- Citrus Research Institute, Southwest University, Chongqing, China
Based on intracellular second messenger cAMP, the cyclic AMP-protein kinase A (cAMP-PKA) pathway transforms extracellular stimuli to activate effectors and downstream signaling components, mediating physiological processes in filamentous fungi. The concentration of intracellular cAMP was regulated by adenylate cyclase biosynthesis and cAMP phosphodiesterase (PDEs) hydrolysis, which mediate signal transduction and termination. In this study, we used a gene deletion and complementary strategy to characterize the functions of AaPdel and AaPdeh genes, which encoded low-affinity PDEs (Pdel) and high-affinity PDEs (Pdeh), respectively, in Alternaria alternata. AaPdel, but not AaPdeh, was found to be a key regulator in conidiation and pathogenesis in A. alternata. ΔAaPdel showed defects in conidiation, producing approximately 65% reduced conidiation and forming lowly pigmented aberrant structures. In response to osmotic stress, ΔAaPdel was more sensitive to non-ionic osmotic stress than ionic osmotic stress. Moreover, AaPdel deletion mutants had defects in vegetative growth and hyphal growth. Further analyses showed that the high chitin content of ΔAaPdel might account for the sensitivity to Congo red. Based on the attenuated pathogenicity and lowly pigmented aberrant structures, the laccase activity analysis found that both AaPdel and AaPdeh were involved in laccase activity regulation. Our data further support the PKA-mediated cAMP signaling pathway, as we have found that AaPdel was involved in intracellular cAMP levels in A. alternata.
Introduction
Seven pathotypes of Alternaria alternata have been identified in tangerine, Japanese pear, strawberry, apple, tomato, rough lemon, and tobacco (Hatta et al., 2002; Thomma, 2003). Alternaria brown spot disease (ABS) caused by the tangerine pathotype of A. alternata reduces quality and yield of tangerine production in China (Lee et al., 2015). Under the optimum condition of 28°C and high humidity, the A. alternata infected both leaves, twigs, and fruits, forming necrosis, and spread extensively (Solel and Kimchi, 1998). Infected leaves act as the primary infection source, and conidia are derived from the lesions of infected leaves (Wang et al., 2018). As a necrotrophic fungus, the tangerine pathotype of A. alternata produced the host selective toxin A. citri toxin (ACT) to kill and colonize the host cells (Tsuge et al., 2013; Ma et al., 2019). In the infection process, the ACT toxin spreads along the veins of the leaves, forming irregular “v”-shaped or like-round lesions. By causing electrolyte leakage, tangerine and its hybrids are susceptible to the ACT toxin (Otani et al., 1995; Ito et al., 2004).
In filamentous fungi, cells respond to external stimuli through cell-surface receptors and complex signal transduction pathways. The cyclic AMP-protein kinase A (cAMP-PKA) pathway is one of the major studied pathways mediating physiological processes in filamentous fungi (Zhu et al., 2017). Based on the cAMP, intracellular second messenger, extracellular stimuli could be transformed to activate effectors and stimulate downstream signaling components (Calvo et al., 2002). The cAMP-PKA pathway consists of G protein-coupled receptors (GPCRs), heterotrimeric G proteins, adenylate cyclase (AC), cAMP, and protein kinase A (PKA) (Houslay et al., 2007). The concentration of intracellular cAMP was regulated by adenylate cyclase biosynthesis and cAMP phosphodiesterase (PDEs) hydrolysis, which mediated signal transduction and termination (Jin et al., 1992). Two identified GTP-binding proteins, Ras1 and Ras2, regulate cAMP production by activating adenylate cyclase (Ramanujam and Naqvi, 2010). And PDEs were directly acting on active site, mediating cAMP hydrolysis to 5′-AMP (Conti and Beavo, 2007; Wilson et al., 2010). PDEs play an important role in the cAMP-PKA pathway, but their specific functions in A. alternata remain poorly understood.
In Saccharomyces cerevisiae, cAMP levels are modulated by two cAMP phosphodiesterase, low-affinity phosphodiesterase Pdel, and high-affinity phosphodiesterase Pde2. There is no obvious homology between Pde1 and Pde2. Simultaneously knockouting of Pde1 and Pde2 doubled the cAMP concentration, increased sensitivity to heat shock, and growth defects in non-fermentable carbon sources (Londesborough and Suoranta, 1983; Uno et al., 1983). Pde2 regulates cAMP level induced by glucose stimulation, while there is no significant difference of cAMP level between the Pde1 deletion mutant and the wild-type strain (Hu et al., 2010). Pde1 does not regulate the cAMP level directly, but mediates a PKA negative feedback loop (Wera et al., 1997; Ma et al., 1999; Zhao et al., 2007; Caza and Kronstad, 2019).
In plant-microbe interactions, the fungus Magnaporthe oryzae PdeH (Pde2) has a dominant role in conidiation, pathogenicity, and intracellular cAMP level regulation. Compared with the wild-type strain, simultaneous knockout of PdeH and PdeL resulted in a 10-fold increase in cAMP level and completed disappearance of pathogenicity, whereas PdeL (Pde1) had no obvious function in M. oryzae (Zhang et al., 2011). The expression of GFP protein shows that PdeH localizes predominantly to the cytoplasm, while PdeL locates to the nucleus. PdeH-involved cAMP signaling is crucial for signal transduction and pathogenicity in M. oryzae (Ramanujam and Naqvi, 2010). In Candida albicans, the PDE2 deletion mutant exhibits reduced invasion and virulence. In the simultaneous knockout of CaPDE2 and CaPDE1, pathogenicity is abolished completely, indicating that Pde1 contributes to virulence as a secondary role (Wilson et al., 2010). In phytopathogenic fungus Botrytis cinerea, the Δbcpde2 exhibits a significant reduction in vegetative growth, spore formation, germination, and pathogenicity, but the Δbcpde1 displays a similar phenotype to the wild-type strain (Harren et al., 2013). In Fusarium graminearum, inactivation of PDE2 but not PDE1 results in activating PKA activities and increases DON production (Jiang et al., 2016).
In A. alternata, the cAMP-PKA pathway remains poorly understood, and no regulated genes have been identified. In this study, characterization of PDEs in A. alternata have been conducted. We have identified high and low-affinity cAMP phosphodiesterase in A. alternata and through targeted gene deletion, analyzed the gene function in vegetative growth, conidiation, stress response, cell wall integrity (CWI), intracellular cAMP level, laccase activity, and pathogenicity.
Materials and Methods
Strains and Growth Condition
The A. alternata Z7 was used as a wild-type strain in this study (Wang et al., 2016). And the fungal strains defective of AaPdel, coding low-affinity cAMP phosphodiesterase, and AaPdeh, coding high-affinity cAMP phosphodiesterase, were used as deletion mutants (ΔAaPdel and ΔAaPdeh). For activating strains and determining characteristics of colonies, all strains were cultured on potato dextrose agar (PDA) plates for 4–8 days at 28°C. After targeted gene deletion, the resulting transformants were selected on TB3 medium (yeast extract 3 g, casamino acids 3 g, sucrose 200 g, and agar 7.5 g per liter of purified water) containing hygromycin (Kong et al., 2018). On the vegetative growth analyses, the wild type and deletion mutants were cultured on minimal medium (KCl 0.5 g, NaNO3 2 g, KH2PO4 1 g, MgSO4⋅7H2O 0.5 g, FeSO4 0.01 g, sucrose 10 g, trace elements 200 μL and agar 20 g per liter of purified water) and complete medium (per liter of minimal medium supplied with yeast extract 1 g, casein hydrolysate 1 g, and peptone 2 g), respectively (Tang et al., 2020).
Targeted Gene Deletion and Complementation Analysis
Gene replacement through the hygromycin-resistance cassette released from pCX62 was used to generate the deletion mutants (Dong et al., 2015). The upper fragment of the target gene (S1) and hygromycin B gene (H1) were amplified and fused together by two-step overlapping PCR. And the lower fragment of the target gene (S2) and hygromycin B gene (H2) were fused to another fragment. The two resulting fragments with a hygromycin-resistance cassette were introduced into the protoplasts of the wild type by the polyethylene glycol (PEG)-mediated knockout technique (Rehman et al., 2016). To confirm the correct gene replacement, the resulting transformants resistant to hygromycin (TB3 medium with 250 mg/ml hygromycin), were identified by PCR assay and southern blot analysis.
Conidiation
The conidia of strains were harvested by blending with distilled water and scraping the colonies grown on V8 medium at 28°C for 8 days (Timmer et al., 1998). After filtering into two layers of sterile gauze, conidia were quantified by hemocytometer (QIUJING, China) and microscope (OLYMPUS, Japan). The conidia germinating rate and conidia diameter were assessed by microscopic examination of 200 conidia each for at least three repetitions. The statistical significance on conidiation of wild type, deletion mutants and complementary strains, was determined by one-way ANOVA and Duncan’s new multiple range test (P < 0.05).
Nucleic Acid Manipulation
The homology of the targeted gene and protein sequences were searched in the Blast program and resources at NCBI1. Analyses of protein subcellular localization were predicted by WoLF PSORT2. Fungal genomic DNA and total RNA extraction were extracted from the mycelia grown in PDB medium for 36 h at 28°C. Fungal genomic DNA extraction was carried out using CTAB as previously described, and total RNA extraction was performed using Trizol (Invitrogen). Plasmid DNA was purified using the Plasmid DNA Miniprep Kit (Qiagen).
Vegetable Growth and Stress Treatment
Four kinds of growth medium [PDA, V8, minimal medium (MM), and complete medium (CM)] were configured to determine the involvement of vegetative growth between deletion mutants and wild-type strain. To analyse the involvement of AaPdel and AaPdeh in cell wall integrity and osmotic stress response, 3 days activated strains were inoculated on PDA amended with different chemicals. The same concentration of osmotic-stress inducer, 1M sorbitol, 1M NaCl, 1M KCl, or 1M sucrose was added to the PDA plate, respectively. And SDS or Congo red (CR) inducing medium was configured to analyse the cell wall integrity of strains. The diameter of colonies were measured in two perpendicular directions after 5 days of incubation at 28°C, and each treatment was replicated for at least three times. And the growth rates were presented as the mean ± SD of at least three repeats. Statistical significance of growth rates of mutants and wild type was determined by one-way ANOVA and Duncan’s new multiple range test (MRT) (P < 0.05).
Quantification of Intracellular cAMP
Mycelia, grown in PDB medium for 36 h at 28°C and freeze-dried for 1.5 h, were quickly ground in liquid nitrogen and mixed with 200 μL of 6% trichloroacetic acid (TCA). Intracellular cAMP extraction was in accordance with the method described previously (Liu et al., 2007), and cAMP levels were measured using the AlphaScreen Assay Kit (PerkinElmer, Waltham, MA) according to the supplier’s instruction.
Pathogenicity Assay
In the pathogenicity assay, the 10 days conidia harvested from V8 medium were diluted to a concentration of 1.0×105 conidia ml–1. At least 20 leaves of tangerine (Citrus reticulata Blanco) with nearly identified size and maturity, were inoculated with 20 μL conidial suspension of wild type, deletion mutants, and complementary mutants (Solel and Kimchi, 1998). Distilled water was inoculated on the leaves as a positive control. The phenotype was recorded at 3–5 dpi (days post inoculation), with three repetitions in each treatment. According to Perina et al. (2019) the average scale levels based on the percentage of lesion area were quantified.
Laccase Activity Assay
Laccase activity was quantified as described previously (Mtibaa et al., 2018). Based on the oxidation of ABTS, the activated strains of wild type, ΔAaPdel, ΔAaPdeh, and complementary mutants were inoculated on the ABTS medium at 28°C. The morphology of these colonies was observed and photographed at 6 dpi. For the enzyme solution preparation, mycelial plugs of activated strains were cultured in 50 mL PDB liquid medium for 36 h. After filtration, mycelial pellets were harvested and mixed with 1 mL Tris-HCl. And the supernatant collected by centrifuge (10,000 rpm, 10 min) was the enzyme solution. Laccase activity was determined by 0.5 mM ABTS oxidation mixing in 0.1 M NaAc-HAc buffer, pH 4.0 at 28°C and measured the spectrophotometer absorbance at 420 nm. The resulted laccase activity was defined as the units U mL–1, with 1 U being the amount of enzyme oxidizing 1 μmol of ABTS per min (Irfan et al., 2018).
Statistical Analysis
Data was presented as the mean ± SD of at least three independent experiments. Statistical significance was analyzed by one-way ANOVA and Duncan’s new multiple range test (MRT). Different letters represent significant differences at P < 0.05.
Results
AaPdel, but Not AaPdeh, Is Involved in Mycelial Growth and Hyphal Growth
Through blasting S. cerevisiae Pde1 and Pde2 in the proteome of A. alternata, the corresponding OWY42401.1 and OWY42000.1 were identified by high protein homology, and named AaPdel and AaPdeh, respectively. The sequence alignment demonstrated that the AaPdel protein exhibited 18.50–52.59% similarity with S. cerevisiae ScPde1, M. oryzae MoPdel, C. albicans CaPdel, and the AaPdeh protein exhibited 30.26–69.59% similarity with S. cerevisiae ScPde2, M. oryzae MoPdeh, C. albicans CaPde2, and Botrytis cinerea Bcpde2. The phylogenetic tree of two identified proteins were shown in Figures 1C,D, respectively. The identified AaPdel gene encodes a 1,100-amino-acid polypeptide, and the identified AaPdeh gene encodes a 961-amino-acid polypeptide. As demonstrated in Figure 1A, the split-marker approach was used to generate deletion mutants. To confirm the correct gene replacement, the resulting transformants were selected based on the resistance to hygromycin (TB3 medium containing 250 mg/ml hygromycin). And AaPdel transformants were verified by three primer pairs of PLP1F/H855R, H856F/PLP2R, and PLF/PLR. AaPdeh transformants were identified through PHP1F/H855R, H856F/PHP2R, and PHF/PHR (Supplementary Figure 1). Southern blot analysis was used for further analysis of the deletion mutants. Genomic DNA of AaPdel and AaPdeh transformants were respectively, digested with HindIII, BgI II, XhoI, and SalI. A 5,535-bp fragment in the deletion mutants of AaPdel and a 4,531-bp fragment in the deletion mutants of AaPdeh were probed with HPH gene. And when probed with homologous target genes, respectively, 3,474 and 3,112-bp fragments were only found in the wild type, indicating correct replacement of the target genes (Figure 1B).
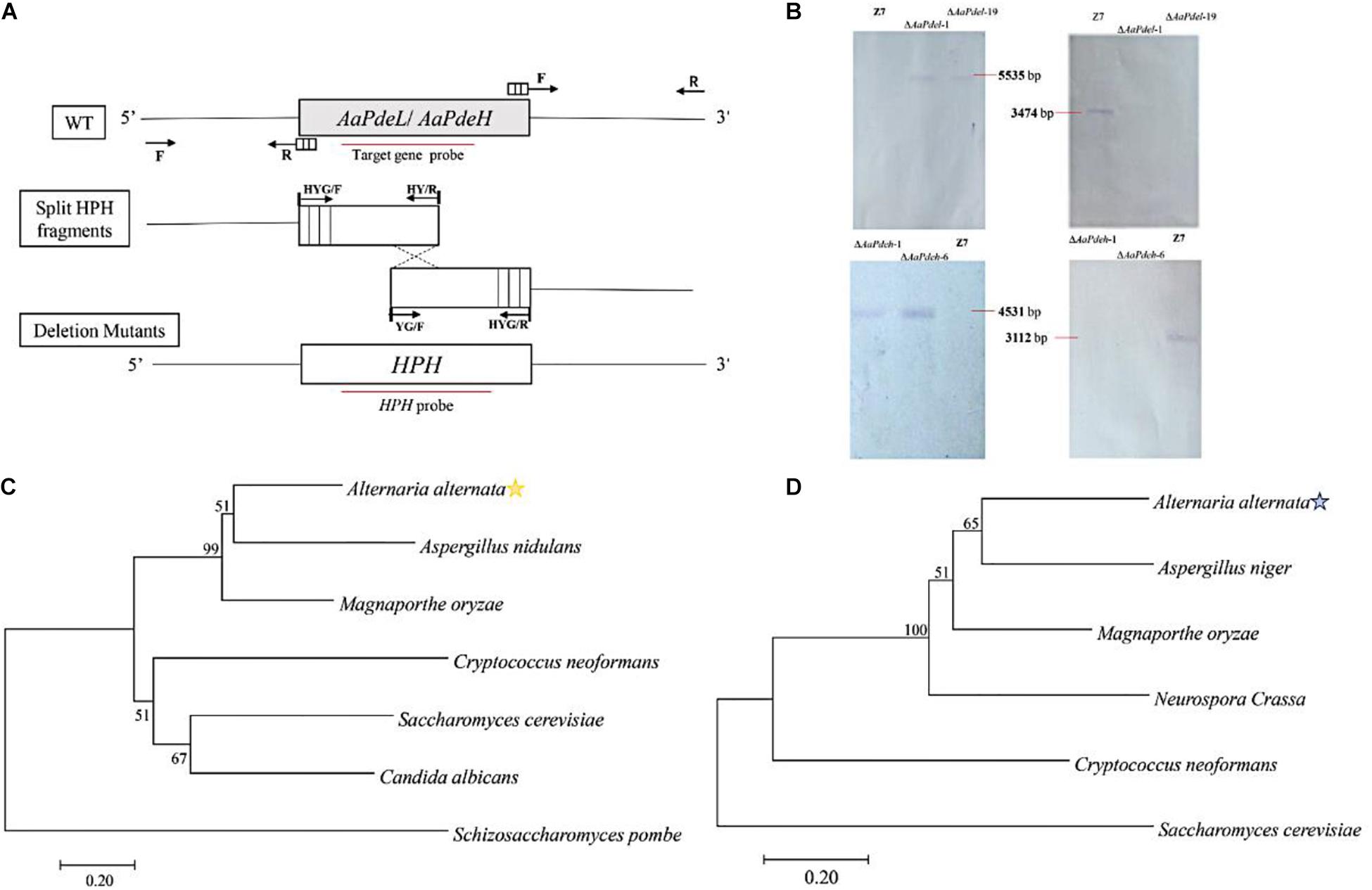
Figure 1. Constructionprotect and verification of the AaPdel and AaPdeh deletion and complementary mutants of Alternaria alternata. (A) Schematic of gene knockout. Targeted disruption of AaPdel and AaPdeh in A. alternata using split-marker gene replacement strategy. Through a homologous integration, overlapping DNA fragments of hygromycin-resistance gene cassette (HPH) were used for gene replacement. Arrows represented to primer binding sites, and the red line delineates the probe used for Southern blot analysis. (B) Southern blot analysis for the wild-type strain and deletion mutants. (C) The phylogenetic tree constructed based on the neighbor-joining method with 1,000 bootstrap replicates by MEGA7.0 program. Figures represent the occurrence percentage, and the value less than 50 was not shown. Phylogenetic tree generated based on the amino acid of AaPdel homologous. (D) Phylogenetic tree generated based on the amino acid of AaPdeh homologous.
The wild type, z7; deletion mutants, ΔAaPdel-1, ΔAaPdel-19, ΔAaPdeh-1, and ΔAaPdeh-6; and complement strains, AaPdel-cp and AaPdeh-cp, were inoculated on four vegetative mediums of PDA, V8, CM, and MM, respectively. The colony morphology and diameter were assessed after growing for 6 days and measured in two orthogonal directions. The ΔAaPdel displayed similar vegetative growth defects on the PDA, MM, CM, and V8 media (Figure 2A). On the CM medium with sufficient nutrient elements, the growth rates of ΔAaPdel were decreased by 14%, significantly lower than the corresponding WT. On the MM covering the basic nutrient elements of fungal growth, the percentage growth rates of ΔAaPdel were decreased by 7.5%. The ΔAaPdel reduced growth on regular PDA medium by 6.6–7.8% compared with the wild type (Figure 2B). By light microscope observation, the wild type exhibited abundant and thick hyphal branch, while the hyphal growth of AaPdel deletion mutants was sparse (Figure 2C). However, the fungal strains defective of AaPdeh, coding high-affinity cAMP phosphodiesterase, exhibited no obvious growth defects in radical growth and colony morphology, which were in line with the wild type. The above results indicate that the AaPdel gene is involved in the mycelial growth of A. alternata while AaPdeh has a limited contribution.
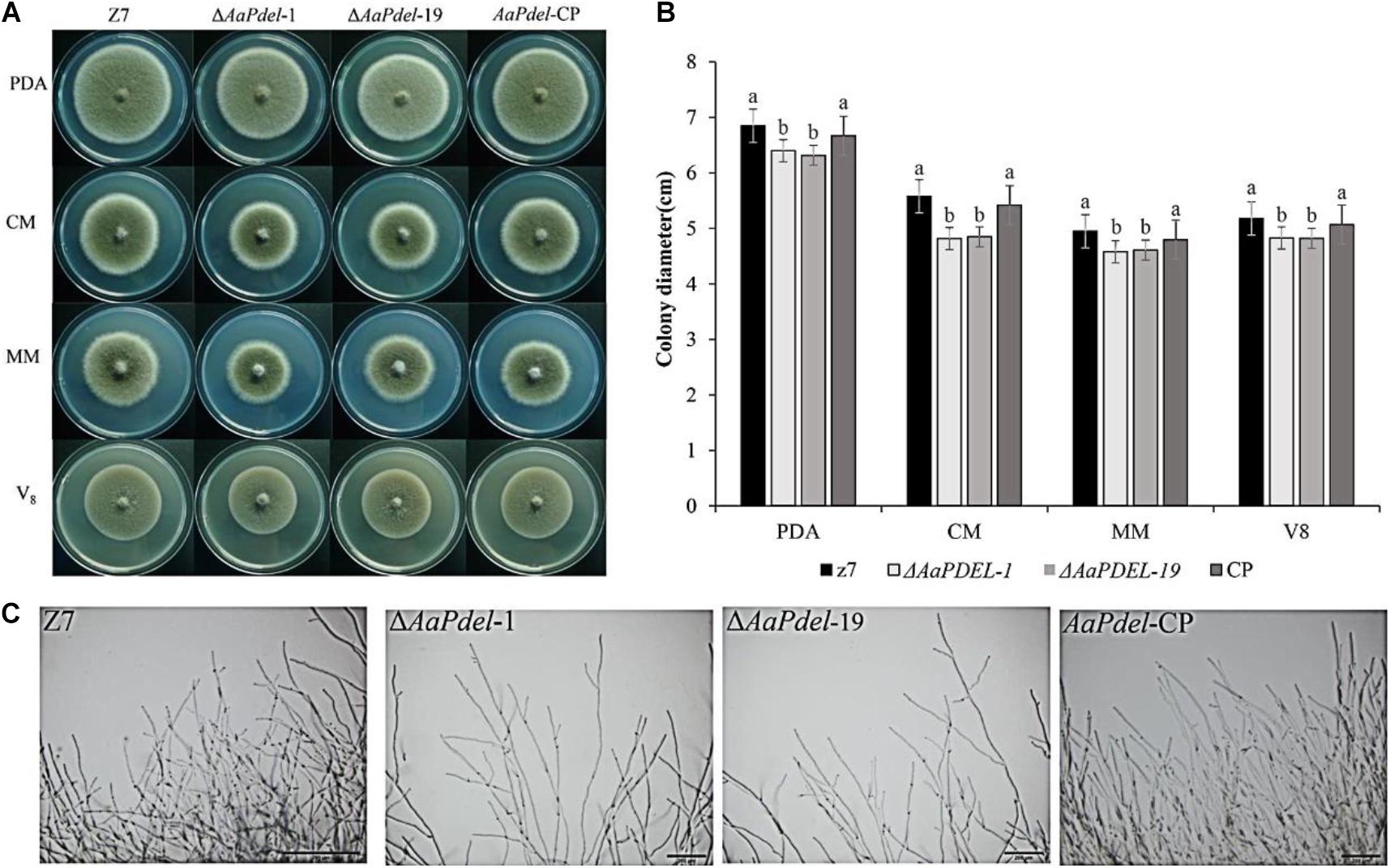
Figure 2. The deletion of AaPdel had significant effects on vegetative growth. (A) The phenotype of the wild-type strain, Z7; the deletion mutants, ΔAaPdel-1 and ΔAaPdel-19; and the complemented strain of ΔAaPdel, AaPdel-CP. All strains were inoculated on potato dextrose agar (PDA), minimal medium (MM), complete medium (CM), and V8 medium for 5 days at 28°C. (B) Statistical analyses of colony diameter. Colony diameter was presented as the mean ± SD of at least three independent experiments. Different letters on a graph denoted statistical significance at P = 0.05. (C) Hyphal morphology of wild type (Z7), AaPdel deletion mutants (ΔAaPdel-1 and ΔAaPdel-19) and complemented (AaPdel-CP) strains.
AaPdel Contributes to Conidia Formation and Morphology
To analyze potential gene functions in conidiation, the conidial suspension of wild type, deletion mutants, and supplementary strains were counted on a hemocytometer and photographed under a microscope at 40 times magnification. On the V8 medium, the ΔAaPdel formed colony with 10% reduced-growth-rate and produced approximately 65% reduced conidiation compared to the wild type (Figure 3A). The sporulation of the wild type Z7 was about 12.38 × 105, while the sporulation of the AaPdel mutant was about 4 × 105 (Figure 3C). Over 200 spore diameters of each strain were measured, and the spore morphology was observed under a microscope. The conidia of ΔAaPdel had varied morphologies, forming lowly pigmented aberrant structures. The conidia of ΔAaPdel were light gray, while the wild type and the complementary strain produced typical dark brown conidia (Figure 3B). However, through statistical analysis, AaPdel was not involved in conidia diameter and germination rate. There was no significant difference between ΔAaPdeh and wild type in terms of conidia production, germination rate, and morphology (Supplementary Figure 2). In summary, the AaPdel gene is involved in regulating the conidia production and pigment synthesis of conidia in A. alternata.
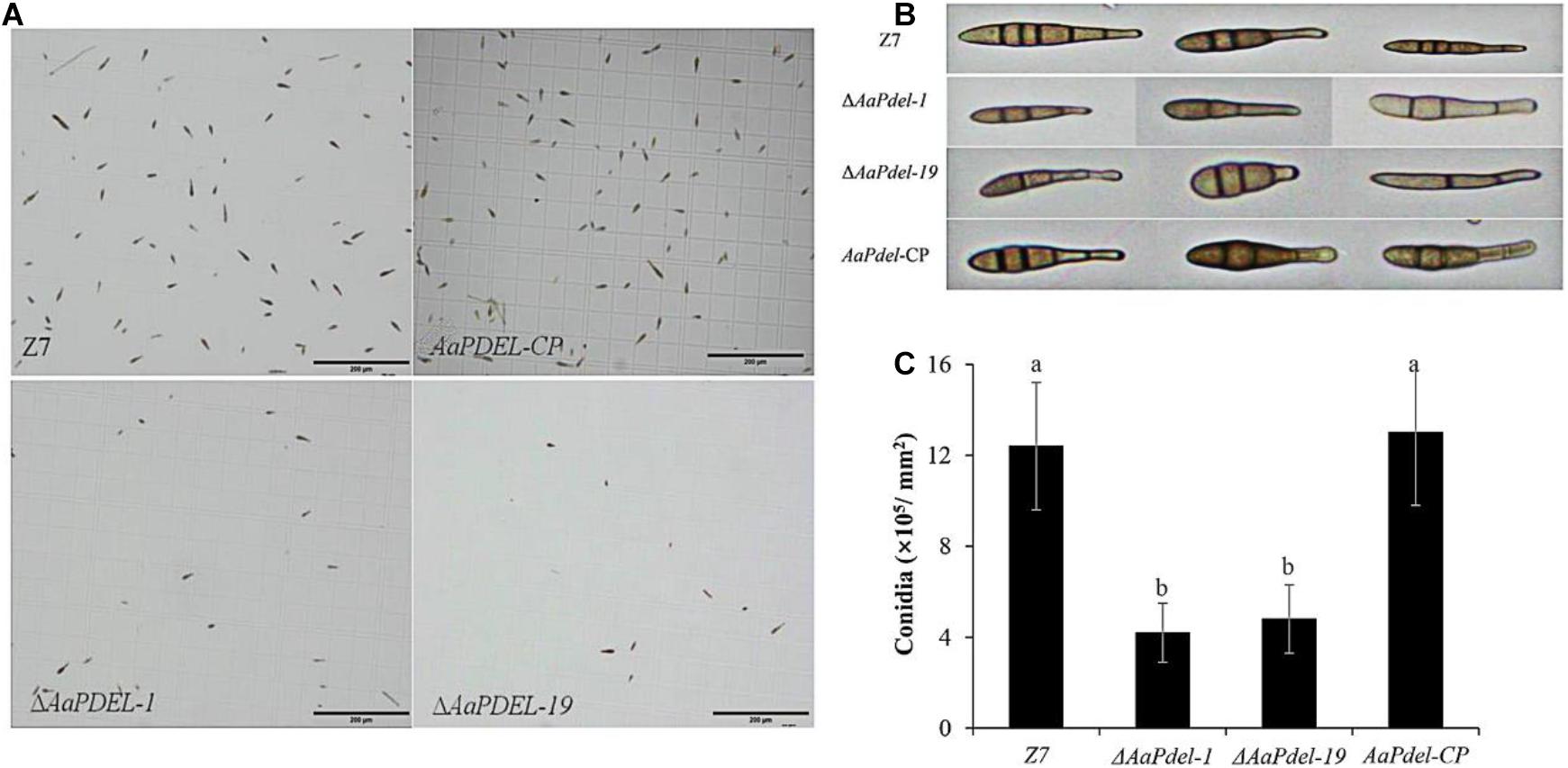
Figure 3. The ΔAaPdel of Alternaria alternata exhibited a significant reduction in conidia formation. (A) Light microscopy images of conidial suspension produced on V8 medium. (B) Conidia morphology of A. alternata strains examined by light microscopy. ΔAaPdel formed lowly pigmented aberrant structures. (C) Statistical analyses of conidia production. Statistical significance was analyzed by one-way ANOVA and Duncan’s new multiple range test (MRT). Different letters represent significant differences at P < 0.05.
AaPdel Plays a Role in Intracellular cAMP Level Regulation
Two cAMP phosphodiesterase-encoding genes were analyzed in the regulation of the intracellular cAMP level. Compared with the wild type, ΔAaPdel led to decreased accumulation of cAMP level, while the loss of AaPdeh led to the similar accumulation of cAMP level with the wild type. The trend was further confirmed by the complementation assay (Figure 4). The intracellular cAMP level of AaPdel-CP and AaPdeh-CP was consistent with the wild-type strain. AaPdel plays a role in intracellular cAMP level regulation.
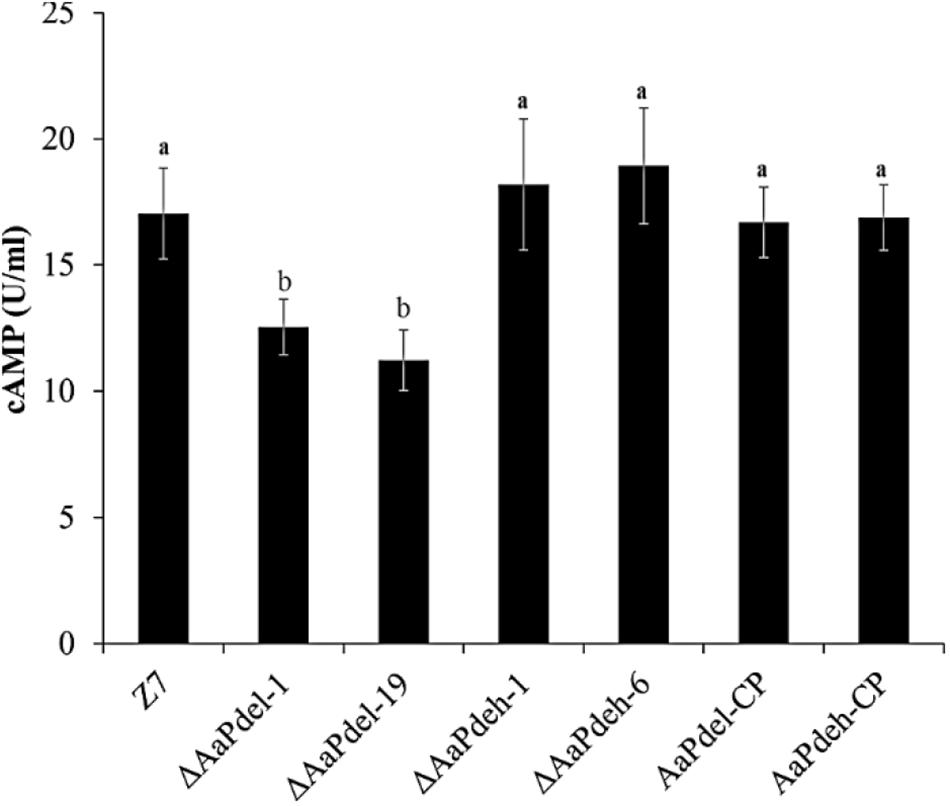
Figure 4. Intracellular cAMP quantification of ΔAaPdel and ΔAaPdeh during the hyphal stage in Alternaria alternata. Bar graphs depicted the levels of intracellular cAMP in the mycelia of ΔAaPdeh, ΔAaPdel, wild type, and complemented strain, which were cultured in liquid PDB for 48 h. Bars represented standard error. Different letters mark significant differences at P < 0.05.
AaPdel Is Involved in Response to Osmotic Stress and Oxidative Stress
To analyze the defects in response to the osmotic stress on the mutants, the activated strains were inoculated on PDA medium amended with 1 M sorbitol, 1 M NaCl, 1M KCl, or 1 M sucrose. Compared with the growth of the PDA medium with no stress factor present, the growth of all tested strains was restrained to the osmotic stress. The ΔAaPdel-1 and ΔAaPdel-19 mutants were hypersensitive to the osmotic stress inducers. The ΔAaPdel exhibited greater sensitivity to the non-ionic osmotic stress (sorbitol and sucrose) than the ionic osmotic stress (NaCl and KCl) (Figure 5A). Among these, the growth rate of ΔAaPdel-1 in sorbitol or sucrose were decreased by 10.58 and 10.11%, respectively, compared with the wild-type strain. Whereas with the ionic osmotic stress, the inhibitory effect of ΔAaPdel, ΔAaPdeh was similar compared with the wild type (Table 1). Online analysis of protein subcellular localization revealed that AaPdel protein was mainly located on the plasma membrane. According to the data, this different phenotype might be caused by the same concentrate Na+ and K+ treatment (Figure 5B).
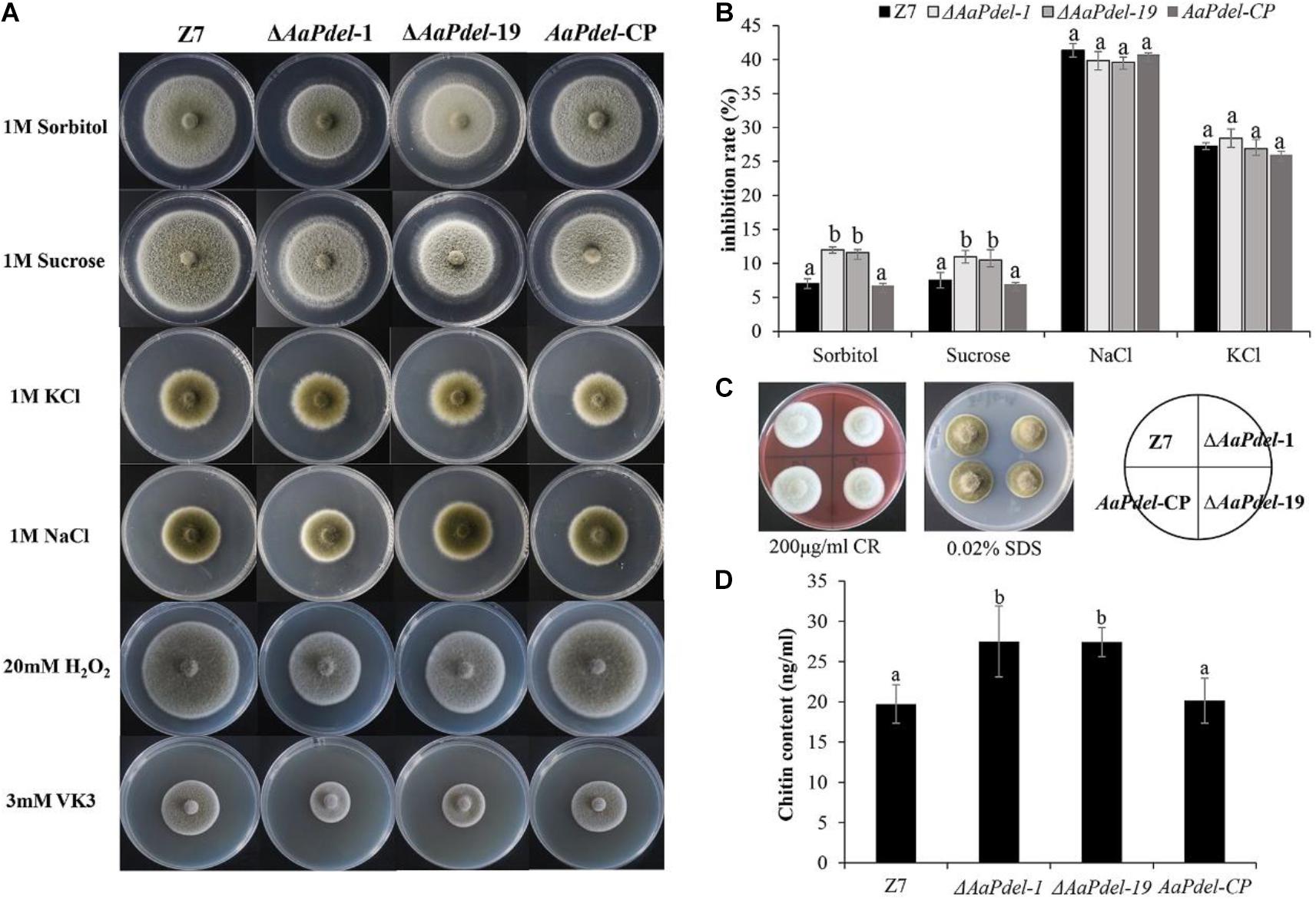
Figure 5. Effect of osmotic stress, oxidative stress and cell wall stress. (A) The phenotype of the wild-type strain (Z7); the deletion mutants (ΔAaPdel-1 andΔAaPdel-19); and the complemented strain of ΔAaPdel (AaPdel-CP) inoculated on PDA medium amended with osmotic stress and oxidative stress. (B) Statistical analyses of inhibition rate on the osmotic stress. Results were presented as the mean ± SD of at least three independent experiments. Statistical significance was analyzed by one-way ANOVA and Duncan’s new multiple range test (MRT). Different letters mark significant differences at P < 0.05. (C) The phenotype of the strains inoculated on PDA medium with cell wall stress factors of sodium dodecyl sulfate (SDS) and Congo red (CR). (D) Quantitative analysis of cell wall chitin levels in wild-type strain, AaPdel deletion mutants, and complemented strains. Bars represented standard error. Different letters represent statistically significant differences (Duncan’s new multiple range test, p < 0.05).

Table 1. Inhibition rate of osmotic, oxidative stress and cell wall integrity assay in the wild type Z7, ΔAaPdel, AaPdel-CP in Alternaria alternata.
In order to analyse the defects in response to the oxidative stress, the activated strains were inoculated on PDA medium added with oxidative stress factors (20 mM H2O2 or 3 mM VK3) after 5 days cultured at 28°C. The ΔAaPdel was hypersensitive to the oxidative stress inducers (Figure 5A). ΔAaPdel-1 had 23.24 and 66.67% inhibition rate, respectively, on PDA supplemented with H2O2 or VK3. The details of inhibition rate were presented in Table 1. However, under osmotic and oxidative stresses, the inhibition rates of ΔAaPdeh-1 and ΔAaPdeh-6 were similar to the wild type (Supplementary Figure 3).
AaPdel Played a Role in the Maintenance of Cell Wall Integrity
Cell wall stress tolerance assays were performed by inoculating WT and mutant strains onto PDA medium supplemented with either 0.02% SDS or 100 μg/ml of CR. By serving PDA as control, the growth of strains was restrained to both SDS and CR. And the AaPdel mutants were hypersensitive to the cell wall stress reagents (Figure 5C). Compared with the wild type, the inhibition rate of the AaPdel mutants was significantly increased in 0.02% SDS (Table 1). Chitin, the major component of the cell wall in A. alternata, was further quantified in deletion mutants and the wild-type strain. The chitin content of ΔAaPdel was higher than that of the wild type and complemented strain, indicating AaPdel was involved in cell wall integrity and architecture (Figure 5D). However, the ΔAaPdeh-1 and ΔAaPdeh-6 were not more sensitive to the cell wall stress reagents. These stresses disturb cell wall biosynthesis in A. alternata, activating the CWI pathway. The CWI of the AaPdel mutant was affected, indicating that AaPdel is involved in the maintenance of CWI.
AaPdel Is Required for Pathogenicity
The pathogenicity was assessed by inoculating the leaves of tangerine with conidial suspension at a concentration of 1.0×105 conidia ml–1. At 3 dpi, there were obvious lesions on the part of the leaves infected by wild type and complementary strains, but only mild lesions were observed on the leaves inoculated with ΔAaPdel-1 and ΔAaPdel-19. At 5 dpi, ΔAaPdel-1 and ΔAaPdel-19 conidial suspensions incited small necrotic lesions in the leave (Figure 6). According to Perina et al. (2019), the average scale levels quantified the disease severity of deletion mutants and Z7 were 4 and 6, respectively. It showed nearly 75% reduced lesion area compared with WT. And Pdel-CP strain complemented the attenuated pathogenicity of ΔAaPdel. In contrast, the necrotic lesions induced by ΔAaPdeh-1 and ΔAaPdeh-6 were as serious as the wild type in pathogenicity (Supplementary Figure 4). Taken together, AaPdel, coding low-affinity cAMP phosphodiesterase, was involved in regulating the pathogenicity of A. alternata.
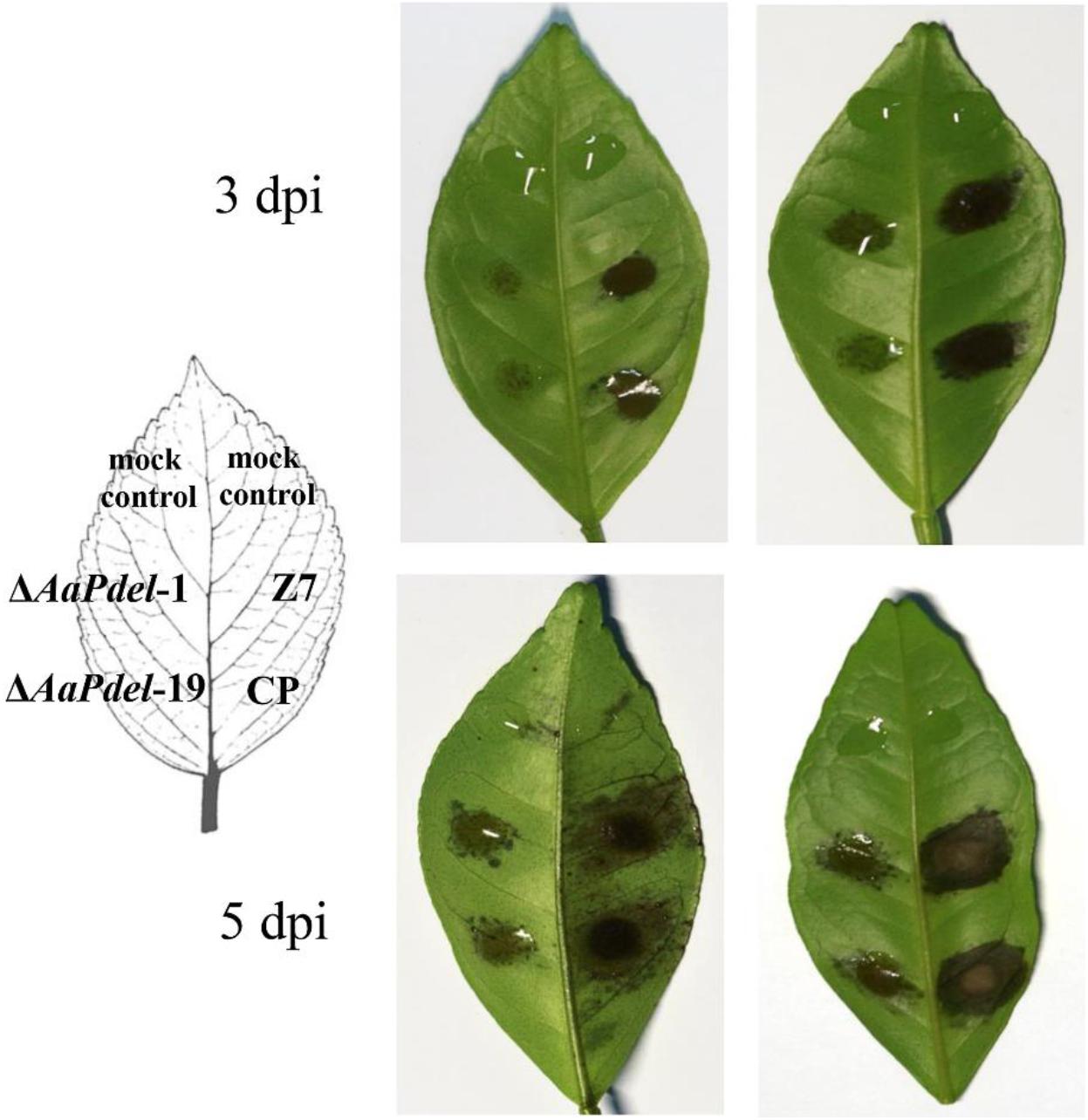
Figure 6. Mutation of AaPdel affected Alternaria alternata pathogenicity. Necrotic lesions of A. alternata on detached tangerine leaves induced by 20 μL conidial suspension (105 conidia per mL) of wild type Z7, ΔAaPdel mutants, and the complemented strain, respectively. The mock controls were treated with distilled water only. Necrotic lesions were observed and photographed 3 and 5 dpi, respectively.
AaPdel and AaPdeh Regulate Laccase Activity
Laccase is a copper-containing oxidase mainly involved in the catalysis of lignin degradation, pigment synthesis, and fungal pathogenicity. To determine the involvement of PDEs in laccase activity, we used a specific substrate, 2,2′-azino-di-3-ethylbenzthiazoline-6-sulfonate (ABTS). The oxidized dark purple stain can be observed at the colonies of both the ΔAaPdel,ΔAaPdeh mutants and the wild-type strain (Figure 7A). To further demonstrate the laccase activity difference between AaPdel and AaPdeh mutants, the crude enzyme solution was extracted and analyzed by spectrophotometer at 420 nm for 5 min. The statistical analyses showed that the laccase activity of both ΔAaPdel and ΔAaPdeh mutants were significantly lower than that of the wild type, and ΔAaPdel had a stronger inhibitory effect on laccase (Figure 7B). The above results indicate that both AaPdel and AaPdeh are involved in regulating laccase activity, and AaPdel plays a primary role in this pathway.
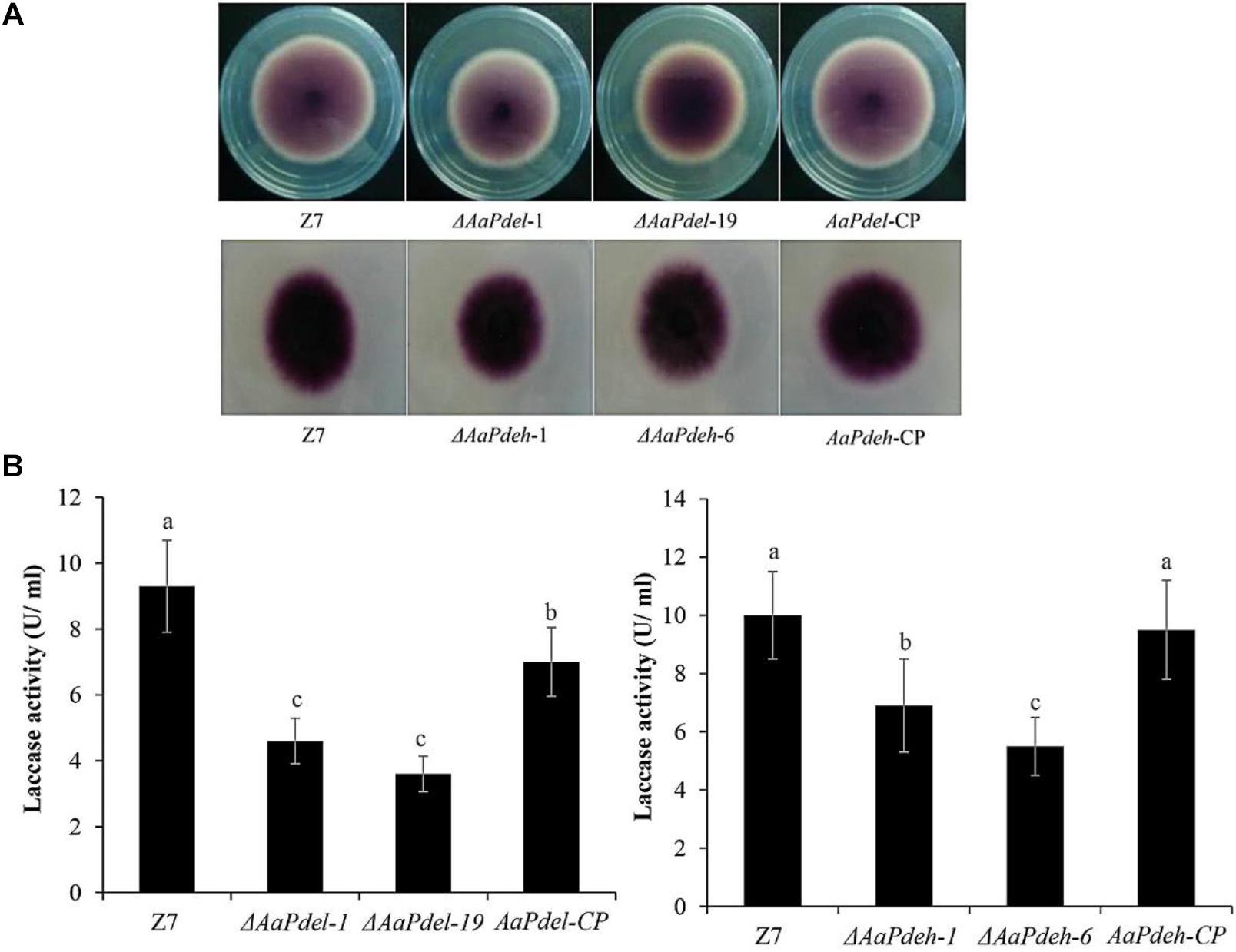
Figure 7. The laccases activity assays of ΔAaPdel and ΔAaPdeh of Alternaria alternata. (A) Laccase activity was tested on PDA medium with 0.03% ABTS culture at 28°C. Discoloration was observed and photographed 6 dpi. (B) Statistical analyses of laccase activity, which was determined by the ABTS oxidizing test. Statistical significance was analyzed by one-way ANOVA and Duncan’s new multiple range test (MRT). Different letters mark significant differences at P < 0.05.
Discussion
In fungi and yeast, cAMP signaling cascade regulate a large amount of cellular and biological processes. Since the discovery of cAMP-PKA pathway, PDEs have been characterized as important phosphodiesterase for cAMP level regulation in plant pathogens, including the budding yeast (S. cerevisiae) and filamentous fungi (F. graminearum, M. oryzae, C. albicans, and so on). Two PDEs, low-affinity phosphodiesterase Pdel (Pde1) and high-affinity phosphodiesterase Pdeh (Pde2), are involved in cAMP level regulation (Bahn et al., 2003). Pdeh is widespread in fungi and mammals, while Pdel is only found in fungi. In this study, the biological functions of the AaPdel and AaPdeh in A. alternata were preliminarily explored through gene replacement strategy, and it was found that AaPdel but not AaPdeh played a dominant role in biological processes of A. alternata, which differed from previously reported findings. Our data further support the PKA-mediated cAMP signaling pathway by showing that the regulator is involved in intracellular cAMP level.
In filamentous fungi, identified PDEL gene functions are mainly concentrated on conidiation, as in F. graminearum and Setosphaeria turcica. Our result was similar to those described above. The conidia production of the AaPdel deletion mutant was 65% lower than that of the wild type and complemented strain of ΔAaPdel. Also, the color of the ΔAaPdel conidia was relatively lighter. In M. oryzae and Aspergillus flavus, the PdeH deletion mutant also exhibited defects in vegetative growth and conidiation (Yang et al., 2017). In S. turcica, the deletion of the StH-PDE gene resulted in a loss of conidiation ability. Whereas the absence of AaPdeh did not result in the defects of conidia production, diameter, germination rate, and vegetative growth. The ΔAaPdel had growth defects on four vegetative growth media, and formed colonies with short, dense aerial hyphae. The growth defects of ΔAaPdel were extremely severe on the CM medium with sufficient nutrient elements. Comparing the utilization of basic nutrient elements, AaPdel might be involved in the utilization of certain nutrient elements. The AaPdel gene plays an important role in regulating conidiation and hypha growth.
The same concentration of osmotic stressors (sorbitol, sucrose, NaCl, and KCl) were used to analyze defects in stress response (Hanin et al., 2016). ΔAaPdel was more sensitive to non-ionic osmotic stress (sorbitol and sucrose) than ionic osmotic stress (NaCl and KCl). Among the analyzed osmotic stressors, sorbitol (a sugar alcohol), had the most significant inhibitory effect on the AaPdel deletion mutant. Whereas in the ionic osmotic stress, the inhibitory effect was the same when comparing the ΔAaPdel, ΔAaPdeh, and wild-type strain. According to the data, this phenotypic difference might be caused by the same concentration Na+ and K+ treatment. Analyses of protein subcellular localization revealed that the AaPdel protein mainly located on the plasma membrane. Hence, it can be hypothesized that AaPdel is involved in sodium-potassium pump regulation on the plasma membrane.
As the first barrier, the fungal cell wall and plasma membrane were of great significance to maintain the structural integrity and against external stresses. The fungal cell wall and plasma membrane are indispensable for maintaining the shape of the cell, and are also involved in signal transmission. In this study, ΔAaPdel was more sensitive to cell wall inhibitors CR and SDS, while ΔAaPdeh growth was similar to the wild type. SDS acts as a cell wall inhibitor by reducing the stability of the membrane to reveal the defect of the cell wall. CR inhibits binding to major components of the fungal cell wall, chitin and β-1,4-glucan (Gong et al., 2020). On the one hand, AaPdel appeared to be the sensor for signaling in response to the cell wall antagonist CR, which interferes with cell wall assembly by binding to chitin. Further analyses showed that the high chitin content of ΔAaPdel might account for the sensitivity to CR. However, the ΔAaPdeh-1 and ΔAaPdeh-6 were not more sensitive to cell wall stress reagents. Maintenance of cell wall integrity relies on the CWI pathway, a conserved cascade pathway, triggered by transmembrane sensors in response to extracellular stresses in fungus. These stresses disturb cell wall biosynthesis in A. alternata, activating the CWI pathway. The CWI of the AaPdel mutant was affected, indicating that AaPdel was involved in the maintenance of CWI.
Laccase is a copper-containing oxidase mainly involved in the catalysis of lignin degradation, pigment synthesis, and fungal pathogenicity (Mtibaa et al., 2018). The phenotype of lighter conidia color and attenuated pathogenicity led us to analyze the laccase activity. To determine the involvement of PDEs, the laccase activity of the ΔAaPdel, ΔAaPdeh, wild type, and complemented strain were tested and compared. We found that the wild type and complemented strain produced laccase, forming a dark purple hole on the ABTS medium, whereas the laccase activity of both ΔAaPdel and ΔAaPdeh was significantly lower than that of wild type and complemented strain. Consistent with our results, previous studies have demonstrated that the MoPdeh and MoPdel genes regulate intracellular laccase activity, and MoPdel plays a regulatory role in M. oryzae.
Research addressing gene functions in fungi has mainly focused on pathogens and those genes involved in pathogenicity or virulence. Among the studied PDEs, some genes encoding high-affinity phosphodiesterase affect pathogenicity. In S. turcica, the StH-PDE gene regulates secondary metabolism, melanin synthesis, and pathogenicity. The gene encoding high-affinity phosphodiesterase in T. serrata is involved in the regulation of mycelial growth and pathogenicity. MoPdeH (Pde2) has a dominant role in conidiation, pathogenicity, and intracellular cAMP level regulation, whereas MoPdel (Pde1) had no obvious function in M. oryzae. In this research, the finding was contrary to previous studies. In A. alternata, the ΔAaPdel mutant induced much smaller lesions than that of the wild type, whereas the ΔAaPdeh mutant induced lesions as drastic as those in the wild-type strain. Chitin is one of the fungal PAMPs, and the high content in ΔAaPdel may triggered rapid and strong plant parttern-triggered immunity. Above all, the attenuated pathogenicity of ΔAaPdel was probably caused by defects in the utilization of certain nutrient elements, osmotic stress response, plasma membrane, low laccase activity, high chitin content in the cell wall, and PKA-mediated cAMP signaling pathway.
PDEs target specific intracellular sites or signal transduction complexes and localize not only in the cytoplasm, but also in membrane, nucleus, and cytoskeleton locations (Houslay et al., 1998). Hu et al. (2010) reported that, like its low-affinity counterpart, the high-affinity phosphodiesterase may also play an important role in PKA negative feedback loop through the Pde2 localization assays. The localization of Pde2-GFP was affected by the carbon sources available and the cAMP-dependent PKA in the yeast S. cerevisiae. Pde2 is concentrated in the nucleus of cells grown on glucose, while it is distributed in the nucleus and cytoplasm of cells grown on some non-fermentable carbon sources. And PKA positively regulates the nuclear accumulation of Pde2. Elevated PKA activity increased the nuclear concentration of Pde2. Whereas in PKA attenuated strains, Pde2 is unable to concentrate in the nucleus of cells grown on glucose. Ramanujam and Naqvi (2010) reported the location of PdeH-GFP and PdeL-GFP during asexual and pathogenic development in M. oryzae. Although PdeH-GFP was cytoplasmic, it was dynamically associated with the plasma membrane and vesicle compartment, and the PdeL-GFP was predominantly localized in the nucleus. In Aspergillus flavus, PdeL-GFP shows a strong fluorescent signal in the nucleus of the hyphae, and PdeH-GFP is likely to be localized to punctate structures in the cytosol, rather than the nucleus. The localization of PDEs is complicated and dynamic. And limited to the resolution of the microscope, the reported location of the PDEs haven’t been directly localized to the organelle. In this study, we use online software to analyze the gene sequence. Online analysis of protein subcellular localization revealed that the AaPdel protein was predominantly located on the plasma membrane and the AaPdeh protein was located in the nucleus. From the perspective of protein subcellular localization, the AaPdeh protein was parallel to MoPdeL and AfPdeL, both in the nucleus. While AaPdel protein was similar to MoPdeH and AfPdeH. Further analysis of the signal peptides and transit peptides in AaPdel protein, revealed that the AaPdel protein was localized to the secretory pathway. We also investigate whether the two cAMP phosphodiesterase-encoding genes directly contribute to the intracellular cAMP level. Compared with the wild type, ΔAaPdel led to decreased accumulation of cAMP level, while the loss of AaPdeh led to the similar accumulation of cAMP level with the wild type. AaPdel regulated the intracellular cAMP level. This inverse trend of gene function in A. alternata was in accordance with the protein location difference between many fungi. We speculate that although PDEs homology has been identified in many filamentous fungi, their functions are not consistent, and there was no clear association between the conserved domain and physiological function. The inconsistent gene function may due to different protein location. It indicated that PKA-mediated cAMP signaling pathway was complicated in A. alternata, and needed further analysis.
In this study, the AaPdel and AaPdeh genes were identified to encode low-affinity PDEs (Pdel) and high-affinity PDEs (Pdeh) in A. alternata. We used a gene deletion and complementation strategy to characterize the gene function of AaPdel and AaPdeh. In A. alternata, AaPdel regulated intracellular cAMP levels during the hyphal stage. Deletion of AaPdel, but not AaPdeh, was found to play a role in conidiation and pathogenicity in A. alternata. ΔAaPdel showed a defect in conidiation, producing approximately 65% reduced conidiation and forming lowly pigmented aberrant structures. Upon exposure to osmotic stressors, ΔAaPdel had growth defects in response to osmotic stress, which was more sensitive to non-ionic osmotic stress than ionic osmotic stress. This different phenotype might be caused by the same concentrate Na+ and K+ treatment. Moreover, AaPdel deletion mutants had defects in vegetative growth, hyphal growth and pathogencity. Further analyses showed that the high chitin content of ΔAaPdel might account for the sensitivity to CR. Based on the attenuated pathogenicity and lowly pigmented aberrant structures, the laccase activity analyses found that both AaPdel and AaPdeh were involved in laccase activity regulation. Our data further support the PKA-mediated cAMP signaling pathway, as we have found the regulators of AaPdel is involved in intracellular cAMP levels in A. alternata. The inconsistent gene function may due to different protein location. In A. alternata, cAMP-PKA pathway played important roles in signal transduction, but the function of the genes involved in this pathway required further study.
Data Availability Statement
The original contributions presented in the study are included in the article/Supplementary Material, further inquiries can be directed to the corresponding author/s.
Author Contributions
KT, XK, and WL conceived and designed the experiments. CZ contributed to reagents, materials, and analysis tools. WL and XK performed the experiments. WL, KT, and CZ analyzed the data and wrote the manuscript. All authors read and approved the final manuscript.
Funding
This research was funded by the earmarked fund for China Agricultural Research System (No. CARS-27) and the Chongqing Program for Science and Technology Development (No. cstc2012gg-yyjsx0001).
Conflict of Interest
The authors declare that the research was conducted in the absence of any commercial or financial relationships that could be construed as a potential conflict of interest.
Supplementary Material
The Supplementary Material for this article can be found online at: https://www.frontiersin.org/articles/10.3389/fmicb.2020.597545/full#supplementary-material
Supplementary Figure 1 | PCR results of ΔAaPdel transformants were verified by three primer pairs of PLP1F/H855R, H856F/PLP2R, and PLF/PLR. AaPdeh transformants were identified through PHP1F/H855R, H856F/PHP2R, and PHF/PHR.
Supplementary Figure 2 | The conidia of AaPdeh deletion mutants exhibit similar phenotype with wild-type strain. (A) The conidia morphology examined by light microscopy. (B) Statistical analysis of conidia production. The quantity of conidia was quantified after cultured on the V8 medium in dark for 8 days. Bars indicate standard errors of at least three repetitions.
Supplementary Figure 3 | The sensitivity of the AaPdeh mutants to osmotic stress. Z7, ΔAaPdeh-1, ΔAaPdeh-6, and AaPdeh-CP strains were inoculated on PDA amended with osmotic stressors, NaCl, KCl, sorbitol or sucrose, respectively.
Supplementary Figure 4 | Similar necrotic lesions induced by ΔAaPdeh strain and wild type Z7 on detached tangerine leaves. The conidial suspension at 1.0 × 105 conidia/ml of Alternaria alternata wild type Z7, ΔAaPdeh and the complemented strains were inoculated on leaves, respectively. The purified water was used as mock control.
Footnotes
References
Bahn, Y. S., Staab, J., and Sundstrom, P. (2003). Increased high-affinity phosphodiesterase PDE2 gene expression in germ tubes counteracts CAP1-dependent synthesis of cyclic AMP, limits hypha production and promotes virulence of Candida albicans. Mol. Microbiol. 50, 391–409. doi: 10.1046/j.1365-2958.2003.03692.x
Calvo, A. M., Wilson, R. A., Bok, J. W., and Keller, N. P. (2002). Relationship between secondary metabolism and fungal development. Microbiol. Mol. Biol. Rev. 66, 447–459. doi: 10.1128/mmbr.66.3.447-459.2002
Caza, M., and Kronstad, J. W. (2019). The cAMP/protein kinase a pathway regulates virulence and adaptation to host conditions in Cryptococcus neoformans. Front. Cell. Infect. Microbiol. 9:212. doi: 10.3389/fcimb.2019.00212
Conti, M., and Beavo, J. (2007). Biochemistry and physiology of cyclic nucleotide phosphocliesterases: essential components in cyclic nucleotide signaling. Annu. Rev. Biochem. 76, 481–511. doi: 10.1146/annurev.biochem.76.060305.150444
Dong, Y. H., Li, Y., Zhao, M. M., Jing, M. F., Liu, X. Y., Liu, M. X., et al. (2015). Global genome and transcriptome analyses of Magnaporthe oryzae epidemic isolate 98-06 uncover novel effectors and pathogenicity-related genes, revealing gene gain and lose dynamics in genome evolution. PLoS Pathog. 11:e1004801. doi: 10.1371/journal.ppat.1004801
Gong, B.-Q., Wang, F.-Z., and Li, J.-F. (2020). Hide-and-seek: chitin-triggered plant immunity and fungal counterstrategies. Trends Plant Sci. 25, 805–816. doi: 10.1016/j.tplants.2020.03.006
Hanin, M., Ebel, C., Ngom, M., Laplaze, L., and Masmoudi, K. (2016). New insights on plant salt tolerance mechanisms and their potential use for breeding. Front. Plant Sci. 7:1787. doi: 10.3389/fpls.2016.01787
Harren, K., Brandhoff, B., Knodler, M., and Tudzynski, B. (2013). The high-Affinity phosphodiesterase BcPde2 has impact on growth, differentiation and virulence of the phytopathogenic ascomycete Botrytis cinerea. PLoS One 8:e0078525. doi: 10.1371/journal.pone.0078525
Hatta, R., Ito, K., Hosaki, Y., Tanaka, T., Tanaka, A., Yamamoto, M., et al. (2002). A conditionally dispensable chromosome controls host-specific pathogenicity in the fungal plant pathogen Alternaria alternata. Genetics 161, 59–70.
Houslay, M. D., Baillie, G. S., and Maurice, D. H. (2007). cAMP-specific phosphodiesterase-4 enzymes in the cardiovascular system - A molecular toolbox for generating compartmentalized cAMP signaling. Circ. Res. 100, 950–966. doi: 10.1161/01.res.0000261934.56938.38
Houslay, M. D., Sullivan, M., and Bolger, G. B. (1998). The multienzyme PDE4 cyclic adenosine monophosphate-specific phosphodiesterase family: intracellular targeting, regulation, and selective inhibition by compounds exerting antiinflammatory and antidepressant actions. Adv. Pharmacol. 44, 225–342. doi: 10.1016/s1054-3589(08)60128-3
Hu, Y., Liu, E. K., Bai, X. J., and Zhang, A. L. (2010). The localization and concentration of the PDE2-encoded high-affnity cAMP phosphodiesterase is regulated by cAMP-dependent protein kinase A in the yeast Saccharomyces cerevisiae. FEMS Yeast Res. 10, 177–187. doi: 10.1111/j.1567-1364.2009.00598.x
Irfan, M., Mehmood, S., Irshad, M., and Anwar, Z. (2018). Optimized production, purification and molecular characterization of fungal laccase through Alternaria alternata. Turk. J. Biochem. Turk. Biyokimya. Dergisi. 43, 613–622. doi: 10.1515/tjb-2017-0239
Ito, K., Tanaka, T., Hatta, R., Yamamoto, M., Akimitsu, K., and Tsuge, T. (2004). Dissection of the host range of the fungal plant pathogen Alternaria alternata by modification of secondary metabolism. Mol. Microbiol. 52, 399–411. doi: 10.1111/j.1365-2958.2004.04004.x
Jiang, C., Zhang, C. K., Wu, C. L., Sun, P. P., Hou, R., Liu, H. Q., et al. (2016). TRI6 and TRI10 play different roles in the regulation of deoxynivalenol (DON) production by cAMP signalling in Fusarium graminearum. Environ. Microbiol. 18, 3689–3701. doi: 10.1111/1462-2920.13279
Jin, S. L. C., Swinnen, J. V., and Conti, M. (1992). Characterization of the structure of a low Km, rolipram-sensitive cAMP phosphodiesterase-mapping of the catalytic domain. J. Biol. Chem. 267, 18929–18939.
Kong, X. W., Tang, F. Y., Lv, W. W., Zhang, Q., and Tang, K. Z. (2018). Optimization of protoplast preparation and regeneration conditions of Alternaria alternata. J. Plant Prot. 45, 1431e1432.
Lee, H. B., Patriarca, A., and Magan, N. (2015). Alternaria in food: ecophysiology, mycotoxin production and toxicology. Mycobiology 43, 93–106. doi: 10.5941/myco.2015.43.2.93
Liu, H., Suresh, A., Willard, F. S., Siderovski, D. P., Lu, S., and Naqvi, N. I. (2007). Rgs1 regulates multiple G alpha subunits in Magnaporthe pathogenesis, asexual growth and thigmotropism. EMBO J. 26, 690–700. doi: 10.1038/sj.emboj.7601536
Londesborough, J., and Suoranta, K. (1983). The zinc-containing high Km cyclic-nucleotide phosphodiesterase of bakers-yeast. J. Biol. Chem. 258, 2966–2972.
Ma, H. J., Zhang, B., Gai, Y. P., Sun, X. P., Chung, K. R., and Li, H. Y. (2019). Cell-wall-degrading enzymes required for virulence in the host selective toxin-producing necrotroph Alternaria alternata of citrus. Front. Microbiol. 10:2514. doi: 10.3389/fmicb.2019.02514
Ma, P. S., Wera, S., Van Dijck, P., and Thevelein, J. M. (1999). The PDE1-encoded low-affinity phosphodiesterase in the yeast Saccharomyces cerevisiae has a specific function in controlling agonist-induced cAMP signaling. Mol. Biol. Cell 10, 91–104. doi: 10.1091/mbc.10.1.91
Mtibaa, R., Barriuso, J., de Eugenio, L., Aranda, E., Belbahri, L., Nasri, M., et al. (2018). Purification and characterization of a fungal laccase from the ascomycete Thielavia sp. and its role in the decolorization of a recalcitrant dye. Int. J. Biol. Macromol. 120(Pt B), 1744–1751. doi: 10.1016/j.ijbiomac.2018.09.175
Otani, H., Kohmoto, K., and Kodama, M. (1995). Alternaria toxins and their effects on host plants. Can. J. Bot. Rev. Can. Bot. 73, S453–S458. doi: 10.1139/b95-282
Perina, F. J., Belan, L. L., Moreira, S. I., Nery, E. M., Alves, E., and Pozza, E. A. (2019). Diagrammatic scale for assessment of alternaria brown spot severity on tangerine leaves. J. Plant Pathol. 101, 981–990. doi: 10.1007/s42161-019-00306-6
Ramanujam, R., and Naqvi, N. I. (2010). PdeH, a high-affinity cAMP phosphodiesterase, is a key regulator of asexual and pathogenic differentiation in Magnaporthe oryzae. PLoS Pathog. 6:e1000897. doi: 10.1371/journal.ppat.1000897
Rehman, L., Su, X. F., Guo, H. M., Qi, X. L., and Cheng, H. M. (2016). Protoplast transformation as a potential platform for exploring gene function in Verticillium dahliae. BMC Biotechnol. 16:9. doi: 10.1186/s12896-016-0287-4
Solel, Z., and Kimchi, M. (1998). Histopathology of infection of Minneola tangelo by Alternaria alternata pv. citri and the effect of host and environmental factors on lesion development. J. Phytopathol. 146, 557–561. doi: 10.1111/j.1439-0434.1998.tb04754.x
Tang, K., Lv, W., Zhang, Q., and Zhou, C. (2020). Coding the alpha-subunit of SNF1 kinase, Snf1 is required for the conidiogenesis and pathogenicity of the Alternaria alternata tangerine pathotype. Fungal Biol. 124, 562–570. doi: 10.1016/j.funbio.2020.02.008
Thomma, B. (2003). Alternaria spp.: from general saprophyte to specific parasite. Mol. Plant Pathol. 4, 225–236. doi: 10.1046/j.1364-3703.2003.00173.x
Timmer, L. W., Solel, Z., Gottwald, T. R., Ibanez, A. M., and Zitko, S. E. (1998). Environmental factors affecting production, release, and field populations of conidia of Alternaria alternata, the cause of brown spot of citrus. Phytopathology 88, 1218–1223. doi: 10.1094/phyto.1998.88.11.1218
Tsuge, T., Harimoto, Y., Akimitsu, K., Ohtani, K., Kodama, M., Akagi, Y., et al. (2013). Host-selective toxins produced by the plant pathogenic fungus Alternaria alternata. FEMS Microbiol. Rev. 37, 44–66. doi: 10.1111/j.1574-6976.2012.00350.x
Uno, I., Matsumoto, K., and Ishikawa, T. (1983). Characterization of a cyclic-nucleotide phosphodiesterase-deficient mutant in yeast. J. Biol. Chem. 258, 3539–3542.
Wang, M., Sun, X., Yu, D., Xu, J., Chung, K., and Li, H. (2016). Genomic and transcriptomic analyses of the tangerine pathotype of Alternaria alternata in response to oxidative stress. Sci. Rep. 6:32437. doi: 10.1038/srep32437
Wang, M., Yang, X., Ruan, R., Fu, H., and Li, H. (2018). Csn5 is required for the conidiogenesis and pathogenesis of the Alternaria alternata tangerine pathotype. Front. Microbiol. 9:508. doi: 10.3389/fmicb.2018.00508
Wera, S., Ma, P. S., and Thevelein, J. M. (1997). Glucose exerts opposite effects on mRNA versus protein and activity levels of Pde1, the low-affinity cAMP phosphodiesterase from budding yeast, Saccharomyces cerevisiae. FEBS Lett. 420, 147–150. doi: 10.1016/s0014-5793(97)01508-1
Wilson, D., Fiori, A., Brucker, K. D., Dijck, P. V., and Stateva, L. (2010). Candida albicans Pde1p and Gpa2p comprise a regulatory module mediating agonist-induced cAMP signalling and environmental adaptation. Fungal Genet. Biol. 47, 742–752. doi: 10.1016/j.fgb.2010.06.006
Yang, K., Liu, Y., Liang, L., Li, Z., Qin, Q., Nie, X., et al. (2017). The high-affinity phosphodiesterase PdeH regulates development and aflatoxin biosynthesis in Aspergillus flavus. Fungal Genet. Biol. 101, 7–19. doi: 10.1016/j.fgb.2017.02.004
Zhang, H., Liu, K., Zhang, X., Tang, W., Wang, J., Guo, M., et al. (2011). Two phosphodiesterase genes, PDEL and PDEH, regulate development and pathogenicity by modulating intracellular cyclic AMP levels in Magnaporthe oryzae. PLoS One 6:e17241. doi: 10.1371/journal.pone.0017241
Zhao, X., Mehrabi, R., and Xu, J.-R. (2007). Mitogen-activated protein kinase pathways and fungal pathogenesis. Eukaryot. Cell 6, 1701–1714. doi: 10.1128/ec.00216-07
Keywords: Alternaria alternata tangerine pathotype, cAMP phosphodiesterase, conidiation, cell wall integrity, osmotic stress, pathogenicity
Citation: Lv W, Kong X, Zhou C and Tang K (2020) Pdel, Encoding a Low-Affinity cAMP Phosphodiesterase, Regulates Conidiation and Pathogenesis in Alternaria alternata Tangerine Pathotype. Front. Microbiol. 11:597545. doi: 10.3389/fmicb.2020.597545
Received: 21 August 2020; Accepted: 16 November 2020;
Published: 07 December 2020.
Edited by:
Jia Liu, Chongqing University of Arts and Sciences, ChinaReviewed by:
Bo Zhu, Shanghai Jiao Tong University, ChinaGiorgio Maresi, Fondazione Edmund Mach, Italy
Copyright © 2020 Lv, Kong, Zhou and Tang. This is an open-access article distributed under the terms of the Creative Commons Attribution License (CC BY). The use, distribution or reproduction in other forums is permitted, provided the original author(s) and the copyright owner(s) are credited and that the original publication in this journal is cited, in accordance with accepted academic practice. No use, distribution or reproduction is permitted which does not comply with these terms.
*Correspondence: Kezhi Tang, dGFuZ2tlemhpQGNyaWMuY24=