- 1Department of Microbiology, Institute for Water and Wetland Research, Radboud University, Nijmegen, Netherlands
- 2School of Physics and Astronomy, Institute for Condensed Matter and Complex Systems, Edinburgh University, Edinburgh, United Kingdom
Volcanic areas emit a number of gases including methane and other short chain alkanes, that may serve as energy source for the prevailing microorganisms. The verrucomicrobial methanotroph Methylacidiphilum fumariolicum SolV was isolated from a volcanic mud pot, and is able to grow under thermoacidophilic conditions on different gaseous substrates. Its genome contains three operons encoding a particulate methane monooxygenase (pMMO), the enzyme that converts methane to methanol. The expression of two of these pmo operons is subjected to oxygen-dependent regulation, whereas the expression of the third copy (pmoCAB3) has, so far, never been reported. In this study we investigated the ability of strain SolV to utilize short-chain alkanes and monitored the expression of the pmo operons under different conditions. In batch cultures and in carbon-limited continuous cultures, strain SolV was able to oxidize and grow on C1–C3 compounds. Oxidation of ethane did occur simultaneously with methane, while propane consumption only started once methane and ethane became limited. Butane oxidation was not observed. Transcriptome data showed that pmoCAB1 and pmoCAB3 were induced in the absence of methane and the expression of pmoCAB3 increased upon propane addition. Together the results of our study unprecedently show that a pMMO-containing methanotroph is able to co-metabolize other gaseous hydrocarbons, beside methane. Moreover, it expands the substrate spectrum of verrucomicrobial methanotrophs, supporting their high metabolic flexibility and adaptation to the harsh and dynamic conditions in volcanic ecosystems.
Introduction
Methane (CH4) is a powerful greenhouse gas, which is released to the atmosphere from both natural and anthropogenic sources. About 70–80% of CH4 is generated biologically and a large part of it is removed in the stratosphere and troposphere through reactions with chlorine and ⋅OH radicals (Le Mer and Roger, 2001). In addition, microbial methane oxidation is an important terrestrial methane sink (Conrad, 2020). Bacteria can convert CH4 to methanol aerobically using the enzyme methane monooxygenase (MMO; Conrad, 2009). Under anaerobic conditions, mostly methanotrophic archaea remove methane via reverse methanogenesis (Welte et al., 2016).
After the original discovery by Soehngen (1906), it was believed for a long time that aerobic methanotrophy was restricted to the phylum Proteobacteria, specifically in the subphyla α- and γ-Proteobacteria (Op den Camp et al., 2009). During the past decade, it was discovered that two bacterial phyla contained new methanotrophic representatives: the intra-aerobic NC10 (Raghoebarsing et al., 2006; Ettwig et al., 2010; Hu et al., 2014) and the Verrucomicrobia (Dunfield et al., 2007; Pol et al., 2007; Islam et al., 2008).
The phylum Verrucomicrobia includes highly acidophilic and mesophilic Methylacidimicrobium species (optimum pH 1–3: temperature 30–44°C) (Sharp et al., 2014; van Teeseling et al., 2014) and thermophilic but less acidophilic strains of the genus Methylacidiphilum (optimum pH 2–2.7; temperature 50–55°C) (Dunfield et al., 2007; Pol et al., 2007; Islam et al., 2008; Erikstad et al., 2019). Methylacidiphilum fumariolicum SolV is the most studied verrucomicrobial methanotroph to date and was initially discovered in the Solfatara volcano near Naples (Italy) (Pol et al., 2007). Strain SolV grows optimally at pH 2.7 and 55°C and it fixes CO2 via the Calvin cycle and N2 gas through a nitrogenase enzyme (Khadem et al., 2010, 2011). Methane can be used as energy source, but strain SolV is also able to use hydrogen gas as substrate, even at sub-atmospheric concentrations (Mohammadi et al., 2017; Schmitz et al., 2020). Beside methane, the Solfatara volcano in Naples (and many other volcanic areas) emits a mixture of gas that also includes ethane (C2H6, 805–1218 ppbv), propane (C3H8, 68–178 ppbv), and butane (C4H10, 8–18 ppbv) (Capaccioni and Mangani, 2001). These gases are particularly important because they could serve as additional substrate for microorganisms. Further, they are present, together with methane, in natural gas, which is commonly used in households and industries.
The oxidation of C2–C4 compounds is mainly observed in a group of bacteria that includes the genera Corynebacterium, Nocardia, Mycobacterium, Rhodococcus, Pseudomonas and the sulfate-reducing bacteria Desulfosarcina/Desulfococcus and Desulfotomaculum (Takahashi, 1980; Ashraf et al., 1994; Hamamura et al., 1999; Kinnaman et al., 2007; Kniemeyer et al., 2007). Recently, oxidation of alkanes under anoxic conditions was reported in archaea and catalyzed by the enzyme ethyl/methyl-coenzyme M reductase (MCR; Laso-Pérez et al., 2016; Borrel et al., 2019; Chen et al., 2019; Seitz et al., 2019; Wang et al., 2019).
In the past, the aerobic oxidation of methane and short-chain alkanes was considered to be carried out by separate groups of microorganisms (Crombie and Murrell, 2014). However, early studies already obtained indications that methanotrophs might be able to oxidize ethane, propane and butane (Leadbetter and Foster, 1960; Hazeu and de Bruyn, 1980; Shennan, 2006). In 2010, Stable Isotope Probing (SIP) experiments linked the oxidation of ethane to the family Methylococcaceae and the oxidation of propane to unclassified γ-Proteobacteria (Redmond et al., 2010). Methylocella silvestris (α-Proteobacteria) was the first strain that showed simultaneous growth on methane and propane. This strain contained both a soluble methane monooxygenase (sMMO) and a soluble propane monooxygenase (PrMMO) (Crombie and Murrell, 2014). Genes encoding proteins of the methylmalonyl-CoA pathway of propionate oxidation were induced during growth on propane.
The enzymes involved in aerobic hydrocarbon oxidation are usually soluble di-iron monooxygenases complexes consisting of multiple associated proteins (Shennan, 2006). sMMO also has this structure and exhibits a larger substrate range than the copper-containing particulate methane monooxygenase (pMMO; Burrows et al., 1984). However, the butane monooxygenases of Nocardiodes CF8 and Mycobacterium probably contain copper (Hamamura and Arp, 2000; Coleman et al., 2012).
The mechanism of hydrocarbon oxidation starts with the conversion of the alkane into an alcohol. More specifically, ethane is oxidized to ethanol, acetaldehyde and acetate; propane can be oxidized at the terminal or subterminal carbon atom, leading to the formation of 1-propanol, propionaldehyde, and propanoic acid in case of terminal oxidation and to 2-propanol and acetone in case of sub-terminal oxidation. Butane, instead, is oxidized to 1-butanol, butyraldehyde, and butyric acid (Shennan, 2006).
The genome of strain SolV does not encode sMMO, nor propane or butane monooxygenases, but it shows the presence of three operons for the membrane-bound pMMO. pmoCAB1 and pmoCAB2 operons are located in close proximity in the genome and their PmoA subunits share 84% amino acid identity. The pmoCAB3 operon, instead, is distantly located and its PmoA3 subunit only shares 41% amino acid identity to PmoA1 and PmoA2. Experimental data have demonstrated that the expression of pmoCAB1 and pmoCAB2 is regulated by oxygen concentrations (Khadem et al., 2012), whereas pmoCAB3 expression was so far not detected under any growth condition tested. One hypothesis proposes that pmoCAB3 is of ancestral origin and its function could differ from methane oxidation (Fuerst, 2014). Therefore, the aim of this study was to test the ability of strain SolV to grow on short-chain alkanes and to investigate the expression of the three pmo operons.
Here we report that M. fumariolicum SolV can grow on ethane and propane, but not on butane. When methanol is supplied to a SolV culture with no oxygen limitation, expression of pmoCAB1 and pmoCAB3 could be detected. Furthermore, pmoCAB3 expression increased upon propane addition.
Materials and Methods
Microorganism and Medium Composition
Methylacidiphilum fumariolicum strain SolV used in this study was initially isolated from the volcanic region Campi Flegrei, near Naples, Italy (Pol et al., 2007). The medium was composed of 0.2 mM MgCl2.6H2O; 0.2 mM CaCl2.2H2O; 1 mM Na2SO4; 2 mM K2SO4; 4 mM (NH4)2SO4, 1 mM NaH2PO4.H2O. A trace element solution was added resulting in the following end concentrations: 1 μM NiCl2, CoCl2, MoO4Na2, ZnSO4 and CeCl3; 5 μM MnCl2 and FeSO4; 10 μM CuSO4 and 40–50 μM nitrilotriacetic acid (NTA). The pH of medium was adjusted to 2.7 using 1 M H2SO4. To avoid precipitation, CaCl2.2H2O and the rest of the medium were autoclaved separately and mixed after cooling.
Chemostat Cultivation With Methanol/Ethane
To test the consumption of ethane, a continuous culture with the standard medium containing 50 mM methanol (CH3OH; added through a 0.2 μm sterile filter to the medium) was used. The bioreactor was operated at 55°C with stirring at 700 rpm using a stirrer bar. The chemostat (liquid volume of 300 ml) was supplied with the medium at a flow rate of 3.9 ml h–1 (D = 0.013 h–1), using a peristaltic pump. The cell-containing medium was removed automatically from the chemostat by a peristaltic pump when the liquid level reached the sensor in the reactor. A supply of 10% O2 (v/v) and 5% CO2 (v/v) in argon (total gas flow = 10.6 ml min–1) was directed to the reactor by mass flow controllers through a sterile filter and sparged into the medium just above the stirrer bar. The initial pH was 2.7 and it was regulated with 0.2 M NaOH connected to the vessel by a peristaltic pump. The pH at steady state was kept at about 2.2. At steady state, while cells were grown under methanol limitation, a supply of ethane was introduced to the reactor. An O2 sensor (Applikon, Delft, Netherlands) in the liquid was coupled to a Biocontroller (Applikon) to monitor the dO2 values during growth.
Chemostat Cultivation With Natural Gas and Methanol/Propane
Chemostat cultivations with methanol and natural gas were performed in a bioreactor (500 ml MiniBio Reactor, Applikon Biotechnology, Delft, Netherlands) with a working volume of 350 ml liquid medium. The system was run at 55°C and 1500 rpm stirring speed. pH, dO2, and medium level were monitored by Applikon MyControl Reactor sensors. The natural gas reactor was operated with a dilution rate of 0.024 h–1 (8.4 ml h–1 fresh medium was added) and a constant gas inflow consisting of air, N2, and natural gas. The natural gas mix used in this experiment consisted of 78.53% methane, 3.34% ethane, 0.46% propane, and 0.09% butane. The remaining 17.58% consisted of N2, O2, CO2, and trace amounts of higher alkanes. In the Netherlands, 1.8 ppm of the sulfur compound tetrahydrothiophene (THT) is added for safety reasons. During the oxygen limiting condition, 0.60 ml/min of natural gas was flowing through the bioreactor, with 2.20 ml/min air and 7.19 ml/min N2. Under methane limiting condition, 0.60 ml/min of natural gas was supplied to the bioreactor, with 4.40 ml/min air and 4.99 ml/min N2. Under both conditions, the total gas flow was 9.99 ml/min. In the experiments where propane was supplied, the reactor was operated with a dilution rate of 0.012 h–1 (medium contained 50 mM methanol) and a constant gas inflow consisting of air and CO2. Propane was added at a rate of 0.1 ml/min, resulting in a total gas flow of 1.6 ml/min. Biomass was measured as optical density at 600 nm (OD600) using 1 ml cuvettes with the spectrophotometer Spectronic 200 (Thermo Fisher Scientific, Waltham, MA, United States).
Dry Weight Determination
To determine biomass dry-weight concentration, 10 ml of the culture suspension (triplicate) were filtered through pre-weighed 0.45 μm filters and dried to stable weight in a vacuum oven at 70°C.
Batch Cultivation
The batch growth experiments were performed using 120 and 250 ml serum bottles containing 10 and 20 ml medium, respectively, with a headspace containing air, CO2 (10%) and CH4 (2%), C2H6 (4%), or C3H8 (4%). All incubations were performed at 55°C at 350 rpm.
Gas Analysis
Alkane concentrations were measured injecting 100 μl of sample with a Hamilton glass syringe in a HP 5890 gas chromatograph (Agilent, United States) equipped with a Porapak Q column (1.8 m, ID 2 mm) and a flame ionization detector.
Respiration Experiments
Respiration rates were determined polarographically in a respiration cell with an oxygen microsensor (RC350, Strathkelvin, Motherwell, United Kingdom) using 3 ml of whole cell suspensions of strain SolV. Methane, propane, or oxygen saturated media were injected into the respiration chamber to obtain the desired dissolved gas concentrations. The O2 signal was monitored and recorded using SensorTrace Basic software (Unisense, Aarhus, Denmark). The temperature and stirring rate in the respiration chamber was adjusted to 55°C and 1000 rpm, respectively. Rates were expressed as nmol O2 min–1 mg DW–1 and when necessary corrected for endogenous respiration.
RNA Extraction and Transcriptome Analysis
A volume of 5 ml cell suspension was harvested from a continuous culture at steady state. After centrifugation (10,000 × g, 5 min) the pellet was used for RNA isolation using the RiboPureTM-Bacteria kit (ThermoFisher, Waltham, MA, United States) according to manufacturer’s instructions. mRNA was purified with the MegaClear kit (Ambion) and MICROBexpressTM kit (Thermo Fisher Scientific, Waltham, MA, United States) according to manufacturer’s protocol. The efficiency of rRNA and small RNAs removal was analyzed using the Agilent 2100 Bioanalyzer (Agilent, Santa Clara, CA, United States). The mRNA extracted from cells grown with natural gas and with ethane was then converted to cDNA with the Ion Total RNA-SeqTM Kit v2 (Thermo Fisher Scientific, Waltham, MA, United States) following manufacturer’s instructions. cDNA was amplified and purified to prepare barcoded libraries. Ion SphereTM Particles (ISPs) were used to create template positive ISPs by the Ion OneTouchTM 2 instrument, which were enriched in the Ion OneTouchTM ES instrument. This was performed with the Ion PGMTM Template OT2 200 Kit (Ion Torrent, Life technologies). Sequencing of these templates was conducted on an Ion 318TM Chip v2 using the Ion PGMTM sequencing 200 Kit v2. The mRNA extracted from cells grown on propane and methanol was used to construct libraries using the TruSeq Stranded mRNA Library Prep protocol (Illumina) according to the manufacturer’s instructions. Libraries were normalized, pooled and sequenced using the Illumina MiSeq sequencing machine. For sequencing, the 150 bp single-read sequencing chemistry was performed using the MiSeq Reagent Kit v3 (Illumina, San Diego, CA, United States) according the manufacturer’s protocol. The RNA-Seq Analysis tool of the CLC Genomic Work bench software (version 10.1.1, CLC-Bio, Aarhus, Denmark) was used for sequence analysis. As a template for the transcriptome analysis, the complete genome sequence of strain SolV, available at the Microscope annotation platform (Vallenet et al., 2009), was used. Expression values were expressed as RPKM (Mortazavi et al., 2008).
Statistical analyses were performed using the DESeq2 package in R Studio (Love et al., 2014). Transcriptomics data were deposited in NCBI Bioproject database with accession number PRJEB39356.
Results
Oxidation of Short-Chain Alkanes by M. fumariolicum SolV
The ability of strain SolV to oxidize short-chain alkanes was tested as follows: cells were incubated in bottles containing natural gas (in v/v: 75% CH4, 3% C2H6, and 0.5% C3H8), and this resulted in exponential growth with a doubling time of 10.2 h and a growth rate of 0.068 h–1 (97% of μmax; Figure 1). After 20 h, a strong drop in the methane concentrations was observed and within two days the values were below the detection limit (5 ppm). Ethane seemed to be consumed simultaneously (Figure 1). Propane consumption only started once ethane and methane became limiting (after 50 h; Figure 1). At the end of the experiment, concentrations of both ethane and propane were below detection levels. Remarkably, the sulfur compound THT seemed to be degraded as well as concluded from effluent gas analysis on a gas chromatograph equipped with a sulfur-specific flame-photometric detector (data not shown).
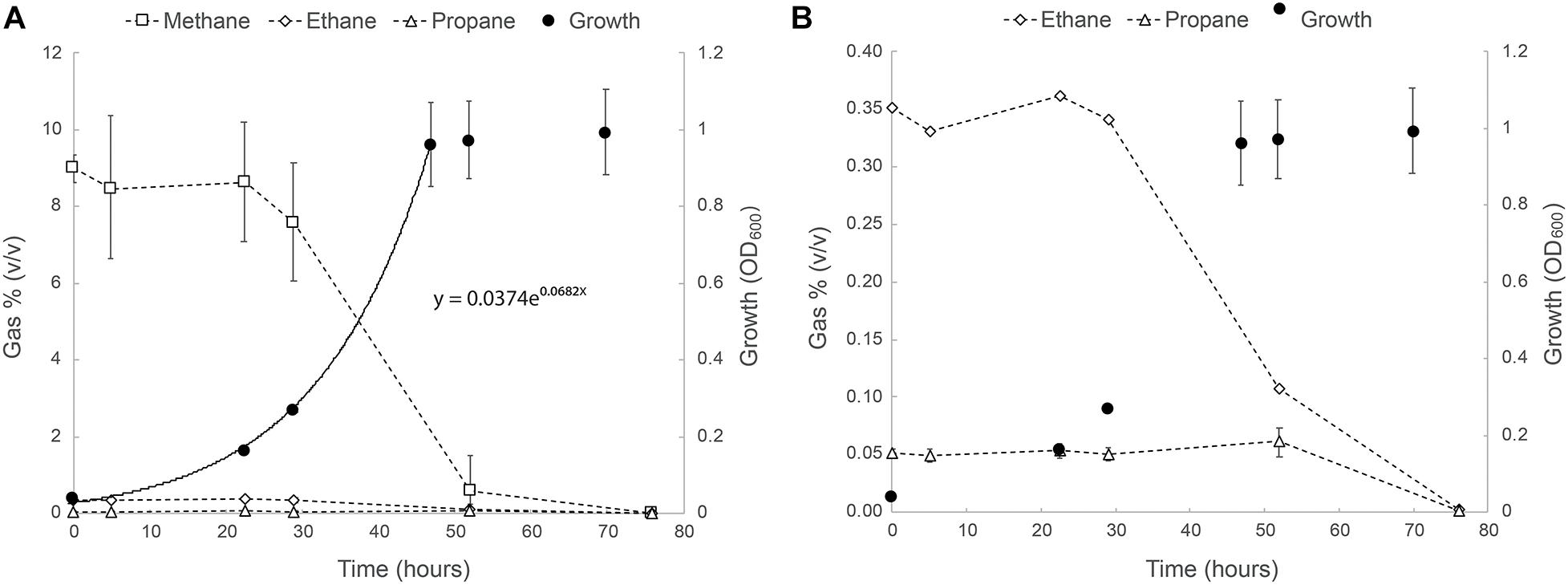
Figure 1. (A) Growth of strain SolV on natural gas (75% CH4, 3% C2H6, and 0.5% C3H8). (B) Close up of the ethane and propane consumption profiles in the same experiment. The data points are the average of three biological replicates. Error bars represent the standard deviation. Where not visible, error bars are smaller than the symbols.
In a follow up experiment, growth of strain SolV was tested in serum bottles with separate methane/alkane mixtures. Results for methane/butane (2 and 4%, v/v), methane/propane (2 and 4%, v/v) and methane/ethane (2 and 4% v/v) are shown in Figures 2A,C,E. Consumption of butane in the presence and/or absence of methane was not observed (Figures 2A,B). Propane was consumed from the start but the rate increased after methane became limiting and, although the exponential growth phase was very short, we could calculate a growth rate of 0.007 h–1 (about 10% of μmax on CH4), equivalent to a doubling time of 102 h (Figures 2C,D).
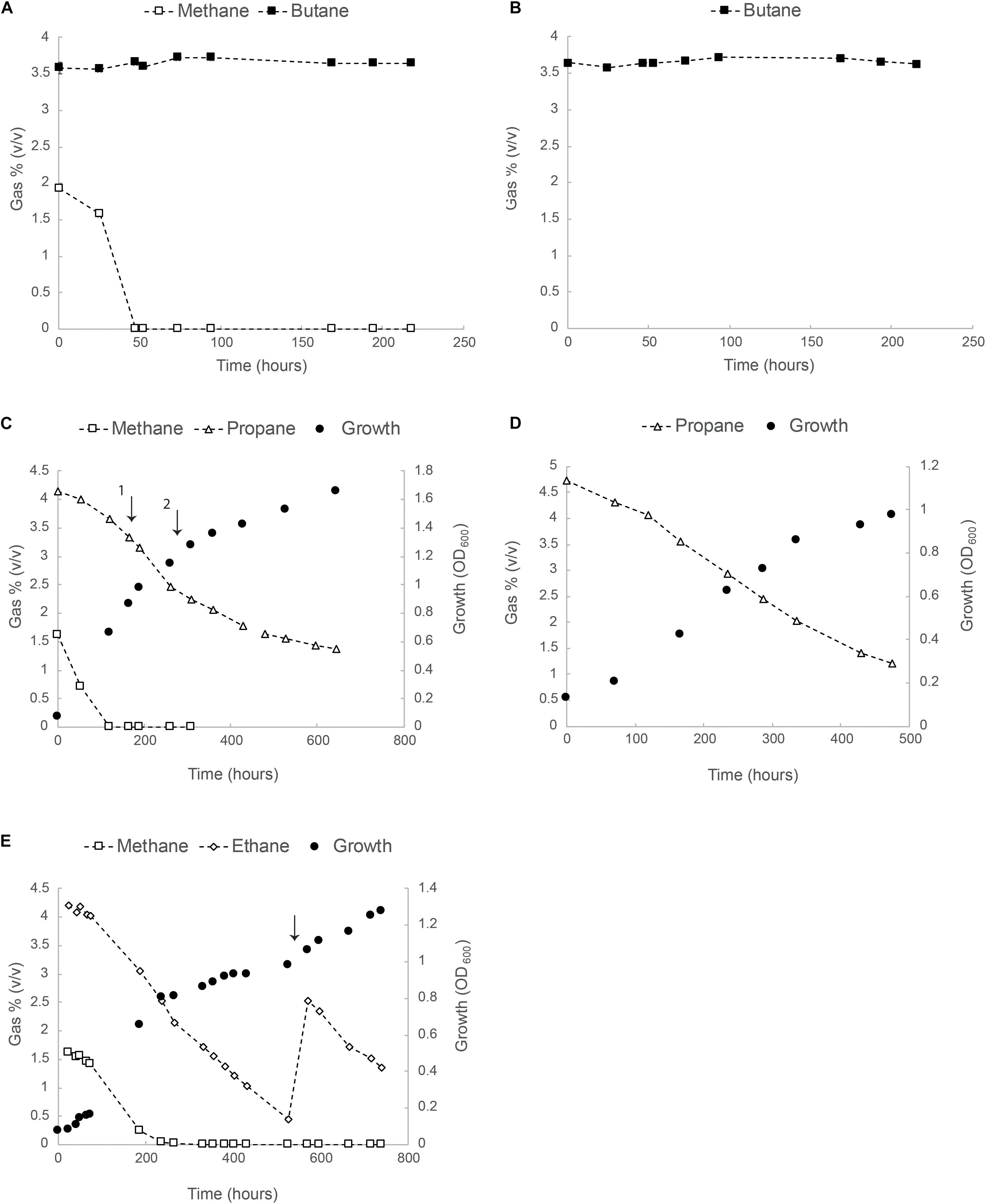
Figure 2. Oxidation of butane, propane and ethane by strain SolV. (A) Consumption of methane and butane in batch cultures. (B) Butane concentration over time in batch cultures containing SolV cells. In panels (A,B) data points represent the average over three biological replicates. All error bars indicating standard deviation (SD) are smaller than the symbols. (C) Batch growth of strain SolV in serum bottles with methane/propane. The first arrow indicates an addition of 4 mM NH4+ and 10 ml O2. The second arrow indicates an addition of extra trace element solution to avoid any limitation. (D) Growth of strain SolV with only propane using an inoculum from the incubation depicted in panel (C). (E) Growth of strain SolV with methane and ethane mixture, followed by a growth only using ethane. The arrow indicates an addition of 5 ml ethane, 10 ml O2, 4 mM NH4+ and extra trace elements. In panels (C–E), data points represent the average over two biological replicates.
The same batch experiments were also performed with methane/ethane (2 and 4%, v/v) at starting OD600 0.07 (Figure 2E). Both gases were consumed simultaneously. Once methane was completely consumed (250 h), OD600 reached 0.8. At this time, about 65% of ethane was still present and growth continued till OD600 1.0 and ethane became limited (Figure 2E). At this point, 5 ml ethane, 10 ml O2, 4 mM NH4+ and extra trace elements (enough for OD600 5) were added. Growth continued to OD600 1.3.
To further investigate the consumption of short-chain alkanes by SolV, cells were cultivated in a continuous system where oxygen and nutrient concentrations can be easily monitored and controlled. The chemostat was first kept under oxygen-limited conditions, using natural gas as energy source. The culture reached a steady state at OD600 0.96 ± 0.09 and primarily consumed methane and ethane (Table 1). After 25 days, the air inflow was doubled, so that the amount of natural gas became limiting. The OD600 increased from 0.96 ± 0.09 to 1.7 ± 0.1 and the dissolved oxygen concentration from 0 to 4%. In this situation, methane consumption doubled and ethane consumption was 4.4 times higher. In addition, significant propane consumption could be detected. Butane consumption was not observed (Table 1). Ethane oxidation happened simultaneously with methane, but propane oxidation only started once methane became limiting, confirming the results of the batch experiments.
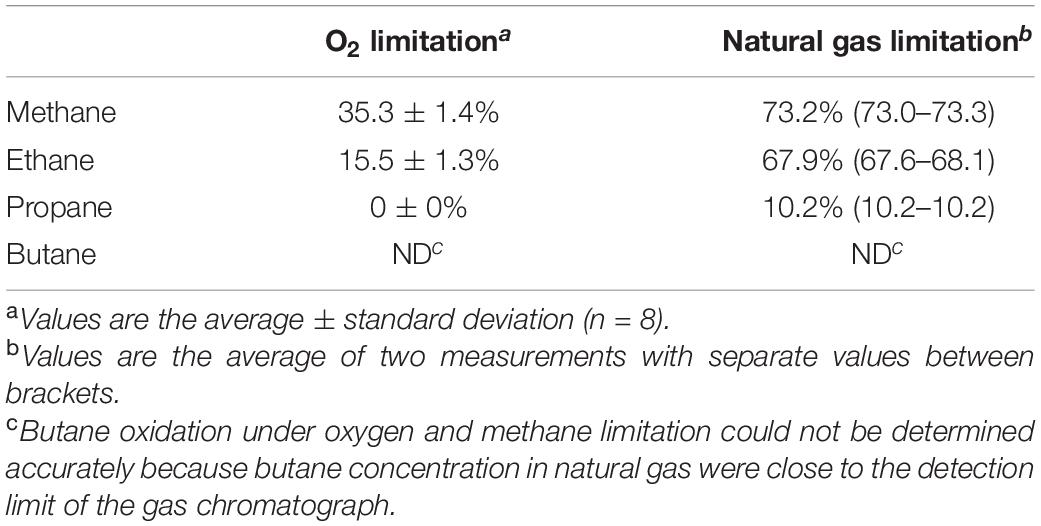
Table 1. Consumption of methane, ethane, propane, and butane by M. fumariolicum SolV during O2 and natural gas limitation.
The capability of multiple substrates consumption by co-metabolism provided further opportunities to establish a correlation between physiological activity and gene expression in strain SolV. The genome of strain SolV shows the presence of three pmo operons. To investigate their expression, a transcriptome analysis was performed under both the oxygen and natural gas limited conditions described above. Under oxygen limited conditions, pmoCAB2 had the highest expression levels, whereas pmoCAB1 had the highest expression under natural gas limitation (Supplementary Figure 1). These data confirmed the oxygen-dependent regulation of these operons observed before in strain SolV (Khadem et al., 2012). The third operon, pmoCAB3, did not show high levels of expression in both conditions.
Natural gas only contains minor amounts of ethane and propane. To investigate the consumptions of these alkanes in more detail, higher concentrations were used in a chemostat with methanol as the additional substrate. Methanol, which is as effective as methane for growth, was used instead of methane to eliminate the need for an additional gas supply.
Oxidation of Ethane and Propane in Strain SolV
A continuous culture using methanol as an electron donor (medium flow rate = 3.9 ml h–1; D = 0.013 h–1) was established. At the steady state, when methanol was the limiting growth factor, the optical density was stable (OD600 = 1.03 ± 0.02) and the consumption of methanol occurred at a rate of 25.9 nmol min–1 mg DW–1. After reaching a steady state, ethane was supplied to the cells. The amount of ethane provided to the bioreactor was slowly increased from 0.36 to 7.2 μmol min–1. Average consumption of the supplied ethane was 50% and this resulted in an increase of OD600 from 1.01 to 1.71 (70% increase). The highest ethane consumption rate measured was 15.6 nmol min–1 mg DW–1, which is about 60% of the methanol consumption rate (25.9 nmol min–1 mg DW–1), supporting the increase in optical density.
Further, cells of M. fumariolicum SolV grown with methanol and ethane were harvested and RNA was extracted to perform transcriptome analysis. Analysis of the expression of the pmo operons showed that pmoCAB1 had the highest expression values while expression of pmoCAB2 was hardly detectable (Figure 3). Surprisingly, pmoCAB3 expression was noticed for the first time, suggesting that either the presence of methanol or ethane could induce its expression. The transcriptome data did not reveal any upregulation of genes that could point to the pathway used for further oxidation of ethane after conversion to acetate.
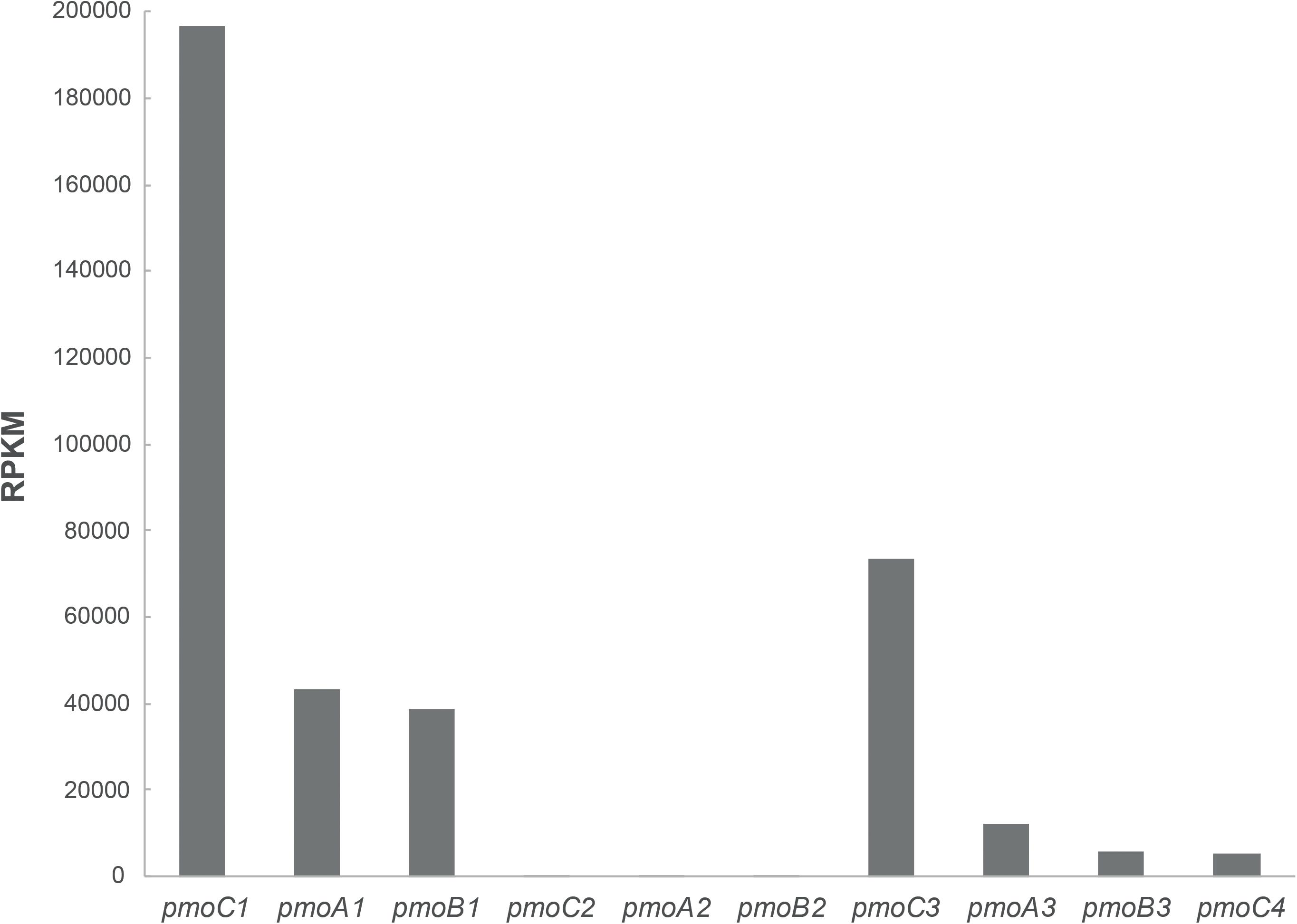
Figure 3. Transcriptome analysis of pMMO encoding operons in M. fumariolicum SolV grown on methanol and ethane. Expression values are reported as RPKM and they represent the average over two samples.
Similarly, to test the growth of strain SolV in a continuous culture grown with propane, another bioreactor using methanol as electron donor was started (D = 0.012 h–1). Under methanol limitation conditions, biomass reached a steady state at OD600 0.84 ± 0.05. Propane was then added at a rate of 4.5 μmol min–1. The propane addition resulted in a new steady state at OD600 1.13 ± 0.04, which equals an increase of 34% in biomass. Out of the supplied propane, only 0.1% was removed and the consumption of propane was measured at a rate of 0.46 ± 0.14 nmol min–1 mg DW–1.
Since the alkane consumption was so low, we checked the propane oxidation rate in respiration experiments using SolV cells from a continuous culture grown on methane (oxygen limited; D = 0.017 h–1) (Khadem et al., 2012) and we calculated a respiration rate on propane of 2.4 nmol O2 min–1 mg DW–1. This value corresponds to 0.48 nmol propane min–1 mg DW–1, which is in agreement with what calculated in the reactor (0.46 nmol propane min–1 mg DW–1).
Also for the methanol/propane culture, a transcriptome analysis was performed, but this time we analyzed both cells growing on methanol/propane and on methanol only. As shown in Figure 4, pmoCAB1 had the highest expression in both conditions, followed by pmoCAB3. The pmoCAB2 operon was not expressed. An upregulation of pmoCAB3 and downregulation of pmoCAB1 in presence of propane seemed to happen. However, the difference in the expression of pmoCAB1 was not statistically significant (p > 0.05), whereas expression of pmoCAB3 was significantly higher (p = 0.00). These data shows that pmoCAB3 expression is linked to the absence of methane or presence of methanol, but its expression levels increase when propane is supplied. The transcriptome data did not reveal upregulation of genes that could point to the pathway used for further oxidation of propane after conversion to propanoate.
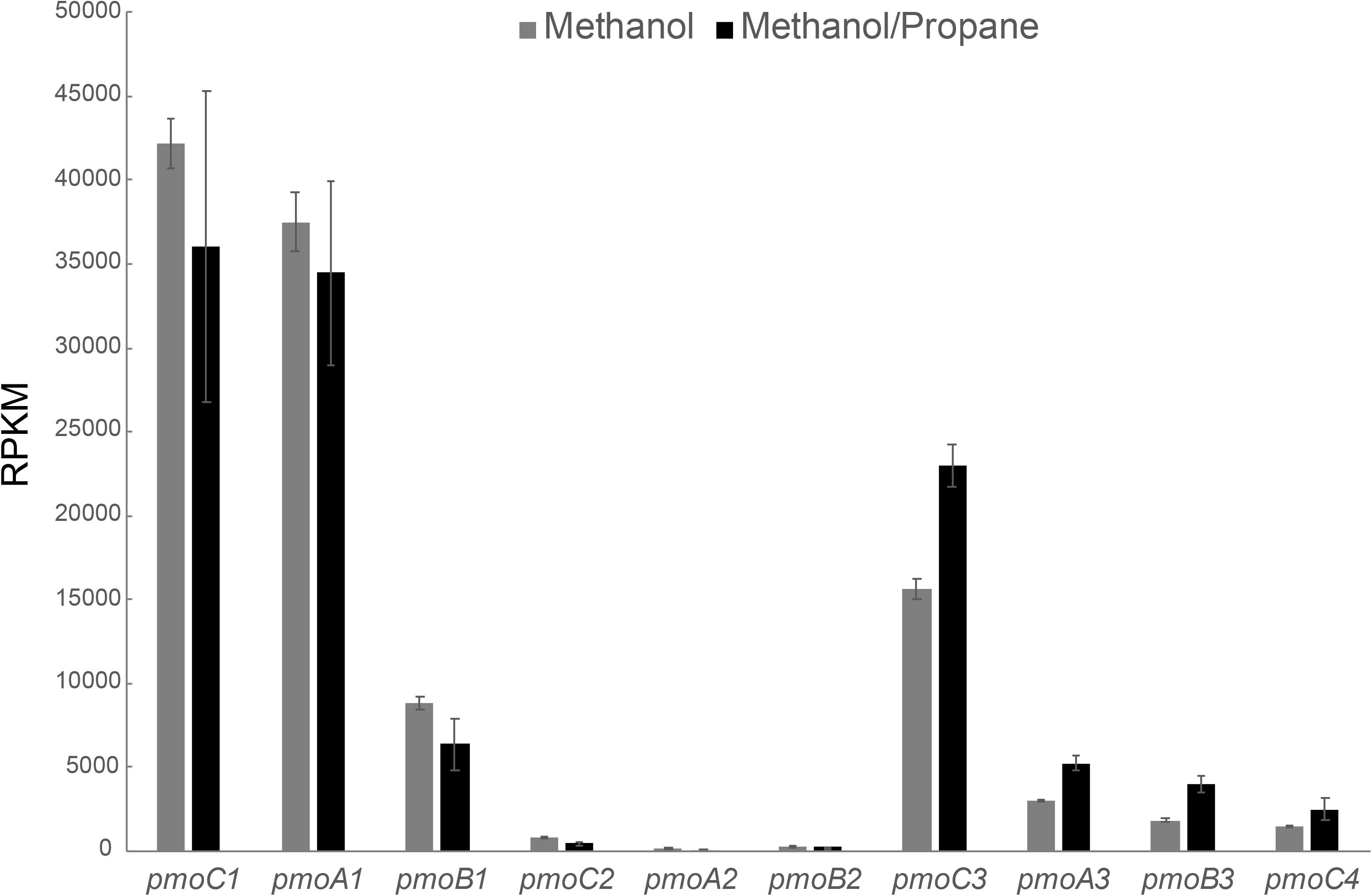
Figure 4. Transcriptome analysis of pMMO encoding operons in M. fumariolicum SolV grown on methanol (gray bars) and methanol plus propane (black bars). Expression values are reported as RPKM. Data is shown as the average over three samples and error bars represent the standard deviation.
Discussion
In this study, we show that M. fumariolicum SolV is able to co-metabolize ethane and propane with methane or methanol, but not butane. To our knowledge, the ability to use other gaseous hydrocarbons for growth is unprecedented in a pMMO-encoding methanotroph (with no sMMO present). Most methanotrophs are highly selective, to the point that they only grow on methane and its one-carbon derivatives such as methanol, and cannot grow on complex, multi-carbon substrates like sugars or organic acids. However, the facultative methanotroph Methylocella silvestris that only contains sMMO (and PrMMO) is able to grow on acetate, ethanol, pyruvate, succinate, malate and propane in addition to methane and methanol (Dedysh et al., 2005; Chen et al., 2010; Crombie and Murrell, 2014). In 2010, Redmond and colleagues (Redmond et al., 2010) suggested an alternative function for pmoA genes, based on the ability of some pmoA-containing microorganisms to incorporate carbon derived from the oxidation of ethane and propane. Our study demonstrates that pMMO is indeed involved in the consumption of alkanes in strain SolV. Further, we could show that without oxygen limitation and in the presence of ethane/propane and methanol, pmoCAB1 and pmoCAB3 were expressed. This is the first time that pmoCAB3 expression in SolV is ever detected. Previous work only documented the alternate expression of pmoCAB1 and pmoCAB2 in relation to oxygen (Khadem et al., 2012). The increased expression of pmoCAB3 upon propane addition suggests that these genes could be involved in alkane oxidation. At the current state we cannot conclude which pMMO enzyme complex performs ethane and propane oxidation nor we can exclude that it is a combination of multiple enzymes. Further experiments, including more detailed and time-resolved RNAseq studies and expression of this operon in an alternative host, need to be performed to elucidate the exact role of pmoCAB3 in alkane consumption.
It is expected that ethane and propane are first converted by pMMO to ethanol and propanol, respectively. The XoxF-type PQQ-dependent methanol dehydrogenase of strain SolV was shown to convert these alcohol to their aldehyde (acetaldehyde, propanaldehyde) and acid (acetate, propanoate) forms (Pol et al., 2014). The transcriptome data did not reveal upregulation of genes that could point to the further oxidation of these metabolites. Acetate could be shuttled into the central metabolism by the acetyl-CoA synthetase present in the genome of strain SolV (Mfumv2_2288). The enzymology of propane oxidation after the first two steps is poorly understood. Crombie and Murrell (2014) found an induction of the methylmalonyl-CoA pathway enzymes during growth on propane. However, the genome of strain SolV does not encode for these enzymes. However, acetyl-CoA synthetase (EC 6.2.1.1) is also know to convert propanoate into propanoyl-CoA. Metabolomic studies could shed light on the pathways involved.
The oxidation of ethane and propane in M. fumariolicum SolV proceeded at different rates. In particular, ethane seemed to be preferred over propane since (i) it could be oxidized simultaneously with methane, (ii) the oxidation rate was faster, and (iii) it led to a higher increase in biomass (70% vs 34%). This probably depends on the ability of pMMO of binding and converting molecules with different number of carbon atoms. Moreover, differences in the ethane oxidation rate could be noticed in different conditions. In particular, a 10-fold increase in the ethane consumption rate was calculated in the continuous culture compared to the batch incubations. This discrepancy could be due to CO2 or NH4+ limitations in the batch experiments. The solubility of the different alkanes at 55°C do not differ much. The values calculated from mole fractions taken from Wilhelm et al. (1977) were 0.92 mM for methane, 1.04 mM for ethane, 0.79 mM for propane, and 0.56 mM for butane.
Contrary to ethane and propane, butane consumption was not observed in strain SolV. Butane oxidation, together with propane and ethane, was detected in Methylosinus trichosporium OB3b. This bacterium encodes both sMMO and pMMO (Burrows et al., 1984), but its pMMO is different than the ones encoded by strain SolV. PmoA from M. trichosporium OB3b only shares 53% amino acid identity to strain SolV’s PmoA1, 51% to PmoA2 and 40% to PmoA3.
The sMMO-containing methanotroph Methylococcus capsulatus Bath also showed the ability of oxidizing C1–C8 compounds (Colby et al., 1977). The oxidation of these alkanes in M. capsulatus and M. trichosporium, however, was not linked to growth. Additionally, butane oxidation has been documented in the genera Nocardioides, Mycobacterium, Giesbergeria, Ramlibacter, Arthrobacter, Brevibacterium (McLee et al., 1972; Hamamura et al., 1999; Hamamura and Arp, 2000; Deng et al., 2018) and in the β-proteobacterium Thauera butanovora (Arp, 1999). The butane monooxygenase of T. butanovora (sBMO) presents high identity (38–65%) to sMMO as it contains three subunits α, β and γ encoded by bmoX, bmoY and bmoZ genes and a non-haem carboxylate-bridged diiron site (Sluis et al., 2002). The sBMO has a much lower affinity for methane (1.1 mM) compared to sMMO (3–13 μM) (Cooley et al., 2009). The butane degrading strain CF8, instead, seems to possess a pBMO similar to pMMO with subunit identities of 34–47% (Hamamura et al., 1999; Kinnaman et al., 2007; Sayavedra-Soto et al., 2011). A copper containing monooxygenase in Mycobacterium able to oxidize C2–C4 alkanes was also described (Coleman et al., 2012).
In conclusion, this study demonstrates that, beside methane and hydrogen, verrucomicrobial methanotrophs are also able to co-metabolize higher alkanes. This result is particularly important in view of the ecological role of these bacteria in the environment. Methanotrophic Verrucomicrobia appear to be not only extremely resistant to thermoacidic geothermal volcanoes, but also remarkably flexible in terms of substrate utilization. Their metabolic flexibility regarding carbon compounds could be partly provided by the differential expression of the pmoCAB copies in relation to the substrate available.
Data Availability Statement
The datasets presented in this study can be found in online repositories. The names of the repository/repositories and accession number(s) can be found below: NCBI BioProject, accession no: PRJEB39356.
Author Contributions
NP, SM, AP, MJ, and HO designed the projects and experiments. NP and AW performed the natural gas experiments. NP, SM, and AP performed the ethane/propane experiments. NP and TA sequenced and analyzed the transcriptomes. NP, AP, and HO carried out the data analysis. NP, SM, and HO wrote the manuscript. All authors contributed to revision of the manuscript, and read and approved the submitted version.
Funding
NP and HO were supported by the European Research Council (ERC Advanced Grant project VOLCANO 669371). MJ was supported by the European Research Council (ERC Advanced Grant projects Eco_MoM 339880 and SIAM 024002002).
Conflict of Interest
The authors declare that the research was conducted in the absence of any commercial or financial relationships that could be construed as a potential conflict of interest.
Acknowledgments
We would like to thank Dr. Christopher Lawson for providing the codes for statistical analysis. Part of the text included in the Materials and Methods section is derived from a Ph.D. thesis (Mohammadi, 2016).
Supplementary Material
The Supplementary Material for this article can be found online at: https://www.frontiersin.org/articles/10.3389/fmicb.2020.604485/full#supplementary-material
References
Arp, D. J. (1999). Butane metabolism by butane-grown ‘Pseudomonas butanovora’. Microbiology 145, 1173–1180. doi: 10.1099/13500872-145-5-1173
Ashraf, W., Mihdhir, A., and Murrell, J. C. (1994). Bacterial oxidation of propane. FEMS Microbiol. Lett. 122, 1–6. doi: 10.1111/j.1574-6968.1994.tb07134
Borrel, G., Adam, P. S., McKay, L. J., Chen, L.-X., Sierra-García, I. N., Sieber, C. M. K., et al. (2019). Wide diversity of methane and short-chain alkane metabolisms in uncultured archaea. Nat. Microbiol. 4, 603–613. doi: 10.1038/s41564-019-0363-3
Burrows, K. J., Cornish, A., Scott, D., and Higgins, I. J. (1984). Substrate specificities of the soluble and particulate methane mono-oxygenases of Methylosinus trichosporium OB3b. J. Gen. Microbiol. 130, 3327–3333. doi: 10.1099/00221287-130-12-3327
Capaccioni, B., and Mangani, F. (2001). Monitoring of active but quiescent volcanoes using light hydrocarbon distribution in volcanic gases: the results of 4 years of discontinuous monitoring in the Campi Flegrei (Italy). Earth Planet. Sci. Lett. 188, 543–555. doi: 10.1016/S0012-821X(01)00338-7
Chen, S.-C., Musat, N., Lechtenfeld, O. J., Paschke, H., Schmidt, M., Said, N., et al. (2019). Anaerobic oxidation of ethane by archaea from a marine hydrocarbon seep. Nature 568, 108–111. doi: 10.1038/s41586-019-1063-0
Chen, Y., Crombie, A., Rahman, M. T., Dedysh, S. N., Liesack, W., Stott, M. B., et al. (2010). Complete genome sequence of the aerobic facultative methanotroph Methylocella silvestris BL2. J. Bacteriol. 192, 3840–3841. doi: 10.1128/JB.00506-10
Colby, J., Stirling, D. I., and Dalton, H. (1977). The soluble methane mono-oxygenase of Methylococcus capsulatus (Bath). Biochem. J. 165, 395–402. doi: 10.1042/bj1650395
Coleman, N. V., Le, N. B., Ly, M. A., Ogawa, H. E., McCarl, V., Wilson, N. L., et al. (2012). Hydrocarbon monooxygenase in Mycobacterium: recombinant expression of a member of the ammonia monooxygenase superfamily. ISME J. 6, 171–182. doi: 10.1038/ismej.2011.98
Conrad, R. (2009). The global methane cycle: recent advances in understanding the microbial processes involved. Environ. Microbiol. Rep. 1, 285–292. doi: 10.1111/j.1758-2229.2009.00038.x
Conrad, R. (2020). Methane production in soil environments—Anaerobic biogeochemistry and microbial life between flooding and desiccation. Microorganisms 8:881. doi: 10.3390/microorganisms8060881
Cooley, R. B., Dubbels, B. L., Sayavedra-Soto, L. A., Bottomley, P. J., and Arp, D. J. (2009). Kinetic characterization of the soluble butane monooxygenase from Thauera butanivorans, formerly ‘Pseudomonas butanovora’. Microbiology 155, 2086–2096. doi: 10.1099/mic.0.028175-0
Crombie, A. T., and Murrell, J. C. (2014). Trace-gas metabolic versatility of the facultative methanotroph Methylocella silvestris. Nature 510, 148–151. doi: 10.1038/nature13192
Dedysh, S. N., Knief, C., and Dunfield, P. F. (2005). Methylocella species are facultatively methanotrophic. J. Bacteriol. 187, 4665–4670. doi: 10.1128/JB.187.13.4665-4670.2005
Deng, Y., Deng, C., Yang, J., Li, B., Wang, E., and Yuan, H. (2018). Novel butane-oxidizing bacteria and diversity of bmoX Genes in Puguang Gas Field. Front. Microbiol. 9:1576. doi: 10.3389/fmicb.2018.01576
Dunfield, P. F., Yuryev, A., Senin, P., Smirnova, A. V., Stott, M. B., Hou, S., et al. (2007). Methane oxidation by an extremely acidophilic bacterium of the phylum Verrucomicrobia. Nature 450, 879–882. doi: 10.1038/nature06411
Erikstad, H.-A., Ceballos, R. M., Smestad, N. B., and Birkeland, N.-K. (2019). Global biogeographic distribution patterns of thermoacidophilic verrucomicrobia methanotrophs suggest allopatric evolution. Front. Microbiol. 10:1129. doi: 10.3389/fmicb.2019.01129
Ettwig, K. F., Butler, M. K., Le Paslier, D., Pelletier, E., Mangenot, S., Kuypers, M. M. M., et al. (2010). Nitrite-driven anaerobic methane oxidation by oxygenic bacteria. Nature 464, 543–548. doi: 10.1038/nature08883
Fuerst, J. A. (ed.) (2014). Planctomycetes: Cell Structure, Origins and Biology. (Totowa, NJ: Humana Press), 1–286. doi: 10.1007/978-1-62703-502-6
Hamamura, N., and Arp, D. J. (2000). Isolation and characterization of alkane-utilizing Nocardioides sp. strain CF8. FEMS Microbiol. Lett. 186, 21–26. doi: 10.1111/j.1574-6968.2000.tb09076.x
Hamamura, N., Storfa, R. T., Semprini, L., and Arp, D. J. (1999). Diversity in butane monooxygenases among butane-grown bacteria. Appl. Environ. Microbiol. 65, 4586–4593. doi: 10.1128/AEM.65.10.4586-4593.1999
Hazeu, W., and de Bruyn, J. C. (1980). Ethane oxidation by methane-oxidizing bacteria. Antonie Van Leeuwenhoek 46, 443–455. doi: 10.1007/BF00395825
Hu, B., Shen, L., Lian, X., Zhu, Q., Liu, S., Huang, Q., et al. (2014). Evidence for nitrite-dependent anaerobic methane oxidation as a previously overlooked microbial methane sink in wetlands. Proc. Natl. Acad. Sci. U.S.A. 111, 4495–4500. doi: 10.1073/pnas.1318393111
Islam, T., Jensen, S., Reigstad, L. J., Larsen, Ø., and Birkeland, N.-K. (2008). Methane oxidation at 55°C and pH 2 by a thermoacidophilic bacterium belonging to the Verrucomicrobia phylum. Proc. Natl. Acad. Sci. U.S.A. 105, 300–304. doi: 10.1073/pnas.0704162105
Khadem, A. F., Pol, A., Jetten, M. S. M., and Op den Camp, H. J. M. (2010). Nitrogen fixation by the verrucomicrobial methanotroph ‘Methylacidiphilum fumariolicum’ SolV. Microbiology 156, 1052–1059. doi: 10.1099/mic.0.036061-0
Khadem, A. F., Pol, A., Wieczorek, A., Mohammadi, S. S., Francoijs, K.-J., Stunnenberg, H. G., et al. (2011). Autotrophic methanotrophy in Verrucomicrobia: Methylacidiphilum fumariolicum SolV uses the Calvin-Benson-Bassham Cycle for carbon dioxide fixation. J. Bacteriol. 193, 4438–4446. doi: 10.1128/JB.00407-11
Khadem, A. F., Pol, A., Wieczorek, A. S., Jetten, M. S. M., and Op den Camp, H. (2012). Metabolic Regulation of “Ca. Methylacidiphilum fumariolicum” SolV cells grown under different nitrogen and oxygen limitations. Front. Microbiol. 3, 266. doi: 10.3389/fmicb.2012.00266
Kinnaman, F. S., Valentine, D. L., and Tyler, S. C. (2007). Carbon and hydrogen isotope fractionation associated with the aerobic microbial oxidation of methane, ethane, propane and butane. Geochim. Cosmochim. Acta 71, 271–283. doi: 10.1016/j.gca.2006.09.007
Kniemeyer, O., Musat, F., Sievert, S. M., Knittel, K., Wilkes, H., Blumenberg, M., et al. (2007). Anaerobic oxidation of short-chain hydrocarbons by marine sulphate-reducing bacteria. Nature 449, 898–901. doi: 10.1038/nature06200
Laso-Pérez, R., Wegener, G., Knittel, K., Widdel, F., Harding, K. J., Krukenberg, V., et al. (2016). Thermophilic archaea activate butane via alkyl-coenzyme M formation. Nature 539, 396–401. doi: 10.1038/nature20152
Le Mer, J., and Roger, P. (2001). Production, oxidation, emission and consumption of methane by soils: a review. Eur. J. Soil Biol. 37, 25–50. doi: 10.1016/S1164-5563(01)01067-6
Leadbetter, E. R., and Foster, J. W. (1960). Bacterial oxidation of gaseous alkanes. Arch. Mikrobiol. 35, 92–104. doi: 10.1007/BF00425597
Love, M. I., Huber, W., and Anders, S. (2014). Moderated estimation of fold change and dispersion for RNA-seq data with DESeq2. Genome Biol. 15:550. doi: 10.1186/s13059-014-0550-8
McLee, A. G., Kormendy, A. C., and Wayman, M. (1972). Isolation and characterization of n-butane-utilizing microorganisms. Can. J. Microbiol. 18, 1191–1195. doi: 10.1139/m72-186
Mohammadi, S., Pol, A., Van Alen, T. A., Jetten, M. S., and Op den Camp, H. J. (2017). Methylacidiphilum fumariolicum SolV, a thermoacidophilic “Knallgas” methanotroph with both an oxygen-sensitive and -insensitive hydrogenase. ISME J. 11, 945–958. doi: 10.1038/ismej.2016.171
Mohammadi, S. S. (2016). One-Carbon and Hydrogen Metabolism of Acidophilic Verrucomicrobial Methanotrophs. PhD thesis, Radboud University Nijmegen, Nijmegen.
Mortazavi, A., Williams, B. A., McCue, K., Schaeffer, L., and Wold, B. (2008). Mapping and quantifying mammalian transcriptomes by RNA-Seq. Nat. Methods 5, 621–628. doi: 10.1038/nmeth.1226
Op den Camp, H. J. M., Islam, T., Stott, M. B., Harhangi, H. R., Hynes, A., Schouten, S., et al. (2009). Environmental, genomic and taxonomic perspectives on methanotrophic Verrucomicrobia. Environ. Microbiol. Rep. 1, 293–306. doi: 10.1111/j.1758-2229.2009.00022.x
Pol, A., Barends, T. R., Dietl, A., Khadem, A. F., Eygensteyn, J., Jetten, M. S. M., et al. (2014). Rare earth metals are essential for methanotrophic life in volcanic mudpots. Environ. Microbiol. 16, 255–264. doi: 10.1111/1462-2920.12249
Pol, A., Heijmans, K., Harhangi, H. R., Tedesco, D., Jetten, M. S. M., and Op den Camp, H. J. M. (2007). Methanotrophy below pH 1 by a new Verrucomicrobia species. Nature 450, 874–878. doi: 10.1038/nature06222
Raghoebarsing, A. A., Pol, A., van de Pas-Schoonen, K. T., Smolders, A. J. P., Ettwig, K. F., Rijpstra, W. I. C., et al. (2006). A microbial consortium couples anaerobic methane oxidation to denitrification. Nature 440, 918–921. doi: 10.1038/nature04617
Redmond, M. C., Valentine, D. L., and Sessions, A. L. (2010). Identification of novel methane-, ethane-, and propane-oxidizing bacteria at marine hydrocarbon seeps by stable isotope probing. Appl. Environ. Microbiol. 76, 6412–6422. doi: 10.1128/AEM.00271-10
Sayavedra-Soto, L. A., Hamamura, N., Liu, C.-W., Kimbrel, J. A., Chang, J. H., and Arp, D. J. (2011). The membrane-associated monooxygenase in the butane-oxidizing Gram-positive bacterium Nocardioides sp. strain CF8 is a novel member of the AMO/PMO family. Environ. Microbiol. Rep. 3, 390–396. doi: 10.1111/j.1758-2229.2010.00239.x
Schmitz, R. A., Pol, A., Mohammadi, S. S., Hogendoorn, C., van Gelder, A. H., Jetten, M. S. M., et al. (2020). The thermoacidophilic methanotroph Methylacidiphilum fumariolicum SolV oxidizes subatmospheric H2 with a high-affinity, membrane-associated [NiFe] hydrogenase. ISME J. 14, 1223–1232. doi: 10.1038/s41396-020-0609-3
Seitz, K. W., Dombrowski, N., Eme, L., Spang, A., Lombard, J., Sieber, J. R., et al. (2019). Asgard archaea capable of anaerobic hydrocarbon cycling. Nat. Commun. 10:1822. doi: 10.1038/s41467-019-09364-x
Sharp, C. E., Smirnova, A. V., Graham, J. M., Stott, M. B., Khadka, R., Moore, T. R., et al. (2014). Distribution and diversity of Verrucomicrobia methanotrophs in geothermal and acidic environments. Environ. Microbiol. 16, 1867–1878. doi: 10.1111/1462-2920.12454
Shennan, J. L. (2006). Utilisation of C2-C4 gaseous hydrocarbons and isoprene by microorganisms. J. Chem. Technol. Biotechnol. 81, 237–256. doi: 10.1002/jctb.1388
Sluis, M. K., Sayavedra-Soto, L. A., and Arp, D. J. (2002). Molecular analysis of the soluble butane monooxygenase from ‘Pseudomanas butanovora’. Microbiology 148, 3617–3629. doi: 10.1099/00221287-148-11-3617
Soehngen, N. L. (1906). Het ontstaan en verdwijnen van waterstof en methaan onder den invloed van organisch leven. Ph.D. thesis, University of Delft, The Netherlands. Available online at: https://resolver.kb.nl/resolve?urn=MMSFUBA02:000012372
Takahashi, J. (1980). Production of intracellular and extracellular protein from n-butane by Pseudomonas butanovora sp. nov. Adv. Appl. Microbiol. 26, 117–127. doi: 10.1016/S0065-2164(08)70332-0
Vallenet, D., Engelen, S., Mornico, D., Cruveiller, S., Fleury, L., Lajus, A., et al. (2009). MicroScope: a platform for microbial genome annotation and comparative genomics. Database 2009:bap021. doi: 10.1093/database/bap021
van Teeseling, M. C. F., Pol, A., Harhangi, H. R., van der Zwart, S., Jetten, M. S. M., Op den Camp, H. J. M., et al. (2014). Expanding the verrucomicrobial methanotrophic world: description of three novel species of Methylacidimicrobium gen. nov. Appl. Environ. Microbiol. 80, 6782–6791. doi: 10.1128/AEM.01838-14
Wang, Y., Wegener, G., Hou, J., Wang, F., and Xiao, X. (2019). Expanding anaerobic alkane metabolism in the domain of Archaea. Nat. Microbiol. 4, 595–602. doi: 10.1038/s41564-019-0364-2
Welte, C. U., Rasigraf, O., Vaksmaa, A., Versantvoort, W., Arshad, A., Op den Camp, H. J. M., et al. (2016). Nitrate- and nitrite-dependent anaerobic oxidation of methane. Environ. Microbiol. Rep. 8, 941–955. doi: 10.1111/1758-2229.12487
Keywords: Methylacidiphilum fumariolicum, higher alkanes, ethane, propane, butane, thermoacidophilic, methanotroph
Citation: Picone N, Mohammadi SS, Waajen AC, van Alen TA, Jetten MSM, Pol A and Op den Camp HJM (2020) More Than a Methanotroph: A Broader Substrate Spectrum for Methylacidiphilum fumariolicum SolV. Front. Microbiol. 11:604485. doi: 10.3389/fmicb.2020.604485
Received: 10 September 2020; Accepted: 23 November 2020;
Published: 14 December 2020.
Edited by:
Mark Alexander Lever, ETH Zürich, SwitzerlandReviewed by:
Marina G. Kalyuzhanaya, San Diego State University, United StatesColin Murrell, University of East Anglia, United Kingdom
Copyright © 2020 Picone, Mohammadi, Waajen, van Alen, Jetten, Pol and Op den Camp. This is an open-access article distributed under the terms of the Creative Commons Attribution License (CC BY). The use, distribution or reproduction in other forums is permitted, provided the original author(s) and the copyright owner(s) are credited and that the original publication in this journal is cited, in accordance with accepted academic practice. No use, distribution or reproduction is permitted which does not comply with these terms.
*Correspondence: Huub J. M. Op den Camp, aC5vcGRlbmNhbXBAc2NpZW5jZS5ydS5ubA==