- 1Faculty of Infectious and Tropical Diseases, London School of Hygiene & Tropical Medicine, London, United Kingdom
- 2”Alexander Fleming” Biomedical Sciences Research Center, Vari, Greece
- 3LifeArc, Accelerator Building, Open Innovation Campus, Stevenage, United Kingdom
The single-celled apicomplexan parasite Plasmodium falciparum is responsible for the majority of deaths due to malaria each year. The selection of drug resistance has been a recurring theme over the decades with each new drug that is developed. It is therefore crucial that future generations of drugs are explored to tackle this major public health problem. Cyclic GMP (cGMP) signaling is one of the biochemical pathways that is being explored as a potential target for new antimalarial drugs. It has been shown that this pathway is essential for all of the key developmental stages of the complex malaria parasite life cycle. This gives hope that targeting cGMP signaling might give rise to drugs that treat disease, block its transmission and even prevent the establishment of infection. Here we review previous work that has been carried out to develop and optimize inhibitors of the cGMP-dependent protein kinase (PKG) which is a critical regulator of the malaria parasite life cycle.
Introduction
Malaria kills more than 400,000 people each year, mainly young children in Africa (WHO, 2019), despite the availability of effective control measures and drug treatments. The selection and spread of drug resistant malaria parasites has made the development of new interventions an urgent priority so that increases in clinical cases and mortality can be avoided. High throughput screening of large chemical libraries has led to a number of promising new candidate antimalarial drugs that are progressing through clinical trials, but we cannot be sure how many of these will be rolled out as new treatments. It is therefore necessary that we continue to explore alternative drug targets that operate through different modes of action and progress the next generation of drugs to combat the serious problem of drug resistance.
One of the targets that has received significant attention in recent years is the cyclic GMP (cGMP)-dependent protein kinase (PKG) which is the central regulator of cGMP signaling in malaria parasites (Baker et al., 2017a; Cabrera et al., 2018). Cyclic GMP is an intracellular messenger molecule that is synthesized from GTP by guanylyl cyclase and broken down (hydrolyzed) by phosphodiesterases (PDEs). The cellular levels of cGMP are balanced by the opposing activities of these two enzyme classes which are in turn regulated by as yet unknown mechanisms. As cGMP reaches a threshold concentration in the cell, it binds to and activates PKG which can then phosphorylate its many intracellular protein substrates. The cGMP signaling pathway is present in most organisms and controls myriad processes across the animal kingdom ranging from transducing environmental signals (Bosgraaf and Van Haastert, 2002) and mediating locomotion in some protozoa (Linder et al., 1999) to controlling cardiovascular function (Bork and Nikolaev, 2018) and regulating phototransduction in humans (Stryer, 1986).
Protozoan parasites of the genus Plasmodium which cause malaria have a complex life cycle, with specialized forms adapted to both establishing infection and proliferation within the mammalian host and specific forms which mediate transmission to and from the Anopheles mosquito vector. We and others have demonstrated that cGMP signaling plays an essential role in all of the important stages of the Plasmodium life cycle (McRobert et al., 2008; Taylor et al., 2008, 2010; Moon et al., 2009; Falae et al., 2010; Collins et al., 2013; Brochet et al., 2014; Lakshmanan et al., 2015; Govindasamy et al., 2016; Koussis et al., 2020) indicating that targeting this pathway with a drug would interrupt the life cycle making it plausible to treat disease, block transmission and even to prevent infection. Cyclic GMP signaling through PKG is required to stimulate the mobilization of calcium from internal stores which in turn activates life cycle stage-restricted calcium-dependent protein kinases (CDPKs) to continue the signaling cascade and stimulate cell function through reversible protein phosphorylation (Dvorin et al., 2010; Brochet et al., 2014; Fang et al., 2018). PKG is also known to stimulate a protease cascade in blood stage parasites which it initiates by triggering the release of the subtilisin-like protease SUB1 from apical organelles called exonemes (Dvorin et al., 2010; Collins et al., 2013; Thomas et al., 2018). Numerous P. falciparum schizont proteins have been identified which require the activity of PKG to be phosphorylated (Alam et al., 2015), but it is not known how these mediate its role in merozoite egress.
The Importance of a Small Gatekeeper Residue
A crucial structural feature of the parasite PKG underpins firstly the experimental approach that has been taken to study the function of PKG and secondly the utility of the enzyme as a drug target because this structural feature is not present in human PKG enzymes. This unique feature is simply the existence of a relatively small amino acid residue (threonine, Thr) in the so-called gatekeeper position of this protein kinase (McRobert et al., 2008). Human PKGs (and the majority of serine/threonine kinases) have a bulkier amino acid (e.g., Methionine, Met) at this position (Huang et al., 2010). The crucial consequence of having a small residue in this position is that it makes available a small hydrophobic pocket (the gatekeeper pocket) that adjoins the ATP-binding pocket (Donald et al., 2002; McRobert et al., 2008). Certain classes of small molecule ATP-competitive inhibitors can also occupy this small adjacent pocket with a protruding side chain which thereby confers exquisite inhibitor selectivity (Gum et al., 1998; Donald et al., 2002; Baker et al., 2017b). The presence of a bulky residue in the gatekeeper position prevents inhibitor access to the gatekeeper pocket and renders the kinase (and cell) insensitive to the inhibitor (Donald et al., 2002; McRobert et al., 2008). Exploitation of a kinase gatekeeper pocket to generate selective compounds has been used to tackle human disease by, for example, targeting p38α MAP kinase to inhibit cytokine-mediated inflammatory responses (Dominguez et al., 2005).
A research group at Merck working on the related parasite Eimeria (and the related genetically tractable Toxoplasma) first identified and utilized the small gatekeeper residue of apicomplexan PKGs to investigate the function of the enzyme in coccidian parasites (Donald et al., 2002) and importantly to demonstrate on-target activity of a potent anticoccidial agent they had identified by high throughput screening [a tri-substituted pyrrole, compound 1 (Gurnett et al., 2002)] and that of a more specific PKG inhibitor (an imidazopyridine, compound 2, Figure 1) derived from a medicinal chemistry effort (Donald et al., 2006). Importantly, 1 was also shown to have inhibitory activity against malaria parasites (Diaz et al., 2006) and has (along with 2) subsequently been used, in conjunction with transgenic lines expressing inhibitor resistant PKG alleles, to unequivocally determine the function of PKG throughout the malaria parasite life cycle (Baker et al., 2017a) (Figure 2). In the first such report, McRobert and colleagues generated a Plasmodium falciparum transgenic cell line in which the small gatekeeper residue (Thr618) was substituted with a bulkier glutamine (Gln) by allelic replacement at the endogenous PKG locus. Crucially, parasites expressing PKG with the bulky gatekeeper residue were several-fold less sensitive to compounds 1 and 2 than parental wild type parasites (McRobert et al., 2008). This approach of comparing the effects of a PKG inhibitor on wild type and gatekeeper mutant parasites has demonstrated an essential role for PKG in blood stage merozoite egress from red blood cells (Collins et al., 2013), release of male and female gametes from red blood cells (gametogenesis) (McRobert et al., 2008), maturation and motility of ookinetes (that develop following fertilization and zygote formation) (Moon et al., 2009; Brochet et al., 2014), motility of sporozoites and invasion of hepatocytes as well as release of merozoites from late liver stage parasites (Falae et al., 2010; Govindasamy et al., 2016). This combined chemical and genetic approach has demonstrated clearly that cGMP signaling mediated by PKG plays a vital role in a number of critical life cycle stage transitions and has simultaneously provided important data validating PKG as a tractable antimalarial drug target.
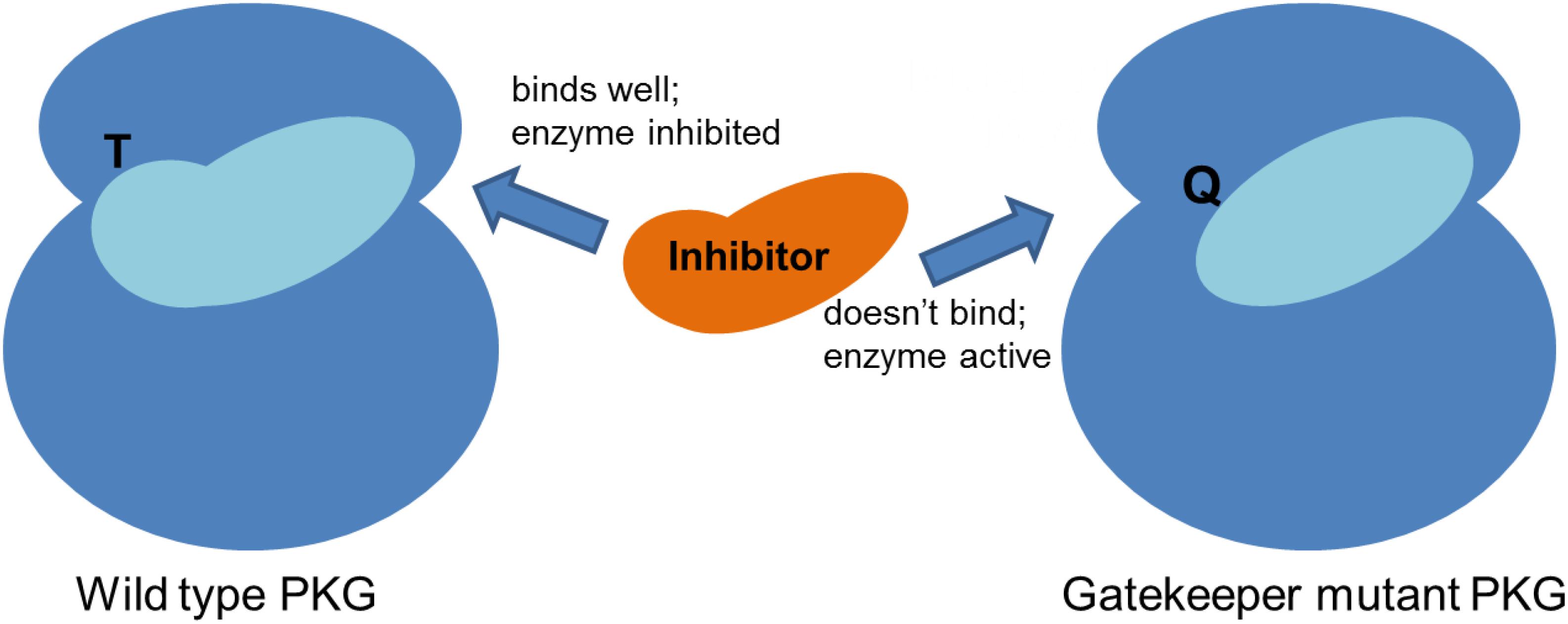
Figure 2. Schematic showing that inhibitors bind effectively to the malaria parasite PKG due to the presence of a small gatekeeper residue (threonine, T), which upon mutation to a bulkier residue (glutamine, Q), prevents inhibitor binding.
PKG Inhibitors Often Have Structurally-Related Secondary Targets
As previously mentioned, the gatekeeper mutant approach has facilitated functional analysis of PKG in apicomplexan parasites and drug target validation for PKG. However, the approach also inherently allows determination of whether PKG is the primary target of a PKG inhibitor of interest and also whether there are significant secondary targets (Donald et al., 2002; Wiersma et al., 2004; McRobert et al., 2008). It is important to realise that IC50 values (derived from purified recombinant PKG) of compounds 1 and 2 for PKG are several logs different for wild type and gatekeeper mutant PKG, suggesting that these inhibitors do not easily access the gatekeeper pocket when a bulky gatekeeper residue is present. However, when EC50 values are compared (derived from e.g., blood stage growth inhibition assays) for different PKG inhibitors, it is clear that compared to IC50 levels, the difference is relatively low (several-fold rather than log-fold) (Donald et al., 2002; Baker et al., 2017b). This at first is perplexing, but actually reflects the fact that some PKG inhibitors have significant secondary targets that they bind to in the cell. This was first recognized in the earlier studies by the Merck team working on compounds 1 and 2 with Eimeria and Toxoplasma (Donald et al., 2006) who identified both CDPK1 and the alpha isoform of casein kinase 1 (CK1) as secondary targets of compound 2. Importantly, it has proved possible by exploring Structure-Activity-Relationship studies (SAR) to generate PKG inhibitors with EC50 values that are also several logs different between wild type and gatekeeper parasites indicating that they are highly specific for PKG and that only very low levels of binding to secondary targets in the parasite and importantly the host occur (Baker et al., 2017b).
PKG Antimalarial Drug Discovery Projects
Baker et al. (2017b) reported a PKG inhibitor development program using the Merck anticoccidial imidazopyridine compound 2 (ML1) as a start point. This resulted in the generation of more potent and selective analogs. The most promising compound 3 (ML10, Figure 1) has an IC50 value of 160 pM against recombinant wild type P. falciparum PKG and of 29.5 μM against gatekeeper mutant PKG in which threonine 618 was replaced by a glutamine. Activity against a panel of 80 human kinases (including 14 small gatekeeper kinases) was low when tested at 100 nM which is >600-fold the IC50 value against recombinant P. falciparum PKG. Of note, activity against human PKGI and PKGII isoforms was also very low as expected since they have bulky gatekeeper residues. 3 also had a clean in vitro cytotoxicity profile when tested against HepG2 cells and other mammalian cell lines. The EC50 value of 3 in 72-hour blood stage growth inhibition assays using wild type parasites (clone 3D7) was 2.1 nM and was >1,100-fold higher (EC50 = 2.4 μM) in gatekeeper parasites. This demonstrates that 3 is highly specific for PKG and that only very low levels of binding to secondary targets occurs which holds promise for developing a drug that selectively targets PKG. Furthermore, this compound has an EC50 of 41.3 nM in a standard membrane feeding assay (SMFA) which measures the ability of an inhibitor to block development of oocysts in mosquitos. The inhibitory activity reflects the known essential role of PKG in male and female gamete formation and in ookinete development and motility. Importantly this study also provided in vivo proof of concept for PKG as an antimalarial drug target by utilizing a P. falciparum humanized SCID mouse model which demonstrated that 3 reduced blood stage parasitaemia to undetectable levels. However, this was achieved by orally dosing with 3 twice daily for 4 days with 100 mg/kg which is a high dose compared with some other candidates undergoing pre-clinical and clinical testing.
To gain insight into the detailed molecular interaction of this chemical series with Plasmodium PKG and to inform future medicinal chemistry optimization efforts, 3 (ML10) and the Merck compound 2 (ML1) were each co-crystallized with P. vivax PKG. This protein proved more amenable to co-crystallization studies but is a good surrogate for the P. falciparum PKG because the orthologues share 92% sequence identity and alignment of the apo structures of the kinase domains show a deviation from each other by a root-mean-square distance of only 0.3 Å. (Baker et al., 2017b). The previously predicted interaction of the fluorophenyl moiety of the chemical series with the gatekeeper pocket (Donald et al., 2002) (on which the chemical genetic approach is based) was confirmed for both compounds and the high degree of selectivity of 3 (ML10) could be explained by the co-structures which included a more pronounced exploitation of the gatekeeper pocket and beyond by 3 than 2, with the sulphonamide group of 3 extending into an additional cavity not reached by 2 (Baker et al., 2017b). The compound-free P. falciparum and P. vivax PKG full length structures obtained as part of this study were the first reported from any organism and contributed important insight into the mechanism by which the PKG is activated by cGMP (El Bakkouri et al., 2019).
One drawback of specific PKG inhibitors is that they are characterized by a moderate/slow killing rate in parasite reduction ratio (PRR) assays similar to that of pyrimethamine (Baker et al., 2017b; Matralis et al., 2019; Penzo et al., 2019). This is thought to be due to the fact that PKG is active within a very narrow temporal window of blood stage development (perhaps less than three hours) just prior to egress, which is thought to persist until completion of invasion (Taylor et al., 2010; Koussis et al., 2020). Specific PKG inhibitors have no activity against parasites outside of this window (Taylor et al., 2010).
Progress has also been reported to further explore and develop the imidazopyridine scaffold to improve key physicochemical parameters whilst retaining potency against blood stages. One aspect of this work focused on modification of the fluorophenyl moiety (that interacts with the gatekeeper pocket) of a set of bicyclic compounds and resulted in improved lipophilicity and in vitro ADME properties (Large et al., 2019a,b). For example, measured LogD could be improved from 2.4 (for compound 2) to 1.5 in key analogs (compound 4, Figure 1). In addition, stability was found to be moderate for compound 2 with 52% of this compound remaining after a 40-minute incubation with liver mouse microsomes. This liability was significantly improved by either appropriately substituting the amino group of 2 (compound 5, Figure 1) or by applying scaffold hopping approaches (compound 6, Figure 1). A parallel study also sought to optimize a set of monocyclic compounds (based on Merck trisubstituted pyrrole compound 1 that was developed to treat Eimeria infection in chickens), with the aims of improving structural alerts of the scaffold and increasing selectivity against human kinases. The design of the new molecules involved initially the replacement of the heteroaromatic pyrrole ring of 1 with its respective isostere thiazole followed by the replacement of the pyridine ring of 1 with that of 2-aminopyrimidine group existing in compound 2. As a result, the trisubstituted thiazole series was generated. Excellent selectivity over human kinases was obtained in this series by modification of the 4-fluorophenyl group (Tsagris et al., 2018). The best selectivity was achieved by the 4-fluoro-3-sulfonamidophenyl group (compound 7, Figure 1) which was also found to contribute significantly to the excellent PKG selectivity exhibited by 3. Compound 7 did not show inhibition across a set of 80 human kinases when measured at a concentration 50 times higher than biochemical IC50 against recombinant PfPKG. In addition, 7 showed an outstanding stability in both mouse and human microsomes (89 and 95% compound remaining, respectively, after 40 min of incubation), a remarkable aqueous solubility and LogD value (190 μM and 1.9, respectively) and good permeability (57 nm/s in the PAMPA assay).
To identify new chemical series that might overcome some of the limitations of existing PKG inhibitor scaffolds, a high throughput assay was developed to screen the GlaxoSmithKline Full Diversity collection of 1.7 million compounds in partnership with the Tres Cantos Open Lab Foundation. This identified nine clusters of interesting PKG inhibitor chemotypes including four clusters of trisubstituted heterocycles. The most interesting of these was a series of thiazoles that showed good potency against blood stage parasites and retained activity against gametogenesis as expected for compounds with low IC50 values against recombinant PKG enzyme activity. Hit expansion was performed using an available collection of more than 3,000 GSK thiazole derivatives. Measurement of EC50 values in 48- and 72-hour blood stage parasite growth inhibition assays indicated that some of the analogs might bind to additional targets apart from PKG. Specific PKG inhibitors exhibit a marked increase in EC50 in the 48-hour assay that reflects the narrow window of activity mentioned above. The existence of additional targets was confirmed using a chemoproteomic approach utilizing Kinobeads-based chemical proteomics. This identified additional targets including CDPK1, CDPK4, and NEK1 as additional targets for the thiazole derivatives studied (Penzo et al., 2019). Thiazoles have previously been developed by GSK as BRAF kinase inhibitors. For instance, Dabrafenib is used to treat a type of melanoma (Rheault et al., 2013) and it showed good selectivity against other human kinases with relatively mild toxicity (Falchook et al., 2014).
A medicinal chemistry project was subsequently carried out to further explore the potential of the thiazole series as antimalarials. The main goal of this research was to develop fast-kill agents given that the thiazole derivative 7 (Figure 1) developed in preceding studies still exhibits a moderately slow parasite killing rate. Toward this end, a chemical diversity SAR study was explored at all possible positions of the thiazole core ring, accompanied by isosterism approaches aiming at refining the structural determinants conferring fast-acting potency as well as favorable biopharmaceutical properties at the same time. Some of the compounds generated (compounds 8–10, Figure 1) had a very fast speed of kill comparable to that of artesunate which is most likely due to inhibition of additional targets. Furthermore, 8–10 exhibited potent transmission blocking activity, attributed possibly to their potent PKG inhibitory activity, and desired ADMET properties (aqueous solubility, permeability, human serum albumin binding, metabolic stability). Compound 10 also showed an improved cytotoxicity (using HepG2 cells) and cardiotoxicity (hERG inhibition) profile compared to compounds 7–9. Again, Kinobeads profiling was used to identify these targets and the most likely candidate conferring the fast killing properties is a protein kinase called SRPK2 (CLK2) which is currently under investigation. This kinase and others identified in both chemoproteomic studies are amongst the relatively small number of Plasmodium kinases that have small gatekeeper residues which explains why they can bind to PKG inhibitors that exploit the gatekeeper pocket. These results raise the possibility of optimizing one of the thiazole leads 8–10 which combines the desirable properties of a PKG inhibitor with the fast killing properties conferred by inhibition of an additional target, likely CLK2 (Matralis et al., 2019).
In another study Green and colleagues reported a series of imidazopyridazine inhibitors that showed potent activity against the P. falciparum calcium-dependent protein kinase CDPK1 in vitro (Ansell et al., 2014). Class 1 of this series (with a pyrimidine linker between the core and the substituted amine) exhibited activity against late P. falciparum schizonts and one of the compounds (Compound 11, Figure 1) had an EC50 value of 23 nM. However, the EC50 in the P. falciparum PKG T618Q gatekeeper mutant line was ∼20-fold higher than with wild type parasites revealing that the primary target of these compounds was actually PKG (Chapman et al., 2014; Green et al., 2015).
Recently, a team of scientists set out to identify the target of a tri-substituted imidazole (MMV030084, compound 12, Figure 1) that was previously shown to have activity against P. falciparum blood stages in a high throughput screen of a GSK library of around two million compounds (Gamo et al., 2010). Further characterization of the activity profile showed that the compound had activity specifically against late stage P. falciparum blood stages with EC50 values of 109 and 120 nM against two different lab isolates, male gamete formation (EC50 = 141 nM) and invasion of hepatocytes by sporozoites (EC50 = 199 nM). A range of approaches including chemoproteomics and the use of transgenic P. falciparum parasites identified PKG as the primary target of this compound which is consistent with the known essential roles of PKG at all of these life cycle stages. A particularly interesting aspect of the study was that resistance-selection experiments with MMV030084 (12) did not generate highly resistant mutants (Vanaerschot et al., 2020). One might anticipate that highly resistant parasites could readily be generated by selection of a mutant under PKG inhibitor pressure where the gatekeeper threonine of PKG might be replaced by a bulkier residue. However, this did not prove to be the case in either this study or our own unpublished work in partnership with the Tres Cantos Open lab Foundation using the imidazopyridine ML10 (compound 3, Figure 1). Reasons for this could include the fact that at least two base pairs would need to mutate to change the gatekeeper threonine to e.g., a glutamine and also that a threonine/glutamine substitution will likely have a fitness cost because although the PKG transgenic T618Q line is viable and can progress through the life cycle, we have shown that the kinetics of the PKG mutant enzyme are less favorable than wild type PKG with a ∼4-fold increase in Km value for ATP but a similar Vmax (Fang et al., 2018). Importantly, drug pressure using either ML10 (Cabrera et al., 2018) or MMV030084 (12) did not result in any mutation in the PKG sequence in either study. Vanaerschot and colleagues were able to select parasites with only low-level resistance to 12 which primarily relied on mutations in another protein kinase (tyrosine kinase-like protein 3) which is itself not a target of the compound (Vanaerschot et al., 2020). Together, the findings of this study strengthen the case for PKG as an antimalarial target worthy of additional study, not least because it appears to be a resistance-refractory target.
Apart from ATP-competitive inhibitors, cGMP analogs have also recently been explored as potential anti-malarials. It has been shown using biochemical and biophysical approaches that 8-NBD-cGMP, although having a similar affinity to cGMP, is a P. falciparum PKG antagonist with a 10-fold reduction of activation of PKG compared to cGMP (Byun et al., 2020). The effects on the parasite have so far not been reported.
Conclusion
PKG has emerged as an exciting potential antimalarial drug target, but at a time when there are a number of other excellent targets that are being progressed and that are undergoing pre-clinical testing and clinical trials. Time will tell whether PKG inhibitors will be pursued in future pre-clinical testing and beyond and will likely depend on how many of the more advanced candidates are rolled out as components of new antimalarial combination treatments.
Author Contributions
DB drafted the manuscript. SO, AM, JL, and MP co-wrote the manuscript. All authors contributed to the article and approved the submitted version.
Funding
The research described in this review that was carried out by the co-authors was supported by the following funders. The United Kingdom Medical Research Council, Developmental Pathway Funding Scheme grant (G10000779) funded a drug discovery partnership between the London School of Hygiene & Tropical Medicine and LifeArc. The Tres Cantos Open Lab Foundation (TC217) funded a PKG inhibitor chemistry program that supported AM. MP was funded by a Tres Cantos Open Lab Marie Curie Cofund Fellowship scheme supported by the 7th Framework Program of the European Commission through its Marie Curie Co-funding (Grant Agreement 291799) and a Tres Cantos Open Lab Foundation grant to DB (Ref TC167) Wellcome Trust (106240/Z/14/Z and 094752/Z/10/Z) funded DB’s research on cyclic nucleotide signaling in malaria parasites. Open Access Funding was arranged by the London School of Hygiene and Tropical Medicine through an institutional grant from the Wellcome Trust and contribution from LifArc and the Tres Cantos Open Lab Foundation.
Conflict of Interest
The authors declare that the research was conducted in the absence of any commercial or financial relationships that could be construed as a potential conflict of interest.
Acknowledgments
We would like to thank the United Kingdom Medical Research Council, the Tres Cantos Open Lab Foundation and the Wellcome Trust for funding the research carried out on PKG drug discovery by DB and his collaborators.
References
Alam, M. M., Solyakov, L., Bottrill, A. R., Flueck, C., Siddiqui, F. A., Singh, S., et al. (2015). Phosphoproteomics reveals malaria parasite Protein Kinase G as a signalling hub regulating egress and invasion. Nat. Commun. 6:7285.
Ansell, K. H., Jones, H. M., Whalley, D., Hearn, A., Taylor, D. L., Patin, E. C., et al. (2014). Biochemical and antiparasitic properties of inhibitors of the Plasmodium falciparum calcium-dependent protein kinase PfCDPK1. Antimicrob. Agents Chemother. 58, 6032–6043. doi: 10.1128/aac.02959-14
Baker, D. A., Drought, L. G., Flueck, C., Nofal, S. D., Patel, A., Penzo, M., et al. (2017a). Cyclic nucleotide signalling in malaria parasites. Open Biol. 7:170213. doi: 10.1098/rsob.170213
Baker, D. A., Stewart, L. B., Large, J. M., Bowyer, P. W., Ansell, K. H., Jimenez-Diaz, M. B., et al. (2017b). A potent series targeting the malarial cGMP-dependent protein kinase clears infection and blocks transmission. Nat. Commun. 8:430.
Bork, N. I., and Nikolaev, V. O. (2018). cGMP signaling in the cardiovascular system-the role of compartmentation and its live cell imaging. Int. J. Mol. Sci. 19:801. doi: 10.3390/ijms19030801
Bosgraaf, L., and Van Haastert, P. J. (2002). A model for cGMP signal transduction in dictyostelium in perspective of 25 years of cGMP research. J. Muscle Res. Cell Motil. 23, 781–791.
Brochet, M., Collins, M. O., Smith, T. K., Thompson, E., Sebastian, S., Volkmann, K., et al. (2014). Phosphoinositide metabolism links cGMP-dependent protein kinase G to essential Ca(2)(+) signals at key decision points in the life cycle of malaria parasites. PLoS Biol. 12:e1001806. doi: 10.1371/journal.pbio.1001806
Byun, J. A., Van, K., Huang, J., Henning, P., Franz, E., Akimoto, M., et al. (2020). Mechanism of allosteric inhibition in the Plasmodium falciparum cGMP-dependent protein kinase. J. Biol. Chem. 295, 8480–8491. doi: 10.1074/jbc.ra120.013070
Cabrera, D. G., Horatscheck, A., Wilson, C. R., Basarab, G., Eyermann, C. J., and Chibale, K. (2018). Plasmodial kinase inhibitors: license to cure? J. Med. Chem. 61, 8061–8077. doi: 10.1021/acs.jmedchem.8b00329
Chapman, T. M., Osborne, S. A., Wallace, C., Birchall, K., Bouloc, N., Jones, H. M., et al. (2014). Optimization of an imidazopyridazine series of inhibitors of Plasmodium falciparum calcium-dependent protein kinase 1 (PfCDPK1). J. Med. Chem. 57, 3570–3587. doi: 10.1021/jm500342d
Collins, C. R., Hackett, F., Strath, M., Penzo, M., Withers-Martinez, C., Baker, D. A., et al. (2013). Malaria parasite cGMP-dependent protein kinase regulates blood stage merozoite secretory organelle discharge and egress. PLoS Pathog. 9:e1003344. doi: 10.1371/journal.ppat.1003344
Diaz, C. A., Allocco, J., Powles, M. A., Yeung, L., Donald, R. G., Anderson, J. W., et al. (2006). Characterization of Plasmodium falciparum cGMP-dependent protein kinase (PfPKG): antiparasitic activity of a PKG inhibitor. Mol. Biochem. Parasitol. 146, 78–88. doi: 10.1016/j.molbiopara.2005.10.020
Dominguez, C., Powers, D. A., and Tamayo, N. (2005). p38 MAP kinase inhibitors: many are made, but few are chosen. Curr. Opin. Drug Discov. Dev. 8, 421–430.
Donald, R. G., Allocco, J., Singh, S. B., Nare, B., Salowe, S. P., Wiltsie, J., et al. (2002). Toxoplasma gondii cyclic GMP-dependent kinase: chemotherapeutic targeting of an essential parasite protein kinase. Eukaryot. Cell 1, 317–328. doi: 10.1128/ec.1.3.317-328.2002
Donald, R. G., Zhong, T., Wiersma, H., Nare, B., Yao, D., Lee, A., et al. (2006). Anticoccidial kinase inhibitors: identification of protein kinase targets secondary to cGMP-dependent protein kinase. Mol. Biochem. Parasitol. 149, 86–98. doi: 10.1016/j.molbiopara.2006.05.003
Dvorin, J. D., Martyn, D. C., Patel, S. D., Grimley, J. S., Collins, C. R., Hopp, C. S., et al. (2010). A plant-like kinase in Plasmodium falciparum regulates parasite egress from erythrocytes. Science 328, 910–912. doi: 10.1126/science.1188191
El Bakkouri, M., Kouidmi, I., Wernimont, A. K., Amani, M., Hutchinson, A., Loppnau, P., et al. (2019). Structures of the cGMP-dependent protein kinase in malaria parasites reveal a unique structural relay mechanism for activation. Proc. Natl. Acad. Sci. U.S.A. 116, 14164–14173. doi: 10.1073/pnas.1905558116
Falae, A., Combe, A., Amaladoss, A., Carvalho, T., Menard, R., and Bhanot, P. (2010). Role of Plasmodium berghei cGMP-dependent protein kinase in late liver stage development. J. Biol. Chem. 285, 3282–3288. doi: 10.1074/jbc.m109.070367
Falchook, G. S., Long, G. V., Kurzrock, R., Kim, K. B., Arkenau, H. T., Brown, M. P., et al. (2014). Dose selection, pharmacokinetics, and pharmacodynamics of BRAF inhibitor dabrafenib (GSK2118436). Clin. Cancer Res. 20, 4449–4458. doi: 10.1158/1078-0432.ccr-14-0887
Fang, H., Gomes, A. R., Klages, N., Pino, P., Maco, B., Walker, E. M., et al. (2018). Epistasis studies reveal redundancy among calcium-dependent protein kinases in motility and invasion of malaria parasites. Nat. Commun. 9:4248.
Gamo, F. J., Sanz, L. M., Vidal, J., de Cozar, C., Alvarez, E., Lavandera, J. L., et al. (2010). Thousands of chemical starting points for antimalarial lead identification. Nature 465, 305–310. doi: 10.1038/nature09107
Govindasamy, K., Jebiwott, S., Jaijyan, D. K., Davidow, A., Ojo, K. K., Van Voorhis, W. C., et al. (2016). Invasion of hepatocytes by Plasmodium sporozoites requires cGMP-dependent protein kinase and calcium dependent protein kinase 4. Mol. Microbiol. 102, 349–363. doi: 10.1111/mmi.13466
Green, J. L., Moon, R. W., Whalley, D., Bowyer, P. W., Wallace, C., Rochani, A., et al. (2015). Imidazopyridazine Inhibitors of Plasmodium falciparum calcium-dependent protein kinase 1 also target cyclic GMP-dependent protein kinase and heat shock protein 90 to kill the parasite at different stages of intracellular development. Antimicrob. Agents Chemother. 60, 1464–1475. doi: 10.1128/aac.01748-15
Gum, R. J., McLaughlin, M. M., Kumar, S., Wang, Z., Bower, M. J., Lee, J. C., et al. (1998). Acquisition of sensitivity of stress-activated protein kinases to the p38 inhibitor, SB 203580, by alteration of one or more amino acids within the ATP binding pocket. J. Biol. Chem. 273, 15605–15610. doi: 10.1074/jbc.273.25.15605
Gurnett, A. M., Liberator, P. A., Dulski, P. M., Salowe, S. P., Donald, R. G., Anderson, J. W., et al. (2002). Purification and molecular characterization of cGMP-dependent protein kinase from Apicomplexan parasites. A novel chemotherapeutic target. J. Biol. Chem. 277, 15913–15922. doi: 10.1074/jbc.m108393200
Huang, D., Zhou, T., Lafleur, K., Nevado, C., and Caflisch, A. (2010). Kinase selectivity potential for inhibitors targeting the ATP binding site: a network analysis. Bioinformatics 26, 198–204. doi: 10.1093/bioinformatics/btp650
Koussis, K., Withers-Martinez, C., Baker, D. A., and Blackman, M. J. (2020). Simultaneous multiple allelic replacement in the malaria parasite enables dissection of PKG function. Life Sci. Alliance 3:e201900626. doi: 10.26508/lsa.201900626
Lakshmanan, V., Fishbaugher, M. E., Morrison, B., Baldwin, M., Macarulay, M., Vaughan, A. M., et al. (2015). Cyclic GMP balance is critical for malaria parasite transmission from the mosquito to the mammalian host. mBio 6:e02330.
Large, J. M., Birchall, K., Bouloc, N. S., Merritt, A. T., Smiljanic-Hurley, E., Tsagris, D. J., et al. (2019a). Potent bicyclic inhibitors of malarial cGMP-dependent protein kinase: approaches to combining improvements in cell potency, selectivity and structural novelty. Bioorg. Med. Chem. Lett. 29:126610. doi: 10.1016/j.bmcl.2019.08.014
Large, J. M., Birchall, K., Bouloc, N. S., Merritt, A. T., Smiljanic-Hurley, E., Tsagris, D. J., et al. (2019b). Potent inhibitors of malarial P. Falciparum protein kinase G: improving the cell activity of a series of imidazopyridines. Bioorg. Med. Chem. Lett. 29, 509–514. doi: 10.1016/j.bmcl.2018.11.039
Linder, J. U., Engel, P., Reimer, A., Kruger, T., Plattner, H., Schultz, A., et al. (1999). Guanylyl cyclases with the topology of mammalian adenylyl cyclases and an N-terminal P-type ATPase-like domain in Paramecium, Tetrahymena and Plasmodium. Embo J. 18, 4222–4232. doi: 10.1093/emboj/18.15.4222
Matralis, A. N., Malik, A., Penzo, M., Moreno, I., Almela, M. J., Camino, I., et al. (2019). Development of chemical entities endowed with potent fast-killing properties against Plasmodium falciparum malaria parasites. J. Med. Chem. 62, 9217–9235. doi: 10.1021/acs.jmedchem.9b01099
McRobert, L., Taylor, C. J., Deng, W., Fivelman, Q. L., Cummings, R. M., Polley, S. D., et al. (2008). Gametogenesis in malaria parasites is mediated by the cGMP-dependent protein kinase. PLoS Biol. 6:e139. doi: 10.1371/journal.pbio.0060139
Moon, R. W., Taylor, C. J., Bex, C., Schepers, R., Goulding, D., Janse, C. J., et al. (2009). A cyclic GMP signalling module that regulates gliding motility in a malaria parasite. PLoS Pathog. 5:e1000599. doi: 10.1371/journal.ppat.1000599
Penzo, M., de Las Heras-Duena, L., Mata-Cantero, L., Diaz-Hernandez, B., Vazquez-Muniz, M. J., Ghidelli-Disse, S., et al. (2019). High-throughput screening of the Plasmodium falciparum cGMP-dependent protein kinase identified a thiazole scaffold which kills erythrocytic and sexual stage parasites. Sci. Rep. 9:7005.
Rheault, T. R., Stellwagen, J. C., Adjabeng, G. M., Hornberger, K. R., Petrov, K. G., Waterson, A. G., et al. (2013). Discovery of dabrafenib: a selective inhibitor of raf kinases with antitumor activity against B-Raf-Driven tumors. ACS Med. Chem. Lett. 4, 358–362. doi: 10.1021/ml4000063
Stryer, L. (1986). Cyclic GMP cascade of vision. Annu. Rev. Neurosci. 9, 87–119. doi: 10.1146/annurev.ne.09.030186.000511
Taylor, C. J., McRobert, L., and Baker, D. A. (2008). Disruption of a Plasmodium falciparum cyclic nucleotide phosphodiesterase gene causes aberrant gametogenesis. Mol. Microbiol. 69, 110–118. doi: 10.1111/j.1365-2958.2008.06267.x
Taylor, H. M., McRobert, L., Grainger, M., Sicard, A., Dluzewski, A. R., Hopp, C. S., et al. (2010). The malaria parasite cyclic GMP-dependent protein kinase plays a central role in blood-stage schizogony. Eukaryot. Cell 9, 37–45. doi: 10.1128/ec.00186-09
Thomas, J. A., Tan, M. S. Y., Bisson, C., Borg, A., Umrekar, T. R., Hackett, F., et al. (2018). A protease cascade regulates release of the human malaria parasite Plasmodium falciparum from host red blood cells. Nat. Microbiol. 3, 447–455. doi: 10.1038/s41564-018-0111-0
Tsagris, D. J., Birchall, K., Bouloc, N., Large, J. M., Merritt, A., Smiljanic-Hurley, E., et al. (2018). Trisubstituted thiazoles as potent and selective inhibitors of Plasmodium falciparum protein kinase G (PfPKG). Bioorg. Med. Chem. Lett. 28, 3168–3173. doi: 10.1016/j.bmcl.2018.08.028
Vanaerschot, M., Murithi, J. M., Pasaje, C. F. A., Ghidelli-Disse, S., Dwomoh, L., Bird, M., et al. (2020). Inhibition of resistance-refractory P. falciparum kinase PKG delivers prophylactic, blood stage, and transmission-blocking antiplasmodial activity. Cell Chem Biol. 27, 80.e8–81.e8.
Keywords: malaria, cyclic GMP, signal transduction, Plasmodium falciparum, drug discovery
Citation: Baker DA, Matralis AN, Osborne SA, Large JM and Penzo M (2020) Targeting the Malaria Parasite cGMP-Dependent Protein Kinase to Develop New Drugs. Front. Microbiol. 11:602803. doi: 10.3389/fmicb.2020.602803
Received: 04 September 2020; Accepted: 23 November 2020;
Published: 17 December 2020.
Edited by:
Annette Elizabeth Kaiser, University of Duisburg-Essen, GermanyReviewed by:
Isabelle Florent, Muséum National d’Histoire Naturelle, FranceSandra Marcia Muxel, University of São Paulo, Brazil
Copyright © 2020 Baker, Matralis, Osborne, Large and Penzo. This is an open-access article distributed under the terms of the Creative Commons Attribution License (CC BY). The use, distribution or reproduction in other forums is permitted, provided the original author(s) and the copyright owner(s) are credited and that the original publication in this journal is cited, in accordance with accepted academic practice. No use, distribution or reproduction is permitted which does not comply with these terms.
*Correspondence: David A. Baker, ZGF2aWQuYmFrZXJAbHNodG0uYWMudWs=