang and Gänzle, 2019). Bacillus spp. are most widely used as probiotics in swine production because their endospores remain viable throughout feed processing and storage at ambient temperature (Liao and Nyachoti, 2017; Ritter et al., 2018). Lactic acid bacteria and particularly lactobacilli have been used experimentally to reduce the pathogen load in suckling and weaned piglets (Liao and Nyachoti, 2017). Lactobacilli are freeze-dried to maintain their viability and functionality for use as probiotics (Iaconelli et al., 2015). In animal production, feed fermentation with lactic acid bacteria is also used; feed fermentation with probiotic lactic acid bacteria not only serves as delivery system for viable probiotic bacteria but also improves the availability of nutrients (Marko et al., 2014; Yang et al., 2015a). Probiotic effects of strains that were administered to swine by these two methods of delivery methods, however, have not been compared directly.
Lactobacilli include three genera that are adapted to vertebrate hosts, i.e., Lactobacillus, Ligilactobacillus, and Limosilactobacillus species (Duar et al., 2017b; Zheng et al., 2020); other lactobacilli, however, are ecologically diverse and are equipped for temporary persistence in multiple habitats including environmental niches and the intestinal tract of vertebrates (Duar et al., 2017b; Zheng et al., 2020). Host-adapted lactobacilli that are stable members of the intestinal microbiota of swine include Limosilactobacillus reuteri (previously Lactobacillus reuteri, Zheng et al., 2020) and Lactobacillus amylovorus (Leser et al., 2002; Duar et al., 2017a); nomadic organisms with documented use as probiotics in swine include Lactiplantibacillus plantarum, Limosilactobacillus fermentum, and Lacticaseibacillus rhamnosus (previously all assigned to the genus Lactobacillus, Wang and Gänzle, 2019; Zheng et al., 2020). Recent studies suggest that host-adapted and nomadic probiotics differ with respect to their survival in the intestinal tract, and with respect to mechanisms of probiotic activity (Maldonado-Gómez et al., 2016; Walter et al., 2018; Zhao et al., 2018; Wang and Gänzle, 2019). Probiotic feeding of the host adapted L. reuteri TMW1.656 resulted in significantly higher cell counts of the probiotic strain during intestinal transit when compared to the nomadic organisms L. fermentum K9-2 and Lacticaseibacillus casei K9-1 that were provided at the same viable cell counts (Zhao et al., 2018), suggesting improved survival. Vertebrate host adapted lactobacilli appear to induce immune tolerance (Lin et al., 2008; Livingston et al., 2010). In contrast, probiotic strains that are allochthonous to pigs are more likely to stimulate a pro-inflammatory immune response against pathogen infection (Wang et al., 2009; Li et al., 2012; Scharek-Tedin et al., 2013; Zhou et al., 2015; Upadhaya et al., 2017). A differential interaction of host-adapted and nomadic lactobacilli with the immune system, however, has not been sufficiently documented.
This study aimed to test the hypothesis that host-adapted and nomadic lactobacilli have different effects on pathogen load and gut microbiota in weanling pigs. Experiments employed commercially available strains of the nomadic species L. fermentum and L. casei, and the host adapted L. reuteri. L. reuteri TMW 1.656, which was previously shown to reduce the pathogen load in weanling pigs. Moreover, its ability to produce reutericyclin was suggested to modulate the development of fecal microbiota of weanling pigs (Yang et al., 2015b). The present experiment was designed to (1) verify a contribution of reutericyclin formation in probiotic function of L. reuteri; (2) compare the effects of freeze-drying and feed fermentation on probiotic effects of L. fermentum and L. casei, and (3) compare the beneficial effects of lactobacilli with different ecological origins in weanling pigs.
Materials and Methods
Microorganisms and Growth Conditions
Reutericyclin-producing L. reuteri TMW1.656 and isogenic reutericyclin-negative L. reuteri TMW1.656ΔrtcN (Lin et al., 2015) were routinely grown on anaerobically on modified MRS agar (Meroth et al., 2003) at 37°C. Two commercial probiotics L. casei K9-1 and L. fermentum K9-2 were provided by CanBiocin Inc. (Edmonton, AB, Canada) and grown under same conditions. Freeze-dried cultures of L. casei K9-1 and L. fermentum K9-2 with 109 CFU/g vegetative cell were also provided by CanBiocin Inc. (Edmonton, AB, Canada), which were stored at 4°C until use.
Experimental Diets and Animals
Overnight cultures of L. reuteri TMW1.656, L. reuteri TMW1.656ΔrtcN, L. casei K9-1, and L. fermentum K9-2 were applied for feed fermentation; a detailed characterization of the feed fermentation and the microbiological characteristics of the feed is provided by Zhao et al. (2018). Significant components of the dietary treatments are listed in Table 1. The fermentation was monitored by enumeration of viable cell counts, by measurement of the pH, and by quantification of the probiotic strains by strain-specific quantitative PCR (Zhao et al., 2018). Wheat was fermented with L. reuteri TMW1.656, L. reuteri TMW1.656ΔrtcN, or with L. casei and L. fermentum; the basal diet (98%) (Supplementary Table S1) was mixed with 2% fermented wheat. Control treatments included addition of unfermented, wheat that was incubated after chemical acidification, and unfermented wheat containing freeze dried cells of L. casei and L. fermentum. After addition of freeze-dried bacterial cells, the cell counts of L. casei and L. fermentum matched the cell counts of the same strains in fermented feed. The cell counts of L. reuteri TMW1.656, L. reuteri TMW1.656ΔrtcN, and L. casei/L. fermentum (freeze-fried and fermentation) in feed ranged from 7.7 ± 0.4 to 8.4 ± 0.5 log (cfu/g) (Zhao et al., 2018).
The feeding trial was approved by the University of Alberta Animal Care and Use Committee for Livestock (Protocol # AUP00000182). A total of 48 male piglets (Duroc × Large White/Landrace F1) with similar bodyweight (6–7 kg) were selected at weaning (21 days of age). Six experimental diets were allocated to 48 piglets with a completely randomized block design, which provided 8 replicates per dietary treatment. All pigs were raised as previous described (Zhao et al., 2018) and weighted at the end of each week. Diets were provided twice per day with equal amounts. The content of dry matter (DM) in feed and leftover were measured each day to calculate the feed intake. The feed efficiency was calculated from average weight gain (g/day) over average feed intake (g DM/day).
Samples Preparation and DNA Extraction
Feces were collected from the pen of each animal at days 0, 7, 14, and 21 and stored at −20°C immediately after sampling. Digesta samples were collected from ileum caecum and colon at euthanasia and stored at −20°C. Total bacterial DNA was extracted from 2 to 3 g of feces or digesta samples after thawing and mixing the frozen samples. Stool samples were homogenized with phosphate buffered saline (pH 7.4, Gibco, Thermo Fisher Scientific, Burlington, United States) and pre-treated by bead-beating (BioSpec Products, Inc., Bartlesville, United States) for 30 s × 8 times. Treated samples were heated at 95°C for 15 min to lyse cell before using QIAamp Fast DNA stool mini kit (Qiagen, Inc., Valencia, CA, United States). Only DNA samples with an A260/280 ratio higher than 1.8 were used for pathogen detection and microbial analysis. Purified DNA was diluted to 50 ng/μL for use.
Detection of Intestinal Pathogens Using qPCR and HRM-qPCR
The detection of intestinal pathogens included species specific primers to enumerate E. coli, and primers targeting genes coding for fimbriae of swine-associated ETEC (F6, F18, F41, K88, and K99) as well as genes coding for toxins produced by ETEC (LT, Sta, and STb). The abundance of clostridial pathogens was determined by primers targeting the Clostridium cluster I and the gene coding for the α-toxin of C. perfringens. PCR was performed by multiplex HRM-qPCR and/or qPCR in 144 digesta and 191 fecal samples. Primers used in this study and their target genes are listed in Table 2. Genes coding for ETEC fimbriae genes were quantified by multiplex HRM-qPCR using Rotor-Gene Q (QIAGEN) HRM-thermo cycler and Type-it HRM Kit (QIAGEN) as described (Wang et al., 2017). A total volume of 25 μL HRM-qPCR reactions contained 12.5 μL 2 × HRM Master Mix, 3 μL template bacterial DNA, 200 nM × 5 targets primers. A standard curve for total content of five targets was established from 10-fold dilutions of the mixed standards, containing 2 × 102 to 2 × 10–8 gene copies/μL of each purified positive control, which were amplified from stool samples with same conditions. The relative abundance of each target was quantified based on the linear correlation between the percentage of melting peak area and relative portion of the respective amplicon in mixed template (Wang et al., 2017). Total E. coli, ETEC toxin gens LT, Sta, and STb, Clostridium cluster I and C. perfringens were determined by qPCR using 7500 Fast real-time PCR system (Thermo Fisher Scientific, Burlington, United States). qPCR conditions were as previous described (Yang et al., 2015b; Wang et al., 2017).
Fecal Microbiological Analysis Using 16S rRNA Gene Sequencing
A total of 191 samples of fecal community DNA was sequenced on pair end Illumina MiSeq platform (2 × 300 bp) by amplifying the V5-V6 domain of the 16S rRNA gene (University of Minnesota Genomics Center, Minneapolis, MN, United States). The forward and reverse primers sequences used for amplification were GTGCCAGCMGCCGCGGTAA and CGACRRCCATGCANCACCT, respectively. An average 17,440 paired 16S rRNA gene sequences per sample with an average length of 280 bp were retained after quality control and denoising from QIIME2 pipeline (QIIME2-2020.2) (Bolyen et al., 2019) by using “DADA2” (Callahan et al., 2016) with parameters: –p-trunc-len-f 277 –p-trunc-len-r 230.
Only sequence variants with relative abundance > 0.005% were retained for downstream analysis. Taxonomy was assigned to representative sequences by aligning to Silva release 132 classifier. Features classified as the genus Lactobacillus in QIIME2 were additionally blasted against the Genome Taxonomy Database release 95 (GTDB1) to reflect the current taxonomy of Lactobacillaceae (Zheng et al., 2020). Principle coordinates analysis (PCoA) and analysis of similarities (ANOSIM) were performed to explore microbial community differences through weighted UniFrac distance matrix. The sequence data are deposited at the National Center for Biotechnology Information (NCBI) under BioProject PRJNA665253.
Statistical Analysis
The data for growth performance (average feed intake, average daily gain, and feed efficiency), intestinal pathogen load of Clostridia Cluster I, C. perfringens α toxin, E. coli and ETEC virulence factors were analyzed by linear mixed-effects model fitted for a completely randomized block design in R (version 5.12.6, The R Foundation for Statistical Computing, 2020). In this model, pig was considered the experimental unit; time, dietary treatments, and time × dietary treatments were calculated as fixed factors; the difference among blocks were regarded as random effects. The normality and homogeneity of all variables were determined by Shapiro-Wilk normality test and Bartlett test, respectively. The date for relative abundance of sequences variants and alpha diversity were analyzed by Kruskal-Wallis rank-sum test in R. The pairwise comparisons were performed with Wilcoxon rank sum test. Analysis of similarities (ANOSIM) for ETEC virulence factors data was calculated by Bray–Curtis dissimilarity; principle component analysis (PCA) for ETEC virulence factors data was analyzed using “kassambara/factoextra” packages in R. ANOSIM and Principle coordinate analysis (PCoA) for 16S rRNA sequence data was calculated from weighted UniFrac distance matrix. Results were presented as means ± standard deviation. P < 0.05 with Bonferroni-adjustment were considered significant.
Results
Animal Health and Growth Performance of Pigs
All pigs remained healthy and none of the pigs exhibited persistent diarrhea during the 3 weeks trial. The parameters reflecting growth performance are listed in Supplementary Table S1. The average feed intake and daily gain increased with time corresponding to feed efficiency increased from 0.65 to 0.8 kg during the first 2 weeks and remained stable in the third week (data not shown). No significant difference in feed efficiency was detected among dietary treatments or factors.
Reduction of Pathogens and Toxins in Weanling Pigs by Feeding Probiotic Lactobacilli
This study employed healthy animals that were housed under conditions that do not result in diarrhea; therefore, the abundance of genes coding for virulence factors was used as an indication of the ability of probiotics to prevent intestinal infections. The pathogen load in weanling pigs was determined by qPCR and HRM-qPCR. Primers targeted Clostridium Cluster I and the C. perfringens α-toxin and ETEC virulence factors including the heat labile toxin (LT), the heat stable toxin a (STa), the heat stable toxin b (STb), and K99, F18, F41, K88, and F6 fimbriae in weanling pigs. Fecal samples were obtained weekly and ileal, cecal, and colonic digesta were collected at day 21. The overall abundance of these pathogens and virulence factors were lower in the ileum than in the caecum and colon (Figure 1 and Supplementary Table S2) and decreased over time (Supplementary Table S3). The gene content of Clostridium Cluster I in digesta samples did not differ (P > 0.05) across dietary treatments (Figure 1 and Supplementary Table S2). Feeding freeze-dried L. casei/L. fermentum or L. reuteri TMW1.656ΔrtcN reduced (P < 0.05) Clostridium Cluster I in feces at day 21. Feeding L. reuteri TMW1.656 or L. reuteri TMW1.656ΔrtcN also decreased (P < 0.05) C. perfringens α toxin in the caecum and colon (Figure 1B and Supplementary Table S2).
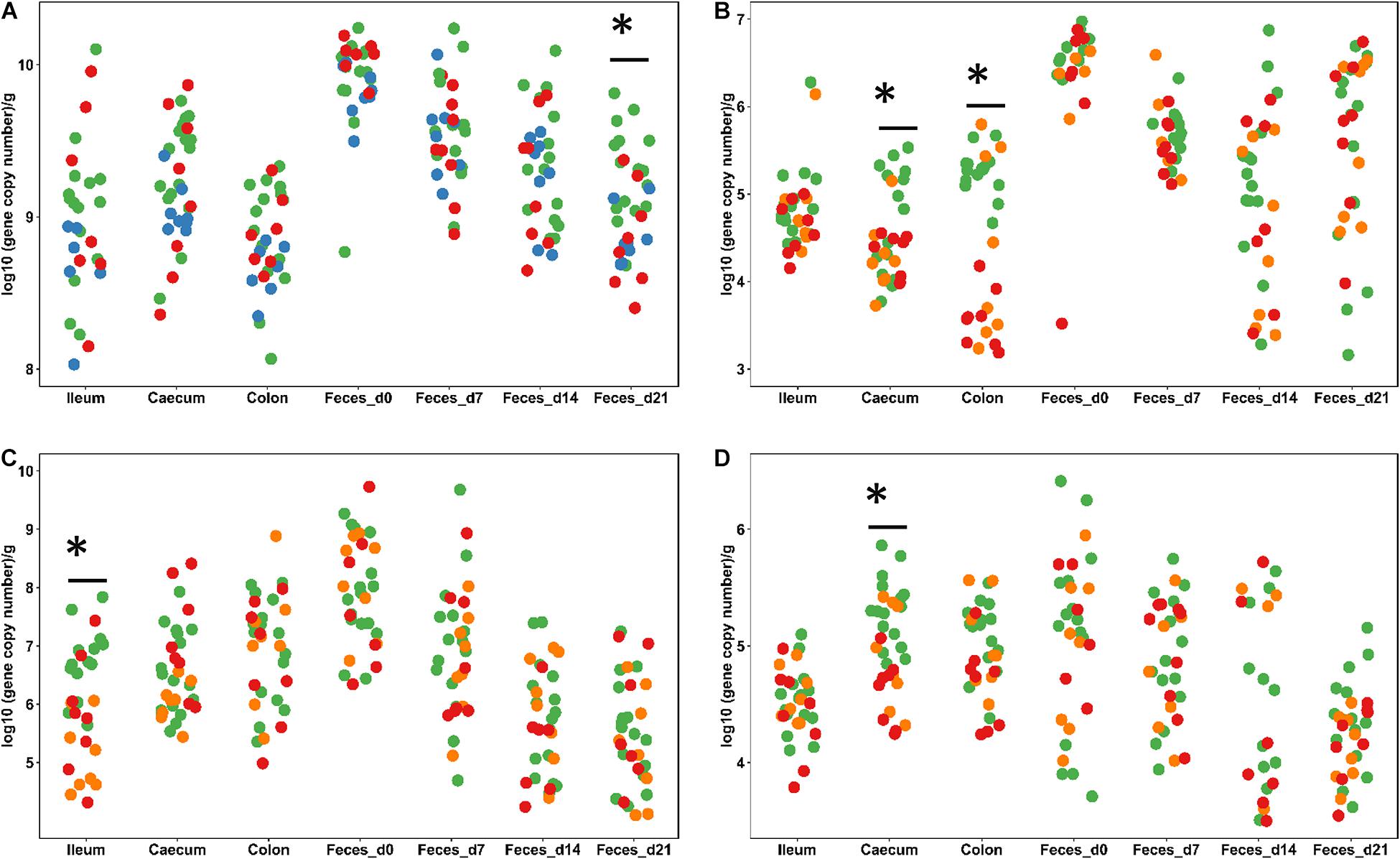
Figure 1. Abundance of Clostridium cluster I (A), C. perfringens α-toxin (B), E. coli (C) and the heat-labile toxin of ETEC (D) in weaned piglets. Digesta samples were collected from ileum, caecum, and colon at day 21. Feces was collected at day 0, 7, 14, and 21. All the specimens were analyzed by qPCR with specific primers. Each dot represents individual samples; samples are colored according to the dietary treatments. Only treatments with significant difference compared to the control are shown: Control and Acidified control (green, n = 16), freeze-dried L. casei/L. fermentum (blue, n = 8), fermented L. casei/L. fermentum (purple, n = 8), L. reuteri TMW1.656 (orange, n = 8) and L. reuteri TMW1.656ΔrtcN (red, n = 8). Data with asterisk (∗) are significantly different (P < 0.05) between dietary groups.
Dietary intervention with probiotic lactobacilli had little influence on the abundance of E. coli and ETEC virulence factors. The abundance of E. coli decreased over time (Figure 1 and Supplementary Table S3); in ileal samples, the abundance of E. coli was reduced (P < 0.05) in samples from pigs fed with L. reuteri TMW1.656 or TMW1.656ΔrtcN (Figure 1C). The gene copy numbers of LT were also reduced (P < 0.05) in cecal samples from pigs fed with L. reuteri TMW1.656 or TMW1.656ΔrtcN (Figure 1C). The content of other ETEC virulence factors did not differ among diet groups but varied with aging.
Longitudinal Changes of ETEC Virulence Factors in Swine Feces During the First 21 Days After Weaning
The longitudinal profile of ETEC virulence factors throughout the 3 weeks after weaning is shown in Figure 2. The time after weaning strongly affected the abundance of E. coli and ETEC virulence factors. The load of these virulence factors was low at weaning and then displayed a chaotic profile during the first week followed by a steadily decrease during the following 2 weeks (Figure 2). LT decreased (P < 0.05) after the first week while STa were higher at day 7 (P < 0.05). F18 and F6 fimbriae increased (P < 0.05) and were the predominant ETEC fimbriae detected 7 days after weaning. Other fimbriae types and the STb toxin were detected in feces with low abundance (< 105 copies/g) (Figure 2 and Supplementary Table S3); values were not different between dietary treatments or different time points.
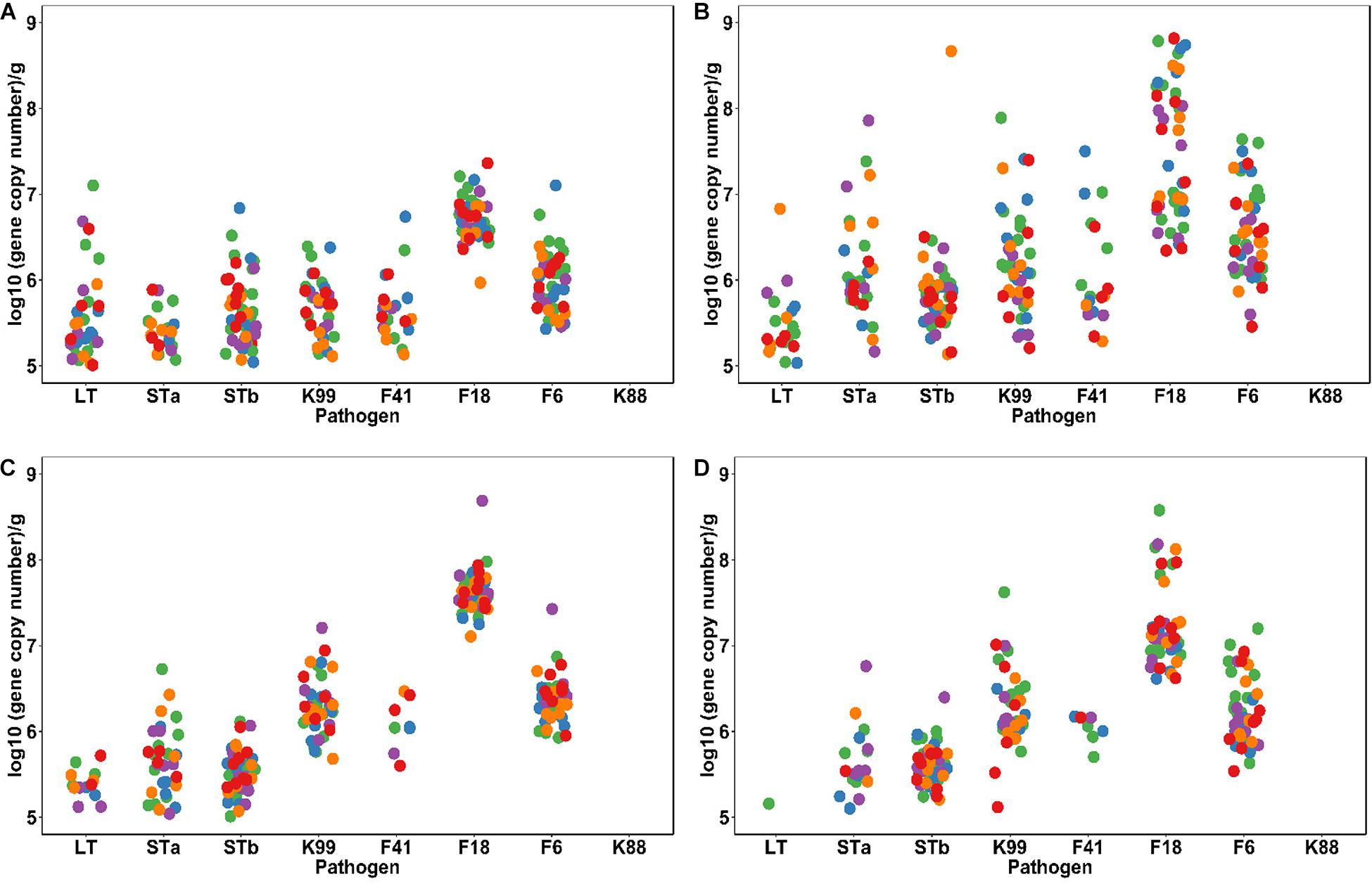
Figure 2. Dynamic profiles of ETEC virulence factors in fecal samples taken at weaning (A), and 7 days (B), 14 days (C), and 21 days (D) after weaning. All the samples were analyzed by qPCR with specific primers. Each dot represents individual fecal samples, colored according to the dietary treatments: Control and Acidified control (green, n = 16), freeze-dried L. casei/L. fermentum (blue, n = 8), fermented L. casei/L. fermentum (purple, n = 8), L. reuteri TMW1.656 (orange, n = 8) and L. reuteri TMW1.656 ΔrtcN (red, n = 8). Different diets did not influence (P > 0.05) the abundance of E. coli virulence factors.
Litter effects on the presence of virulence factors were evaluated by PCA (Figure 3) and by ANOSIM (Table 3). Seven days after weaning, PCA analysis of the abundance of ETEC virulence factors clustered pigs from different litters separately, this separation was not apparent 14 or 21 days after weaning (Figure 3). ANOSIM confirmed that sow or litter effects influenced (P < 0.05) the variations in ETEC virulence factors 7 days post-weaning but not at other time points; overall, the time after weaning significantly contributed to the variations through the experimental period (Table 3).
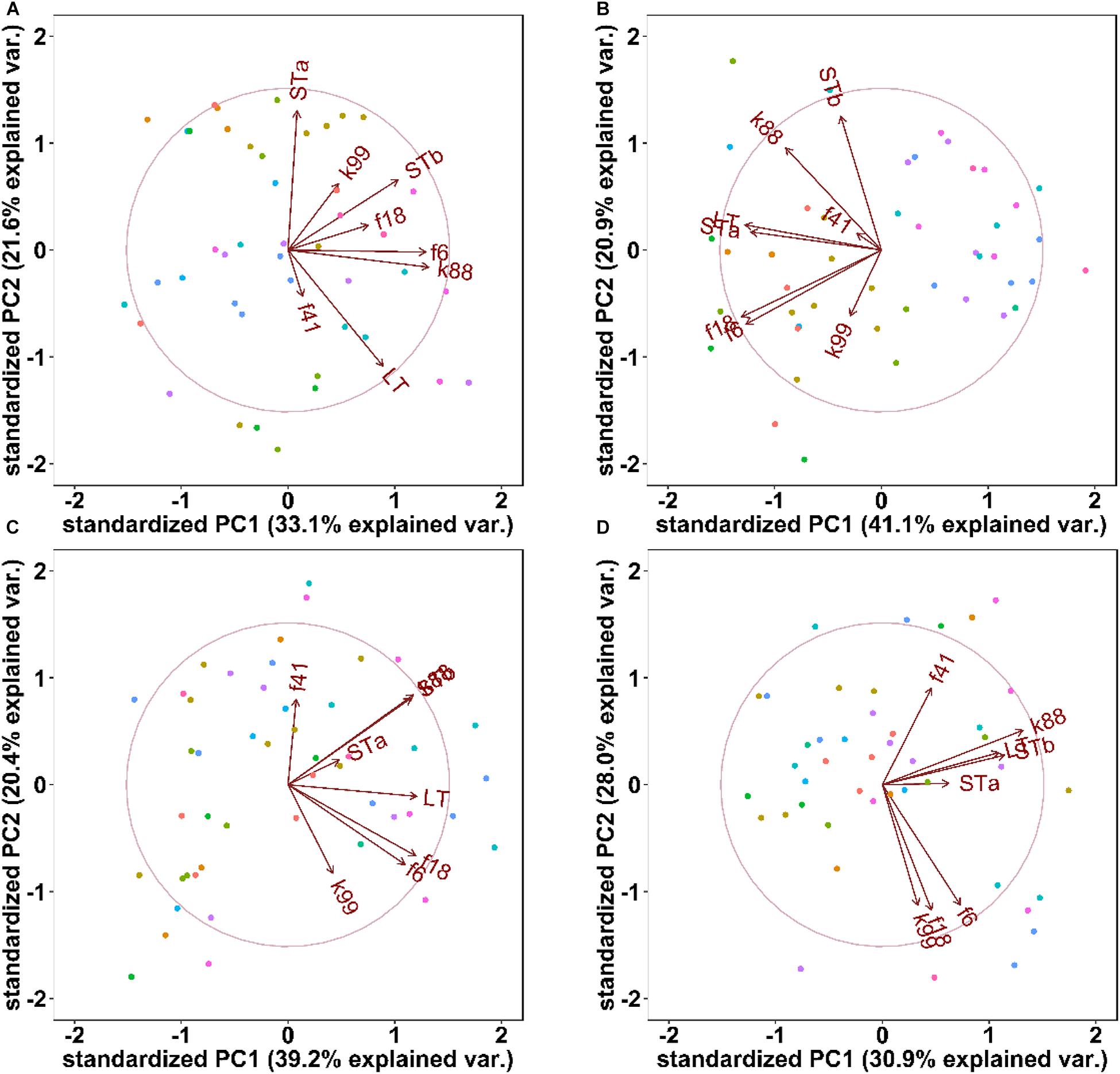
Figure 3. Principle component analysis of ETEC virulence factors at 0 days (Panel A), 7 days (B), 14 days (C), and 21 days (D) days after weaning. Each dot represents individual fecal samples, colored by sow: dots with same color are born from the same sow. The arrows represent the original variables, where the corresponding directions represent the correlations between the original variables and the principal components, and the length represents the contribution of the original data to the principal component.
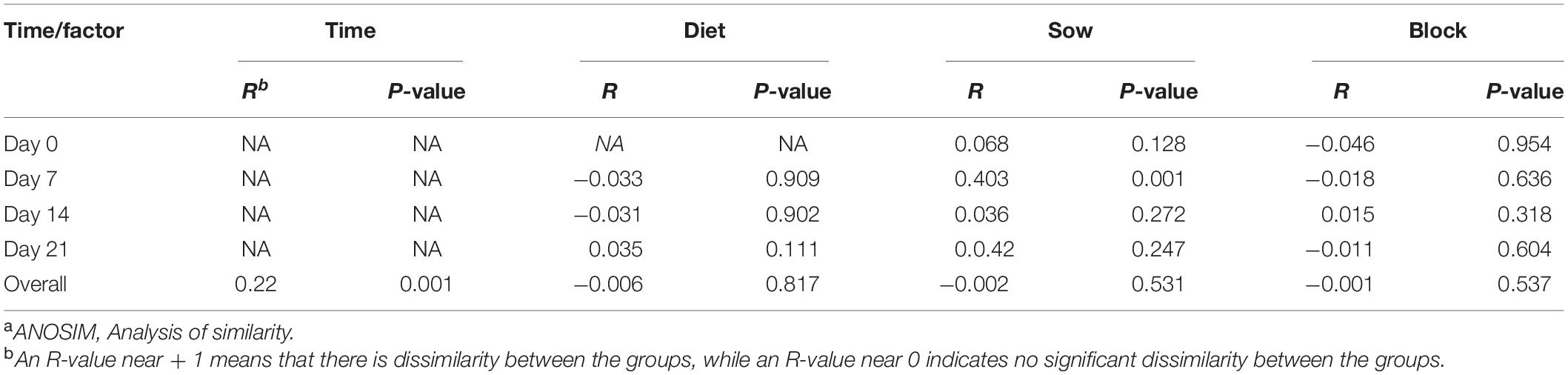
Table 3. ANOSIMa of Bray–Curtis dissimilarity calculated with ETEC virulence factor genes content in feces at 0, 7, 14, and 21 days after weaning.
Composition of the Community of Lactobacilli and of the Overall Intestinal Microbiota After Feeding Lactobacilli to Weanling Pigs
To determine the effects of probiotic lactobacilli on commensal microbes α-diversity (Figure 4) and β-diversity (Table 4 and Supplementary Figure S1) of fecal microbiota and Lactobacillaceae were assessed on the basis of 16S rRNA gene sequence data obtained with fecal samples from weanling pigs. The overall number of bacterial types in fecal microbiota significantly increased in the first 2 weeks and plateaued in the last week (Figure 4A); this was also reflected bacterial by an increased α-diversity as measured by the Shannon index (Figure 4C). Pigs feed with acidified wheat were noted for the reduction of α-diversity (Figure 4C, P < 0.05). The number of OTUs attributed to Lactobacillaceae remained overall stable while the diversity of the Lactobacillaceae community showed a transient decrease during the second week after weaning (Figure 4D). Dietary intervention with probiotic lactobacilli did not impact the diversity of the Lactobacillaceae community; in pigs fed with acidified wheat, the observed OTU types identified as intestinal lactobacilli was transiently reduced 7 days after weaning (P < 0.05; Figures 4B,D).
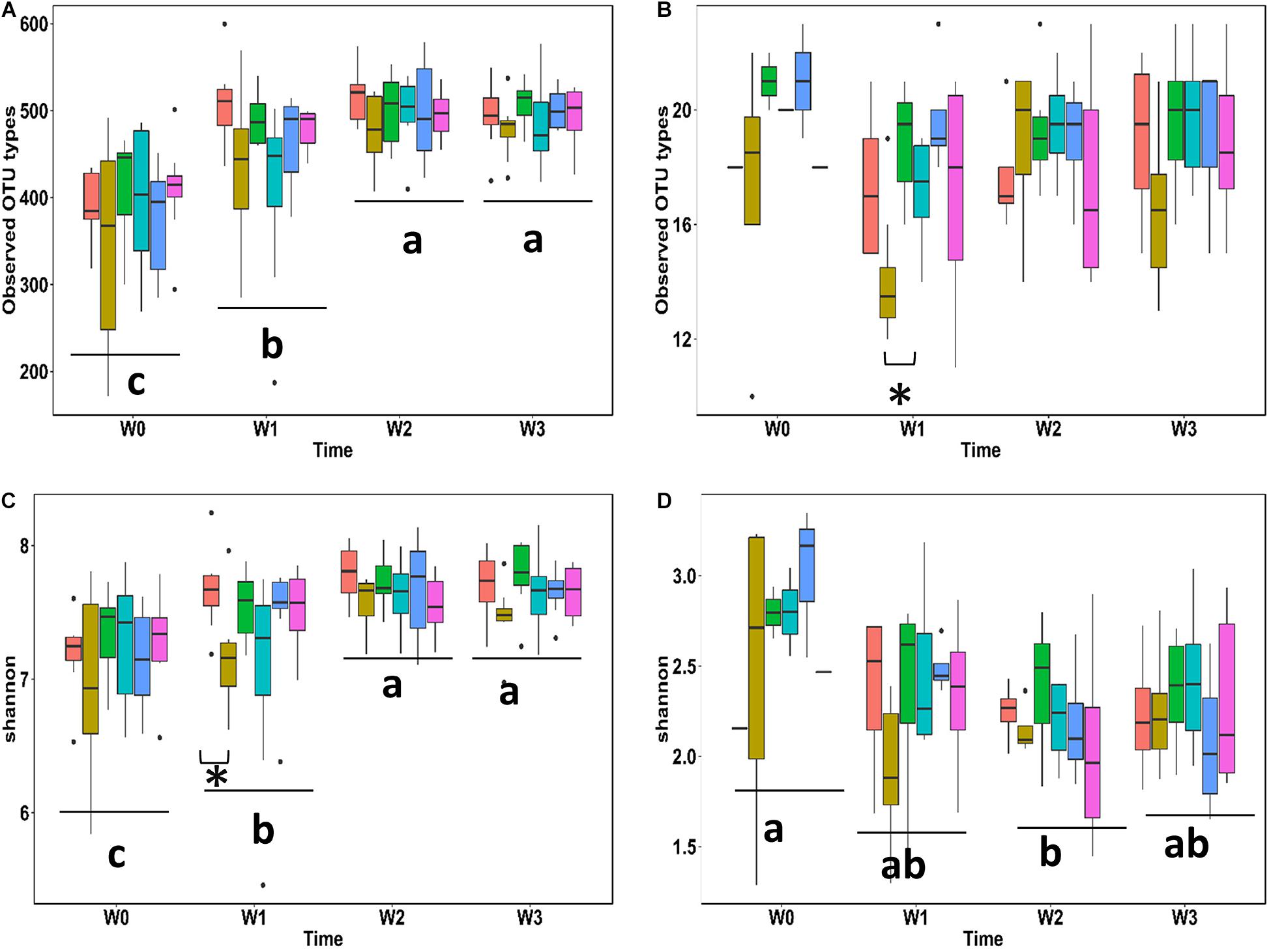
Figure 4. Fecal microbiota and diversity of lactobacilli during the first 3 weeks after weaning. Total number of bacteria (A) or lactobacilli (B) types observed and Shannon diversity index of fecal microbiota (C) or the community of lactobacilli (D) were analyzed based on partial 16S rRNA sequences. Each box represents one dietary treatment colored as following: Control (light red, n = 8), Acidified control (brown, n = 16), freeze-dried L. casei/L. fermentum (green, n = 8), fermented L. casei/L. fermentum (cyan, n = 8), L. reuteri TMW1.656 (light blue, n = 8) and L. reuteri TMW1.656 ΔrtcN (plum, n = 8). Indexes with different letters are significantly different (P < 0.05) between time points.
The dissimilarities between pigs fed with different lactobacilli were further assessed by analysis of β-diversity (Table 4 and Supplementary Figure S1). The overall fecal microbiota of weanling pigs was differentiated by the week of feeding and litters (P < 0.05, Table 4). Lactobacilli were also altered (P < 0.05) by the feed phase but not influenced by litter effects (P = 0.166). The inclusion of probiotic lactobacilli in feed did not change the structure of fecal microbiota (P = 0.683) but contributed to the difference of lactobacilli (P < 0.05). This influence was also reflected in an effect (P < 0.05) of dietary intervention on the composition of Lactobacillaceae communities.
Contribution of Feeding Lactobacilli in Restoring Fecal Microbiota From Weaning Dysbiosis
The relative abundance of major bacterial taxa was compared to determine the effects of probiotic lactobacilli on the evolution of intestinal microbiota after weaning (Table 5 and Figure 5). Increased abundance was mainly detected in genus Prevotella and family Prevotellaceae from the phyla Bacteroidetes, genus Lactobacillus, Limosilactobacillus, Agathobacter, Terrisporobacter, and family Ruminococcaceae from the phyla Firmicutes. Reductions were mainly observed in Bacteroidetes classified of the genera Bacteroides and Parabacteroides, Firmicutes classified as Christensenellaceae, Ruminococcaceae, Erysipelotrichaceae, Clostridiales, Lachnoclostridium and most genera of the phylum Proteobacteria. Compared to control or acidified control diet, feeding lactobacilli did not change the relative abundance of fecal microbiota after weaning. Differences were only detected between pigs feeding with different lactobacilli. Feeding with L. reuteri TMW1.656 significantly increased relative abundance of Clostridium sensu strictu (P < 0.05) at day 0 compared to freeze-dried L. fermentum and L. casei but decreased Limosilactobacillus (previously known as L. reuteri group) compared to L. fermentum and L. casei at day 21.
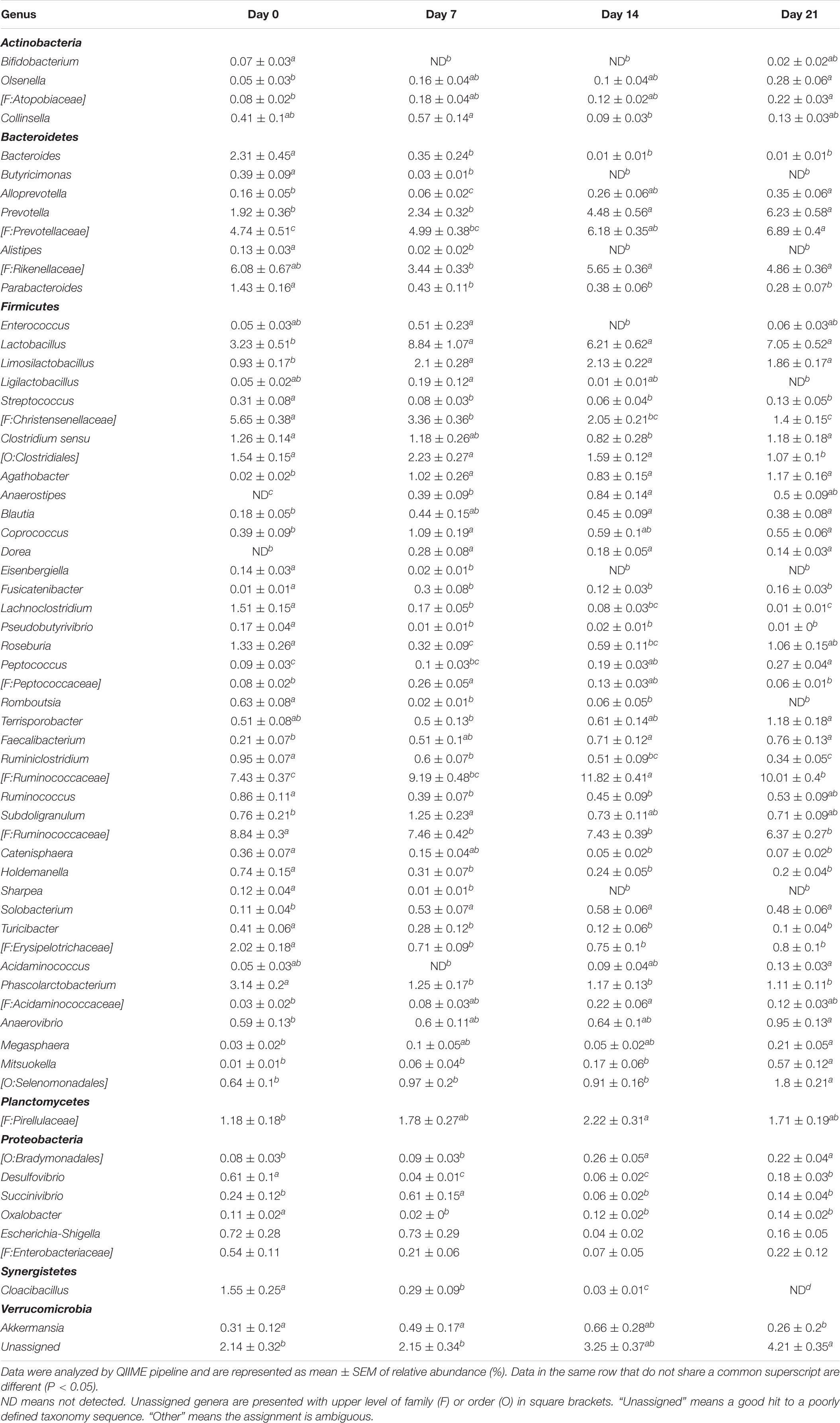
Table 5. Bacterial genera with significant difference in fecal microbiota of pigs during the first 3 weeks after weaning, determined by sequencing of 16S rRNA tags.
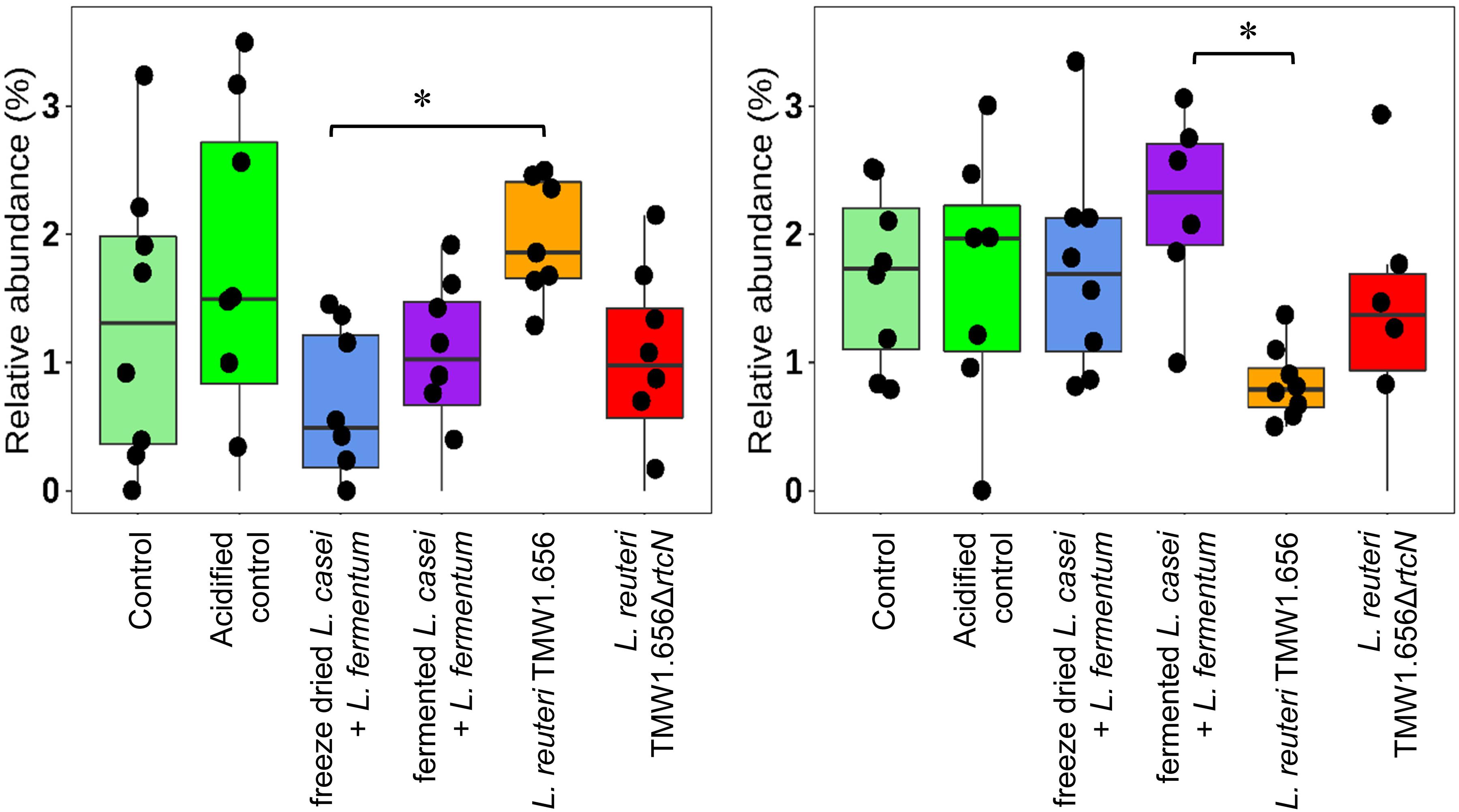
Figure 5. Relative abundance (%) of Clostridium sensu strictu (left) and Limosilactobacillus (right) in fecal microbiota of the pigs feed with experimental diets at 0 and 21 days after weaning, respectively. Data were calculated from sequencing of 16S rRNA tags using DADA2 workflow. Experimental diets were distinguished by color codes as shown: Control (light green, n = 8), acidified control (green, n = 8), freeze-dried L. casei/L. fermentum (blue, n = 8), fermented L. casei/L. fermentum (purple, n = 8), L. reuteri TMW1.656 (orange, n = 8) and L. reuteri TMW1.656ΔrtcN (red, n = 8). Data with asterisk (*) are significantly different (P < 0.05) between dietary groups.
Discussion
Probiotic Activity of Host Adapted and Nomadic Lactobacilli
Immunomodulation and competitive colonization are among the major mechanisms of probiotics to inhibit pathogen infection (Hill et al., 2014). The ecological origin of the probiotic strain was suggested to determine whether one or the other is more important (Wang et al., 2009; Scharek-Tedin et al., 2013; Sonia et al., 2014; Zheng et al., 2016; Upadhaya et al., 2017). This study observed a reduction of E. coli and ETEC toxins mainly in pigs supplied with host-adapted L. reuteri. Anti-ETEC effects were also reported in studies of applying host-adapted L. amylovorus (previously L. sobrius) and L. reuteri (Kim et al., 2007; Yang et al., 2015a; Roselli et al., 2018). In contrast, the nomadic organisms L. fermentum and L. casei decreased the abundance of Clostridium cluster I but not of E. coli. Similar inhibition of C. perfringens was observed in weaning piglets that received L. plantarum but not in animals that received L. reuteri (Takahashi et al., 2008; Yang et al., 2015b). A possible explanation for this divergent effect is that host-adapted L. reuteri eliminated ETEC colonization through competitive exclusion in the small intestine. The strain used in the present study represents a rodent-adapted lineage of L. reuteri (Zheng et al., 2016), however, rodent-lineage strains of L. reuteri colonize the swine intestine equally well as strains from swine-adapted lineages (Duar et al., 2017a). However, the current study compared only small numbers of strains and the prevention of ETEC-induced diarrhea in piglets, along with an enhanced pro-inflammatory response, was also observed with the nomadic L. rhamnosus GG (Zhang et al., 2010). Therefore, differential effects of host adapted and nomadic lactobacilli on intestinal microbial ecology and host immunity remain to be validated in future studies that employ a larger number of host adapted and nomadic strains, and determine their impact on host immunity (Wang and Gänzle, 2019). The performance of L. fermentum and L. casei was not influenced by the mode of delivery, i.e., freeze dried or feed fermentation. Appropriate drying processes facilitate the development of probiotic cultures with high viability and stable immunomodulatory properties (Isolauri et al., 1991; Iaconelli et al., 2015). Of note, the strain L. reuteri TMW1.656 was more effective against E. coli when administered in higher cell counts (Yang et al., 2015a). Feed fermentations are a suitable tool to deliver adequate and high numbers of probiotic cells and may reduce cost in animal production. In addition, feed fermentations take advantages of nutritional advantages of feed fermentations (Stanton et al., 2005; Jørgensen et al., 2010).
Impact of Reutericyclin on Intestinal Microbiota
Probiotic lactobacilli exerted only limited effects on the overall composition of fecal microbiota of weanling pigs. Metagenomic analysis of the same animal experiment demonstrated that the initial composition of fecal microbial community varied among litters and was then reshaped by feed over time, which agreed with significance of the diet in modulating development of swine gut microbiota (Wang et al., 2019). Reutericyclin-producing L. reuteri TMW1.656 reduced Limosilactobacillus (present study) and transiently inhibited other lactobacilli (Zhao et al., 2018). Bacteriocin expression by commensal bacteria or probiotic lactobacilli was suggested to favor their persistence in the host intestine and to enhance their probiotic effects (Kommineni et al., 2015). However, the low prevalence of DNA sequences coding for bacteriocins in human colonic microbiota implies that bacteriocin production is less relevant in the colon when compared to other body sites, particularly the oral cavity and the vagina (Kommineni et al., 2015; Zheng et al., 2015; Liu et al., 2016). Only few studies report on the relative impact of bacteriocin-producing and bacteriocin non-producing probiotics on swine intestinal microbiota, and even fewer compare isogenic mutant strains to account for strain-specific probiotic effects. A bacteriocin producing strain of L. salivarius exerted a subtle but significant impact on mouse and pig gut microbiota (Riboulet-Bisson et al., 2012). Likewise, previous studies that compared the reutericyclin producing L. reuteri TM1.656 with a reutericyclin-negative wild type strain of L. reuteri reported significant but minor changes in the composition of colonic microbiota (Yang et al., 2015b). Reutericyclin is produced to active concentrations in cereal fermentations (Gänzle and Vogel, 2003) and thus likely is also produced in the mainly wheat-based swine diet (this study). The comparison of L. reuteri TMW1.656 with the isogenic reutericyclin-negative strain L. reuteri TMW1.656ΔrtcN confirmed that the antimicrobial compound has only a limited impact on swine intestinal microbiota. In particular, the use of reutericyclin producing probiotic strains had no impact on the abundance of C. perfringens, an organisms that is sensitive to reutericyclin activity in vitro (Hofstetter et al., 2013). A possible explanation for the failure of L. reuteri to inhibit C. perfringens may be the site of the intestinal tract where the respective organisms are active. L. reuteri colonizes the osophagus of swine while C. perfringens colonizes the large intestine (Leser et al., 2002; Walter, 2008).
Impact of Probiotic Intervention and Other Factors on Virulence Factors of Enterotoxigenic Escherichia coli
The primary cause of post weaning diarrhea, ETEC, uses diverse virulence factors to trigger diarrhea. In particular multiple different fimbriae are alternatively used to mediate adhesion to the small intestinal mucosa, and production of one or more of three diarrheal toxins causes fluid loss and diarrhea (Francis, 2002). Immunization with recombinant vaccines (Lin et al., 2013; Pereira et al., 2015) or the prevention of infection by analogs of the fimbriae receptor glycans (Shoaf-Sweeney and Hutkins, 2008; Chen et al., 2014) requires accurate diagnosis of dominant virulence factors. Our high-resolution analysis of ETEC virulence factors described the evolution of virulence factors of ETEC at suckling and post-weaning stages. As the receptor for F18 fimbriae is absent in neonatal pigs, the relevance of ETEC with F18 fimbriae increases post-weaning (Nagy et al., 1997; Fairbrother et al., 2005), an effect that was also observed in the current study. The decreasing abundance of the LT and an increased abundance of Sta confirms the higher frequency of ETEC expressing the combination of Stb/F18 and LT/K88 in weanling pigs (Wilson and Francis, 1986; Osek, 1999; Frydendahl, 2002). Few ETEC strains additionally carry Stx2e, which are referred as hybrid Shiga toxin-producing E. coli/ETEC (STEC/ETEC) but was not included in our detection spectrum (Leonard et al., 2016).
The quantification of virulence factors of ETEC also demonstrated that piglets from the same sow harbored a more similar profile of virulence factors when analyzed shortly after weaning. This demonstrates that maternal-neonatal transmission is an important contributor to the early colonization of ETEC. The importance of strain-level transmission of commensal organisms has been demonstrated for mothers and their children (Yassour et al., 2018) but not yet for sows and piglets. In consequence, maintaining good sanitary conditions and gut health of the sows are key to successful ETEC prevention in swine production.
Time or age significantly contributed to the variations in patterns of ETEC virulence factors (this study) and microbiota assembly in weanling pigs (Wang et al., 2019). Therefore, it was not accidental that the longitudinal profiles of ETEC virulence factor coincided with development of gut microbiota in weanling pigs. In previous studies, the abundance of Bacteroidetes, including genera from Prevotellaceae, Lachnospiraceae, and Ruminococcaceae families were higher in health pig than that in diarrheal pigs (Gorkiewicz et al., 2013; Dou et al., 2017), which positively correlated with the increasing colonization of Bacteroidetes after weaning (Molist et al., 2012). Thus, promoting an earlier colonization of Bacteroidetes may be a strategy to decease the incidence of ETEC infection in early weaned pigs.
In conclusion, host-adapted and nomadic probiotic lactobacilli inhibited different pathogens in healthy weanling pigs. The host adapted L. reuteri was more effective against ETEC while nomadic L. casei and L. fermentum were effective against C. perfringens. Bacteriocin production was less relevant to antimicrobial effects of lactobacilli in the colon. Early colonization of ETEC was associated with maternal-neonatal transmission and decreased with colonization of Bacteroidetes over time after weaning. Feed fermentation with probiotic strains may find a wider application in animal production as an appropriate tool for cost-effective delivery of probiotic strains.
Data Availability Statement
The sequence data are deposited at the National Center for Biotechnology Information (NCBI) under BioProject PRJNA665253.
Ethics Statement
The animal study was reviewed and approved by the University of Alberta Animal Care and Use Committee for Livestock.
Author Contributions
MG and RZ conceived and designed the experiments. WW performed the experiments and analyzed the data. WW and MG wrote the manuscript. WW, MG, and RZ reviewed the manuscript. All authors read and approved the final manuscript.
Funding
MG and RZ acknowledge funding by the Alberta Livestock and Meat Agency. WW was supported by China Scholarship Council. MG was supported by the Canada Research Chairs Program. The project was supported by the Alberta Livestock and Meat Agency grant nos. 2013R002R and 2015B009R.
Conflict of Interest
The authors declare that the research was conducted in the absence of any commercial or financial relationships that could be construed as a potential conflict of interest.
Acknowledgments
We wish to thank Xin Zhao for sourdough preparation.
Supplementary Material
The Supplementary Material for this article can be found online at: https://www.frontiersin.org/articles/10.3389/fmicb.2020.608293/full#supplementary-material
Footnotes
References
Bolyen, E., Rideout, J. R., Dillon, M. R., Bokulich, N. A., Abnet, C. C., Al-Ghalith, G. A., et al. (2019). Reproducible, interactive, scalable and extensible microbiome data science using QIIME 2. Nat. Biotechnol. 37, 852–857. doi: 10.1038/s41587-019-0209-9
Callahan, B. J., McMurdie, P. J., Rosen, M. J., Han, A. W., Johnson, A. J. A., and Holmes, S. P. (2016). DADA2: high-resolution sample inference from Illumina amplicon data. Nat. Methods 13, 581–583. doi: 10.1038/nmeth.3869
Chen, X. Y., Woodward, A., Zijlstra, R. T., and Gänzle, M. G. (2014). Exopolysaccharides synthesized by Lactobacillus reuteri protect against enterotoxigenic Escherichia coli in piglets. Appl. Environ. Microbiol. 80, 5752–5760. doi: 10.1128/AEM.01782-14
Dou, S., Gadonna-Widehem, P., Rome, V., Hamoudi, D., Rhazi, L., Lakhal, L., et al. (2017). Characterisation of early-life fecal microbiota in susceptible and healthy pigs to post-weaning diarrhoea. PLoS One 12:e0169851. doi: 10.1371/journal.pone.0169851
Duar, R. M., Frese, S. A., Lin, X. B., Fernando, S. C., Burkey, T. E., Tasseva, G., et al. (2017a). Experimental evaluation of host adaptation of Lactobacillus reuteri to different vertebrate species. Appl. Environ. Microbiol. 83:e0132-17. doi: 10.1128/AEM.00132-17
Duar, R. M., Lin, X. B., Zheng, J., Martino, M. E., Grenier, T., Pérez-Muñoz, M. E., et al. (2017b). Lifestyles in transition: evolution and natural history of the genus Lactobacillus. FEMS Microbiol. Rev. 41, S27–S48. doi: 10.1093/femsre/fux030
Fairbrother, J. M., and Nadeau, É, and Gyles, C. L. (2005). Escherichia coli in postweaning diarrhea in pigs: an update on bacterial types, pathogenesis, and prevention strategies. Anim. Heal. Res. Rev. 6, 17–39. doi: 10.1079/AHR2005105
Felder, C. B., Vorlaender, N., Gander, B., Merkle, H. P., and Bertschinger, H. U. (2001). Microencapsulated enterotoxigenic Escherichia coli and detached fimbriae for peroral vaccination of pigs. Vaccine 19, 706–715. doi: 10.1016/S0264-410X(00)00264-4
Francis, D. H. (2002). Enterotoxigenic Escherichia coli infection in pigs and its diagnosis. J. Swine Health Prod. 10, 171–175. doi: 10.1074/jbc.M112.404608
Frydendahl, K. (2002). Prevalence of serogroups and virulence genes in Escherichia coli associated with postweaning diarrhoea and edema disease in pigs and a comparison of diagnostic approaches. Vet. Microbiol. 85, 169–182. doi: 10.1016/S0378-1135(01)00504-1
Gänzle, M. G., and Vogel, R. F. (2003). Contribution of reutericyclin production to the stable persistence of Lactobacillus reuteri in an industrial sourdough fermentation. Int. J. Food Microbiol. 80, 31–45. doi: 10.1016/S0168-1605(02)00146-0
Gorkiewicz, G., Thallinger, G. G., Trajanoski, S., Lackner, S., Stocker, G., Hinterleitner, T., et al. (2013). Alterations in the colonic microbiota in response to osmotic diarrhea. PLoS One 8:e55817. doi: 10.1371/journal.pone.0055817
Gresse, R., Chaucheyras-Durand, F., Fleury, M. A., Van de Wiele, T., Forano, E., and Blanquet-Diot, S. (2017). Gut microbiota dysbiosis in postweaning piglets: understanding the keys to health. Trends Microbiol. 25, 851–873. doi: 10.1016/J.TIM.2017.05.004
Hill, C., Guarner, F., Reid, G., Gibson, G. R., Merenstein, D. J., Pot, B., et al. (2014). The International scientific association for probiotics and Prebiotics consensus statement on the scope and appropriate use of the term probiotic. Nat. Rev. Gastroenterol. Hepatol. 11, 506–514. doi: 10.1038/nrgastro.2014.66
Hofstetter, S., Gebhardt, D., Ho, L., Gänzle, M., and McMullen, L. M. (2013). Effects of nisin and reutericyclin on resistance of endospores of Clostridium spp. to heat and high pressure. Food Microbiol. 34, 46–51. doi: 10.1016/J.FM.2012.11.001
Iaconelli, C., Lemetais, G., Kechaou, N., Chain, F., Bermúdez-Humarán, L. G., Langella, P., et al. (2015). Drying process strongly affects probiotics viability and functionalities. J. Biotechnol. 214, 17–26. doi: 10.1016/J.JBIOTEC.2015.08.022
Isolauri, E., Rautanen, T., Juntunen, M., Sillanaukee, P., and Koivula, T. (1991). A human Lactobacillus strain (Lactobacillus casei sp strain GG) promotes recovery from acute diarrhea in children. Pediatrics 88, 90–97.
Jørgensen, H., Sholly, D., Pedersen, A. Ø, Canibe, N., and Knudsen, K. E. B. (2010). Fermentation of cereals - influence on digestibility of nutrients in growing pigs. Livest. Sci. 134, 56–58. doi: 10.1016/J.LIVSCI.2010.06.096
Katrien, V., Vesna, M., Eric, C., Jean Paul, R., and Chris, V. (2010). Adjuvant effect of Gantrez® AN nanoparticles during oral vaccination of piglets against F4+enterotoxigenic Escherichia coli. Vet. Immunol. Immunopathol. 139, 148–155. doi: 10.1016/j.vetimm.2010.09.009
Katsuda, K., Kohmoto, M., Kawashima, K., and Tsunemitsu, H. (2006). Frequency of enteropathogen detection in suckling and weaned pigs with diarrhea in Japan. J. Vet. Diagnost. Investig. 18, 350–354. doi: 10.1177/104063870601800405
Kim, P. I. L., Jung, M. Y., Chang, Y.-H., Kim, S., Kim, S.-J., and Park, Y.-H. (2007). Probiotic properties of Lactobacillus and Bifidobacterium strains isolated from porcine gastrointestinal tract. Appl. Microbiol. Biotechnol. 74, 1103–1111. doi: 10.1007/s00253-006-0741-7
Kommineni, S., Bretl, D. J., Lam, V., Chakraborty, R., Hayward, M., Simpson, P., et al. (2015). Bacteriocin production augments niche competition by Enterococci in the mammalian gastrointestinal tract. Nature 526, 719–722. doi: 10.1038/nature15524
Kyriakis, S. C., Sarris, K., Kritas, S. K., Tsinas, A. C., and Giannakopoulos, C. (1996). Effect of salinomycin in the control of Clostridium perfringens type C infection in suckling pigs. Vet. Rec. 138, 281–283. doi: 10.1136/vr.138.12.281
Leonard, S. R., Mammel, M. K., Rasko, D. A., and Lacher, D. W. (2016). Hybrid shiga toxin-producing and enterotoxigenic Escherichia sp. cryptic lineage 1 strain 7v harbors a hybrid plasmid. Appl. Environ. Microbiol. 82, 4309–4319. doi: 10.1128/AEM.01129-16
Leser, T. T. D., Amenuvor, J. Z. J., Jensen, T. T. K., Lindecrona, R. R. H., Boye, M., and Møller, K. (2002). Culture-independent analysis of gut bacteria: the pig gastrointestinal tract microbiota revisited. Appl. Environ. Microbiol. 68, 673–690. doi: 10.1128/aem.68.2.673-690.2002
Li, X.-Q., Zhu, Y.-H., Zhang, H.-F., Yue, Y., Cai, Z.-X., Lu, Q.-P., et al. (2012). Risks associated with high-dose Lactobacillus rhamnosus in an Escherichia coli model of piglet diarrhoea: intestinal microbiota and immune imbalances. PLoS One 7:e40666. doi: 10.1371/journal.pone.0040666
Liao, S. F., and Nyachoti, M. (2017). Using probiotics to improve swine gut health and nutrient utilization. Anim. Nutr. 3, 331–343. doi: 10.1016/j.aninu.2017.06.007
Lin, J., Mateo, K. S., Zhao, M., Erickson, A. K., Garcia, N., He, D., et al. (2013). Protection of piglets against enteric colibacillosis by intranasal immunization with K88ac (F4ac) fimbriae and heat labile enterotoxin of Escherichia coli. Vet. Microbiol. 162, 731–739. doi: 10.1016/j.vetmic.2012.09.025
Lin, X. B., Lohans, C. T., Duar, R., Zheng, J., Vederas, J. C., Walter, J., et al. (2015). Genetic determinants of reutericyclin biosynthesis in Lactobacillus reuteri. Appl. Environ. Microbiol. 81, 2032–2041. doi: 10.1128/AEM.03691-14
Lin, Y. P., Thibodeaux, C. H., Peña, J. A., Ferry, G. D., and Versalovic, J. (2008). Probiotic Lactobacillus reuteri suppress proinflammatory cytokines via c-Jun. Inflamm. Bowel Dis. 14, 1068–1083. doi: 10.1002/ibd.20448
Liu, L., Hao, T., Xie, Z., Horsman, G. P., and Chen, Y. (2016). Genome mining unveils widespread natural product biosynthetic capacity in human oral microbe Streptococcus mutans. Sci. Rep. 6:37479. doi: 10.1038/srep37479
Livingston, M., Loach, D., Wilson, M., Tannock, G. W., and Baird, M. (2010). Gut commensal Lactobacillus reuteri 100-23 stimulates an immunoregulatory response. Immunol. Cell Biol. 88, 99–102. doi: 10.1038/icb.2009.71
Maldonado-Gómez, M. X., Martínez, I., Bottacini, F., O’Callaghan, A., Ventura, M., van Sinderen, D., et al. (2016). Stable engraftment of Bifidobacterium longum AH1206 in the human gut depends on individualized features of the resident microbiome. Cell Host Microb. 20, 515–526. doi: 10.1016/j.chom.2016.09.001
Marko, A., Rakická, M., Mikušová, L., Valík, L., and Šturdík, E. (2014). Lactic acid fermentation of cereal substrates in nutritional perspective. Int. J. Res. Chem. Environ. 4, 80–92.
Meroth, C. B., Walter, J., Hertel, C., Brandt, M. J., and Hammes, W. P. (2003). Monitoring the bacterial population dynamics in sourdough fermentation processes by using PCR-denaturing gradient gel electrophoresis. Appl. Environ. Microbiol. 69, 475–482. doi: 10.1128/AEM.69.1.475-482.2003
Molist, F., Manzanilla, E. G., Pérez, J. F., and Nyachoti, C. M. (2012). Coarse, but not finely ground, dietary fibre increases intestinal firmicutes: bacteroidetes ratio and reduces diarrhoea induced by experimental infection in piglets. Br. J. Nutr. 108, 9–15. doi: 10.1017/S0007114511005216
Nagy, B., Whipp, S. C., Imberechts, H., Bertschinger, H. U., Dean-Nystrom, E. A., Casey, T. A., et al. (1997). Biological relationship between F18ab and F18ac fimbriae of enterotoxigenic and verotoxigenic Escherichia coli from weaned pigs with oedema disease or diarrhoea. Microb. Pathog. 22, 1–11. doi: 10.1006/MPAT.1996.0085
Osek, J. (1999). Prevalence of virulence factors of Escherichia coli strains isolated from diarrheic and healthy piglets after weaning. Vet. Microbiol. 68, 209–217. doi: 10.1016/S0378-1135(99)00109-1
Pereira, D. A., Silva, C. A., Ono, M. A., Vidotto, O., and Vidotto, M. C. (2015). Humoral immune response of immunized sows with recombinant proteins of enterotoxigenic Escherichia coli. World J. Vacc. 05, 60–68. doi: 10.4236/wjv.2015.51008
Riboulet-Bisson, E., Sturme, M. H. J., Jeffery, I. B., O’Donnell, M. M., Neville, B. A., Forde, B. M., et al. (2012). Effect of Lactobacillus salivarius bacteriocin Abp118 on the mouse and pig intestinal microbiota. PLoS One 7:e31113. doi: 10.1371/journal.pone.0031113
Ritter, A. C., Paula, A., Correa, F., Veras, F. F., and Brandelli, A. (2018). Characterization of Bacillus subtilis available as probiotics. J. Microbiol. Res. 8, 23–32. doi: 10.5923/j.microbiology.20180802.01
Roselli, M., Finamore, A., Britti, M. S., Konstantinov, S. R., Smidt, H., de Vos, W. M., et al. (2018). The novel porcine Lactobacillus sobrius strain protects intestinal cells from enterotoxigenic Escherichia coli K88 infection and prevents membrane barrier damage. J. Nutr. 137, 2709–2716. doi: 10.1093/jn/137.12.2709
Scharek-Tedin, L., Pieper, R., Vahjen, W., Tedin, K., Neumann, K., and Zentek, J. (2013). Bacillus cereus var. Toyoi modulates the immune reaction and reduces the occurrence of diarrhea in piglets challenged with Salmonella typhimurium DT1041. J. Anim. Sci. 91, 5696–5704. doi: 10.2527/jas.2013-6382
Shoaf-Sweeney, K. D., and Hutkins, R. W. (2008). Chapter 2 Adherence, Anti-Adherence, and Oligosaccharides: preventing pathogens from sticking to the host. Adv. Food Nutr. Rese. 55, 101–161. doi: 10.1016/S1043-4526(08)00402-6
Sonia, T. A., Ji, H., Hong-Seok, M., and Chul-Ju, Y. (2014). Evaluation of Lactobacillus and Bacillus-based probiotics as alternatives to antibiotics in enteric microbial challenged weaned piglets. Afr. J. Microbiol. Res. 8, 96–104. doi: 10.5897/AJMR2013.6355
Stanton, C., Ross, R. P., Fitzgerald, G. F., and Van Sinderen, D. (2005). Fermented functional foods based on probiotics and their biogenic metabolites. Curr. Opin. Biotechnol. 16, 198–203. doi: 10.1016/J.COPBIO.2005.02.008
Takahashi, S., Yoshida, Y., Nakanishi, N., Tsukahara, T., and Ushida, K. (2008). Quantitative real-time PCR monitoring of Escherichia coli and Clostridium perfringens with oral administration of Lactobacillus plantarum strain Lq80 to weaning piglets. Anim. Sci. J. 79, 737–744. doi: 10.1111/j.1740-0929.2008.00588.x
Upadhaya, S. D., Shanmugam, S. K., Kang, D. K., and Kim, I. H. (2017). Preliminary assessment on potentials of probiotic B. subtilis RX7 and B. methylotrophicus C14 strains as an immune modulator in Salmonella-challenged weaned pigs. Trop. Anim. Health Prod. 49, 1065–1070. doi: 10.1007/s11250-017-1278-8
Walter, J. (2008). Ecological role of lactobacilli in the gastrointestinal tract: implications for fundamental and biomedical research. Appl. Environ. Microbiol. 74, 4985–4996. doi: 10.1128/AEM.00753-08
Walter, J., Maldonado-Gomez, M. X., Martinez, I., Maldonado-Gómez, M. X., and Martínez, I. (2018). To engraft or not to engraft: an ecological framework for gut microbiome modulation with live microbes. Curr. Opin. Biotechnol 49, 129–139. doi: 10.1016/j.copbio.2017.08.008
Wang, A., Haifeng, A. E., Ae, Y., Gao, X., Xiaojie, A. E., Ae, L., et al. (2009). Influence of Lactobacillus fermentum I5007 on the intestinal and systemic immune responses of healthy and E. coli challenged piglets. Antonie Van Leeuwenhoek 96, 89–98. doi: 10.1007/s10482-009-9339-2
Wang, W., and Gänzle, M. (2019). “Towards rational selection criteria for selection of probiotics in pigs,” in Advances in Applied Microbiology, eds G. M. Gadd and S. Sariaslani (Amsterdam: Elsevier), 84–112. doi: 10.1016/bs.aambs.2019.03.003
Wang, W., Hu, H., Zijlstra, R. T., Zheng, J., and Gänzle, M. G. (2019). Metagenomic reconstructions of gut microbial metabolism in weanling pigs. Microbiome 7:48. doi: 10.1186/s40168-019-0662-1
Wang, W., Zijlstra, R. T., and Gänzle, M. G. (2017). Identification and quantification of virulence factors of enterotoxigenic Escherichia coli by high-resolution melting curve quantitative PCR. BMC Microbiol. 17:114. doi: 10.1186/s12866-017-1023-5
Wilson, R. A., and Francis, D. H. (1986). Fimbriae and enterotoxins associated with Escherichia coli serogroups isolated from pigs with colibacillosis. Am. J. Vet. Res. 47, 213–217.
Yang, Y., Galle, S., Le, M. H. A., Zijlstra, R. T. R. T., and Gänzle, M. G. (2015a). Feed fermentation with reuteran- and levan-producing Lactobacillus reuteri reduces colonization of weanling pigs by enterotoxigenic Escherichia coli. Appl. Enviorn. Microbiol. 81, 5743–5752. doi: 10.1128/AEM.01525-15
Yang, Y., Zhao, X., Le, M. H. A., Zijlstra, R. T., and Gänzle, M. G. (2015b). Reutericyclin producing Lactobacillus reuteri modulates development of fecal microbiota in weanling pigs. Front. Microbiol. 6:762. doi: 10.3389/fmicb.2015.00762
Yassour, M., Jason, E., Hogstrom, L. J., Arthur, T. D., Tripathi, S., Siljander, H., et al. (2018). Strain-level analysis of mother-to-child bacterial transmission during the first few months of life. Cell Host Microb. 24, 146–154.e4. doi: 10.1016/J.CHOM.2018.06.007
Zhang, L., Xu, Y.-Q., Liu, H.-Y., Lai, T., Ma, J.-L., Wang, J.-F., et al. (2010). Evaluation of Lactobacillus rhamnosus GG using an Escherichia coli K88 model of piglet diarrhoea: effects on diarrhoea incidence, faecal microflora and immune responses. Vet. Microbiol. 141, 142–148. doi: 10.1016/J.VETMIC.2009.09.003
Zhao, X., Wang, W., Blaine, A., Kane, S. T. T., Zijlstra, R. T. T., and Gänzle, M. G. G. (2018). Impact of probiotic Lactobacillus sp.on autochthonous lactobacilli in weaned piglets. J. Appl. Microbiol. 126, 242–254. doi: 10.1111/jam.14119
Zheng, J., Gänzle, M. G., Lin, R., Xiaoxi, B., Ruan, L., and Sun, M. (2015). Diversity and dynamics of bacteriocins from human microbiome. Environ. Microbiol. 17, 2133–2143. doi: 10.1111/1462-2920.12662
Zheng, J., Wittouck, S., Salvetti, E., Franz, C. M. A. B., Harris, H. M. B., Mattarelli, P., et al. (2020). A taxonomic note on the genus Lactobacillus: description of 23 novel genera, emended description of the genus Lactobacillus Beijerinck 1901, and union of Lactobacillaceae and Leuconostocaceae. Int. J. Syst. Evol. Microbiol. 70, 2782–2858. doi: 10.1099/ijsem.0.004107
Zheng, J., Zhao, X., Lin, X. B., and Gänzle, M. (2016). Comparative genomics Lactobacillus reuteri from sourdough reveals adaptation of an intestinal symbiont to food fermentations. Sci. Rep. 5:18234. doi: 10.1038/srep18234
Keywords: probiotics, Lactobacillus fermentum, Lactobacillus casei, Lactobacillus reuteri, reutericyclin, ETEC, weanling pigs
Citation: Wang W, Zijlstra RT and Gänzle MG (2020) Feeding Limosilactobacillus fermentum K9-2 and Lacticaseibacillus casei K9-1, or Limosilactobacillus reuteri TMW1.656 Reduces Pathogen Load in Weanling Pigs. Front. Microbiol. 11:608293. doi: 10.3389/fmicb.2020.608293
Received: 19 September 2020; Accepted: 23 November 2020;
Published: 17 December 2020.
Edited by:
Fernanda Mozzi, CONICET Centro de Referencia para Lactobacilos (CERELA), ArgentinaReviewed by:
Julio Villena, CONICET Centro de Referencia para Lactobacilos (CERELA), ArgentinaRobert Pieper, Freie Universität Berlin, Germany
Copyright © 2020 Wang, Zijlstra and Gänzle. This is an open-access article distributed under the terms of the Creative Commons Attribution License (CC BY). The use, distribution or reproduction in other forums is permitted, provided the original author(s) and the copyright owner(s) are credited and that the original publication in this journal is cited, in accordance with accepted academic practice. No use, distribution or reproduction is permitted which does not comply with these terms.
*Correspondence: Michael G. Gänzle, bWdhZW56bGVAdWFsYmVydGEuY2E=