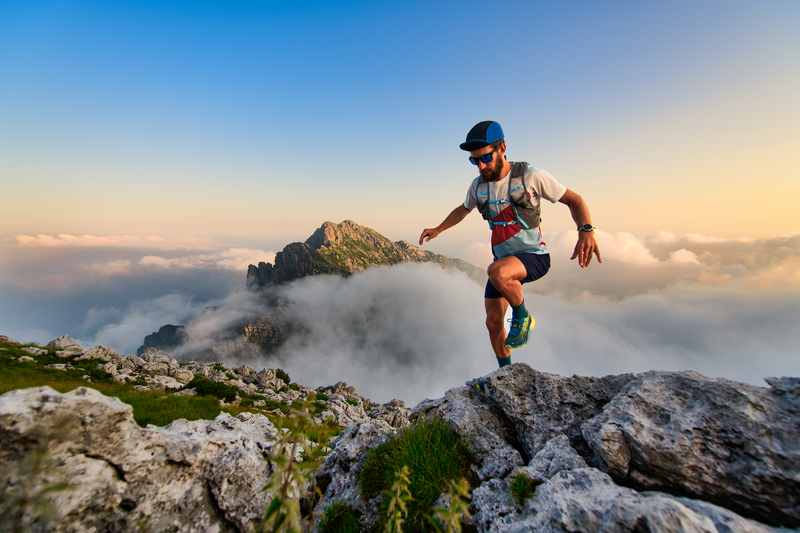
94% of researchers rate our articles as excellent or good
Learn more about the work of our research integrity team to safeguard the quality of each article we publish.
Find out more
REVIEW article
Front. Microbiol. , 12 January 2021
Sec. Antimicrobials, Resistance and Chemotherapy
Volume 11 - 2020 | https://doi.org/10.3389/fmicb.2020.607099
This article is part of the Research Topic Insights into New Strategies to Combat Biofilms View all 29 articles
The drug resistance developed by bacteria during antibiotic treatment has been a call to action for researchers and scientists across the globe, as bacteria and fungi develop ever increasing resistance to current drugs. Innovative antimicrobial/antibacterial materials and coatings to combat such infections have become a priority, as many infections are caused by indwelling implants (e.g., catheters) as well as improving postsurgical function and outcomes. Pathogenic microorganisms that can exist either in planktonic form or as biofilms in water-carrying pipelines are one of the sources responsible for causing water-borne infections. To combat this, researchers have developed nanotextured surfaces with bactericidal properties mirroring the topographical features of some natural antibacterial materials. Protein-based adhesives, secreted by marine mussels, contain a catecholic amino acid, 3,4-dihydroxyphenylalanine (DOPA), which, in the presence of lysine amino acid, empowers with the ability to anchor them to various surfaces in both wet and saline habitats. Inspired by these features, a novel coating material derived from a catechol derivative, dopamine, known as polydopamine (PDA), has been designed and developed with the ability to adhere to almost all kinds of substrates. Looking at the immense potential of PDA, this review article offers an overview of the recent growth in the field of PDA and its derivatives, especially focusing the promising applications as antibacterial nanocoatings and discussing various antimicrobial mechanisms including reactive oxygen species-mediated antimicrobial properties.
A considerable number of catecholic residues similar to those found in the mussel foot proteins (mytilus foot proteins, MFP-3 and 5) and melanin have attracted the attention of researchers around the globe. A plethora of articles/reviews has been presented on the structure, reaction mechanism, and biomedical and biotechnological applications of materials containing these functionalities (Solano, 2014, 2017; Ahn, 2017; Ball, 2017; Forooshani and Lee, 2017; Maier and Butler, 2017; Rahimnejad and Zhong, 2017; Moulay, 2018; Park et al., 2019). Polydopamine (PDA) is one such material and is an extremely interesting polymer, ennobled with unique features – such as adherence to all types of surfaces even under water, a characteristic attributed to the catechol moieties in its monomeric building blocks. The polymer, reaped by dopamine oxidation, contains indole and dopamine units in various oxidation states and also, to a smaller extent, pyrroles. The oxidized o-quinone and o-hydroquinone groups in its chemical structure underlie the complex oxidation chemical characteristics of PDA (Lynge et al., 2011; Jia et al., 2019). Further, it has been shown that, during oxidation of dopamine, reactive oxygen species are generated, which act as bactericidal against both Gram-positive and Gram-negative bacteria (Lynge et al., 2011). Hence, the coating of such materials on the surfaces affects the growth and survival of microorganisms. Work has also indicated that PDA has the potential to reduce the in vivo toxicity of biomaterials, which come in contact with the tissues or blood and suggest it to be a versatile platform (Hong et al., 2011).
Since the method reported by Lee et al. (2007) for dopamine self-polymerization to form thin films, surface-adherent, multifunctional PDA coatings through simple dip-coating of a wide-range of inorganic and organic materials, many PDA-based nanomaterials have been generated. In a recent study, it has been shown that PDA nanoparticles synthesized by laccase catalysis were more stable in strongly alkaline and acidic solutions than those synthesized by more traditional chemical routes (Li et al., 2018), although chemical oxidation processes are more cost effective for mass production and reproducibility. Various additives incorporated during synthesis can tune material properties via covalent cross-linking or physical entrapment and through non-covalent interactions (Ang et al., 2016; Chen et al., 2018; Wang et al., 2018), such as cation–π interactions (Hong et al., 2018). In a similar vein, chemically reactive nanoparticles can act as core templates for multifunctional use, such as coatings on the surface of other template/core materials (Lin et al., 2015; Hong et al., 2017). A review article by Ball has described the synthesis methods that yield PDA nanoparticles in the absence/presence of templating agents along with the use of thin PDA layers on nanoparticles or nanotubes (Ball, 2017). The results of synthesis in the presence of templating agent indicate a strong correlation between the catecholamine and the templating molecules with awaited investigation on the nature and the strength of interactions (Ball, 2018).
Despite the research being carried out, the chemical basis of the amazing underwater adhesion properties of PDA remains unclear. Mussel adhesiveness has persuaded scientists to explore the self-polymerization of dopamine under alkaline conditions. The solution to this puzzle could provide a universal type of coating for polymers, metals, and ceramics irrespective of their physical and chemical properties. The polymerized layer, enriched with catechol groups, can immobilize primary amine or thiol-based biomolecules through a simple dipping process. In one of the studies, Hong et al. (2012) have demonstrated that a small amount of free dopamine left physically entrapped in synthesized PDA does not exhibit cytotoxicity, as it is rarely released due to strong non-covalent π–π interactions with aromatic rings.
Work to understand the PDA structure is still under deliberation and suggests that it is composed of dihydroxyindole, indoledione, and dopamine units, covalently linked (Figure 1). Further verification of the presence of covalently linked dihydroxyindole and indoledione units between their aromatic rings leading to different degrees of unsaturation has been suggested by Liebscher et al. (2013). The work by Alfieri et al. (2017) has concluded that PDA is different from 5,6-dihydroxyindole and melanin, and PDA film formation requires amine-containing structural components for adhesion and film-forming properties. Further, the group has noticed that PDA film formation involves competition/dynamic interactions among the intermolecular amine–quinone condensation processes, while film deposition accelerates with quinone-forming oxidants and depends on dopamine concentration and becomes practically negligible below 1 mM dopamine (Alfieri et al., 2018). The catechol groups of PDA have the ability to reduce noble metal salts (Ag+, Au3+) to metal nanoparticles and immobilize the nanoparticles within the scaffolds, thereby preventing aggregation or leaching (Holten-Andersen et al., 2009). A review by Ho and Ding (Ho and Ding, 2014) has highlighted the probable adhesion mechanisms, chemical/physical properties, and applications of PDA. Liebscher has presented an overview on the chemistry and properties of PDA and its analogs (Liebscher, 2019). Among all the rationale for the overall mechanism for the polydopamine formation, two different pathways have also been suggested: self-assembled (dopamine)2/5,6-dihydroxyindole (DHI) trimmers, assembled via quadrupole–quadrupole, hydrogen bonding, and π–π interactions, and dopamine–DHI–DHI conjugates, formed via covalent bond (Hong et al., 2012). However, all the views conclude that the insoluble biopolymer PDA system, formed by autoxidation of the catecholamine neurotransmitter dopamine, encompasses non-cyclized dopamine units, 5,6-dihydroxyindole (DHI), indole-5,6-quinone (IQ), and their oligomers as the main structural units (Barclay et al., 2017).
As PDA and eumelanins are found in various parts of the human body, they possess excellent physicochemical properties and provide good biocompatibility (d’Ischia et al., 2014). Hence, PDA holds considerable promise for integration with biological tissues. Polydopamine coatings are accepted as the foremost outfit for functionalization of practically any kind of material surface. The absence of strong π-conjugated system of catechol in DHI is caused by protonated uncyclized amines supporting the formation of polydopamine (Lee et al., 2019). Several factors, viz., initial dopamine concentration, pH, reaction temperature, and choice of buffer and oxidant, are reported to play important roles during the preparation of PDA coatings, and, subsequently, the physical properties of PDA coatings control the surface characteristics, which, in turn, might significantly alter the antibacterial activities (Ding et al., 2016).
Surface wettability is an important property based on surface energy and morphology of the material that addresses adhesion. The hydrophobic surface exhibits stronger adhesion with the PDA coatings compared to the hydrophilic surfaces, as a “hydrophobic depletion layer” on the hydrophobic substrate is suggested to enhance the PDA adhesion by allowing an intimate contact between PDA and the substrate (Zhang et al., 2017). The hydrophilic behavior of PDA due to the presence of polar functional groups supports cell attachment and growth. Various co-deposition processes have been suggested to address the insufficient hydrophilicity in PDA (Qiu et al., 2018).
Polydopamine is moderately hydrophilic due to the large volumes of catechol, quinine, and amine groups. Reactants bearing functionalities such as amines and thiols can target the diketone or catechol groups exposed on the PDA surface via aza- or thio-Michael addition and Schiff base reactions, which can tune the surface properties of the polymer (Figure 2). Hence, PDA coatings can play the role as anchor to tether biopolymers consisting of thiols and amines (Lee et al., 2009; Huang et al., 2015; Liu and Huang, 2016; Tang et al., 2018; Yang et al., 2019). This has allowed immobilization of oligonucleotide-based aptamers and molecular probes and multifunctional nanoparticles for potential applications in nanomedicine and biosensors (Wang C. et al., 2016; Zheng et al., 2018; Jia et al., 2019). The phenolic groups of PDA have been reported to enhance the nucleation sites in the carbon dot formation to fabricate fluorescent PDA-passivated N-doped carbon dots (Bai et al., 2018).
Figure 2. Schiff base and Michael addition reactions of aminated and thiolated ligands with reactive functionalities of polydopamine (PDA).
Polydopamine finds numerous applications even as stable suspension of nanoparticles (NPs), particularly as biomaterials. The use of PDA/hybrids in various fields, e.g., converting light to heat for cancer treatment by hyperthermia (Liu F. et al., 2015; Dong et al., 2016; Wang X. et al., 2016; Li Y. et al., 2017; Nieto et al., 2018; Tian and Lei, 2019), catalytic therapy for tumors (Zhu et al., 2019), and for sensing (Kong et al., 2016; Wang Z. et al., 2017; Zhao et al., 2017; Gu et al., 2018), as well as PDA NPs loaded scaffolds in the field of tissue engineering (Deng et al., 2019), are all worth mentioning. A review article that highlights the progress in fluorescent PDA nanomaterials has been published by Yang et al. (2020b). PDA nanoparticles as sensors (Jiao et al., 2017; Ma et al., 2017), theranostics (Dong et al., 2016), or for drug release (Li W.Q. et al., 2017; Wang X. et al., 2019)/gene delivery (Priyam et al., 2018) are exploited regularly; for example, the toxic nature of polyethylenimine could be suppressed during covalent conjugation with PDA for gene delivery (Priyam et al., 2018). A review by Xiong et al. (2019) has discussed in detail the developments of PDA nanocarriers to deliver photosensitizers through chemical conjugation, physical absorption, and encapsulation strategies. A review by Batul et al. (2017) has discussed about the developments made in the area of drug delivery, photothermal therapy, and bone and tissue engineering using polydopamine nanostructures.
Polydopamine nanoparticles have been observed to display synergistic therapeutic effects for combined chemotherapy and photothermal therapy for cancer (Zhu and Su, 2017). PDA in association with photosensitizers conjugated to hyaluronic acid complexed nanoparticles has been reported to allow tumor-specific photodynamic therapy with degradation of the hyaluronic acid by the tumor-localized intracellular enzymes releasing the photosensitizer nanoparticles (Han et al., 2016).
Similarly, calcium carbonate–polydopamine (CaCO3–PDA) composite hollow nanoparticles have been reported to offer a multifunctional theranostic nanoplatform. The pH-dependent nanoparticles, quenched by PDA, could be photoactivated only in the acidic environment of a tumor whereby they released the photosensitizer and displayed multimodal imaging capability due to strong affinity between metal ions and PDA and exhibit high antitumor PDT efficacy (Dong et al., 2018). Various metal ions have been loaded onto PDA nanoparticles for bioimaging and photothermal cancer therapy simultaneously (Miao et al., 2015; Ge et al., 2017; Feng et al., 2020; Liu et al., 2020). Photocatalytic organic nanoparticles composed of flavin-conjugated PDA NPs have been described to display xenobiotic-degrading enzyme analogous activity (Fruk and Crocker, 2019).
Polydopamine coatings have been designed to enhance mucopenetration as well as cell uptake of NPs for mucosal drug delivery applications (Poinard et al., 2019). Mallinson et al., with the help of atomic force microscopy, suggested that PDA could be a useful coating to reduce interaction with proteins, which would otherwise lead to fouling (Mallinson et al., 2018). The hydrophilicity, aqueous durability under the physiological conditions, biocompatibility, as well as ease of functionalization make PDA nanocoatings apt candidates for numerous tissue engineering applications. PDA-assisted hydroxyapatite coating onto porous Ti6Al4V scaffolds has been reported to promote osteointegration and osteogenesis in vivo and could be useful for bone defect repair (Li et al., 2015). Ku and Park (2010) introduced PDA coatings for potential vascular tissue engineering applications (Ku and Park, 2010). It has been shown that PDA offers simple, effective, and inexpensive alternative for bone tissue engineering compared to traditional surface modification and tissue regeneration (Huang S. et al., 2016; Lee et al., 2017; Zhou et al., 2019). Madhurakkat Perikamana et al. (2015) have discussed in detail various techniques for versatile surface modification for tissue engineering.
The redox activities of catechol groups, pro- and antioxidant, in mussel adhesive proteins are a potential treasure for clinical applications. Catechol oxidation by air generates H2O2 and O2⋅– as reactive by-products. Forooshani et al. (2017) have reviewed the regulation of reactive oxygen species (ROS) and their effects in living system. The group further applied this redox chemistry of adhesive moiety to design microgels, which had the potential to generate H2O2 as and when needed for antimicrobial and antiviral applications (Meng et al., 2019). The antimicrobial property of PDA could also be accredited to the catechol moieties, which are released in a controlled manner and autoxidize in the presence of oxygen, forming semiquinone and quinone. The oxidation process generates ROS including two well-known disinfectants, superoxide anions (O2–) and H2O2 (Alfieri et al., 2017; Ryu et al., 2018). PDA is able to remove ROS that are generated during inflammatory responses. PDA nanoparticles have been shown to possess antioxidative properties to remove ROS and suppress ROS-induced inflammation in periodontal diseases without any side effects (Bao et al., 2018) and have been used effectively in the treatment of acute inflammation-induced injury (Zhao et al., 2018). PDA-coated hemoglobin nanoparticles showed oxidative protection of hemoglobin and antioxidative properties to remove ROS as well as reduce ROS generation besides exhibiting high oxygen affinity and low cytotoxicity (Wang Q. et al., 2017). Arginine-doped PDA has been shown to be a free-radical scavenger due to greater accessibility to free radicals and so has exhibited superior antioxidant performance than PDA-melanins (Yang et al., 2020a). A nanocomposite based on V2O5/PDA/MnO2 has been reported to exhibit an ability to remove intracellular reactive oxygen species and mimic intracellular antioxidant enzyme-based defense system flaunting potential for inflammation therapy (Huang Y. et al., 2016). Cu-loaded PDA coatings are being seen as a favorable platform for blood contact materials, which have the capability to catalyze the decomposition of S-nitrosothiols and release NO for a longer period thus maintaining the anticoagulant effects (Zhou et al., 2020).
Polydopamine has been illustrious for antibacterial and antifungal effects against several microorganisms (Figure 3). Su et al. (2016) have raised the scope of PDA by developing a simple shaking-assisted method to produce roughened polydopamine (rPDA) coatings at a variety of substrates. In the absence of an external antibacterial agent, the projected rPDA coatings displayed significantly enhanced antibacterial activity against Gram-positive and Gram-negative bacteria. Patel et al. (2018) have revealed that PDA coatings using different buffers could help in manipulating antibacterial activities, since the selection of the buffer could control the percentage of a particular functional group present in that PDA coating. Tris and sodium hydroxide-mediated PDA coating exhibited higher antibacterial activity as compared to that obtained using sodium bicarbonate and phosphate-buffered saline (PBS), which might be due to the presence of abundant hydroxyl groups on the surface of the coating. The findings of the study outlined the effect of different chemistries on the morphology and physicochemical properties of the PDA coatings that ultimately affected the antibacterial or antifouling properties. Zhou et al. have also suggested an alternative route to expedite PDA coating on the surfaces. High temperature with rapid shaking for 30 min produced the coated surfaces similar to properties exhibited by the surfaces coated for 24 h (Zhou et al., 2014).
Polydopamine has been observed to possess redox-dependent properties, which make this material to perform as an antioxidant as well as a pro-oxidant. It can accept or donate electrons repeatedly to exhibit beneficial radical-scavenging properties as well as to generate ROS, which account for its antimicrobial properties (Liu and Huang, 2016; Lakshminarayanan et al., 2018; Liu et al., 2019). Its chemistry involves two electron transfer between quinone and hydroquinone structures. Catecholic moieties of PDA are responsible for donating electrons to oxygen molecule to generate hydrogen peroxide, which subsequently produces hydroxyl radical advocating the localized and instant antibacterial activity. These properties are greatly influenced by the presence of metal ions and near-infrared (NIR) irradiation. The series of reactions that occur during electron transfer (respiratory chain) is important for bacterial growths as well as PDA redox state, as respiratory chain inhibitors have been illustrated in Figure 4. Mechanistically, in situ formation of different forms of polydopamine acts as pro-oxidant/antioxidant owing to acceptance of electrons (e–) from the environmental oxygen and vice versa. The oxidized form of PDA attained by metal binding (Ag+, Au3+, Pt4+, Cu2+) inflates the generation of quinone moieties and affinity for binding other biomolecules to enhance microbial inhibitory effect (Liebscher, 2019). Electron-donating behavior of PDA produces detrimental pro-oxidant effects on bacterial survival via generation of reactive oxygen species (ROS) via conversion of environmental oxygen (O2) to superoxide radical (O2–). Generated ROS expedite the activity of superoxide dismutase (SOD; metalloenzyme), thus forming H2O2, which further produces OH. Combined effects of hydroxyl ion and the presence of NADH on Fe–S cluster along with cofactor FMN enrich bacterial cellular membrane with quinones (Quinone pool) (Larosa and Remacle, 2018). Synthesized quinone facilitates the formation of heme b and heme o3 for survival of prokaryotes. The transfer of electrons is an essential pathway for cellular respiration within microbes, especially bacteria. Herein, the pro-oxidant effect of PDA escalates ROS production and disrupts the chain of Fe–S cluster, thus leading to inhibition of formation of quinone and thus famine quinone pool. Irradiation and metal binding also alter the redox state behavior of PDA for exhibiting antimicrobial properties. PDA nanocoating exhibits a broad absorption range from visible to NIR, which can be tailored for various photothermal behaviors (Zou et al., 2020). The study by Lei et al. (2016) has reported that this PDA nanocoating possesses very high photothermal conversion properties upon NIR irradiation, which leads to the killing of all local microbes independently on genus. Medical-device-associated infections have been drastically increasing in the current scenario. Surface functionalization via altering surface topography (surface roughness/wettability/surface energy), chemical modification (smart surfaces), and multifunctional surfaces (nanoform layer/antimicrobial coating using antibiotics, antimicrobial peptides: AMPs) help in combating microbial adhesion using different pathways, i.e., contact killing mechanism, electrostatic repulsion between surface and bacterial cell, rupturing cellular membrane by nanopillars, hydrophilicity block action of bacterial cell adhesins (proteins, flagella, pilli), cationic/zwitterionic moieties repel negative charge strains and bind to + ve charge strain, thus rupturing cell membrane leading to leakage of cellular matrix (Ghilini et al., 2019).
Alteration in the topographic pattern of various substrates (glass, polycarbonate, implants, pristine, etc.) helps in mitigating microbial adhesion, i.e., biofilm formation. This approach is well suited for coating medical implants and biomedical devices to combat microbial-associated infections. Nowadays, compromised surface geometry allows wide range of microbes for the attachment. Adhered microbes attract other microbial community for biofilm formation, and fouling tendency also supports biofilm formation. A recent study by Khanzada et al. revealed that cross-linked PDA-GO functionalized RO membranes exhibited bactericidal and antifouling properties. They demonstrated that hydrophilic behavior and smooth edges of the surface hindered bacterial adhesion and long-term survival. The rationale behind this outcome was the negative charge on the surface due to the presence of COOH, leading to electrostatic repulsion between the surface and Gram-negative strain and PDA-GO imparted synergistic effects on bacterial cellular damage via ROS production (Khanzada et al., 2020). In another study, an increase in surface roughness and hydrophilicity during surface modification with PDA-functionalized adhesions have been shown as important factors that expedite the binding of bacteria onto surfaces (Su et al., 2016); however, positively charged functional moieties in PDA cause lysis. Likewise, cationic polymer (chitooligosaccharide)-conjugated PDA-polyurethane (PDA-PU) membranes have shown significantly improved antibacterial activity as compared to PDA-PU membranes, which showed marginal enhancement in the properties over native PU membranes (Luo et al., 2017). In yet another report, Ahmed et al. have demonstrated the importance of hydrophilic behavior of PDA-functionalized polysulfone (PSF) substrate enriched with hydrophilic natural organic matter (NOM) for combating microbial adhesion. Herein, microbial adherence has been blocked by inhibiting interaction between cell surface adhesins, i.e., pilli and flagella and PDA-functionalized surface (Ahmed et al., 2018). A summary of the important PDA-based systems along with their mechanism of action on the microbes is depicted in Table 1.
Table 1. Summary of some of the polydopamine (PDA)-associated nanostructures and their bacterial inhibitory mechanism.
Chemical moieties used for surface modifications such as cationic polymers, antimicrobial peptides (AMPs), zwitterionic polymers, and quaternary ammonium compounds (QAC) have been employed to introduce antifouling, antimicrobial, and antibiofilm properties, and the resulting surfaces have been used for combating device-associated infections (Ghilini et al., 2019). Gram-negative bacterial strains specifically adhere to cationic surfaces for longer time period, leading to penetration of bioactive molecules into the cell membrane via different transporter and porins. These frontrunners advocate for hindering series of metabolic activities essential for bacterial survival. On the other hand, Gram-positive bacterial community repels surfaces due to electrostatic repulsion, thus unable to promote biofilm formation. PDA has been proven as a versatile biopolymer for surface functionalization in conjugation with various positively charge compounds, as shown by Yao et al. (2018) in the fabrication of zwitterionic polysulfone surface to mitigate biofouling process and microbial adhesion. The approach helped in designing and developing smart surfaces that could inhibit biofilm formation, thus decreasing biofilm-associated infections. A recent study by Zhou et al. scrutinized the activity of cocktail functional polymers, cationic monomer, and cross-linker deposited on the catheter surface. These combinations inflated surface hydrophilicity as well as cationic charge, expediting biofilm inhibition (Zhou et al., 2018).
A review by Jia et al. (2019) has mentioned about the role of PDA-based coatings to improve the antimicrobial and osseointegration of orthopedic implants. The adhesive character of PDA has been used to attach the antimicrobial enzyme lysostaphin, covalently, to various surfaces to produce antibacterial and antibiofilm interfaces (Yeroslavsky et al., 2015). An easy, two-step, shaking-assisted PDA coating technique has been suggested to create antimicrobial polypropylene (PP) mesh having the capability to produce H2O2 (Forooshani et al., 2019). The coating was found to be more effective against Gram-negative bacteria, while Gram-positive bacteria showed a resistance. However, the generation of a higher amount of H2O2 as well as longer exposure time was found to be enough to destroy Gram-positive bacteria. Aminoglycoside conjugates of PDA have been evaluated for antimicrobial potency against different bacterial strains (clinical as well as resistant ones) e.g., PDA–kanamycin, PDA–gentamicin, and PDA–neomycin nanoconjugates (Singh et al., 2020). PDA deposited onto the polydimethylsiloxane (PDMS) surface has been used to tether synthetic antimicrobial cysteinylated tryptophane–arginine-rich peptide (CWFWKWWRRRRR-NH2) (CWR11) that displayed antimicrobial functionality over 21 days on catheter-relevant surfaces to combat catheter-associated urinary tract infections (Lim et al., 2015). Similarly, immobilization of liposomal amphotericin B (LAmB) on a PDA-coated PDMS surface, a material commonly used in the manufacturing of urinary catheters, was shown to impart the ability to resist Candida albicans colonization (Alves et al., 2019).
Antifouling surfaces composed of a zwitterionic copolymer, made up of 2-methacryloyloxyethyl phosphorylcholine and dopamine methacrylamide followed by covalent codeposition on polyethylenimine (PEI)/PDA surfaces, showed antibacterial activity against Gram-negative and Gram-positive bacteria along with adsorption resistance to bovine serum albumin after in situ deposition of AgNPs (Asha et al., 2018).
A material surface, composed of PDA-coated Fe3O4 nanoparticles followed by covalent tethering of barbituric acid, has been developed. Postreaction with sodium hypochlorite generated N-halamine groups on the imide functions of the barbituric acid, and the resulting surface displayed excellent antimicrobial activity against both Gram-positive and Gram-negative bacteria. These bifunctional nanoparticles with excellent antibacterial as well as magnetic properties not only destroyed targeted bacterial colonies but could also be recovered by applying an external magnetic field (Akter et al., 2018). In a similar type of study, N-halamine functions have been generated on PDA surface, and the resulting surface has showed significantly higher antibacterial activity toward Gram-positive and Gram-negative bacteria within a brief contact time as compared to the activity displayed by the only PDA-coated substrate (Chien et al., 2020).
The contribution of PDA to provide stability as an adhesive and antibacterial activity has been validated during evaluation of PDA/alginate/Fe3O4 hydrogel beads. The coating of PDA on to Alg/Fe3O4 beads not only significantly increased the stability in different pH, hydrophilicity, and elasticity but also performed strongly against bacterial strains (Matai et al., 2019). These beads have been demonstrated to be used repeatedly as antibacterial agents.
Polydopamine coatings have been employed on the surface of satellite telemetry tags, with coating conditions tailored to generate varying amounts of hydrogen peroxide. The coating displayed the ability to diminish the adhesion of E. coli and Psychrobacter cryohalolentis and, therefore, has the potential to decrease the chances for tissue infection at the tag implant site (Tyo et al., 2019).
Nitric oxide at low concentration affects cellular signaling within biofilms. Park et al. (2016) and Adnan et al. (2018) have reported PDA nanoparticles with diazeniumdiolate groups that could deliver nitric oxide (NO) for antibacterial therapy with negligible toxicity. A technology, using catecholamine to coat surfaces of body-implantable materials having diazeniumdiolate groups that could supply NO in vivo and that could be used without triggering cytotoxicity, has been patented (Kim et al., 2017). Further, PDA films with low-fouling and NO-releasing capabilities containing both diazeniumdiolate and polyethylene glycol (PEG) functionalities with NO release capability to over 48 h have been reported and showed the ability to inhibit the attachment of a multidrug-resistant strain and efficiently destroy the biofilm (Sadrearhami et al., 2019). Since catechol groups in PDA provide the ability to coordinate metal ions, Cu2+-loaded PDA coatings were able to catalyze NO release (Zhou et al., 2020).
The mechanism suggested in the synthesis of PDA/copper-doped calcium silicate (Cu-CaSil) bioactive hydrogel validated the coordination between PDA and Cu2+ ions. The projected complex showed enhanced photothermal performance of the hydrogel as observed by mass extinction coefficient profile and improved bioactivity. Laser irradiation of this hydrogel showed an excellent antibacterial activity. Stronger NIR absorption has been attributed to the presence of PDA polymer and Cu-CaSil powder, two hydrogel components, both possessing photothermal property, and the right PDA/Cu ratio leads to a number of tetracoordinated structures in PDA–Cu complex responsible for enhancing absorption intensity. Since heat treatments are known to kill bacteria, the photothermal effect of the composite hydrogel PDA/Cu-CaSil with synergistic antibacterial function of Cu2+ ions created unique “hot ions effect” by heating Cu2+ ions through laser irradiation (Xu et al., 2020). Further, Cu/Ag/PDA/PET fabrics have been shown to possess good antibacterial property against E. coli (∼99%) due to the adhesive ability of PDA and its strong binding to Cu NPs. The self-polymerization of dopamine under alkaline conditions forming PDA nanoparticles assisted a change in morphology of PET fabrics. The catechol moieties and amine functional groups in PDA reduced Ag+ to Ag NPs that were fastened on fiber surface via chelation between PDA and Ag+ ions. These Ag NPs, as catalytic seeds, facilitated deposition of Cu NPs on the surface of fabrics through chemical copper plating. Thus, PDA, functioning as template, expedited Cu NPs deposition onto the surface of fabric that displayed killing of most of the adhered bacteria (Wang K. et al., 2020).
The antimicrobial activity of Ag implants is mainly attributed to binding of Ag+ to thiol groups present in bacterial enzymes. Its use has been exploited with many PDA-assisted coatings (Yun’an Qing et al., 2018). Ag-coated PDA microspheres have been used to kill Staphylococcus aureus cells due to the elevated ROS level (Guo et al., 2019). The abundance of catechol and amine groups on the surface of PDA particles are the active sites for in situ reduction in the silver precursors, and the formed AgNPs can be fixed there; Wu C. et al. (2015) used [Ag(NH3)2]+ ions as a precursor for polydopamine-assisted electroless Ag metallization, which showed an excellent antibacterial performance against Escherichia coli and S. aureus.
The PDA coatings incorporated with Ag ions illustrate an attractive approach, as the combination facilitates interactions with biological system without cytotoxicity and exhibits antibacterial capability as well. The synergistic effects between PDA coating and AgNPs have been highlighted in a study based on X-ray photoelectron spectroscopy (XPS) and Fourier-transform infrared spectroscopy (FTIR) analysis by Niyonshuti et al. (2020). The study indicated that the PDA coating becomes thicker as the PDA deposition time is increased. The coordination between Ag and catechol groups on the PDA coating was found to significantly increase the potency of AgNPs against E. coli. Thus, a PDA coating is observed to play a significant role in enhancing the antimicrobial properties of AgNPs. The result indicated that catechol-rich PDA coating resulted in an increased ROS generation and significant damage to the bacterial membrane. The reducing catechol groups of PDA have further been explored to form well-dispersed AgNPs on different substrates for controlling biofouling and as antimicrobial reverse osmosis membranes, which, in turn, expose the potential of PDA to modify surface chemistries and morphological features of the membranes (Tang et al., 2015; Yang et al., 2016). Since the catechol groups in the PDA films chelate with Ag, the in situ reduction in Ag+ to Ago and binding of Ago to N- and O-sites in PDA film produce seed precursor, which helps in building up of AgNPs on the central venous catheters (CVCs) surface, demonstrating significant antimicrobial potency and appropriate biological safety (Wu K. et al., 2015).
A bio-nanocomposite coating using the mycogenerated AgNPs and PDA has been reported to display antibiofilm activity on biofilms of multidrug-resistant A. baumannii used in central venous catheter (Neethu et al., 2020). AgNPs formed by reduction through catechol have been reported not to be sensitive to oxygen and so have a longer-lasting antimicrobial effect (Wu H. et al., 2018). Zhang et al. (2019) combined the antimicrobial effect of three materials to boost the potential of bio-coated PLA-Au@PDA@Ag nanofibers. The large surface area of the AuNPs in the fibers facilitated the enhancement of the cell penetration with simultaneous production of oxidative stress by the biological system, thereby exhibiting high antibacterial activity. PDA, with its antibacterial effects, too, acted as a binder for different interfaces with effective anchoring groups.
Metal-containing PDA-NPs are suggested to be highly microbicidal and display effective antibiofilm activity (Yeroslavsky et al., 2016). Besides this, sonochemically synthesized Ag–PDA NPs and Cu/Ag–PDA hybrid NPs with copper shell and Ag core have been demonstrated to convert the surface to antibacterial. The projected system also gets benefited from the partial contribution to the antimicrobial activity from the stable PDA-semiquinone and ROS generated by the metal–PDA NPs under physiological conditions as suggested by Yeroslavsky et al. (2016). The synergistic actions of two or more antibacterial drugs as nanohybrids have been established to be effective in the treatment of refractory bacterial infections. The colistin-loaded PDA nanospheres decorated with silver nanodots are reported by Ran et al. (2020) to exhibit powerful antibacterial and antibiofilm effects.
The metal-ion-reducing ability of catechol in PDA with consequently depositing the Ag nanoparticles has been utilized to support antibacterial coatings for poly(ether ether ketone) (PEEK) implants. The PEEK–PDA–Ag implant was reported to inhibit growth of E. coli and S. aureus on the surface and the surrounding bone tissue compared to PEEK without affecting osseointegration (Gao et al., 2017). Further, PDA and silk fibroin have been introduced to the porous PEEK surface to balance the biocompatibility and antibacterial ability of PEEK implant by Yan et al. (2018). The study reported a first time PDA-assisted in situ growth of AgNPs and immobilization of silk fibroin (SF)/gentamicin sulfate (GS) coating upon porous PEEK surface. The dual application of PDA, reducing Ag+ into AgNPs followed by firm fastening onto PEEK surface and biocompatibility serving osteogenesis, was presented as a synergistic bacteria killing ability in AgNP-incorporated SF/GS coating constructed upon porous PEEK surface (Yan et al., 2018). PDA synthesized in one step is reported by Cai et al. (2017) to act as both a metal ion chelating as well as reducing agent to synthesize, in situ, AgNPs on the sericin/poly(vinyl alcohol) (PVA) composite film, which displayed long-standing antibacterial activities. In another study, Liu M. et al. (2018) have demonstrated prominent antibacterial activity of a porous thermoplastic polyurethane (TPU) membrane coated with PDA NPs followed by a layering of nanosilver and its use as a profound antibacterial invasion dressing (i.e., wound dressing) with high biocompatibility for wounds caused by clinical and antibiotic-resistant bacteria. Using mussel-inspired PDA coating technology, a scaffold (PP–PDA–Ag–COL) was generated by Qian et al. in a multistep process. An electrospun poly (lactide-co-glycolide) (PLGA)/poly(epsilon-caprolactone) (PCL) matrix was first coated with PDA NPs followed by coating with AgNPs via in situ reduction to incorporate antibacterial and osteogenic properties. The resulting PP–PDA–Ag matrix was then coated with type I collagen (COL) to further improve the properties including biocompatibility of the scaffold. Collagen I coating not only enhanced the cytocompatibility but also resulted in the consistent release of silver ions over more extended periods. The tailored PP–PDA–Ag–COL structure maintained the three-dimensional interfiber architecture of the scaffold (Qian et al., 2019). The emergence of PDA thin layer on the AgNPs, which exhibit cytotoxicity, has been presented to be highly biocompatible with almost no toxic effects on the cells and that too without compromising on the optical characteristics (Yilmaz, 2020). Various groups have also developed diversified types of PDA-coated surfaces; for instance, PDA-dyed hair prevented the scalp from bacterial infection. Likewise, PDA-coated cotton fabric, on treatment with quaternized nanosilica followed by hexadecyltrimethoxysilane, produced a superhydrophobic and excellent antibacterial material with good washable properties (Song et al., 2019). The design and development of antimicrobial contact lenses is another area where PDA-based coatings have been tested against bacterial adhesion and formation of biofilm. Dhand et al. examined the covalent and non-covalent interactions of antimicrobial agents with PDA coating on contact lenses. The results showed that surface properties of the lenses remained unaffected, and the coating displayed excellent antimicrobial activity to prevent biofilm formation for longer duration without any side effects on ocular cells (Dhand et al., 2020). The current state of the art for polydopamine research has revealed PDA as a smart adhesive biopolymer that has the potential to deal with diversified medical as well as environmental challenges.
The incorporation of antimicrobial agents on otherwise corrosion-resistant Ti surfaces is one of the effective modifications to enhance the biological properties of the implants, especially useful for orthopedic implant applications. The study on Ti–PDA–cefotaxime sodium (Ti–PDA–CS) substrates by He et al. indicated that the CS retained its biological activity even after immobilization onto the surface. The conjugated surface exhibited excellent in vitro antibacterial activity (He et al., 2014). The combination of PDA with hydroxyapatite (HA), a naturally occurring mineral form, with the formula Ca10(PO4)6(OH)2, calcium-phosphate (CaP)-based material, imparts good mechanical strength as well as corrosion resistance on the surface of implant materials. The formation of CaP biominerals onto the PDA-coated surface grown by layer-by-layer model brings morphological change emphasizing strong affinity between CaP and PDA moieties. The chemistry in PDA-HA coatings works well for antibacterial resistance. A review on functionalization of PDA-HA with applications toward bone–tissue engineering has highlighted the antimicrobial resistance (Kaushik et al., 2020). In vitro studies of a hybrid coating composed of bioactive species, hydroxyapatite (HA)/chitosan (CS)/Ag matrix, an organic–inorganic hybrid, onto the surface of a PDA-modified Ti implant could control the release of silver ion for good self-antibacterial execution as well as good osteoinductive ability. The catechol groups of PDA are supported to accelerate the formation of HA crystals and bridge the binding strength between HA and Ti substrate (Li et al., 2016). PDA-Ag coating on Ti surface has also been testified by Choi et al. (2019) to inhibit the formation of biofilm, microbial colonization, and pathogenesis of gum disease in the mouth, i.e., retardation of microbial growth. Furthermore, CS/AgNPs coating has been reinforced by employing PDA as an intermediate bridging layer on the urinary catheter and Ti surfaces, a simple immersion method in acid solution that is reported to exhibit phenomenal refinement in preventing bacterial adhesion. The availability of hydroxyl groups in chitosan (CS) molecules to reinforce antimicrobial AgNPs and control the Ag ions release besides strong attachment by PDA worked for a durable and efficient antibacterial coating (Wang B.B. et al., 2020).
The PDA acts as bonding glue for coating metal ions on the substrates. Cu(II) ions immobilization through PDA chelation on Ti surface for implant materials is reported to display antibacterial property (He et al., 2015). Wang et al. evaluated the antibacterial activity and osseointegration performance of Cu-deposited Ti substrates with PDA coating in the presence of bacterial infection both in vitro and in vivo. The Cu2+ ions affixed through coordination interaction inside the PDA coating showed sustained release of the metal ions. It was proposed that the released Cu2+ ions could effectively inhibit the bacteria in contact with the Ti–PDA–Cu substrate surfaces (Wang L. et al., 2017). Besides metal ion–PDA chemistry, there is interest to validate the effect of concentration of various metal ions on the antibacterial effect of Ti implants induced as self-assembled layer of adhesive PDA. The bacterial viability has been found to be inversely correlated with the ion concentration gradient of divalent Cu2+, Zn2+, and Sr2+ metal ions on PDA-coated Ti implant surface. The coated Ti implants may leach these ions to produce positive antibacterial efficacy. The chemical binding of metal ions with PDA has been suggested to control the release rate of the ion coating. Even though a strong Zn–PDA coordination bond in Zn coating slowed the release rate compared to Sr coating at the same concentration, 2% Zn coating and 10% Sr coating could inhibit bacterial growth of bacteria without any toxic effect on cells. The controversial results regarding the antibacterial activities of the PDA coatings suggest that the mechanism of bactericidal properties is still obscure (Kao et al., 2019).
The covalent adhesion and chelating talent of PDA have been exploited with bioactive and biocompatible titania coatings, TiO2, on Ti substrate and AgNPs, respectively, by Jia et al. (2016) for orthopedic coatings. Gao et al. also presented in vitro and in vivo bactericidal and antibiofilm activities of TiO2–PDA–Ag coating. The comparison in Ag release kinetics between the TiO2–Ag (without PDA) coatings and TiO2–PDA–Ag coating on Ti implants by electrochemical anodization synthesis showed a burst release in the former and a controlled-release pattern in the latter. Larger reduction of bacterial growth with overproduction of ROS observed in TiO2–Ag coating was recommended for the burst release, but the balance of cytotoxicity and the antibacterial effects lied in TiO2–PDA–Ag coatings (Gao et al., 2019). On the other hand, Guan et al. (2019) reported more durable and efficient antibacterial property in Ag–TiO2@PDA nanorods (NRDs) by hydrothermal synthesis than that of AgTiO2 NRDs, based on the synergistic effect of selective physical punctual and controlled release of silver ions. The ability of PDA to effectively transfer photoinduced electrons and protons and improve the photocatalytic activity has been applied in a three-dimensional Ag/TiO2/PDA nanofilm. PDA, as transformation interface, could support the photocatalytic mechanism to generate some ROS such as hydroxyl radical, hydrogen peroxide, and superoxides, which could cause bacterial inactivation. The study supported the future aspect of intermediate PDA layer as a favorable antibacterial coating on a wide variety of substrates such as plastic glass and metal alloy (Wen et al., 2020). Yuan et al. (2019) designed a functional MoS2/PDA–Arg–Gly–Asp–OH coating on Ti implants to inhibit in situ bacterial infection and mend osseointegration.
The material surface analyses of PDA onto the zirconia surface showed significant increase in cell adhesion and proliferation as compared with pristine zirconia. The coating suggested as peri-implant soft-tissue integration influenced human gingival fibroblasts and decreased adherent bacteria (Liu M. et al., 2015).
Silver nanoparticles developed using PDA coatings on rod-like mesoporous silica (SBA-15) gave SBA-15/PDA/Ag nanocomposites, which exhibited prolonged inhibitory effect on the growth of E. coli, S. aureus, and Aspergillus fumigatus (Song et al., 2018). A bioinspired coating with dual functioning antimicrobial, due to PDA and Cu2+ ions with a coating of zwitterionic sulfobetaine, via aza-Michael addition reaction, has been tested on commercial silicone-based urinary catheters (Fan et al., 2018). The hydrophobic–hydrophilic character, due to presence of amino, hydroxyl, and phenyl functions, good degradability, and antimicrobial properties of PDA have been exploited by Liu C. et al. (2018) to develop PDA-coated amorphous silica nanoparticle for hemorrhage control. PDA/SiNP displayed promising character for aggregating cells and inducing clotting. Compared to the commercial formulation, Celox, these coated nanoparticles shortened the blood clotting time to 150 s. These particles were found to achieve adequate hemostasis by accelerating coagulation and reducing blood loss during femoral artery and vein injury. PDA/SiNPs exhibited antimicrobial activity even for a longer duration with high hemocompatibility.
A PDA layer coating, synthesized by the H2O2/horseradish peroxidase method, has been found to reduce the cytotoxicity of carbon nanotubes (CNTs), enhance their dispersion, and endow better broad-spectrum photothermal antimicrobial activities in a gelatin-grafted dopamine/chitosan composite hydrogel (Liang et al., 2019). A PDA-based colloidal material synthesized through one-step dopamine polymerization by nitrogen-doped carbon dots has been reported to show an antibacterial effect against S. aureus through microorganism entrapment/ROS generation (Maruthapandi et al., 2019). The antibacterial activity of bio-photocatalyst on PDA-graphitic carbon nitride (g-C3N4) with uniformly dispersed AgNPs has been described (Wu Y. et al., 2018). Liao et al. used graphene oxide to form antibacterial Ag–PDA–RGO nanocomposites and evaluated their antimicrobial performance against Gram-positive and Gram-negative bacteria. The projected nanocomposites not only exhibited excellent antimicrobial activity but also opened newer avenues for a wide range of modern biomedical applications (Liao et al., 2019). Similarly, MoS2–PDA–Ag nanosheets demonstrated good antibacterial activity and eradicated S. aureus biofilms and wound infections (Yuwen et al., 2018).
This article makes a feature of the antibacterial role of PDA and the recent developments in the biomedical fields. The proficiency in robust adhesion of this polymer inspired by a structure similar to DOPA present in the amino acid sequence of mussel foot protein appeals to the researchers to try various substrates for surface modification. The self-polymerization of dopamine (DA) is still a mystery, but it has not stopped work on its functionalization, reacting quickly with amine and thiol-containing moieties.
The strong metal-chelating, covalent cross-linking, and redox capabilities have made it an innovative coating. Various forms of PDA nanomaterials, viz., NPs, microcapsules, and PDA hybrid nanospheres, hydrogels, and nanocomposites applicable for cell interfacing, biosensing, drug delivery, and tissue engineering, have been synthesized. As discussed for antimicrobial activities, PDA quandaries the cell membrane and barricades the cell surface, preventing diffusion of nutrients and wastes inside/out of the cell cytosol, leading to cell lysis. The positive charge on the functional groups in PDA is also responsible for lysis by contacting the bacterial cell wall.
The antimicrobial activity of PDA could be attributed to the presence of catechol, which forms semiquinone and quinones that get auto-oxidized in the presence of oxygen, generating ROS and subsequently preventing the growth of bacteria. However, the basis of different responses to different bacteria is still unclear. In some of the nanohybrid systems, the PDA role is restricted to stabilization of the adhesion of antimicrobial films or control the release of antimicrobial metal ions. PDA itself is being known for antibacterial activity due to regulated production of H2O2 during auto-oxidation; hence, optimization in its release is desired.
The versatile chemistry of biocompatible PDA warrants its study in medically relevant materials with or without passive and active agents, which prevent microbial biofilm formation and thus hold great potential for growth for diverse applications.
IS and SG compiled the manuscript. GD helped in editing and compilation. PK conceived the idea, edited, and supervised the task to completion. All authors contributed to the article and approved the submitted version.
The authors declare that the research was conducted in the absence of any commercial or financial relationships that could be construed as a potential conflict of interest.
Adnan, N. N. M., Sadrearhami, Z., Bagheri, A., Nguyen, T. K., Wong, E. H., Ho, K. K., et al. (2018). Exploiting the versatility of polydopamine-coated nanoparticles to deliver nitric oxide and combat bacterial biofilm. Macromol. Rapid Commun. 39:1800159. doi: 10.1002/marc.201800159
Ahmed, S. B., Hasane, A., Wang, Z., Mansurov, A., and Castrilloìn, S. R. V. (2018). Bacterial adhesion to ultrafiltration membranes: role of hydrophilicity, natural organic matter, and cell-surface macromolecules. Environ. Sci. Technol. 52, 162–172. doi: 10.1021/acs.est.7b03682
Ahn, B. K. (2017). Perspectives on mussel-inspired wet adhesion. J. Am. Chem. Soc. 139, 10166–10171. doi: 10.1021/jacs.6b13149
Akter, N., Chowdhury, L., Uddin, J., Ullah, A. A., Shariare, M. H., and Azam, M. S. (2018). N-halamine functionalization of polydopamine coated Fe3O4 nanoparticles for recyclable and magnetically separable antimicrobial materials. Mater. Res. Exp. 5:115007. doi: 10.1088/2053-1591/aadc56
Alfieri, M. L., Micillo, R., Panzella, L., Crescenzi, O., Oscurato, S. L., Maddalena, P., et al. (2017). Structural basis of polydopamine film formation: Probing 5, 6-dihydroxyindole-based eumelanin type units and the porphyrin issue. ACS Appl. Mater. Inter. 10, 7670–7680. doi: 10.1021/acsami.7b09662
Alfieri, M. L., Panzella, L., Oscurato, S. L., Salvatore, M., Avolio, R., Errico, M. E., et al. (2018). The chemistry of polydopamine film formation: The amine-quinone interplay. Biomimetics 3:26. doi: 10.3390/biomimetics3030026
Alves, D. F., Vaz, A. T., Grainha, T., Rodrigues, C. F., and Pereira, M. O. (2019). Design of an antifungal surface embedding liposomal amphotericin B through a mussel adhesive-inspired coating strategy. Front. Chem. 7:431. doi: 10.3389/fchem.2019.00431
Ang, J. M., Du, Y., Tay, B. Y., Zhao, C., Kong, J., Stubbs, L. P., et al. (2016). One-pot synthesis of Fe (III)–polydopamine complex nanospheres: Morphological evolution, mechanism, and application of the carbonized hybrid nanospheres in catalysis and Zn–air battery. Langmuir 32, 9265–9275. doi: 10.1021/acs.langmuir.6b02331
Asha, A. B., Chen, Y., Zhang, H., Ghaemi, S., Ishihara, K., Liu, Y., et al. (2018). Rapid mussel-inspired surface zwitteration for enhanced antifouling and antibacterial properties. Langmuir 35, 1621–1630. doi: 10.1021/acs.langmuir.8b03810
Bai, Y., Zhang, B., Chen, L., Lin, Z., Zhang, X., Ge, D., et al. (2018). Facile one-pot synthesis of polydopamine carbon dots for photothermal therapy. Nanoscale Res. Lett. 13, 1–9.
Ball, V. (2017). Composite materials and films based on melanins, polydopamine, and other catecholamine-based materials. Biomimetics 2:12. doi: 10.3390/biomimetics2030012
Ball, V. (2018). Polydopamine nanomaterials: Recent advances in synthesis methods and applications. Front. Bioeng. Biotechnol. 6:109. doi: 10.3389/fbioe.2018.00109
Bao, X., Zhao, J., Sun, J., Hu, M., and Yang, X. (2018). Polydopamine nanoparticles as efficient scavengers for reactive oxygen species in periodontal disease. ACS Nano 12, 8882–8892. doi: 10.1021/acsnano.8b04022
Barclay, T. G., Hegab, H. M., Clarke, S. R., and Ginic-Markovic, M. (2017). Versatile surface modification using polydopamine and related polycatecholamines: Chemistry, structure, and applications. Adv. Mater. Interf. 4:1601192. doi: 10.1002/admi.201601192
Batul, R., Tamanna, T., Khaliq, A., and Yu, A. (2017). Recent progress in the biomedical applications of polydopamine nanostructures. Biomater. Sci. 5, 1204–1229. doi: 10.1039/c7bm00187h
Cai, R., Tao, G., He, H., Song, K., Zuo, H., Jiang, W., et al. (2017). One-step synthesis of silver nanoparticles on polydopamine-coated sericin/polyvinyl alcohol composite films for potential antimicrobial applications. Molecules 22:721. doi: 10.3390/molecules22050721
Chen, M., Wen, Q., Gu, F., Gao, J., Zhang, C. C., and Wang, Q. (2018). Mussel chemistry assembly of a novel biosensing nanoplatform based on polydopamine fluorescent dot and its photophysical features. Chem. Eng. J. 342, 331–338. doi: 10.1016/j.cej.2018.02.099
Chien, H.-W., Chiu, T.-H., and Stable, N. - (2020). halamine on polydopamine coating for high antimicrobial efficiency. Eur. Polym. J. 130:109654. doi: 10.1016/j.eurpolymj.2020.109654
Choi, S.-H., Jang, Y.-S., Jang, J.-H., Bae, T.-S., Lee, S.-J., and Lee, M.-H. (2019). Enhanced antibacterial activity of titanium by surface modification with polydopamine and silver for dental implant application. J. Appl. Biomater. Funct. Mater. 17:2280800019847067.
d’Ischia, M., Napolitano, A., Ball, V., Chen, C. T., and Buehler, M. J. (2014). Polydopamine and eumelanin: From structure–property relationships to a unified tailoring strategy. Acc. Chem. Res. 47, 3541–3550. doi: 10.1021/ar500273y
Deng, Y., Yang, W.-Z., Shi, D., Wu, M., Xiong, X.-L., Chen, Z.-G., et al. (2019). Bioinspired and osteopromotive polydopamine nanoparticle-incorporated fibrous membranes for robust bone regeneration. NPG Asia Mater. 11, 1–13.
Dhand, C., Ong, C. Y., Dwivedi, N., Varadarajan, J., Halleluyah Periayah, M., Jianyang Lim, E., et al. (2020). Mussel-inspired durable antimicrobial contact lenses: The role of covalent and noncovalent attachment of antimicrobials. ACS Biomater. Sci. Eng. 6, 3162–3173. doi: 10.1021/acsbiomaterials.0c00229
Ding, Y. H., Floren, M., and Tan, W. (2016). Mussel-inspired polydopamine for bio-surface functionalization. Biosurf. Biotribol. 2, 121–136. doi: 10.1016/j.bsbt.2016.11.001
Dong, Z., Feng, L., Hao, Y., Chen, M., Gao, M., Chao, Y., et al. (2018). Synthesis of hollow biomineralized CaCO3–polydopamine nanoparticles for multimodal imaging-guided cancer photodynamic therapy with reduced skin photosensitivity. J. Am. Chem. Soc. 140, 2165–2178. doi: 10.1021/jacs.7b11036
Dong, Z., Gong, H., Gao, M., Zhu, W., Sun, X., Feng, L., et al. (2016). Polydopamine nanoparticles as a versatile molecular loading platform to enable imaging-guided cancer combination therapy. Theranostics 6:1031. doi: 10.7150/thno.14431
Fan, Y.-J., Pham, M. T., and Huang, C.-J. (2018). Development of antimicrobial and antifouling universal coating via rapid deposition of polydopamine and zwitterionization. Langmuir 35, 1642–1651. doi: 10.1021/acs.langmuir.8b01730
Feng, J., Xu, Z., Luo, D., and Liu, X. (2020). Multiplexed imaging with coordination nanoparticles for cancer diagnosis and therapy. ACS Appl. Bio Mater. 3, 713–720. doi: 10.1021/acsabm.9b01038
Forooshani, P. K., and Lee, B. P. (2017). Recent approaches in designing bioadhesive materials inspired by mussel adhesive protein. J. Polym. Sci. 55, 9–33. doi: 10.1002/pola.28368
Forooshani, P. K., Meng, H., and Lee, B. P. (2017). “Catechol redox reaction: reactive oxygen species generation, regulation, and biomedical applications,” in Advances in Bioinspired and Biomedical Materials, Vol. 1, eds Y. Ito, X. Chen, and Kang I-K (Washington, DC: ACS Symposium Series), 179–196. doi: 10.1021/bk-2017-1252.ch010
Forooshani, P. K., Polega, E., Thomson, K., Akream, M. S., Pinnaratip, R., Trought, M., et al. (2019). Antibacterial properties of mussel-inspired polydopamine coatings prepared by simple two-step shaking-assisted method. Front. Chem. 7:631. doi: 10.3389/fchem.2019.00631
Fruk, L., and Crocker, L. (2019). Flavin Conjugated polydopamine nanoparticles displaying light-driven monooxygenase activity. Front. Chem. 7:278. doi: 10.3389/fchem.2019.00278
Gao, C., Cheng, H., Xu, N., Li, Y., Chen, Y., Wei, Y., et al. (2019). Poly (dopamine) and Ag nanoparticle-loaded TiO2 nanotubes with optimized antibacterial and ROS-scavenging bioactivities. Nanomedicine 14, 803–818. doi: 10.2217/nnm-2018-0131
Gao, C., Wang, Y., Han, F., Yuan, Z., Li, Q., Shi, C., et al. (2017). Antibacterial activity and osseointegration of silver-coated poly (ether ether ketone) prepared using the polydopamine-assisted deposition technique. J. Mater. Chem. B 5, 9326–9336. doi: 10.1039/c7tb02436c
Ge, R., Lin, M., Li, X., Liu, S., Wang, W., Li, S., et al. (2017). Cu2+-loaded polydopamine nanoparticles for magnetic resonance imaging-guided pH-and near-infrared-light-stimulated thermochemotherapy. ACS Appl. Mater. Inter. 9, 19706–19716. doi: 10.1021/acsami.7b05583
Ghilini, F., Pissinis, D. E., Miñán, A., Schilardi, P. L., and Diaz, C. (2019). How functionalized surfaces can inhibit bacterial adhesion and viability. ACS Biomater. Sci. Eng. 5, 4920–4936. doi: 10.1021/acsbiomaterials.9b00849
Gu, G. E., Park, C. S., Cho, H.-J., Ha, T. H., Bae, J., Kwon, O. S., et al. (2018). Fluorescent polydopamine nanoparticles as a probe for zebrafish sensory hair cells targeted in vivo imaging. Sci. Rep. 8, 1–8.
Guan, M., Chen, Y., Wei, Y., Song, H., Gao, C., Cheng, H., et al. (2019). Long-lasting bactericidal activity through selective physical puncture and controlled ions release of polydopamine and silver nanoparticles–loaded TiO2 nanorods in vitro and in vivo. Int. J. Nanomed. 14:2903. doi: 10.2147/ijn.s202625
Guo, A., Mu, Q., Cai, A., and Wang, X. (2019). Mussel-inspired green synthesis of Ag-coated polydopamine microspheres for selective antibacterial performance. Micro Nano Lett. 14, 394–398. doi: 10.1049/mnl.2018.5357
Han, J., Park, W., Park, S.-J., and Na, K. (2016). Photosensitizer-conjugated hyaluronic acid-shielded polydopamine nanoparticles for targeted photomediated tumor therapy. ACS Appl. Mater. Inter. 8, 7739–7747. doi: 10.1021/acsami.6b01664
He, S., Zhou, P., Wang, L., Xiong, X., Zhang, Y., Deng, Y., et al. (2014). Antibiotic-decorated titanium with enhanced antibacterial activity through adhesive polydopamine for dental/bone implant. J. Royal Soc. Inter. 11:20140169. doi: 10.1098/rsif.2014.0169
He, T., Zhu, W., Wang, X., Yu, P., Wang, S., Tan, G., et al. (2015). Polydopamine assisted immobilization of copper (II) on titanium for antibacterial applications. Mater. Technol. 30(Suppl.6), B68–B72.
Ho, C.-C., and Ding, S.-J. (2014). Structure, properties and applications of mussel-inspired polydopamine. J. Biomed. Nanotechnol. 10, 3063–3084. doi: 10.1166/jbn.2014.1888
Holten-Andersen, N., Mates, T. E., Toprak, M. S., Stucky, G. D., Zok, F. W., and Waite, J. H. (2009). Metals and the integrity of a biological coating: The cuticle of mussel byssus. Langmuir 25, 3323–3326. doi: 10.1021/la8027012
Hong, S. H., Sun, Y., Tang, C., Cheng, K., Zhang, R., Fan, Q., et al. (2017). Chelator-free and biocompatible melanin nanoplatform with facile-loading gadolinium and copper-64 for bioimaging. Bioconjug. Chem. 28, 1925–1930. doi: 10.1021/acs.bioconjchem.7b00245
Hong, S., Kim, K. Y., Wook, H. J., Park, S. Y., Lee, K. D., Lee, D. Y., et al. (2011). Attenuation of the in vivo toxicity of biomaterials by polydopamine surface modification. Nanomedicine 6, 793–801.
Hong, S., Na, Y. S., Choi, S., Song, I. T., Kim, W. Y., and Lee, H. (2012). Non-covalent self-assembly and covalent polymerization co-contribute to polydopamine formation. Adv. Funct. Mater. 22, 4711–4717. doi: 10.1002/adfm.201201156
Hong, S., Wang, Y., Park, S. Y., and Lee, H. (2018). Progressive fuzzy cation-π assembly of biological catecholamines. Sci. Adv. 4:eaat7457. doi: 10.1126/sciadv.aat7457
Huang, N., Zhang, S., Yang, L., Liu, M., Li, H., Zhang, Y., et al. (2015). Multifunctional electrochemical platforms based on the Michael addition/Schiff base reaction of polydopamine modified reduced graphene oxide: Construction and application. ACS Appl. Mater. Inter. 7, 17935–17946. doi: 10.1021/acsami.5b04597
Huang, S., Liang, N., Hu, Y., Zhou, X., and Abidi, N. (2016). Polydopamine-assisted surface modification for bone biosubstitutes. BioMed. Res. Int. 2016, 1–9. doi: 10.1155/2016/2389895
Huang, Y., Liu, Z., Liu, C., Ju, E., Zhang, Y., Ren, J., et al. (2016). Self-assembly of multi-nanozymes to mimic an intracellular antioxidant defense system. Angew. Chem. Int. Ed. 55, 6646–6650. doi: 10.1002/anie.201600868
Jia, L., Han, F., Wang, H., Zhu, C., Guo, Q., Li, J., et al. (2019). Polydopamine-assisted surface modification for orthopaedic implants. J. Orthop. Translat. 17, 82–95. doi: 10.1016/j.jot.2019.04.001
Jia, Z., Xiu, P., Li, M., Xu, X., Shi, Y., Cheng, Y., et al. (2016). Bioinspired anchoring AgNPs onto micro-nanoporous TiO2 orthopedic coatings: trap-killing of bacteria, surface-regulated osteoblast functions and host responses. Biomaterials 75, 203–222. doi: 10.1016/j.biomaterials.2015.10.035
Jiao, L., Xu, Z., Du, W., Li, H., and Yin, M. (2017). Fast preparation of polydopamine nanoparticles catalyzed by Fe2+/H2O2 for visible sensitive smartphone-enabled cytosensing. ACS Appl. Mater. Inter. 9, 28339–28345. doi: 10.1021/acsami.7b10564
Kao, H., Chen, C. C., Huang, Y. R., Chu, Y. H., Csík, A., and Ding, S. J. (2019). Metal ion-dependent tailored antibacterial activity and biological properties of polydopamine-coated titanium implants. Surf. Coat. Technol. 378:124998. doi: 10.1016/j.surfcoat.2019.124998
Kaushik, N., Nguyen, L. N., Kim, J. H., Choi, E. H., and Kaushik, N. K. (2020). Strategies for using polydopamine to induce biomineralization of hydroxyapatite on implant materials for bone tissue engineering. Int. J. Mol. Sci. 21:6544. doi: 10.3390/ijms21186544
Khanzada, N. K., Rehman, S., Leu, S. Y., and An, A. K. (2020). Evaluation of anti-bacterial adhesion performance of polydopamine cross-linked graphene oxide RO membrane via in situ optical coherence tomography. Desalination 479:114339. doi: 10.1016/j.desal.2020.114339
Kim, W. J., Kim, J., Lee, H., and Hong, S. (2017). Method of preparing coating film containing nitrogen monoxide on surface of material using catecholamine. Google Patents:US20140127277A1. Pohang-city, KR: Postech Academy-Industry Foundation.
Kong, X.-J., Wu, S., Chen, T.-T., Yu, R.-Q., and Chu, X. (2016). MnO2-induced synthesis of fluorescent polydopamine nanoparticles for reduced glutathione sensing in human whole blood. Nanoscale 8, 15604–15610. doi: 10.1039/c6nr04777g
Ku, S. H., and Park, C. B. (2010). Human endothelial cell growth on mussel-inspired nanofiber scaffold for vascular tissue engineering. Biomaterials 31, 9431–9437. doi: 10.1016/j.biomaterials.2010.08.071
Lakshminarayanan, R., Madhavi, S., and Sim, C. P. C. (2018). Oxidative polymerization of dopamine: A high-definition multifunctional coatings for electrospun nanofibers-an overview. London, UK: Intechopen.
Larosa, V., and Remacle, C. (2018). Insights into the respiratory chain and oxidative stress. Biosci. Rep. 38:BSR20171492.
Lee, D. J., Lee, Y.-T., Zou, R., Daniel, R., and Ko, C.-C. (2017). Polydopamine-laced biomimetic material stimulation of bone marrow derived mesenchymal stem cells to promote osteogenic effects. Sci. Rep. 7, 1–14. doi: 10.1155/2016/4851081
Lee, H. A., Ma, Y., Zhou, F., Hong, S., and Lee, H. (2019). Material-independent surface chemistry beyond polydopamine coating. Acc. Chem. Res. 52, 704–713. doi: 10.1021/acs.accounts.8b00583
Lee, H., Dellatore, S. M., Miller, W. M., and Messersmith, P. B. (2007). Mussel-inspired surface chemistry for multifunctional coatings. Science 318, 426–430. doi: 10.1126/science.1147241
Lee, H., Rho, J., and Messersmith, P. B. (2009). Facile conjugation of biomolecules onto surfaces via mussel adhesive protein inspired coatings. Adv. Mater. 21, 431–434. doi: 10.1002/adma.200801222
Lei, W., Ren, K., Chen, T., Chen, X., Li, B., Chang, H., et al. (2016). Polydopamine nanocoating for effective photothermal killing of bacteria and fungus upon near-infrared irradiation. Adv. Mater. Interfaces 3:1600767. doi: 10.1002/admi.201600767
Li, F., Yu, Y., Wang, Q., Yuan, J., Wang, P., and Fan, X. (2018). Polymerization of dopamine catalyzed by laccase: Comparison of enzymatic and conventional methods. Enzyme Microb. Technol. 119, 58–64. doi: 10.1016/j.enzmictec.2018.09.003
Li, M., Liu, X., Xu, Z., Yeung, K., and Wu, S. (2016). Dopamine modified organic–inorganic hybrid coating for antimicrobial and osteogenesis. ACS Appl. Mater. Inter. 8, 33972–33981. doi: 10.1021/acsami.6b09457
Li, W.-Q., Wang, Z., Hao, S., He, H., Wan, Y., Zhu, C., et al. (2017). Mitochondria-targeting polydopamine nanoparticles to deliver doxorubicin for overcoming drug resistance. ACS Appl. Mater. Inter. 9, 16793–16802. doi: 10.1021/acsami.7b01540
Li, Y., Jiang, C., Zhang, D., Wang, Y., Ren, X., Ai, K., et al. (2017). Targeted polydopamine nanoparticles enable photoacoustic imaging guided chemo-photothermal synergistic therapy of tumor. Acta Biomater 47, 124–134. doi: 10.1016/j.actbio.2016.10.010
Li, Y., Yang, W., Li, X., Zhang, X., Wang, C., Meng, X., et al. (2015). Improving osteointegration and osteogenesis of three-dimensional porous Ti6Al4V scaffolds by polydopamine-assisted biomimetic hydroxyapatite coating. ACS Appl. Mater. Inter. 7, 5715–5724. doi: 10.1021/acsami.5b00331
Liang, Y., Zhao, X., Hu, T., Han, Y., and Guo, B. (2019). Mussel-inspired, antibacterial, conductive, antioxidant, injectable composite hydrogel wound dressing to promote the regeneration of infected skin. J. Coll. Inter. Sci. 556, 514–528. doi: 10.1016/j.jcis.2019.08.083
Liao, J., He, S., Guo, S., Luan, P., Mo, L., and Li, J. (2019). Antibacterial performance of a mussel-inspired polydopamine-treated Ag/graphene nanocomposite material. Materials 12:3360. doi: 10.3390/ma12203360
Liebscher, J. (2019). Chemistry of polydopamine–Scope, variation, and limitation. Eur. J. Org. Chem. 2019, 4976–4994. doi: 10.1002/ejoc.201900445
Liebscher, J., Mroìwczynìski, R., Scheidt, H. A., Filip, C., Ha?dade, N. D., Turcu, R., et al. (2013). Structure of polydopamine: A never-ending story? Langmuir 29, 10539–10548. doi: 10.1021/la4020288
Lim, K., Chua, R. R. Y., Ho, B., Tambyah, P. A., Hadinoto, K., and Leong, S. S. J. (2015). Development of a catheter functionalized by a polydopamine peptide coating with antimicrobial and antibiofilm properties. Acta Biomater. 15, 127–138. doi: 10.1016/j.actbio.2014.12.015
Lin, J.-H., Yu, C.-J., Yang, Y.-C., and Tseng, W.-L. (2015). Formation of fluorescent polydopamine dots from hydroxyl radical-induced degradation of polydopamine nanoparticles. Phys. Chem. Chem. Phys. 17, 15124–15130. doi: 10.1039/c5cp00932d
Liu, C., Yao, W., Tian, M., Wei, J., Song, Q., and Qiao, W. (2018). Mussel-inspired degradable antibacterial polydopamine/silica nanoparticle for rapid hemostasis. Biomaterials 179, 83–95. doi: 10.1016/j.biomaterials.2018.06.037
Liu, C.-Y., and Huang, C.-J. (2016). Functionalization of polydopamine via the aza-Michael reaction for antimicrobial interfaces. Langmuir 32, 5019–5028. doi: 10.1021/acs.langmuir.6b00990
Liu, F., He, X., Zhang, J., Chen, H., Zhang, H., and Wang, Z. (2015). Controllable synthesis of polydopamine nanoparticles in microemulsions with pH-activatable properties for cancer detection and treatment. J. Mater. Chem. B 3, 6731–6739. doi: 10.1039/c5tb01159k
Liu, H., Qu, X., Tan, H., Song, J., Lei, M., Kim, E., et al. (2019). Role of polydopamine’s redox-activity on its pro-oxidant, radical-scavenging, and antimicrobial activities. Acta Biomater. 88, 181–196. doi: 10.1016/j.actbio.2019.02.032
Liu, M., Liu, T., Chen, X., Yang, J., Deng, J., He, W., et al. (2018). Nano-silver-incorporated biomimetic polydopamine coating on a thermoplastic polyurethane porous nanocomposite as an efficient antibacterial wound dressing. J. Nanobiotechnol. 16, 1–19.
Liu, M., Zhou, J., Yang, Y., Zheng, M., Yang, J., and Tan, J. (2015). Surface modification of zirconia with polydopamine to enhance fibroblast response and decrease bacterial activity in vitro: a potential technique for soft tissue engineering applications. Coll. Surf. B 136, 74–83. doi: 10.1016/j.colsurfb.2015.06.047
Liu, Y., Li, Z., Yin, Z., Zhang, H., Gao, Y., Huo, G., et al. (2020). Amplified photoacoustic signal and enhanced photothermal conversion of polydopamine coated gold nanobipyramids for photo-theranostics and synergistic chemotherapy. ACS Appl. Mater. Inter. 12, 14866–14875. doi: 10.1021/acsami.9b22979
Luo, C., Liu, W., Luo, B., Tian, J., Wen, W., Liu, M., et al. (2017). Antibacterial activity and cytocompatibility of chitooligosaccharide-modified polyurethane membrane via polydopamine adhesive layer. Carbohyd. Polym. 156, 235–243. doi: 10.1016/j.carbpol.2016.09.036
Lynge, M. E., van der Westen, R., Postma, A., and Städler, B. (2011). Polydopamine—A nature-inspired polymer coating for biomedical science. Nanoscale 3, 4916–4928. doi: 10.1039/c1nr10969c
Ma, L., Liu, F., Lei, Z., and Wang, Z. (2017). A novel upconversion@ polydopamine core@ shell nanoparticle based aptameric biosensor for biosensing and imaging of cytochrome c inside living cells. Biosens. Bioelectron. 87, 638–645. doi: 10.1016/j.bios.2016.09.017
Madhurakkat Perikamana, S. K., Lee, J., Lee, Y. B., Shin, Y. M., Lee, E. J., Mikos, A. G., et al. (2015). Materials from mussel-inspired chemistry for cell and tissue engineering applications. Biomacromolecules 16, 2541–2555. doi: 10.1021/acs.biomac.5b00852
Maier, G. P., and Butler, A. (2017). Siderophores and mussel foot proteins: the role of catechol, cations, and metal coordination in surface adhesion. JBIC J. Biol. Inorg. Chem. 22, 739–749. doi: 10.1007/s00775-017-1451-6
Mallinson, D., Mullen, A. B., and Lamprou, D. A. (2018). Probing polydopamine adhesion to protein and polymer films: microscopic and spectroscopic evaluation. J. Mater. Sci. 53, 3198–3209. doi: 10.1007/s10853-017-1806-y
Maruthapandi, M., Natan, M., Jacobi, G., Banin, E., Luong, J. H., and Gedanken, A. (2019). Antibacterial activity against Methicillin-resistant staphylococcus aureus of colloidal polydopamine prepared by carbon dot stimulated polymerization of dopamine. Nanomaterials 9:1731. doi: 10.3390/nano9121731
Matai, I., Garg, M., Rana, K., and Singh, S. (2019). Polydopamine functionalized hydrogel beads as magnetically separable antibacterial materials. RSC Adv. 9, 13444–13457. doi: 10.1039/c9ra00623k
Meng, H., Forooshani, P. K., Joshi, P. U., Osborne, J., Mi, X., Meingast, C., et al. (2019). Biomimetic recyclable microgels for on-demand generation of hydrogen peroxide and antipathogenic application. Acta Biomater. 83, 109–118. doi: 10.1016/j.actbio.2018.10.037
Miao, Z.-H., Wang, H., Yang, H., Li, Z.-L., Zhen, L., and Xu, C.-Y. (2015). Intrinsically Mn2+-chelated polydopamine nanoparticles for simultaneous magnetic resonance imaging and photothermal ablation of cancer cells. ACS Appl. Mater. Inter. 7, 16946–16952. doi: 10.1021/acsami.5b06265
Moulay, S. (2018). Recent trends in mussel-inspired catechol-containing polymers (A Review). Orient. J. Chem. 34, 1153–1197. doi: 10.13005/ojc/340301
Neethu, S., Midhun, S. J., Radhakrishnan, E., and Jyothis, M. (2020). Surface functionalization of central venous catheter with mycofabricated silver nanoparticles and its antibiofilm activity on multidrug resistant Acinetobacter baumannii. Microb. Pathog. 138:103832. doi: 10.1016/j.micpath.2019.103832
Nieto, C., Vega, M. A., Marcelo, G., and del Valle, E. M. M. (2018). Polydopamine nanoparticles kill cancer cells. RSC Adv. 8, 36201–36208. doi: 10.1039/c8ra05586f
Niyonshuti, I. I., Krishnamurthi, V. R., Okyere, D., Song, L., Benamara, M., Tong, X., et al. (2020). Polydopamine surface coating synergizes the antimicrobial activity of silver nanoparticles. ACS Appl. Mater. Interfaces 12, 40067–40077. doi: 10.1021/acsami.0c10517
Park, D., Kim, J., Lee, Y. M., Park, J., and Kim, W. J. (2016). Polydopamine hollow nanoparticle functionalized with N-diazeniumdiolates as a nitric oxide delivery carrier for antibacterial therapy. Adv. Healthc. Mater. 5, 2019–2024. doi: 10.1002/adhm.201600150
Park, J., Moon, H., and Hong, S. (2019). Recent advances in melanin-like nanomaterials in biomedical applications: A mini review. Biomater. Res. 23, 1–10.
Patel, K., Singh, N., Yadav, J., Nayak, J. M., Sahoo, S. K., Lata, J., et al. (2018). Polydopamine films change their physicochemical and antimicrobial properties with a change in reaction conditions. Phys. Chem. Chem. Phys. 20, 5744–5755. doi: 10.1039/c7cp08406d
Poinard, B., Kamaluddin, S., Tan, A. Q. Q., Neoh, K. G., and Kah, J. C. Y. (2019). Polydopamine coating enhances mucopenetration and cell uptake of nanoparticles. ACS Appl. Mater. Inter. 11, 4777–4789. doi: 10.1021/acsami.8b18107
Priyam, A., Nagar, P., Sharma, A. K., and Kumar, P. (2018). Mussel-inspired polydopamine-polyethylenimine conjugated nanoparticles as efficient gene delivery vectors for mammalian cells. Coll. Surf. B 161, 403–412. doi: 10.1016/j.colsurfb.2017.10.063
Qian, Y., Zhou, X., Zhang, F., Diekwisch, T. G., Luan, X., Yang, J., et al. (2019). /PCL scaffold modification including silver impregnation, collagen coating, and electrospinning significantly improve biocompatibility, antimicrobial, and osteogenic properties for orofacial tissue regeneration. ACS Appl. Mater. Inter. 11, 37381–37396. doi: 10.1021/acsami.9b07053
Qiu, W. Z., Yang, H. C., and Xu, Z. K. (2018). Dopamine-assisted co-deposition: An emerging and promising strategy for surface modification. Adv. Coll. Interface Sci. 256, 111–125. doi: 10.1016/j.cis.2018.04.011
Rahimnejad, M., and Zhong, W. (2017). Mussel-inspired hydrogel tissue adhesives for wound closure. RSC Adv. 7, 47380–47396. doi: 10.1039/c7ra06743g
Ran, H.-H., Cheng, X., Gao, G., Sun, W., Jiang, Y.-W., Zhang, X., et al. (2020). Colistin-loaded polydopamine nanospheres uniformly decorated with silver nanodots: A nanohybrid platform with improved antibacterial and antibiofilm performance. ACS Appl. Bio Mater. 3, 2438–2448. doi: 10.1021/acsabm.0c00163
Ryu, J. H., Messersmith, P. B., and Lee, H. (2018). Polydopamine surface chemistry: A decade of discovery. ACS Appl. Mater. Inter. 10, 7523–7540. doi: 10.1021/acsami.7b19865
Sadrearhami, Z., Shafiee, F. N., Ho, K. K., Kumar, N., Krasowska, M., Blencowe, A., et al. (2019). Antibiofilm nitric oxide-releasing polydopamine coatings. ACS Appl. Mater. Inter. 11, 7320–7329. doi: 10.1021/acsami.8b16853
Singh, I., Priyam, A., Jha, D., Dhawan, G., Gautam, H. K., and Kumar, P. (2020). Polydopamine–aminoglycoside nanoconjugates: Synthesis, characterization, antimicrobial evaluation and cytocompatibility. Mater. Sci. Eng. C 107:110284. doi: 10.1016/j.msec.2019.110284
Solano, F. (2014). Melanins: Skin pigments and much more—types, structural models, biological functions, and formation routes. N. J. Sci. 2014:498276.
Solano, F. (2017). Melanin and melanin-related polymers as materials with biomedical and biotechnological applications—Cuttlefish ink and mussel foot proteins as inspired biomolecules. Int. J. Mol. Sci. 18:1561. doi: 10.3390/ijms18071561
Song, J., Chen, P., and Liu, W. (2019). A superhydrophobic and antibacterial surface coated on cotton fabrics by polydopamine. Fibers Polym. 20, 1380–1386. doi: 10.1007/s12221-019-1183-z
Song, Y., Jiang, H., Wang, B., Kong, Y., and Chen, J. (2018). Silver-incorporated mussel-inspired polydopamine coatings on mesoporous silica as an efficient nanocatalyst and antimicrobial agent. ACS Appl. Mater. Inter. 10, 1792–1801. doi: 10.1021/acsami.7b18136
Su, L., Yu, Y., Zhao, Y., Liang, F., and Zhang, X. (2016). Strong antibacterial polydopamine coatings prepared by a shaking-assisted method. Sci. Rep. 6:24420.
Tang, L., Livi, K. J., and Chen, K. L. (2015). Polysulfone membranes modified with bioinspired polydopamine and silver nanoparticles formed in situ to mitigate biofouling. Environ. Sci. Technol. Lett. 2, 59–65. doi: 10.1021/acs.estlett.5b00008
Tang, L., Mo, S., Liu, S. G., Liao, L. L., Li, N. B., and Luo, H. Q. (2018). Synthesis of fluorescent polydopamine nanoparticles by Michael addition reaction as an analysis platform to detect iron ions and pyrophosphate efficiently and construction of an IMPLICATION logic gate. Sensor Actuat B- Chem. 255, 754–762. doi: 10.1016/j.snb.2017.08.069
Tian, Y., and Lei, M. (2019). Polydopamine-based composite nanoparticles with redox-labile polymer shells for controlled drug release and enhanced chemo-photothermal therapy. Nanoscale Res. Lett. 14, 1–10.
Tyo, A. G., Welch, S., Hennenfent, M., Forooshani, P. K., Lee, B. P., and Rajachar, R. M. (2019). Development and characterization of an antimicrobial polydopamine coating for conservation of Humpback Whales. Front. Chem. 7:618. doi: 10.3389/fchem.2019.00618
Wang, B. B., Quan, Y. H., Xu, Z. M., and Zhao, Q. (2020). Preparation of highly effective antibacterial coating with polydopamine/chitosan/silver nanoparticles via simple immersion. Prog. Org. Coat. 149:105967. doi: 10.1016/j.porgcoat.2020.105967
Wang, C., Zhou, J., Wang, P., He, W., and Duan, H. (2016). Robust nanoparticle–DNA conjugates based on mussel-inspired polydopamine coating for cell imaging and tailored self-assembly. Bioconjug. Chem. 27, 815–823. doi: 10.1021/acs.bioconjchem.6b00021
Wang, D., Wu, H., Zhou, J., Xu, P., Wang, C., Shi, R., et al. (2018). In situ one-pot synthesis of MOF–polydopamine hybrid nanogels with enhanced photothermal effect for targeted cancer therapy. Adv. Sci. 5:1800287. doi: 10.1002/advs.201800287
Wang, K., Ma, Q., Zhang, Y., Wang, S., and Han, G. (2020). Ag NPs-assisted synthesis of stable Cu NPs on PET fabrics for antibacterial and electromagnetic shielding performance. Polymers 12:783. doi: 10.3390/polym12040783
Wang, L., Yang, X., Cao, W., Shi, C., Zhou, P., Li, Q., et al. (2017). Mussel-inspired deposition of copper on titanium for bacterial inhibition and enhanced osseointegration in a periprosthetic infection model. RSC Adv. 7, 51593–51604. doi: 10.1039/c7ra10203h
Wang, Q., Zhang, R., Lu, M., You, G., Wang, Y., Chen, G., et al. (2017). Bioinspired polydopamine-coated hemoglobin as potential oxygen carrier with antioxidant properties. Biomacromolecules 18, 1333–1341. doi: 10.1021/acs.biomac.7b00077
Wang, X., Wang, N., Yang, Y., Wang, X., Liang, J., Tian, X., et al. (2019). Polydopamine nanoparticles carrying tumor cell lysate as a potential vaccine for colorectal cancer immunotherapy. Biomater. Sci. 7, 3062–3075. doi: 10.1039/c9bm00010k
Wang, X., Zhang, J., Wang, Y., Wang, C., Xiao, J., Zhang, Q., et al. (2016). Multi-responsive photothermal-chemotherapy with drug-loaded melanin-like nanoparticles for synergetic tumor ablation. Biomaterials 81, 114–124. doi: 10.1016/j.biomaterials.2015.11.037
Wang, Z., Zhang, J., Chen, F., and Cai, K. (2017). Fluorescent miRNA analysis enhanced by mesopore effects of polydopamine nanoquenchers. Analyst 142, 2796–2804. doi: 10.1039/c7an00528h
Wen, L., Wanpei, H., Qian, L., Xu, L., Rongsheng, C., Hongwei, N., et al. (2020). Antibacterial properties of Ag/TiO2/PDA nanofilm on anodized 316L stainless steel substrate under illumination by a normal flashlight. J. Mater. Sci. 2020, 1–13. doi: 10.1007/s10853-020-04610-w
Wu, C., Zhang, G., Xia, T., Li, Z., Zhao, K., Deng, Z., et al. (2015). Bioinspired synthesis of polydopamine/Ag nanocomposite particles with antibacterial activities. Mater. Sci. Eng. C 55, 155–165. doi: 10.1016/j.msec.2015.05.032
Wu, H., Liu, Y., Huang, J., Mao, L., Chen, J., and Li, M. (2018). Preparation and characterization of antifouling and antibacterial polysulfone ultrafiltration membranes incorporated with a silver–polydopamine nanohybrid. J. Appl. Polym. Sci. 135:46430. doi: 10.1002/app.46430
Wu, K., Yang, Y., Zhang, Y., Deng, J., and Lin, C. (2015). Antimicrobial activity and cytocompatibility of silver nanoparticles coated catheters via a biomimetic surface functionalization strategy. Int. J. Nanomed. 10, 7241–7252. doi: 10.2147/ijn.s92307
Wu, Y., Zhou, Y., Xu, H., Liu, Q., Li, Y., Zhang, L., et al. (2018). Highly active, superstable, and biocompatible Ag/polydopamine/g-C3N4 bactericidal photocatalyst: synthesis, characterization, and mechanism. ACS Sust. Chem. Eng. 6, 14082–14094. doi: 10.1021/acssuschemeng.8b02620
Xiong, Y., Xu, Z., and Li, Z. (2019). Polydopamine-based nanocarriers for photosensitizer delivery. Front. Chem. 7:471. doi: 10.3389/fchem.2019.00471
Xu, Q., Chang, M., Zhang, Y., Wang, E., Xing, M., Gao, L., et al. (2020). PDA/Cu bioactive hydrogel with “Hot Ions Effect” for inhibition of drug-resistant bacteria and enhancement of infectious skin wound healing. ACS Appl. Mater. Interfaces 12, 31255–31269. doi: 10.1021/acsami.0c08890
Yan, J., Zhou, W., Jia, Z., Xiong, P., Li, Y., Wang, P., et al. (2018). Endowing polyetheretherketone with synergistic bactericidal effects and improved osteogenic ability. Acta Biomater. 79, 216–229. doi: 10.1016/j.actbio.2018.08.037
Yang, P., Gu, Z., Zhu, F., and Li, Y. (2020a). Structural and functional tailoring of melanin-like polydopamine radical scavengers. CCS Chemistry 2, 128–138. doi: 10.31635/ccschem.020.201900077
Yang, P., Zhang, S., Chen, X., Liu, X., Wang, Z., and Li, Y. (2020b). Recent developments in polydopamine fluorescent nanomaterials. Mater. Horizons 7, 746–761. doi: 10.1039/c9mh01197h
Yang, X., Niu, X., Mo, Z., Guo, R., Liu, N., Zhao, P., et al. (2019). Electrochemical chiral interface based on the Michael addition/Schiff base reaction of polydopamine functionalized reduced graphene oxide. Electrochim. Acta 319, 705–715. doi: 10.1016/j.electacta.2019.07.040
Yang, Z., Wu, Y., Wang, J., Cao, B., and Tang, C. Y. (2016). In situ reduction of silver by polydopamine: A novel antimicrobial modification of a thin-film composite polyamide membrane. Environ. Sci. Technol. 50, 9543–9550. doi: 10.1021/acs.est.6b01867
Yao, L., He, C., Chen, S., Zhao, W., Xie, Y., and Sun, S. (2018). Co-deposition of polydopamine and zwitterionic polymer on membrane surface with enhanced stability and antibiofouling property. Langmuir 35, 1430–1439. doi: 10.1021/acs.langmuir.8b01621
Yeroslavsky, G., Girshevitz, O., Foster-Frey, J., Donovan, D. M., and Rahimipour, S. (2015). Antibacterial and antibiofilm surfaces through polydopamine-assisted immobilization of lysostaphin as an antibacterial enzyme. Langmuir 31, 1064–1073. doi: 10.1021/la503911m
Yeroslavsky, G., Lavi, R., Alishaev, A., and Rahimipour, S. (2016). Sonochemically-produced metal-containing polydopamine nanoparticles and their antibacterial and antibiofilm activity. Langmuir 32, 5201–5212. doi: 10.1021/acs.langmuir.6b00576
Yilmaz, A. (2020). The employment of a conformal polydopamine thin layer reduces the cytotoxicity of silver nanoparticles. Turk. J. Zool. 44, 126–133. doi: 10.3906/zoo-1912-38
Yuan, Z., Tao, B., He, Y., Liu, J., Lin, C., Shen, X., et al. (2019). Biocompatible MoS2/PDOPA-RGD coating on titanium implant with antibacterial property via intrinsic ROS-independent oxidative stress and NIR irradiation. Biomaterials 217:119290. doi: 10.1016/j.biomaterials.2019.119290
Yun’an Qing, L. C., Li, R., Liu, G., Zhang, Y., Tang, X., Wang, J., et al. (2018). Potential antibacterial mechanism of silver nanoparticles and the optimization of orthopedic implants by advanced modification technologies. Int. J. Nanomed. 13:3311. doi: 10.2147/ijn.s165125
Yuwen, L., Sun, Y., Tan, G., Xiu, W., Zhang, Y., Weng, L., et al. (2018). MoS2@ polydopamine-Ag nanosheets with enhanced antibacterial activity for effective treatment of Staphylococcus aureus biofilms and wound infection. Nanoscale 10, 16711–16720. doi: 10.1039/c8nr04111c
Zhang, C., Gong, l, Xiang, l, Du, Y., Hu, W., and Zeng, H. (2017). Deposition and adhesion of polydopamine on the surfaces of varying wettability. ACS Appl. Mater. Interfaces 9, 30943–30950. doi: 10.1021/acsami.7b09774
Zhang, Q., Wang, Y., Zhang, W., Hickey, M. E., Lin, Z., Tu, Q., et al. (2019). In situ assembly of well-dispersed Ag nanoparticles on the surface of polylactic acid-Au@polydopamine nanofibers for antimicrobial applications. Coll. Surf. B 184:110506. doi: 10.1016/j.colsurfb.2019.110506
Zhao, H., Zeng, Z., Liu, L., Chen, J., Zhou, H., Huang, L., et al. (2018). Polydopamine nanoparticles for the treatment of acute inflammation-induced injury. Nanoscale 10, 6981–6991. doi: 10.1039/c8nr00838h
Zhao, Y.-Y., Li, L., Yu, R.-Q., Chen, T.-T., and Chu, X. (2017). CoOOH-induced synthesis of fluorescent polydopamine nanoparticles for the detection of ascorbic acid. Anal. Methods 9, 5518–5524. doi: 10.1039/c7ay01566f
Zheng, A., Zhang, X., Huang, Y., Cai, Z., Liu, X., and Liu, J. (2018). Polydopamine-assisted versatile modification of a nucleic acid probe for intracellular microRNA imaging and enhanced photothermal therapy. RSC Adv. 8, 6781–6788. doi: 10.1039/c8ra00261d
Zhou, C., Song, H., Loh, J. L. C., She, J., Deng, L., and Liu, B. (2018). Grafting antibiofilm polymer hydrogel film onto catheter by SARA SI-ATRP. J. Biomater. Sci., Polym. Ed. 29, 2106–2123. doi: 10.1080/09205063.2018.1507268
Zhou, L., Li, X., Wang, K., Shen, F., Zhang, L., Li, P., et al. (2020). Cu∥-loaded polydopamine coatings with in situ nitric oxide generation function for improved hemocompatibility. Regen. Biomater. 7, 153–160. doi: 10.1093/rb/rbz043
Zhou, P., Deng, Y., Lyu, B., Zhang, R., Zhang, H., Ma, H., et al. (2014). Rapidly-deposited polydopamine coating via high temperature and vigorous stirring: Formation, characterization and biofunctional evaluation. PLoS One 9:e113087. doi: 10.1371/journal.pone.0113087
Zhou, T., Yan, L., Xie, C., Li, P., Jiang, L., Fang, J., et al. (2019). A mussel-inspired persistent ROS-scavenging, electroactive, and osteoinductive scaffold based on electrochemical-driven in situ nanoassembly. Small 15:1805440. doi: 10.1002/smll.201805440
Zhu, Y., Xin, N., Qiao, Z., Chen, S., Zeng, l, Zhang, Y., et al. (2019). Novel tumor-microenvironment-based sequential catalytic therapy by Fe (II)-engineered polydopamine nanoparticles. ACS Appl. Mater. Inter. 11, 43018–43030. doi: 10.1021/acsami.9b17951
Zhu, Z., and Su, M. (2017). Polydopamine nanoparticles for combined chemo-and photothermal cancer therapy. Nanomaterials 7:160. doi: 10.3390/nano7070160
Keywords: biofilm, polymerization, ROS, polydopamine, antimicrobial, surface coating
Citation: Singh I, Dhawan G, Gupta S and Kumar P (2021) Recent Advances in a Polydopamine-Mediated Antimicrobial Adhesion System. Front. Microbiol. 11:607099. doi: 10.3389/fmicb.2020.607099
Received: 16 September 2020; Accepted: 02 December 2020;
Published: 12 January 2021.
Edited by:
Silvia Buroni, University of Pavia, ItalyReviewed by:
Qi Zhao, University of Dundee, United KingdomCopyright © 2021 Singh, Dhawan, Gupta and Kumar. This is an open-access article distributed under the terms of the Creative Commons Attribution License (CC BY). The use, distribution or reproduction in other forums is permitted, provided the original author(s) and the copyright owner(s) are credited and that the original publication in this journal is cited, in accordance with accepted academic practice. No use, distribution or reproduction is permitted which does not comply with these terms.
*Correspondence: Gagan Dhawan, Z2FnYW5kaGF3YW5AYW5kYy5kdS5hYy5pbg==; Seema Gupta, c2VlbWFndXB0YUBhbmRjLmR1LmFjLmlu; Pradeep Kumar, cGt1bWFyQGlnaWIucmVzLmlu
Disclaimer: All claims expressed in this article are solely those of the authors and do not necessarily represent those of their affiliated organizations, or those of the publisher, the editors and the reviewers. Any product that may be evaluated in this article or claim that may be made by its manufacturer is not guaranteed or endorsed by the publisher.
Research integrity at Frontiers
Learn more about the work of our research integrity team to safeguard the quality of each article we publish.