- 1Departamento de Genética, Microbiología y Estadística, Sección Microbiología, Virología y Biotecnología, Facultad de Biología, Universidad de Barcelona, Barcelona, Spain
- 2National Research Council Canada, Human Health Therapeutics Research Centre, Ottawa, ON, Canada
- 3Faculty of Science, Carleton University, Ottawa, ON, Canada
Polar flagella from mesophilic Aeromonas strains have previously been shown to be modified with a range of glycans. Mass spectrometry studies of purified polar flagellins suggested the glycan typically includes a putative pseudaminic acid like derivative; while some strains are modified with this single monosaccharide, others modified with a heterologous glycan. In the current study, we demonstrate that genes involved in polar flagella glycosylation are clustered in highly polymorphic genomic islands flanked by pseudaminic acid biosynthetic genes (pse). Bioinformatic analysis of mesophilic Aeromonas genomes identified three types of polar flagella glycosylation islands (FGIs), denoted Group I, II and III. FGI Groups I and III are small genomic islands present in Aeromonas strains with flagellins modified with a single monosaccharide pseudaminic acid derivative. Group II were large genomic islands, present in strains found to modify polar flagellins with heterogeneous glycan moieties. Group II, in addition to pse genes, contained numerous glycosyltransferases and other biosynthetic enzymes. All Group II strains shared a common glycosyltransferase downstream of luxC that we named flagella glycosylation island 1, fgi-1, in A. piscicola AH-3. We demonstrate that Fgi-1 transfers the first sugar of the heterogeneous glycan to the pseudaminic acid derivative linked to polar flagellins and could be used as marker for polysaccharidic glycosylation of Aeromonas polar flagella.
Introduction
Protein glycosylation is a common post-translational modification in bacteria and has been described in both gram-negative and gram-positive bacteria (De Maayer and Cowan, 2016; Schäffer and Messner, 2017). This post-translational modification has been identified in many target proteins, including surface proteins such as pili, flagella, adhesins and surface layer proteins (Benz and Schmidt, 2002; Abu-Qarn et al., 2008). Glycoproteins are reported to play roles in adhesion, proteins stabilization, motility and evasion of immune responses, however in many cases, the precise function of the glycan modification has not been determined (Szymanski and Wren, 2005; Tan et al., 2015). Bacterial glycans show high variability in structures and composition (Benz and Schmidt, 2002), and are linked to the amide group of asparagine residues (N-glycosylation) or to the hydroxyl group of serine (Ser) or threonine (Thr) residues (O-glycosylation) (Nothaft and Szymanski, 2010).
Aeromonads are rod-shaped, Gram-negative bacteria, ubiquitous in the environment, and frequently associated with fresh or estuarine water. They are emerging as the causative agents of gastrointestinal and extraintestinal disease in a vast evolutionary range of animals (Janda and Abbott, 2010). Although the most common complications from these pathogens are easily tractable, the number of reported infections caused by these microorganisms in humans has been steadily rising in recent years (Igbinosa et al., 2012). Aeromonas infections present a serious threat to the increasing population of immunocompromised patients, causing severe septicaemia, and death (Parker and Shaw, 2011). The pathogenicity of Aeromonads is multifactorial, and depends on specific strain characteristics, but common pathogenic factors across Aeromonadaceae are toxins and secretion systems, outer-membrane proteins, capsules, polysaccharides, cell-wall proteins and flagella (Tomás, 2012).
Mesophilic Aeromonas have a single polar flagellum which is produced constitutively. In addition, 50–60% of clinical isolates also express a lateral inducible flagella (Gavín et al., 2002). Polar flagellins, the structural protein of the flagellar filament, of strains analyzed to date are reported to be O-glycosylated at 5–8 Ser or Thr residues of the central immunogenic D2/D3 domains (Tabei et al., 2009; Wilhelms et al., 2012; Fulton et al., 2015). However, the observed glycans show diversity in their carbohydrate composition and chain length between strains. In Aeromonas caviae Sch3N and Aeromonas hydrophila AH-1, the glycan modifying polar flagellin was observed to be a single monosaccharide, which was speculated to be a pseudaminic acid derivative (Tabei et al., 2009; Fulton et al., 2015); in Aeromonas piscicola AH-3, the glycan modifying polar flagellin was reported to be a heptasaccharide comprised of one putative pseudaminic acid derivative, three N-acetylhexosamines (HexNAc), two hexoses (Hex) and one unknown glycan of 102 Da (Wilhelms et al., 2012). In all strains, a putative pseudaminic acid derivative was found to be the linking sugar that directly modifies the Ser or Thr residue. Regardless of glycan composition, the post-translational modification has been shown to be required for the correct polar flagellum assembly in all Aeromonadaceae with characterized glycosylation to date (Parker et al., 2012; Merino and Tomás, 2014; Fulton et al., 2015) and is essential for adhesion, biofilm formation and colonization (Merino et al., 1999; Rabaan et al., 2001; Gavín et al., 2002).
In many bacteria, the sugar biosynthetic pathways and associated glycosyltransferases required for flagellin modification are encoded in close proximity to the genes encoding the flagellar apparatus. In Aeromonas, the pseudaminic acid biosynthesis locus, formed by PseB, PseC, PseF, PseG, and PseI (also known as the flm locus, or flmA, flmB, neuA-like, flmD, and neuB-like, respectively), is reported to exist in two different chromosomal locations (Canals et al., 2007; Tabei et al., 2009). In A. caviae Sch3N these genes are located adjacent to the O-antigen lipopolysaccharide (LPS) biosynthesis locus and its mutation abolishes both O-antigen LPS and polar flagellum formation (Tabei et al., 2009). However, in A. hydrophila AH-1 and A. piscicola AH-3 many of these genes are adjacent to the polar flagella region 2, which contains the polar flagellin genes. Mutations in this region have been reported only to affect the flagella formation (Canals et al., 2007; Fulton et al., 2015). Furthermore, in A. caviae Sch3N the glycosyltransferase maf-1, located adjacent to the polar flagella region 2, was identified as the prime candidate to transfer the pseudaminic acid derivative to the flagellin monomers prior to their deliver to the flagella export apparatus (Parker et al., 2012, 2014). Homologous and identically localized glycosyltransferases were found in A. hydrophila AH-1 (AO056-RS01190) and A. piscicola AH-3 (maf-1), which are also adjacent to the pseudaminic acid biosynthetic genes (Canals et al., 2006; Forn-Cuní et al., 2016a,b) (Figure 1).
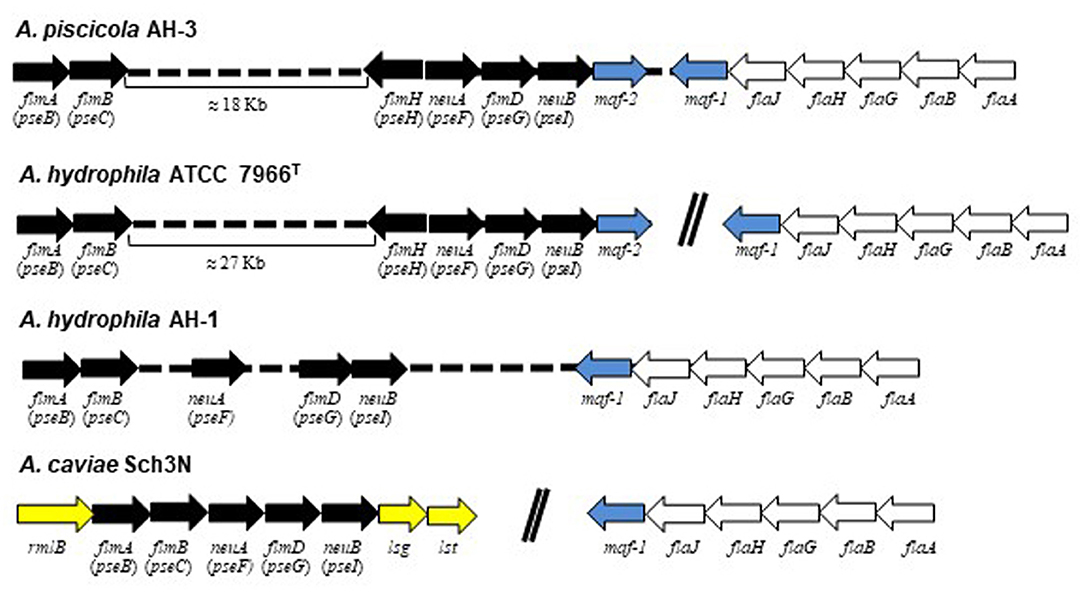
Figure 1. Comparative schematic representation of A. piscicola AH-3, A. hydrophila ATCC7966TM, A. hydrophila AH-1 and A. caviae Sch3N pseudaminic acid biosynthetic genes flmA (pseB), flmB (pseC), flmH (pseH), neuA (pseF), flmD (pseG), and neuB (pseI), also known as the flm locus, in black; maf glycosiltransferases in blue; polar flagella genes in white; and O-antigen LPS genes in yellow. Arrows of the same color indicates homologous genes among these bacteria.
In this study, we analyze and classify the Aeromonadaceae flagella glycosylation islands (fgi), across all the publicly available mesophilic Aeromonas genomes and characterize for the first time a common but unstudied glycosyltransferase, Fgi-1, in strains with heterogeneous glycans, as marker for polysaccharidic glycosylation of Aeromonas polar flagellum.
Materials and Methods
Identification and Characterization of Polar Flagellin Glycosylation Islands (fgi)
The genome sequences of 265 mesophilic Aeromonads strains were retrieved from the NCBI database regardless of their assembly completeness. To locate the pseudaminic acid biosynthesis cluster, we performed a local tblastn of the A. piscicola AH-3 PseI and PseC in each genome. We retained 50 complete genomes and a further 160 genomes in which both genes were present in the same contig or scaffold, independently of the space between the two genes. Genes on the selected region were predicted with Glimmer v3.0.2 (Delcher et al., 2007), and explored using Sybil (Riley et al., 2012) as implemented in the CloVR Comparative Pipeline (Angiuoli et al., 2011). GC percentage was calculated based on the sequences of the species A. piscicola AH-3, A. hydrophila ATCC7966T and AH-1. All the following bioinformatic analysis with at least one of each three clusters, when not mentioned, were based on AH-3. Alignment of the 97 Fgi-1 protein sequences was performed by ClustalW and the percentage of identity between them was calculated using the Clustal Omega service (Sievers et al., 2011) of the EMBL-EBI website (Li et al., 2015). Protein domains were determined with the NCBI Conserved Domain Database (CDD) (Marchler-Bauer et al., 2014), and the SMART sequence analysis (Letunic et al., 2014). The presence of signal peptides was explored with the SignalP 4.1 Server (Nielsen, 2017) and of transmembrane helices using the TMHMM Server v2.0 (Krogh et al., 2001). The tertiary structure of Fgi-1 was modeled with the Intensive method from the Phyre2 Protein Fold Recognition Server (Kelley et al., 2015) and the I-Tasser Suite (Yang et al., 2014). Finally, the Ligand Binding Sites of the Phyre2 model were predicted with 3DLigandSite (Wass et al., 2010).
Bacterial Strains, Plasmids and Growth Conditions
Bacterial strains and plasmids used in this study are listed in Table 1. E. coli strains were grown on Luria-Bertani (LB) Miller broth and LB Miller agar at 37°C. Aeromonas strains were grown either in tryptic soy broth (TSB) or agar (TSA) at 25°C. When required, chloramphenicol (25 μg/ml), rifampicin (100 μg/ml) and spectinomycin (50 μg/ml) were added to media. Media were supplemented with 0.2% (w/v) L-arabinose to induce recombinant protein expression under the arabinose promoter on pBAD33.
General DNA Techniques and Nucleotide Sequencing
DNA manipulations were carried out according to standard procedures (Sambrook et al., 1989). DNA restriction endonucleases were obtained from Promega. T4 DNA ligase and alkaline phosphatase were obtained from Invitrogen and GE Healthcare, respectively. Polymerase chain reaction (PCR) was performed using the BioTaq DNA polymerase (Ecogen) in a Gene Amplifier PCR System 2400 Perkin Elmer Thermal Cycler. Plasmid DNA for sequencing was isolated by Qiagen plasmid purification kit (Qiagen, Inc. Ltd.) as recommended by the suppliers. Double-strand DNA sequencing was performed by using the Sanger dideoxy-chain termination method (Sanger et al., 1977) with the BigDye Terminator v3.1 cycle sequencing kit (Applied Biosystem). Custom-designed primers used for DNA sequencing were purchased from Sigma-Aldrich. The DNA sequences were compared with those available in the GenBank and EMBL databases at the National Center for Biotechnology Information (NCBI) (Altschul et al., 1997).
Constructions of Defined in Frame Mutants
The in-frame deletion of fgi locus and the single defined deletion of AHA_4171 and fgi-1 were obtained by allelic exchange as described by Milton et al. (1996) using the primers listed in Table 2. Briefly, deletion of fgi locus of A. piscicola AH-3 was performed by amplification of DNA regions upstream of fgi-1 and downstream of fgi-12 in two sets of asymmetric PCRs. The single defined deletions were performed by amplification of DNA regions upstream and downstream of fgi-1 of A. piscicola AH-3 and AHA_4171 of A. hydrophila ATCC7966T in two sets of asymmetric PCRs. Primer pairs A-Flgi1 and B-Flgi1, and C-Flgi1 and D-Flgi1, amplify DNA fragments of 776bp (AB-Fgi1) and 687bp (CD-Fgi1) upstream and downstream of fgi-1, respectively. Primer pairs C-Flgi12 and D-Flgi12 amplify a DNA fragment of 732 bp (CD-Fgi12) downstream of fgi-12. Primer pairs A-4171 and B-4171, and C-4171 and D-4171, amplify DNA fragments of 610 bp (AB-4171) and 764 bp (CD-4171) upstream and downstream of AHA_4171, respectively. DNA fragments AB-Fgi1 and CD-Fgi1, AB-Fgi1 and CD-Fgi12, or AB-4171 and CD-4171 were annealed at their overlapping regions and amplified as a single fragment using primers A-Fgi1 and D-Fgi1, A-Fgi1 and D-Fgi12, or A-4171 and D-4171, respectively. The AD fusion products were purified, BglII or BamHI digested, ligated into BglII-digested and phosphatase-treated pDM4 vector (Milton et al., 1996) and electroporated into E. coli MC1061 (λpir) and plated on chloramphenicol plates at 30°C to obtain pDM-Fgi-1, pDM-AH3Fgi, and pDM-AHA4171 plasmids, respectively. Introduction of the plasmids into A. piscicola AH-3 rifampicin-resistant (Rifr), AH-405, or A. hydrophila ATCC7966-Rif was performed by triparental matings using the E. coli MC1061 (λpir) containing the insertion constructs and the mobilizing strain HB101/pRK2073. Transconjugants were selected on plates containing chloramphenicol and rifampicin. PCR analysis confirmed that the vector had integrated correctly into the chromosomal DNA. After sucrose treatment, transformants that were rifampicin-resistant (Rifr) and chloramphenicol sensitive (CmS) were chosen and confirmed by PCR.
Plasmid Construction
Plasmid pBAD33-AHA4171, pBAD33-Fgi-1 and pBAD33-M15300, containing the complete AHA_4171 gene from A. hydrophila ATCC7966T, fgi-1 gene from A. piscicola AH-3 and M001_15300 gene from A. veronii HM21, respectively, under the arabinose promoter (pBAD) on pBAD33 (Guzman et al., 1995) were obtained by PCR amplification of genomic DNAs. Oligonucleotides 5′-GCGCCCGGGAAATCCAGCAGCTTCAATGG-3′ and 5′-GCGTCTAGATGT ATTAGG GCCGCTAGGTG-3′ generated a band of 1204 bp containing the AHA_4171 gene. Oligonucleotides 5′-GGCGATATCGGTAGCCTTGCCCATTTTCT-3′ and 5′-GGCTC TAGAGCGA CAGGTAATCCCACACT-3′ generated a band of ~1,250 bp containing the fgi-1 or M001_15300 gene (the SmaI site is underlined, the XbaI site double-underlined and the EcoRV site is in italic). The amplified band containing the AHA_4171 gen was SmaI and XbaI digested and the amplified bands containing the fgi-1 or M001_15300 gene digested with EcoRV and XbaI. The digested bands were independently ligated into SmaI-XbaI digested pBAD33 vector to construct the pBAD33-AHA4171, pBAD33-Fgi-1 and pBAD33-M15300 plasmids. Recombinant plasmids were introduced by electroporation into the E. coli DH5α (Hanahan, 1983) and sequenced. For complementation assay, the recombinant plasmids were introduced into the AH-3ΔFgi-1 or ATCCΔAHA4171 mutants (Rifr) by triparental mating using the E. coli DH5α containing the recombinant plasmids and the mobilizing strain HB101/pRK2073. Transconjugants were selected on plates containing chloramphenicol and rifampicin.
Motility Assays
Fresh bacterial grown colonies were transferred with a sterile toothpick onto the center of a soft agar plate (1% tryptone, 0.5% NaCl, 0.25% agar). Plates were incubated face up for 24-48 h at 25°C and motility was assessed by examining the migration of bacteria through the agar from the center toward the periphery of the plate. Moreover, swimming motility was assessed by light microscopy observations in liquid media.
Aeromonas Polar Flagella Purification
Purification of Aeromonas polar flagella was carried out from overnight cultures in tryptic soy broth (TSB) at 25°C. Cells were collected by centrifugation at 5,000 × g, and suspended in 100 mM Tris buffer (pH 7.8). Flagella were removed from the cells by shearing in a vortex with a glass bar for 3–4 min, and then passing repetitively (minimum six times) through a syringe. Cells were removed by centrifugation at 8,000 × g for 30 min, and the supernatant centrifuged at 18,000 × g for 20 min. From the remaining supernatant the flagella were pelleted by ultracentrifugation at 100 000 x g for 60 min, and resuspended in 100 mM Tris (pH 7.8) plus 2 mM EDTA buffer. This flagella enriched fraction was purified using a cesium chloride gradient by ultracentifugation at 60 000 × g for 48 h. The band containing the flagella was collected, the cesium chloride removed by extensive dialysis against the same buffer (100 mM Tris 2 mM EDTA). Purified flagella were analyzed by SDS-PAGE or by mass spectrometry analysis.
Mass Spectrometry Analysis of Flagellin Glycopeptides
Purified flagellin was proteolytically digested using trypsin diluted in 50 mM ammonium bicarbonate at a 30:1 protein to enzyme ratio overnight at 37°C, as described previously (Twine et al., 2008). Flagellin digests were analyzed by reversed phase nano liquid chromatography tandem mass spectrometry (nLC-MS/MS) using an M-class high performance liquid chromatography (HPLC) system (Waters Corp). Peptides were first loaded onto a 5 mm × 300 μm C8 (Dionex) and a 20 mm × 180 μm C18 (Waters Corp) trap columns in series. They were subsequently eluted onto a 100 mm × 100 μm C18 BEH column (Waters Corp) for analytical separation. The gradient was applied at 0.5 μL/min as follows: 1–45% solvent B over 18 min, 45–85% solvent B over 3 min, 85–1% solvent B of 1 min, and finally an 8 min re-equilibration at 1% solvent B. Solvent A was 0.1% formic acid in HPLC grade water (Fisher Scientific) and solvent B was 0.1% formic acid in HPLC grade acetonitrile. Peptides were analyzed by electrospray ionization (ESI) using a Synapt G2 quadrupole time of flight (QTOF) mass spectrometer (Waters Corp). Flagellin glycopeptide spectra were annotated by de novo sequencing.
Immunological Methods
Western blot of purified polar flagella was performed as briefly described (Merino et al., 2014). After SDS-PAGE separation of flagella and transfer to nitrocellulose membrane at 1.3 A for 1 h, the membranes were blocked with bovine serum albumin (3 mg/mL), and probed with polyclonal rabbit anti-AH-3 polar flagella antibodies (1:1,000) (Gavin et al., 2002). The unbound antibody was removed by three washes in phosphate buffered saline (PBS), and a goat anti-rabbit immunoglobulin G alkaline phosphatase conjugated secondary antibody (Sigma) (1:1,000) was added. The unbound secondary antibody was removed by three washes in PBS. The bound conjugate was then detected by the addition of 5-bromo-4-chloroindolylphosphate disodium-nitroblue tetrazolium. Incubations were carried out for 1 h, and washing steps with 0.05% Tween 20 in PBS were included after each incubation step.
Results
Identification of the Polar Flagellin Glycosylation Islands (fgi) in A. piscicola AH-3
In A. piscicola AH-3 and A. hydrophila ATCC7699T the genes of the pseudaminic acid biosynthesis locus, pseB (flmA), pseC (flmB), pseF (neuA), pseG (flmD), pseI (neuB) and pseH (flmH), are distributed in two different chromosomal locations. The pseBC genes are separated from the pseHFGI genes by 18 Kb in A. piscicola AH-3, and by 27 Kb in A. hydrophila ATCC7699T (Figure 1). The A. piscicola AH-3 fragment contains 17 open reading frames (ORFs) transcribed in the same direction. The five ORFs downstream of pseH (orf1-5) encode proteins that have shared homology to proteins involved in the channeling of fatty acids into the fatty aldehyde substrate. The remaining ORFs, named fgi-1 to fgi-12, encode proteins with shared homology to a variety of transferases, many of them glycosyltransferases (Figure 2). In order to establish whether genes within this fragment are involved in the biosynthesis of the heptasaccharide that modifies the polar flagellin of A. piscicola AH-3, an in frame deletion of all the genes between the gene downstream of pseC and the gene downstream of the acyl-CoA reductase (luxC) was generated. This mutant, denoted AH-3ΔFgi in A. piscicola AH-3, showed reduced swimming motility in liquid medium and by light microscopy, in comparison to the wild-type strain. Furthermore, its motility in soft agar showed a decreased radial expansion (34% reduction) in relation with the wild-type strain (Figure 3A). Analysis of purified polar flagellum, by SDS-PAGE and western blot, using anti-AH-3 polar flagellum antibodies (Figures 3B,C), showed polar flagellins with lower molecular weight in the AH-3ΔFgi mutant than in the wild-type strain. This difference fit with the loss of most part of the heptasaccharide. Furthermore, the amounts of polar flagellins assembled on the bacterial surface of the AH-3ΔFgi mutant are 3.5 times lower than in the wild-type AH-3 (Figure 3B).
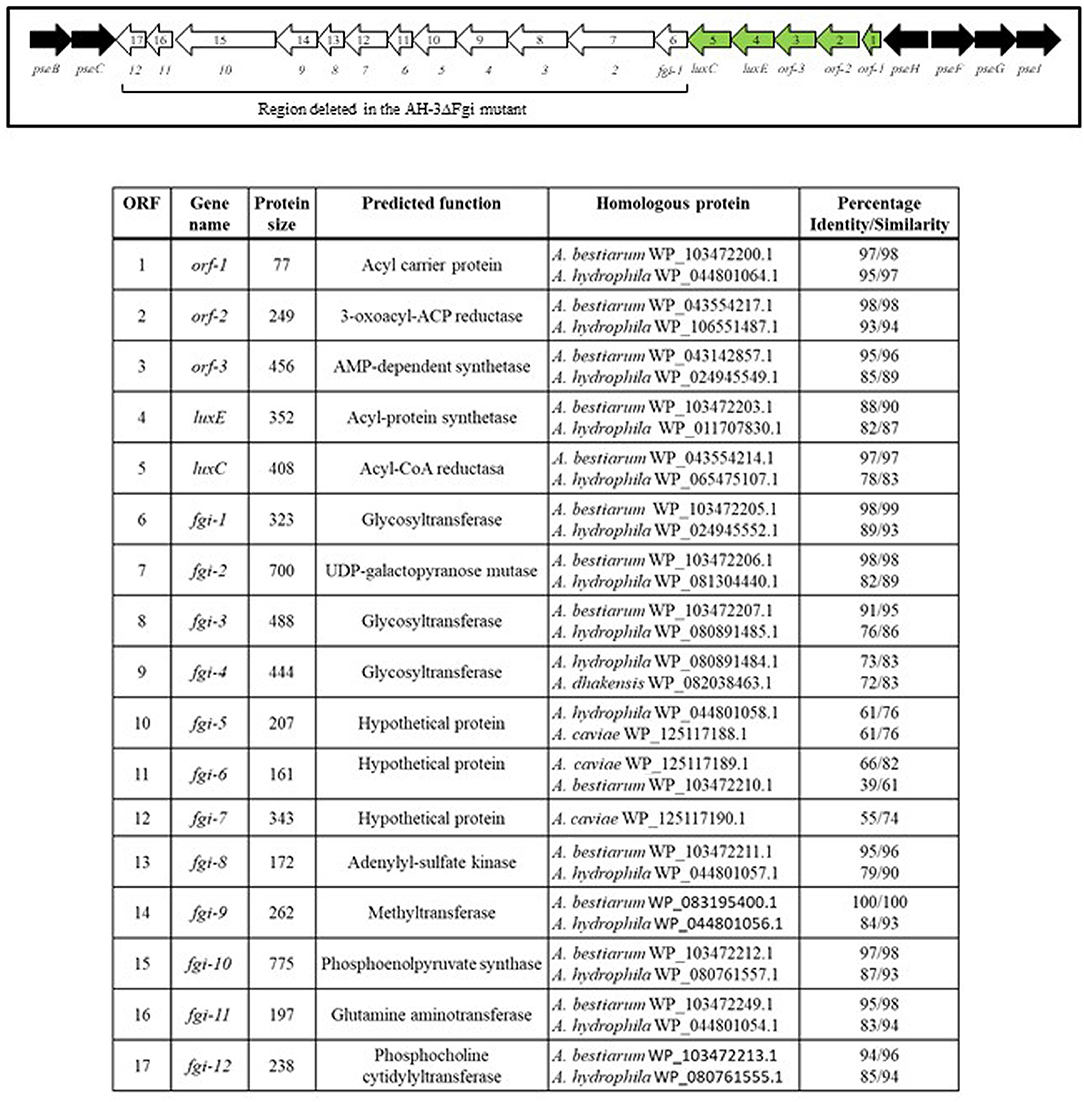
Figure 2. Schematic representation of A. piscicola AH-3 polar flagellum glycosylation island (FGI). Pseudaminic acid biosynthetic genes, in black; polar flagellum glycosylation genes that were deleted in the AH-3ΔFgi mutant, in white; and genes involved in the channeling of fatty acids into the fatty aldehyde substrate, in green.
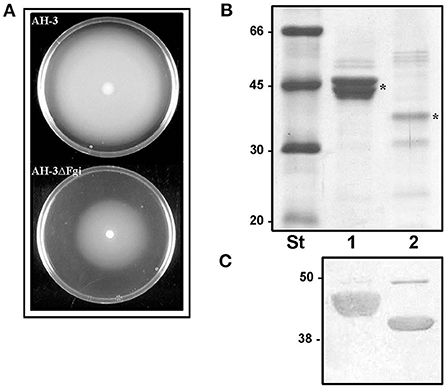
Figure 3. (A) Motility of A. piscicola AH-3 and AH-3ΔFgi mutant grown for 20 h at 25°C on soft agar. Purified polar flagellum from AH-3 (lane1) and AH-3ΔFgi mutant (lane 2) were analyzed using 12% SDS-PAGE (B) and by western blot using A. piscicola anti-AH-3 polar flagella antiserum (1:1,000) (C). Size standard (St). Polar flagellins (*).
Bioinformatic Characterization of the Polar Flagellin Glycosylation Islands (FGIs) in Aeromonas
After identifying and demonstrating the role of this FGI in the polar flagellin glycosylation of A. piscicola AH-3, we characterized the FGIs in 265 mesophilic Aeromonas genomes retrieved from Genbank. The A. piscicola AH-3 PseC (an aminotransferase which aminates at C4 the UDP-4-keto-6-deoxy-L-AltNAc) and PseI (a pseudaminic acid synthase which pyruvylates the 2,4,6-tridoxy-2,4-NAc-L-altrose) were used to locate the pseudaminic acid biosynthesis clusters. Homologous pseC and pseI genes were found in 50 of 52 complete genomes and were not detected in the Aeromonas media WS and the Aeromonas veronii AVNIH1 genomes. Homologs to both genes were also detected in 202 of 213 non-complete genomes. However, 42 of these 202 non-complete genomes present assembly breaks inside this region and these genes are in different contig or scaffold and therefore were not be used in this study.
In 194 of 210 genomes retained for analysis (50 complete and 160 non-complete genomes) the genes related to polar flagellin glycosylation were found aggregated in a genomic island delimited by the pseudaminic acid biosynthetic genes pseB and pseI. However, in 16 of 160 non-complete genomes, the genomic island was delimited by the pseudaminic acid biosynthetic genes pseB and pseF. Homologs of the pseudaminic acid biosynthesis genes pseBCFI were present in all of polar flagellin genomic island of the strains analyzed. However, the presence of pseG and pseH homologs was variable. While 125 strains have both genes, 58 have only the pseG, 22 have only the pseH and four strains do not possess either pseG or pseH.
The genomic regions were observed to be complex, with low average %GC of 49.4–55% and a length ranging from 7.5 to 32.8 Kb. Based on their structure and presence of gene homologs, this genomic glycosylation island was categorized into three main distinct groups across Aeromonadaceae (Figure 4 and Supplementary Table 1).
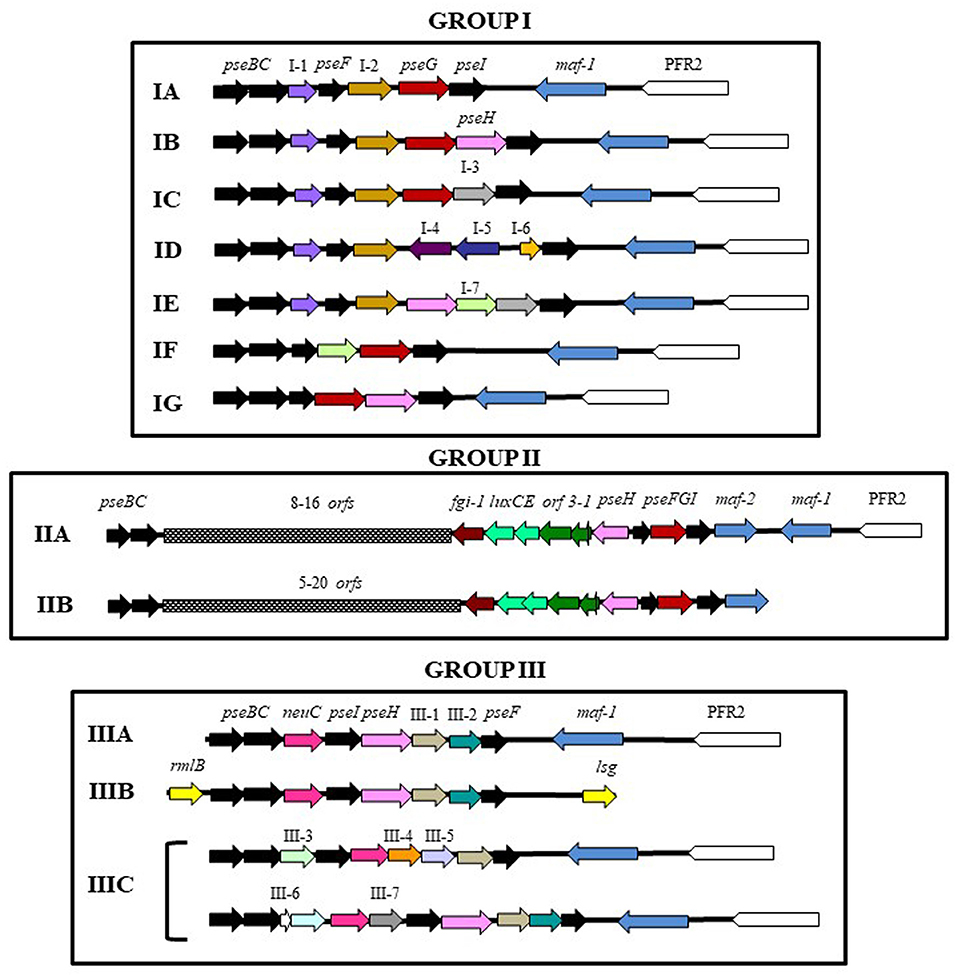
Figure 4. Schematic diagram of the polar flagellin glycosylation islands (FGIs) of mesophilic Aeromonas. PFR2: Polar flagellum region 2. Black, red and pink arrows shows pseudaminic biosynthetic genes. In group I, arrows labeled as I-1 (violet): alpha/keto reductase; I-2 (brown): class III aminotransferase; I-3 (gray): glucuronic acid dehydrogenase; I-4 (purple): HAD-IA family hydrolase; I-5 (dark-blue): methyltransferase; I-6 (orange): hypothetical protein; I-7 (green): deacetylase. In group II, orf1: acyl carrier protein; orf2: 3-oxoacyl-ACP-reductase; orf3: AMP-dependent synthetase; and fgi-1: glycosyltransferase. In group III, yellow arrows (rmlB, lsg) shows O-antigen biosynthetic genes, and arrows labeled as III-1 (light-brown): CBS-domain containing protein; III-2 (blue-green):Gfo/Idh/MocA family oxidoreductase; III-3 (light-green): GNAT family N-acetyltransferase; III-4 (dark-orange): pyridoxal phosphate-dependent transferase; III-5 (light-violet): methyltransferase; III-6 (light-blue): acyl dehydratase; and III-7 (dark-gray): pyridoxal phosphate-dependent transferase.
Group I shows a small polar flagellin glycosylation genomic island ranging from 7.5 to 9.5 Kb, downstream of polar flagellum region 2 (Wilhelms et al., 2011), containing the pseudaminic acid biosynthesis genes, pseBCFI, and genes involved in transference or modification of functional groups, such as deacetylases, reductases, methyltransferases and aminotransferases. In this region, only a gene encoding a glycosyltransferase orthologous to the Maf-1 motility accessory factor of A. caviae Sch3N was found (Parker et al., 2014). This gene is between the polar flagellum region 2 and the flagellin glycosylation island (FGI). Group I contains 96 strains of mesophilic Aeromonas and was divided in seven subgroups (A-G). Subgroup IA shows only a gene encoding an alpha/keto reductase between pseC and pseF, and a gene encoding a class III aminotransferase between pseF and pseG. This subgroup contains 39 strains: 27 belong to A. hydrophila, four belong to each of the A. allosaccharophila and A. veronii species, three belong to Aeromonas sp. and one belongs to A. cavernicola. Since the genomic region containing the polar flagella region 2 and the FGI of A. cavernicola are in different genomic contigs, it is impossible to know if they are adjacent. Subgroup IB and IC show identical gene distribution as IA, but they have additional genes between pseG and pseI. Subgroup IB shows a gene which encoded an N-acetyltransferase (PseH) and IC has a gene encoding a glucuronic acid dehydrogenase. Subgroup IB contains 24 strains: 10 strains belong to the A. caviae species, three belong to Aeromonas sp., two belong to each of the A. encheleia and A. hydrophila species, and one belongs to each A. aquatic, A. eucrenophila, A. lusitana, A. media, A. molluscorum, A. rivipollensis, and A. tecta species. Subgroup IC contains 17 strains: seven belong to A. veronii, three belong to each of the A. bivalvium and A. caviae species, and one belongs to each A. jandaei, A. sobria, Aeromonas sp. and A. veronii bv sobria species. Subgroup ID and IE show identical gene distribution as subgroup IA in the fragment contained between pseB and the gene encoding the class III aminotransferase. None of them shows a gene encoding an ortholog of PseG. Subgroup ID has three genes between the class III aminotransferase gene and pseI: a hypothetical protein, a gene encoding a methyltransferase and a gene encoding a protein belonging to the HAD-IA family hydrolases. This subgroup includes four strains belonging to each of the A. jandaei, A. sobria, Aeromonas sp. and A. veronii species. In A. sobria 08005 and A. veronii FC951 this region also contains a transposase gene. Subgroup IE shows a gene encoding a protein orthologous to PseH downstream of the class III aminotransferase gene and two genes between pseH and pseI: one encoding a deacetylase and the other, encoding a glucuronic acid dehydrogenase. This subgroup includes six strains within the A. caviae and A. veronii species (four and two strains, respectively). Subgroups IF and IG do not show homologous to the alpha/keto reductase and class III aminotransferase genes, which are present in the other subgroups. In subgroup IG, genes involved in the pseudaminic acid biosynthesis, pseBCFGHI, are contiguous and in subgroup IF only a gene encoding a deacetylase is located between pseF and pseG. Subgroup IF includes one strain in each of the A. caviae and A. veronii bv. sobria species and IG includes two strains of A. media, and one strain in each of the A. caviae and A. rivuliii species. However, in the A. caviae GEO_23_Down_B, the genomic region containing the polar flagella region 2 and the FGI are not adjacent (Figure 4 and Supplementary Table 1).
The second FGI group (Group II) was found in 98 strains of mesophilic Aeromonas and the FGI is more complex than in group I. Pseudaminic acid biosynthesis genes, pseBC and pseFGHI, are clustered in two genomic regions. These regions are separated by a fragment of variable length which encodes a variable number of transferases: these are mainly glycosyltransferases, but there are also methyl-, acetyl-, and phospho- transferases depending on the strain. There are also several proteins involved in flatty acid synthesis: an acyl carrier protein (orf1), a 3-oxoacyl-ACP-reductase (orf2), an AMP-dependent synthetase (orf3), an acyl-protein synthetase (luxE), and an acyl-CoA reductase (luxC). A high number of transposases were also found in this region of 32 strains. All the strains, except A. caviae Aer268, show a conserved and specific uncharacterized glycosyltransferase downstream of luxC, that we named flagella glycosylation island 1, fgi-1. Furthermore, they also show, downstream of pseI, a gene encoding a motility factor protein orthologous to Maf-2 of A. piscicola AH-3 (Canals et al., 2007). According to the chromosomal localization of FGI we differentiate two subgroups: IIA and IIB. In subgroup IIA, the FGI is downstream of polar flagellum region 2; however, this is not the location in subgroup IIB, although in all A. veronii and A. caviae strains, as well as in 1 of A. jandaei and 2 of Aeromonas sp. strains it is only 4.5–5.5 Kb away. Independently of their chromosomal localization, all strains have a gene encoding a motility factor protein orthologous to Maf-1 downstream of polar flagella region 2 (Parker et al., 2014). Subgroup IIA contain 32 mesophilic Aeromonas strains: 19 of these strains belong to A. veronii, five belong to A. caviae, two belong to each of the A. enteropelogenes, A. piscicola and A. schubertii species, and 1 belongs to A. bestiarum and Aeromonas sp. Subgroup IIB include 66 strains: 30 belong to A. hydrophila, 15 to A. dhakensis, 9 to A. veronii, 5 to Aeromonas sp., 2 to A. caviae and A. jandaei, and 1 to A. bestiarum, A. enteropelogenes and A. hydrophila subsp. ranae.
The third FGI group (Group III) was found in 16 strains of mesophilic Aeromonas that shows a small FGI delimited by the pseudaminic acid biosynthetic genes pseB and pseF instead of pseI. Furthermore, it also contains a gene encoding an ortholog of UDP-N-acetylglucosamine 2-epimerase, neuC, related to the sialic acid biosynthesis. The group was divided in three subgroups (A-C) according to their chromosomal location, distribution of pseudaminic acid biosynthetic genes and additional genes. Subgroup IIIA and IIIB shows neuC between pseBC and pseIH, as well as two additional genes between pseH and pseF. These additional genes encode a CBS-domain containing protein or an alcohol dehydrogenase and a Gfo/Idh/MocA family oxidoreductase. These two subgroups differ in the chromosomal location of the FGI. In IIIA, it is adjacent to maf-1 which is downstream of the polar flagella region 2; however, genes that could be involved in the biosynthesis of O-antigen lipopolysaccharide are found upstream and downstream of the IIIB glycolyslation island. Subgroup IIIA contain four strains belonging to species A. austraiensis, A. caviae, A. veronii and Aeromonas sp., although in A. caviae CH129, the genes of GGI are transcribed in the same direction than genes of polar flagella region 2. Subgroup IIIB contain eight strains: six belong to A. veronii and the other to A. caviae and A. simiae. Furthermore, A. caviae 8LM and Aeromonas sp. ASNIH5 which shows subgroup IB, also have subgroup IIIB (Figure 4 and Supplementary Table 1). Subgroup IIIC only contains four strains: one belongs to each of A. media and Aeromonas sp., and two belong to A. hydrophila. The chromosomal location of this GGI is such as IIIA but has additional genes. A. media S2_009_000_R3_19 show a gene which encodes a GlcNAc-PI de-N-acetylase, between pseI and neuC, and two additional genes which encode a GNAT family N-acetyltransferase and an acyl dehydratase, between neuC and pseC. The other strains do not show a gene orthologous to pseH and pseI is located upstream neuC. Furthermore, they show an additional gene downstream of pseC which encodes a GNAT family N-acetyltransferase; however, they have three additional genes upstream of pseF which encode for a CBS-domain containing protein, a methyltransferase and a pyridoxal phosphate-dependent transferase (Figure 4 and Supplementary Table 1).
Bioinformatic Characterization of Glycosyltransferase Fgi-1
With the exception of A. caviae Aer268, all mesophilic Aeromonas strains grouped within FGI Group II (97 strains), showed a conserved glycosyltransferase downstream of luxC. In A. piscicola AH-3, this glycosyltransferase was named flagella glycosylation island 1, fgi-1 (Figure 4). The Fgi-1 protein sequence has 327 amino acid residues, a predicted molecular weight of 37.44 kDa and a predicted pI of 4.98. Homologous proteins in polar FGI group II range between 313-327 residues, with predicted molecular weights of 35.67-37.39 kDa and predicted isoelectric points (pI) of 4.98-6.16 (Supplementary Figure 1). Sequence identity ranges between 100 and 54% across all sequences and according to CDD and SMART, all sequences shows a glycosyltransferase family 2 (GT-2) conserved domain in the first 100 amino acids. No signal peptide or transmembrane domains were predicted in the protein sequences according to SignalP-HMM v4.1 and TMHMM Server v2.0, therefore Fgi-1 is predicted to be active in the bacterial cytoplasm.
Clustering of the 97 Fgi-1 protein sequences shows higher conservation in the N-terminal sequence containing the GT-2 domain compared to the rest of the sequence. This highlighted three different groups with more than 80% intragroup sequence identity (Figure 5 and Supplementary Figure 1). A phylogenetic tree generated by the neighbor-joining method on the basis of the Fgi-1 amino acid sequences also shows these three groups (Figure 6). The first group (Group 1) contains 30 mesophilic Aeromonas strains: 16 belong to A. veronii, eight belong to A. hydrophila, two belong to A. dhakensis, one belongs to each of the A. jandaei, A. shubertii, A. enteropelogenes and Aeromonas sp. The second group (Group 2) contains 10 mesophilic Aeromonas strains: five belong to A. veronii, three belong to A. caviae, and one belongs to each of the A. enteropelogenes and Aeromonas sp species. The third group (Group 3) is the most extended and contain 57 mesophilic Aeromonas strains: 22 belong to A. hydrophila, 13 belong to A. dhakensis, seven belong to A. veronii, four belong to Aeromonas sp, three belong to A. caviae, two belong to each of the A. piscicola and A. bestiarum species, and one belongs to each of the A. jandaei, A. shubertii, A. enteropelogenes and A. hydrophila subs ranae.
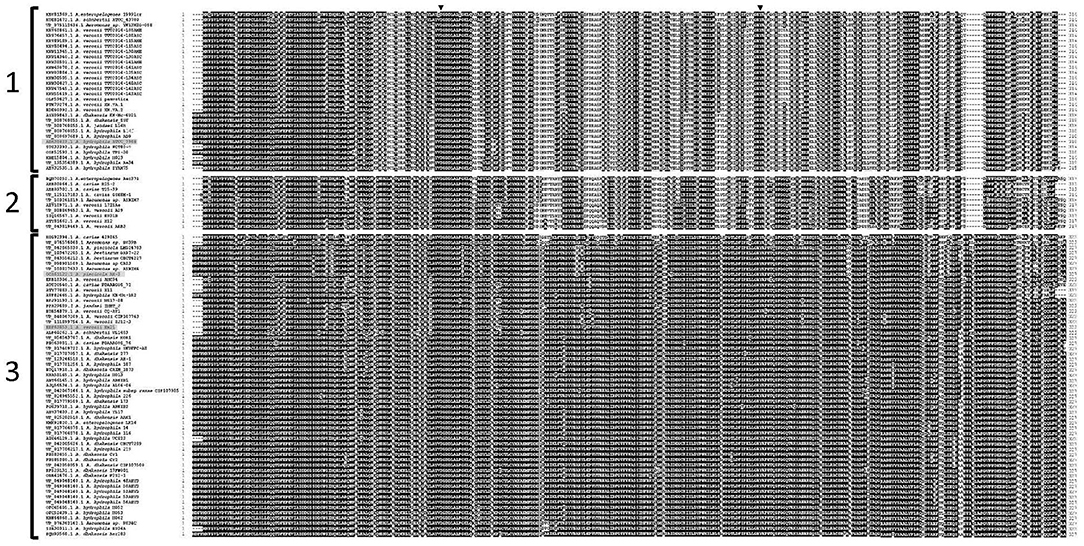
Figure 5. Schematic alignment, performed by ClustalW, of the Fgi-1 amino acid sequences of mesophilic Aeromonas strains with polar flagella glycosylation islands group II. Fgi-1 groups are separated by brackets and sequences labeled in gray were used in the complementation assays. Black triangles show the Asp94 (D94) and Tyr215 (Y215) interacting residues at the protein pocket.
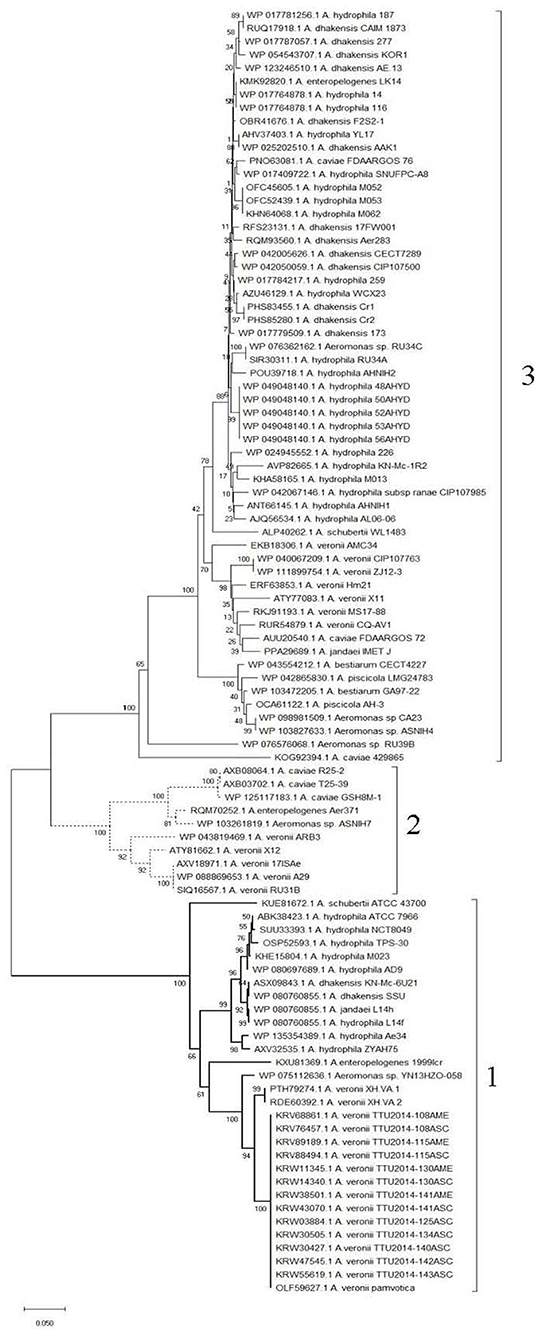
Figure 6. Phylogenetic tree generated by the neighbor-joining method on the basis of the Fgi-1 amino acid sequences. Brackets show the three Fgi-1 groups.
The Fgi-1 of A. piscicola AH-3 (OCA61122.1), C7U63_02395 of A. veronii 17ISAe (AXV18971.1) and AHA_4171of A. hydrophila ATCC7966 (ABK38423.1) were used as models of group 1, 2, and 3, respectively, to analyze intergroup sequence identities. Sequence identities were 66, 60, and 55% between groups 2 and 3, 1 and 2, and 1 and 3, respectively.
Secondary structure of the protein analyzed with SOPMA predicted that it is predominantly alpha helixical (41.90%) and random coils (34.56%), with a minor part of the structure predicted to be extended strands (15.60%). Finally, we studied the Fgi-1 tertiary structure using two different methods: homology modeling with Phyre2, and threading modeling with LOMETS in I-Tasser (Figures 7A,B). Both approaches modeled most of the Fgi-1 tertiary structure based on the conformation of available glycosyltransferase enzymes. Phyre2 used five templates (PDB: c2z86D, c5tz8C, c5heaA, d1gg8a and c4hg6A) with >99% confidence and sequence identity of 21 to 18%, while the I-Tasser model obtained a maximum C-Score of−0.6 based on a Family 2 glycosyltransferase from S. parasanguinis (PDB: c5heaA).
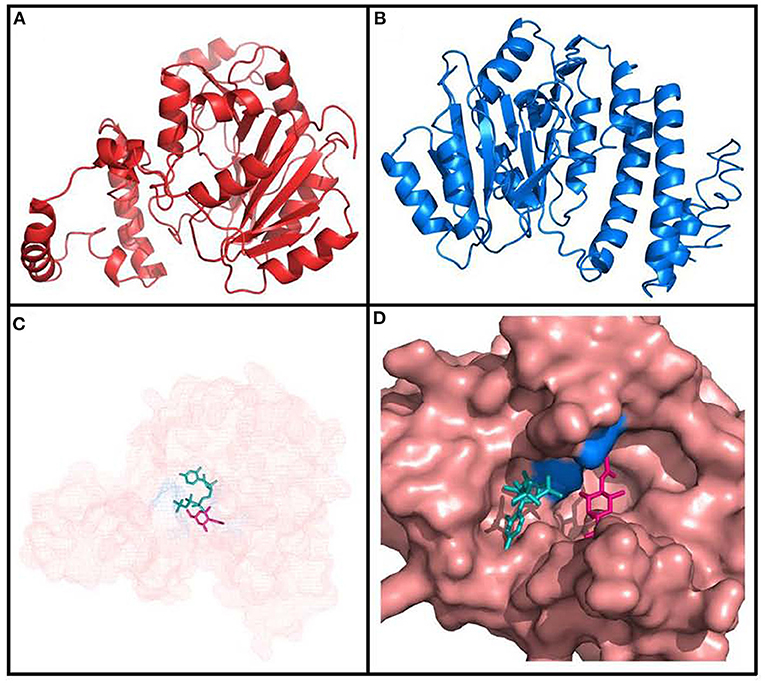
Figure 7. Tertiary structure of Fgi-1 as modeled by (A) Phyre2, and (B) I-Tasser. (C) The glycosyltransferase main pocket [amplified in (D)] has predicted binding sites with complex carbohydrates, as N-acetyl-D-hexosamine (pink), energy donors (ATP/CTP, in green), and Mg ions. The ASP94 and TYR215 aminoacids (blue) are predicted to be ligand binding sites.
The Phyre2 model was further sent to the 3DLigandSite server to predict three-dimensional ligand binding sites with crystallized components available on PDB. 3DLigandSite found four possible ligand binding sites with carbohydrates with an average MAMMOTH score of 18.0995 (>7 is significant). In some instances, other possible binding sites with Mg and Cu ions as cofactors were also reported. Sucrose, Galactose, N-acetyl-D-galactosamine, ATP/CTP, ADP/CDP, and Mg were identified as possible heterogens interacting in the most significant protein pocket, and Asp94 and Tyr215 as the active residues interacting with them (Figures 7C,D). The other possible binding sites also predicted interaction with the heterogens N-acetyl-D-galactosamine, galactose, and Mg. These results are strengthened by the total conservation of the aforementioned putative active binding site residues across all homologous Fgi-1 sequences (Figure 5 and Supplementary Figure 1). I-Tasser results largely agreed with the previous results, predicting ligand binding sites with UDP, N-acetyl-D-galactosamine, and alpha-D-mannose, and marking the ASP94 as predicted active site residue.
Functional Characterization of Fgi-1 of A. piscicola AH-3 and AHA_4171 of A. hydrophila ATCC7966T
Amino acid sequence analysis shows that Fgi-1 of A. piscicola AH-3 (OCA61122.1) and AHA_4171 of A. hydrophila ATCC7966T (ABK38423.1) are included in different Fgi-1 groups (Figures 5, 6). While Fgi-1 of A. piscicola AH-3 belongs to group 1, AHA_4171 of A. hydrophila ATCC7966T belongs to group 3. To elucidate their involvement in polar flagellum glycosylation, we constructed specific in-frame mutants in the Fgi-1 of A. piscicola AH-3 (AH-3ΔFgi-1) and the AHA_4171 of A. hydrophila ATCC7966T (ATCCΔAHA4171) using the suicide plasmids pDM-Fgi-1 or pDM-AHA4171, respectively. Specific deletion of genes was confirmed by PCR and sequence analysis. Both mutants showed a reduced swimming motility in liquid medium, by light microscopy, and their motilities in soft agar showed a decreased radial expansion (36 and 39% reduction) in relation with their respective wild-type strain (Figure 8A). Furthermore, as described in the AH-3ΔFgi mutant, analysis of purified polar flagellum, in 12% SDS-PAGE, showed a reduction in the amount of polar flagellins assembled in the bacterial surface and polar flagellins with lower molecular weight, in relation to their respective wild-type strain. In addition, polar flagellins of the AH-3ΔFgi-1 mutants show the same molecular weight than the AH-3ΔFgi mutant (Figure 8B).
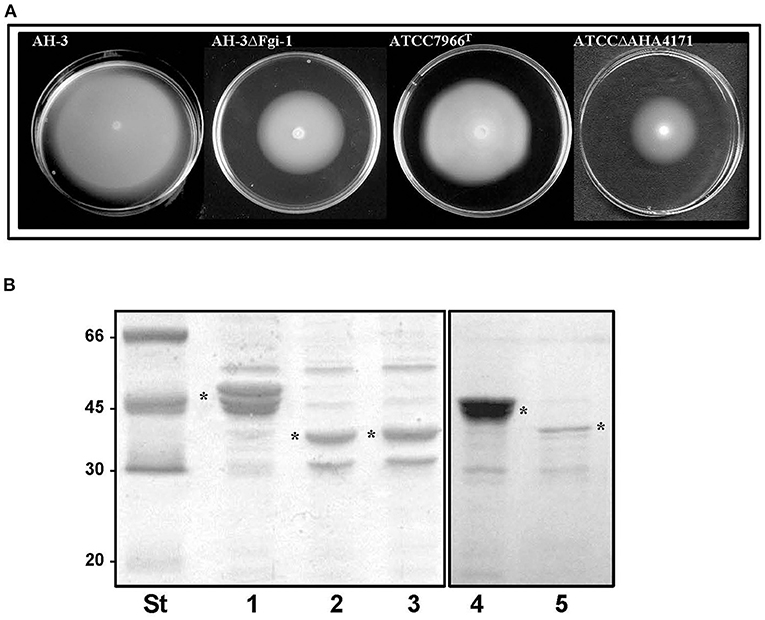
Figure 8. (A) Motility on soft agar of A. piscicola AH-3 and A. hydrophila ATCC7966T, and their mutants AH-3ΔFgi-1 and ATCCΔAHA4171grown 20 h at 25°C on soft agar. (B) Purified polar flagellum from AH-3 (lane1), AH-3ΔFgi-1 (lane 2), AH-3ΔFgi (lane 3), ATCC7966T (lane 4) and ATCCΔAHA4171 (lane 5) analyzed in 12% SDS-PAGE. Size standard (St). Polar flagellins (*).
Mass Spectrometry Characterization of Fgi-1 of A. piscicola AH-3
Our previous work described the normal polar flagellin glycosylation of A. piscicola AH3 (Wilhelms et al., 2012). Several sites of modification with a complex and heterogenous heptasaccharide were observed. The glycan chain consisted of a pseudaminic acid derivative (376 Da) linking sugar, followed by two consecutive hexoses (Hex; 162 Da each), three N-acetylhexosamines (HexNAc; 203 Da) with and without a variable number of additional phosphate (+80 Da) and methyl (+14 Da) modifications, and finally an unknown carbohydrate moiety of 102 Da. Figure 9A shows a representative spectrum of the wild type T18 peptide 207AASSAQLAMANLDFMIK223 of FlaB. In this case, the mass difference between the precursor ion (m/z 1092.13+) and the unmodified peptide ion (m/z 1781.9+) is 1491.5 Da. This mass difference combined with the observation of several glycan oxonium ions in the lower m/z range of the spectrum (i.e., ions at 204.1+, 284.1+, 377.2+, 407.2+, 487.1+, 528.2+, 690.2+, and 731.3+ m/z) indicates that the glycan is in fact a heptasaccharide comprised of the pseudaminic acid derivative, along with two Hex, two HexNAc, a phosphorylated HexNAc, and the unknown 102 Da moiety.
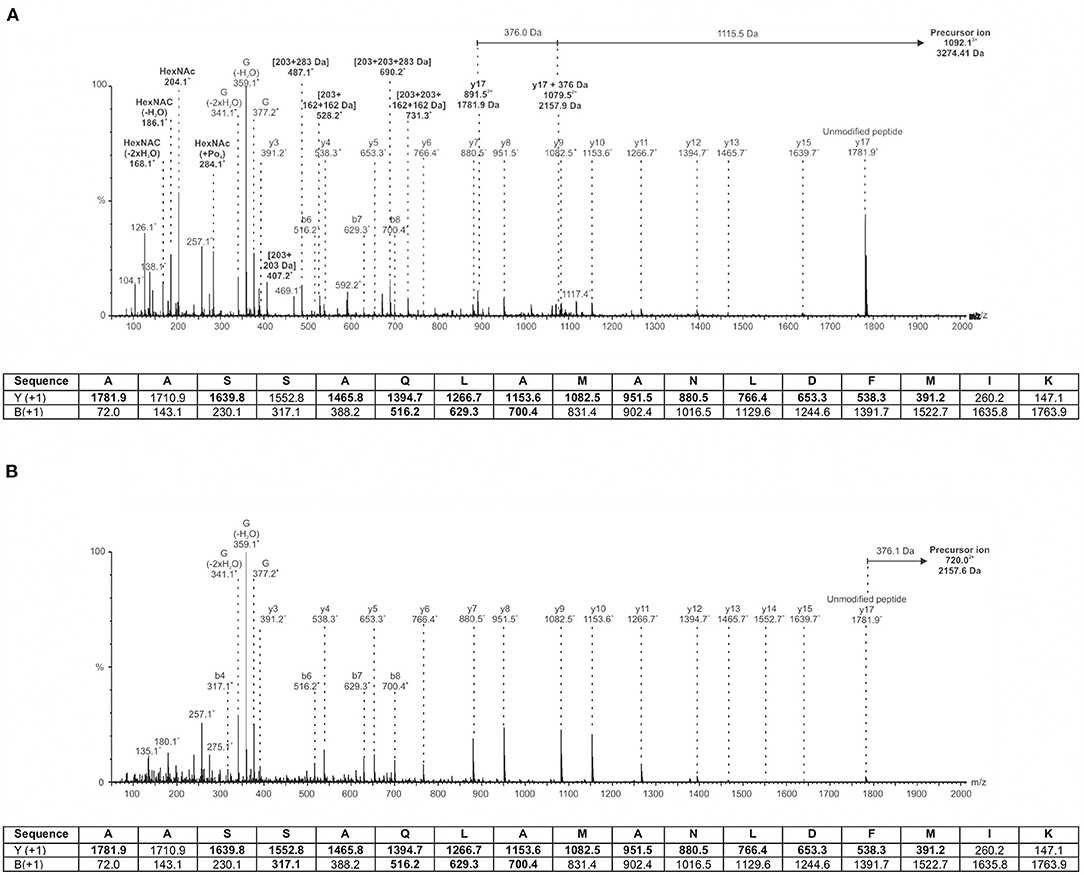
Figure 9. MS/MS spectra of an A. piscicola AH-3 polar flagellin glycopeptide. (A) Wild-type flagellin is modified by a heterogenous heptasaccharide containing a pseudaminic acid derivative, hexoses, HexNAc with variable phosphorylation and methylation, and an unknown moiety of 102 Da, as observed previously (Wilhelms et al., 2012). Depicted is a representative spectrum of the T18 peptide 207AASSAQLAMANLDFMIK223 harboring a 1491 Da heptasaccharide glycan that is comprised of a pseudaminic acid derivative of mass 376.14 Da (G) along with two hexoses, two N-acetylhexosamines, a phosphorylated N-actecylhexosamine, and the unknown 102 Da moiety. (B) AH-3ΔFgi-1 mutant flagellin is modified by a single monosaccharide only. Depicted is a representative spectrum of the T18 peptide 207AASSAQLAMANLDFMIK223 harboring the 376 Da pseudaminic acid derivative only. Ions indicating carbohydrate related ions in wild-type (A) that are not observed in the glycosyltransferase mutant (B) are denoted in bold text. The table below each spectrum indicates the predicted peptide type y and b fragment ions, with those detected indicated in bold.
In contrast, deletion of the putative glycosylatransferase fgi-1 results in a single monosaccharide modification of 376 Da, as shown in Figure 9B. The mass difference between the precursor ion (m/z 720.03+) and the same unmodified FlaB peptide ion (m/z 1781.9+) is 376.1 Da. There is a corresponding glycan oxonium ion observed at m/z 377.2. However, there are no ions indicating the presence of Hex, HexNAc (with or without variable phosphorylation and methylation), or the 102 Da moiety. The observation of a single pseudaminic acid derivative modification is consistent for all observed FlaA and FlaB glycopeptides in the AH-3ΔFgi-1 mutant (data not shown).
Complementation and Cross-Complementation of Homologous Fgi-1 of Aeromonas
To determine whether homologous Fgi-1 are able to complement the AH-3ΔFgi-1 and ATCCΔAHA4171 mutants, we cloned the Fgi-1 of A. piscicola AH-3 (pBAD33-Fgi-1), the AHA_4171 of A. hydrophila ATCC7966T (pBAD33-AHA4171) and the M15300 of A. veronii Hm21 (pBAD33-M15300). Fgi-1 of A. piscicola AH-3 and M15300 of A. veronii Hm21 are included in the Fgi-1 group 1, while AHA_4171 of A. hydrophila ATCC7966T is included in the Fgi-1 group 3. Complementation and cross-complementation assays showed that the molecular weight of the polar flagellin of AH-3ΔFgi-1 mutant was increased significantly when Fgi-1 of A. piscicola AH-3 or M15300 of A. veronii Hm21 were introduced, suggesting that the heteroglycan is transferred to polar flagellin (Figures 10A,C). Both complemented strains show similar polar flagellin profiles and have a molecular weight identical to the wild-type AH-3 flagellin. However, cross-complementation of AH-3ΔFgi-1 mutant with AHA_4171 of A. hydrophila ATCC7966T only slightly increased the polar flagellin molecular weight (Figures 10A,B), suggesting that this glycosyltransferase was able to link some sugars to the pseudaminic acid derivative on the polar flagellin. When ATCCΔAHA4171 mutant was complemented and cross-complemented, only its own glycosyltransferase was able to increase the polar flagellin molecular weight back to the molecular weight of the wild type, while trans-complementation only showed a partial complementation of the flagellin molecular weight (Figures 10D,E).
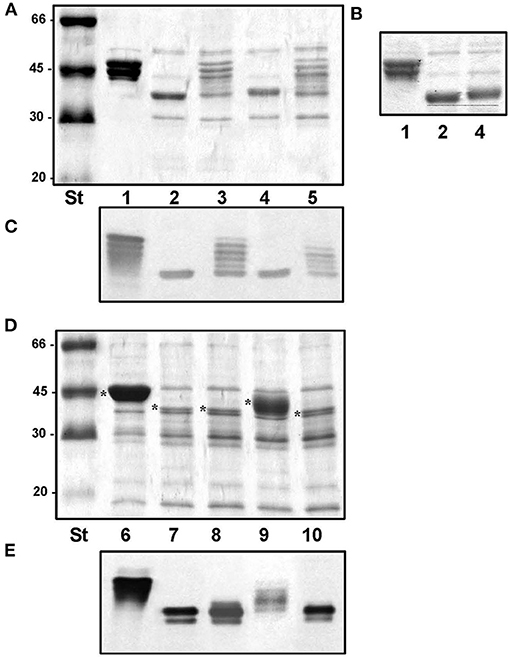
Figure 10. Purified polar flagellum of AH-3 (lane1), AH-3ΔFgi-1 (lane 2), AH-3ΔFgi-1 complemented with the Fgi-1 of A. piscicola AH-3 (pBAD33-Fgi-1) (lane 3), with AHA_4171 of A. hydrophila (pBAD33-AHA4171) (lane 4) and with M15300 of A. veronii (pBAD33-M15300) (lane 5) analyzed using 12% SDS-PAGE (A). Analyzed using 7.5% SDS-PAGE (B). Western blot using A. piscicola anti-AH-3 polar flagella antiserum (1:1,000) (C). The dotted line shows the difference in molecular weight between AH-3ΔFgi-1 and AH-3ΔFgi-1 complemented with pBAD33-AHA4171. Size standard (St). Purified polar flagellum from ATCC7966T (lane 6), ATCCΔAHA4171 (lane 7) and ATCCΔAHA4171 complemented with pBAD33-Fgi-1 (lane 8), pBAD33-AHA4171 (lane 9) and pBAD33-M15300 (lane 10) analyzed using 12% SDS-PAGE (D). Western blot using A. piscicola anti-AH-3 polar flagella antiserum (1:1,000) (E). Size standard (St). Polar flagellins (*).
Discussion
Glycosylation of secreted proteins is a crucial co- and post-translational modification not only with structural roles but also involved in intrinsic organism intercellular communication, as well as in host-pathogen and symbiont interactions. Glycans and glycoproteins have been widely used as a self/non-self and immune recognition mechanism throughout evolution. Examples include the ABO blood types in humans, vertebrate toll-like receptors (Barton and Medzhitov, 2003), lectins in animals and plants, and the bacteriophage tail spike proteins in viruses (Broeker and Barbirz, 2017). It has been recently reported that glycosylation of extracellular proteins is also common in Gram-negative bacteria, such as Aeromonadaceae and Enterobacteriaceae, and that the glycans involved in this process have functional roles and immunogenic properties (De Maayer and Cowan, 2016).
Mesophilic Aeromonas possess a constitutive and O-glycosylated polar flagellum, whose glycan composition ranges from a single pseudaminic acid derivative to a heteropolysaccharide (Canals et al., 2007; Tabei et al., 2009). The extended glycan has been reported to attach to the flagellin monomers via a pseudaminic acid derivative linking sugar and its presence is structurally required for the assembly and function of the polar flagellum filament (Canals et al., 2007). Glycosylation of flagellin has been shown to be essential for flagellar filament assembly in other bacteria as well, with elimination of glycosylation having a significant impact on motility which is a known virulence factor in host-pathogen interactions. For example, in Clostridium difficile, disruption of the flagellin glycosyltransferase CD0240 resulted in improperly formed flagellar filaments and a significant reduction in bacterial motility by stab agar assay (Twine et al., 2009). Similar observations have been made for Campylobacter jejuni (Goon et al., 2003), Helicobacter pylori (Ménard et al., 2014), and Listeria monocytogenes (Schirm et al., 2004), when key glycosyltransferase enzymes are genetically disrupted.
In this report, we looked at the genetic basis of O-glycosylation of polar flagellin in Aeromonas, exploring the different genomic loci that are responsible for the extensive diversity of glycans which modify polar flagellins. In 98 mesophilic Aeromonas strains, including A. piscicola AH3 and A. hydrophila ATCC7966T, we identified homologs of known pseudaminic acid biosynthetic genes pseBCFGIH (known as flm and neu in some Aeromonas) distributed in two chromosomal locations. It is known that disruption of the pse genes completely eliminates lateral and polar flagellin glycosylation in A. piscicola AH3, which consequently abolishes flagellar filament formation and bacterial motility (Wilhelms et al., 2012). Here, we have further investigated the genomic fragment of A. piscicola AH3 that lies between the pseBC and pseFGIH genes to better characterize the genetic basis of polar flagellin glycosylation. This region encodes for several glycosyltransferase homologs, and deletion of these genes (fgi-1 to fgi-12) leads to partial disruption of polar flagellin glycosylation in A. piscicola AH3, but do not affect lateral flagellin glycosylation. This is indicated by the reduced molecular weight of the polar flagellin proteins and a corresponding reduction of bacterial motility. This suggests that enzymes within this region are involved in the synthesis of carbohydrates or assembly of the glycan beyond the linking sugar. By contrast, Aeromonas strains whose polar flagellin glycan has only a single pseudaminic acid derivative to begin with, such as A. hydrophila AH-1 and A. caviae Sch3N (Tabei et al., 2009; Fulton et al., 2015), do not contain glycosyltransferase homolog genes between the pseudaminic acid biosynthetic genes. Collectively, these data suggest that the Pse sugar is essential for assembly of the flagellar filament and that full glycosylation mediated by glycosyltransferases within pse genes are required for full glycosylation and consequently, flagella filament stability and its full motility.
The bioinformatic analysis of 265 mesophilic Aeromonas genomes retrieved from Genbank shows that A. media WS and A. veronii AVNIH1 complete genomes do not possess pse homologous genes, which are essential for pseudaminic acid biosynthesis. This suggests that their polar flagella are probably not modified with a pseudaminic acid derivative. Furthermore, in 194 genomes examined, the genes putatively related to polar flagellin glycosylation are clustered in highly polymorphic genomic islands with low average GC content and flanked by pseudaminic acid biosynthetic genes. All of these polar flagella glycosylation islands (FGIs) contain pseBCFI genes, with the presence of pseG and pseH being variable. This variability is produced because the glycosyltransferase domain of PseG and the RimL-like acetyltransferase domain of PseH can be found in the same protein as described in A. caviae Sch3N (Tabei et al., 2009). Therefore, strains with only one of these genes may have a chimeric protein with both domains, although a more thorough analysis of all these proteins would be required to confirm this hypothesis. Nevertheless, the presence of pse genes and a gene homolog of maf-1, which encodes a motility-accessory factor, adjacent to the polar flagella region 2, suggest that most mesophilic Aeromonas strains have polar flagellins modified by at least one pseudaminic acid derivative. Maf proteins are considered as candidate transferase enzymes in Campylobacter jejuni (Karlyshev et al., 2002) and, in this regard, Maf-1 of A. caviae Sch3N, has been shown to be responsible for the transfer of pseudaminic acid to the polar flagellins prior to secretion through the flagellar type III secretion system (Parker et al., 2012).
Aeromonas FGIs are highly polymorphic but the genetic organization of their homologous genes allows us to classify them in three groups. Groups I and III are small genomic glycosylation islands almost exclusively containing the pse genes and some genes involved in the transference or modification of functional groups, such as deacetylases, reductases, and methyl- and amino- transferases. Group II is a large genomic island containing different glycosyltransferases and proteins involved in fatty acid synthesis, located between the pse genes. A. piscicola AH3 and A. hydrophila ATCC7966T belong to this group.
The majority of aeromonads, when classified using the described FGIs, fall into Groups I and II, with only 16 strains classified in Group III. Strains of A. hydrophila, A. caviae and A. veronii are broadly distributed across all three groups, although they show a different subgroup distribution. Thus, while FGIs of most A. hydrophila strains belong to subgroups IA and IIB, A. veronii strains belong to subgroup IIA, and A. caviae strains to subgroup IB. FGIs of A. media strains belong to group I or III, and A. jandaei to group I or II. FGIs of other mesophilic Aeromonas species only belong to one FGI group. In particular, FGIs of 12 species only belong to Group I (A. allosaccharophila and A. cavernicola to IA; A. aquatic, A. encheleia, A. eucrenophila, A. lusitana, A. molluscorum, A. rivipollensis and A. tecta to IB; A. bivalvium and A. sobria to IC; and A. rivuli to IG), FGIs of five species only belong to Group II (A. piscicola and A. schubertii to IIA; A. dhakensis to IIB; and A. bestiarum and A. enteropelogenes to IIA and B), and FGIs of two species only belong to Group III (A. australiensis to IIIA; and A. simiae to IIIB).
As described in C. jejuni, C. coli and P. aeruginosa (Arora et al., 2001; Szymanski et al., 2003), most Aeromonas FGIs are located downstream of their glycosylation target, the polar flagellins. However, while all Group I FGIs are found downstream of the polar flagella region 2, some Group II (IIB) and III (IIIB) FGIs are not closely located to the genes encoding flagellar structural proteins. Group IIIB FGIs were chromosomally located adjacent to homologs of genes thought to be involved in O-antigen biosynthesis. This genomic distribution suggests that Group IIB and IIIB FGIs could be involved in the transfer of glycans to different bacterial structures. This has been described in A. caviae Sch3N, whose FGI is involved in both polar flagella glycosylation and O-antigen biosynthesis, using different and specific transferases (Tabei et al., 2009).
The genomic distribution of pse genes in Group I and II FGIs is compared with that observed in Group III. While all FGI groups show the pseBC genes at one end, Groups I and II are flanked by pseI gene at the other end, and Group II by pseF. Interestingly, Group III contains a homolog of neuC, which encodes an ortholog of UDP-N-acetylglucosamine 2-epimerase, involved in sialic acid synthesis (Ringenberg et al., 2003). NeuC initiates the biosynthesis of CMP-sialic acid from UDP-N-acetylglucosamine and, given the similarity between the enzymes involved in different nonulosonic acid biosynthetic pathways, strains with FGIs of Group III might contain sialic acid in addition to pseudaminic acid modifying the polar flagellins.
All mesophilic Aeromonas genomes with FGIs show, downstream of the polar flagella region 2, a motility accessory factor gene homologous to maf-1 of A. caviae Sch3N. The encoded protein is responsible for the transfer of pseudaminic acid to the polar flagellins (Parker et al., 2012, 2014). However, all the strains with Group II FGIs have a second motility accessory factor (maf-2) adjacent to the pseHGI genes, whose function has not been described. Therefore, a relatively small FGI, containing pse homologs and a unique maf gene downstream of the polar flagellins (Group I or III), suggest that flagellin proteins of strains are modified with a single nonulosonic acid. An example of this has been reported in A. hydrophila AH-1 (Fulton et al., 2015). In contrast, the presence of an FGI with several glycosyltransferases between pse genes (Group II) and a second motility accessory factor closely linked to the FGIs, could be related to the biosynthesis of heteropolysaccharidic glycans observed to modify polar flagellins, as described in A. piscicola AH-3 (Wilhelms et al., 2012). Furthermore, the presence of gene homologs encoding proteins putatively involved in fatty acid synthesis suggests that polar flagellin glycans may incorporate a liposugar component in strains with FGIs of Group II.
Although pse genes, maf-2 and the genes encoding proteins involved in fatty acid synthesis are highly conserved in strains with Group II FGIs, the genetic variability of the chromosomal fragment between luxC and pseC is very high. This fragment contains genes encoding different glycosyltransferases, enzymes involved in the transference or modification of functional groups and, in some strains, also sugar biosynthetic genes and transposases. This suggests that the presence of several different glycosyltransferases in this genomic region dictates the number of carbohydrates of the polar flagella glycan. Furthermore, except for A. caviae Aer268, all Group II strains show a gene downstream of luxC encoding a conserved glycosyltransferase with a GT-2 domain and a GT-A type structural fold that we named Fgi-1 in A. piscicola AH-3. In-frame mutants of Fgi-1 and its homologous protein in A. hydrophila ATCC7966T, AHA_4171, showed a reduction in the amount of polar flagellins assembled in the bacterial surface. Furthermore, the polar flagellins in these mutant strains also had lower molecular weights as compared to their respective wild-type strains, and compromised swimming motility. Furthermore, AH-3ΔFgi-1 polar flagellins show the same molecular weight as those of the AH-3ΔFgi mutant. Mass spectrometry analysis of glycopeptides confirms that AH-3ΔFgi-1 polar flagellin is modified by a single O-linked pseudaminic acid derivative linking sugar whereas the wildtype is modified by the heptasaccharide that includes a pseudaminic acid linking sugar. These data suggest that this conserved glycosyltransferase is an important enzyme for the polar flagellin glycosylation in Aeromonas strains with type II FGIs, as well as Fgi-1 and homologous proteins found in many Aeromonads could be involved in the transfer of additional sugars to the pseudaminic acid derivative linked to the polar flagellins.
The alignment of Fgi-1 homologous proteins shows a highly conserved N-terminal sequence. This region corresponds to the Rossmann-type nucleotide-binding domain that is terminated by the DxD motif. This motif, as well as the requirement of a divalent cation for activity, is a general feature of the GT-A family of proteins (Breton and Imberty, 1999). Analysis of Fgi-1 of A. piscicola AH-3, using the 3DLigandSite server, shows several possible binding sites with Mg and Cu ions in the most significant protein pocket, being Asp94, into the DxD motif, and Tyr215 the interacting residues. The C-terminal region of the protein shows more variability, as described in GT-A glycosyltransferases, and we classified the Fgi-1 homologous proteins in three groups with more than 80% intragroup identity and 55 to 66% intergroup identity. Cross-complementation assays with Fgi-1 homologous proteins, such as M15300 of A. veronii Hm21 and AHA_4171 of A. hydrophila ATCC7966T, show that only glycosyltransferases belonging to the same group are able to fully restore the AH-3ΔFgi-1 and ATCCΔAHA4171 mutants to their respective wild-type phenotypes. Thus, while Fgi-1 of A. piscicola AH-3 and M15300 of A. veronii Hm21 are able to fully restore the AH-3 phenotype in the AH-3ΔFgi-1 mutant, AHA_4171 of A. hydrophila ATCC7966T only slightly increases the polar flagellin molecular weight of this mutant. In a similar way, only AHA_4171 of A. hydrophila ATCC7966T is able to fully restore the ATCC7966T phenotype in the ATCCΔAHA4171 mutant. Collectively, these data could be related to the conserved C-terminal region of Fgi-1 homologous proteins, given that the C-terminal portion of GT-A glycosyltransferases involved in the recognition of the acceptor and, in some proteins, the region corresponding to β6-α4-α5 contains residues that interact with both the donor and the acceptor sugars (Persson et al., 2001). Taken together, these data suggest that Fgi-1 homologous proteins belonging to different groups could transfer the first sugar to the pseudaminic acid derivative which is linked to the amino acid residue, as observed in the AH-3ΔFgi-1 mutant complemented with the AHA_4171 of A. hydrophila ATCC7966T and in ATCCΔAHA4171 complemented with the Fgi-1 of A. piscicola AH-3. However, the heteropolysaccharidic glycan cannot be built, perhaps because the sugar transferred is not identical to that found in the parental strain or because its linkage with the pseudaminic acid derivative is different and cannot be recognized by other glycosyltransferases involved in the construction of the glycan. Further research is needed to clarify this question.
In the context of host-pathogen interactions, adherence for colonization and infection is an essential step in bacterial pathogenesis. In many motile pathogenic bacteria, flagellin glycosylation can be considered a key virulence factor as it is often required for proper flagella filament assembly and stability, immune evasion, and adhesion to specific host receptors (Twine et al., 2009; Asakura et al., 2010; Nothaft and Szymanski, 2010; Merino and Tomás, 2014). Therefore, the mechanisms of flagellin glycosylation may represent a novel target for antimicrobial development (Fulton et al., 2016) and as such, understanding the genetic factors responsible will continue to be important.
Data Availability Statement
The original contributions presented in the study are included in the article/Supplementary Material, further inquiries can be directed to the corresponding author/s.
Author Contributions
SM and JT conceived the project. GF-C and SM performed the genome analyses and conducted the bioinformatics analyses. KF, JS, and ST performed the mass spectrometry analysis. EM-B and SM constructed the defined mutants and plasmid. EM-B and JT performed and analyzed the cross-complementation assays. SM, KF, and ST prepared the manuscript. All authors read and approved the final manuscript. All authors contributed to the article and approved the submitted version.
Funding
This work was supported by the Plan Nacional de I + D of the Ministerio de Economía y Competitividad (grant number BIO2016-80329P) and for the Consolidate Research group of the Generalitat de Catalunya (reference group 2017SGR170).
Conflict of Interest
The authors declare that the research was conducted in the absence of any commercial or financial relationships that could be construed as a potential conflict of interest.
Acknowledgments
We thank Maite Polo for her technical assistance and the Servicios Científico-Técnicos from University of Barcelona.
Supplementary Material
The Supplementary Material for this article can be found online at: https://www.frontiersin.org/articles/10.3389/fmicb.2020.595697/full#supplementary-material
References
Abu-Qarn, M., Eichler, J., and Sharon, N. (2008). Not just for Eukarya anymore: protein glycosylation in Bacteria and Archaea. Curr. Opin. Struct. Biol. 18, 544–550. doi: 10.1016/j.sbi.2008.06.010
Altarriba, A., Merino, S., Gavín, R., Canals, R., Rabaan, A., Shaw, J. G., et al. (2003). A polar flagellum operon (flg) of Aeromonas hydrophila contains genes required for lateral flagella expression. Microb. Pathog. 34, 249–259. doi: 10.1016/S0882-4010(03)00047-0
Altschul, S. F., Madden, T. L., Schäffer, A. A., Zhang, J., Zhang, Z., Miller, W., et al. (1997). Gapped BLAST and PSI-BLAST: a new generation of protein database search programs. Nucleic Acid Res. 25, 3389–3402. doi: 10.1093/nar/25.17.3389
Angiuoli, S. V., Matalka, M., Gussman, A., Galens, K., Vangala, M., Riley, D. R., et al. (2011). CloVR: a virtual machine for automated and portable sequence analysis from the desktop using cloud computing. BMC Bioinformatics 12:356. doi: 10.1186/1471-2105-12-356
Arora, S. K., Bangera, M., Lory, S., and Ramphal, R. (2001). A genomic island in Pseudomonas aeruginosa carries the determinants of flagellin glycosylation. PNAS. 98, 9342–9347. doi: 10.1073/pnas.161249198
Asakura, H., Churin, Y., Bauer, B., Boettcher, J. P., Bartfeld, S., Hashii, N., et al. (2010). Helicobacter pylori HP0518 affects flagellin glycosylation to alter bacterial motility. Mol. Microbiol. 78, 1130–1144. doi: 10.1111/j.1365-2958.2010.07393.x
Barton, G. M., and Medzhitov, R. (2003). Toll-like receptor signaling pathways. Science 300, 1524–1525. doi: 10.1126/science.1085536
Benz, I., and Schmidt, M. A. (2002). Never say never again: protein glycosylation in pathogenic bacteria. Mol. Microbiol. 45, 267–276. doi: 10.1046/j.1365-2958.2002.03030.x
Bomar, L., Stephens, W. Z., Nelson, M. C., Velle, K., Guillemin, K., and Graf, J. (2013). Draft Genome sequence of Aeromonas veronii Hm21, a symbiotic isolate from the medicinal leech digestive tract. Genome Announc. 1, e00800–e00813. doi: 10.1128/genomeA.00800-13
Breton, C., and Imberty, A. (1999). Structure-function studies of glycosyltransferases. Curr. Opin. Struct. Biol. 9, 563–571. doi: 10.1016/S0959-440X(99)00006-8
Broeker, N. K., and Barbirz, S. (2017). Not a barrier but a key: How bacteriophages exploit host's O-antigen as an essential receptor to initiate infection. Mol. Microbiol. 105, 353–357. doi: 10.1111/mmi.13729
Canals, R., Ramirez, S., Vilches, S., Horsburgh, G., Shaw, J. G., Tomás, J. M., et al. (2006). Polar flagellum biogenesis in Aeromonas hydrophila. J. Bacteriol. 188, 542–555. doi: 10.1128/JB.188.2.542-555.2006
Canals, R., Vilches, S., Wilhelms, M., Shaw, J. G., Merino, S., and Tomás, J. M. (2007). Non-structural flagella genes affecting both polar and lateral flagella-mediated motility in Aeromonas hydrophila. Microbiology 153, 1165–1175. doi: 10.1099/mic.0.2006/000687-0
De Maayer, P., and Cowan, D. A. (2016). Flashy flagella: flagellin modification is relatively common and highly versatile among the Enterobacteriaceae. BMC Genomics 17:377. doi: 10.1186/s12864-016-2735-x
Delcher, A. L, Bratke, K. A., Powers, E. C., and Salzberg, S. L. (2007). Identifying bacterial genes and endosymbiont DNA with Glimmer. Bioinformatics. 23, 673–679. doi: 10.1093/bioinformatics/btm009
Forn-Cuní, G., Tomás, J. M., and Merino, S. (2016a). Whole-genome sequence of Aeromonas hydrophila strain AH-1 (Serotype O11). Genome Announc. 4:e00920-16. doi: 10.1128/genomeA.00920-16
Forn-Cuní, G., Tomás, J. M., and Merino, S. (2016b). Genome sequence of Aeromonas hydrophila strain AH-3 (Serotype O34). Genome Announc. 4:e00919-16. doi: 10.1128/genomeA.00919-16
Fulton, K. M., Mendoza-Barberá, E., Twine, S. M., Tomás, J. M., and Merino, S. (2015). Polar glycosylated and lateral non-glycosylated flagella from Aeromonas hydrophila strain AH-1 (Serotype O11). Int. J. Mol. Sci. 16, 28255–28269. doi: 10.3390/ijms161226097
Fulton, K. M., Smith, J. C., and Twine, S. M. (2016). Clinical applications of bacterial glycoproteins. Expert Rev. Proteomics. 13, 345–353. doi: 10.1586/14789450.2016.1166054
Gavín, R., Rabaan, A. A., Merino, S., Tomás, J. M., Gryllos, I., and Shaw, J. G. (2002). Lateral flagella of Aeromonas species are essential for epithelial cell adherence and biofilm formation. Mol. Microbiol. 43, 383–397. doi: 10.1046/j.1365-2958.2002.02750.x
Goon, S., Kelly, J. F., Logan, S. M., Ewing, C. P., and Guerry, P. (2003). Pseudaminic acid, the major modification on Campylobacter flagellin, is synthesized via the Cj1293 gene. Mol. Microbiol. 50, 659–671. doi: 10.1046/j.1365-2958.2003.03725.x
Guzman, L. M., Belin, D., Carson, M. J., and Beckwith, J. (1995). Tight regulation, modulation, and high-level expression by vectors containing the arabinose PBAD promoter. J. Bacteriol. 177, 4121–4130. doi: 10.1128/JB.177.14.4121-4130.1995
Hanahan, D. (1983). Studies on transformation of Escherichia coli with plasmids. J. Mol. Biol. 166, 557–580. doi: 10.1016/S0022-2836(83)80284-8
Igbinosa, I. H., Igumbor, E. U., Aghdasi, F., Tom, M., and Okoh, A. I. (2012). Emerging Aeromonas species infections and their significance in public health. Sci. World J. 2012:625023. doi: 10.1100/2012/625023
Janda, J. M., and Abbott, S. L. (2010). The genus Aeromonas: taxonomy, pathogenicity, and infection. Clin. Microbiol. Rev. 23, 35–73. doi: 10.1128/CMR.00039-09
Karlyshev, A. V., Linton, D., Gregson, N. A., and Wren, B. W. (2002). A novel paralogous gene family involved in phase-variable flagella-mediated motility in Campylobacter jejuni. Microbiology 48. 473–480. doi: 10.1099/00221287-148-2-473
Kelley, L. A., Mezulis, S., Yates, C. M., Wass, M. N., and Sternberg, M. J. (2015). The Phyre2 web portal for protein modeling, prediction and analysis. Nat. Protoc. 10, 845–858. doi: 10.1038/nprot.2015.053
Krogh, A., Larsson, B., von Heijne, G., and Sonnhammer, E. L. (2001). Predicting transmembrane protein topology with a hidden Markov model: application to complete genomes. J. Mol. Biol. 305, 567–580. doi: 10.1006/jmbi.2000.4315
Letunic, I., Doerks, T., and Bork, P. (2014). SMART: recent updates, new developments and status in 2015. Nucleic Acids Res. 43, D257–D260. doi: 10.1093/nar/gku949
Li, W., Cowley, A., Uludag, M., Gur, T., McWilliam, H., Squizzato, S., et al. (2015). The EMBL-EBI bioinformatics web and programmatic tools framework. Nucleic Acids Res. 43, W580–W584. doi: 10.1093/nar/gkv279
Marchler-Bauer, A., Derbyshire, M. K., Gonzales, N. R., Lu, S., Chitsaz, F., Geer, L. Y., et al. (2014). CDD: NCBI's conserved domain database. Nucleic Acids Res. 43, D222–D226. doi: 10.1093/nar/gku1221
Ménard, R., Schoenhofen, I. C., Tao, L., Aubry, A., Bouchard, P., Reid, C. W., et al. (2014). Small-molecule inhibitors of the pseudaminic acid biosynthetic pathway: targeting motility as a key bacterial virulence factor. Antimicrob. Agents Chemother. 58, 7430–7440. doi: 10.1128/AAC.03858-14
Merino, S., Rubires, X., Aguilar, A., and Tomás, J. M. (1999). The role of flagella and motility in the adherence and invasion to fish cell lines by Aeromonas hydrophila serogroup O:34 strains. FEMS Microbiol. Lett. 151, 213–217. doi: 10.1111/j.1574-6968.1997.tb12572.x
Merino, S., and Tomás, J. M. (2014). Gram-negative flagella glycosylation. Int. J. Mol. Sci. 15, 2840–2857. doi: 10.3390/ijms15022840
Merino, S., and Tomás, J. M. (2016). The FlgT protein is involved in Aeromonas hydrophila polar flagella stability and not affects anchorage of lateral flagella. Front. Microbiol. 7:1150. doi: 10.3389/fmicb.2016.01150
Merino, S., Wilhelms, M., and Tomás, J. M. (2014). Role of Aeromonas hydrophila flagella glycosylation in adhesion to Hep-2 cells, biofilm formation and immune stimulation. Int. J. Mol. Sci. 15, 21935–21946. doi: 10.3390/ijms151221935
Milton, D. L., O'Toole, R., Horstedt, P., and Wolf-Watz, H. (1996). Flagellin A is essential for the virulence of Vibrio anguillarum. J. Bacteriol. 178, 1310–1319. doi: 10.1128/JB.178.5.1310-1319.1996
Nielsen, H. (2017). Predicting secretory proteins with signalP. Methods Mol. Biol. 1611, 59–73. doi: 10.1007/978-1-4939-7015-5_6
Nothaft, H., and Szymanski, C. M. (2010). Protein glycosylation in bacteria: sweeter than ever. Nat. Rev. Microbiol. 8, 765–778. doi: 10.1038/nrmicro2383
Parker, J. L., Day-Williams, M. J., Tomás, J. M., Stafford, G. P., and Shaw, J. G. (2012). Identification of a putative glycosyltransferase responsible for the transfer of pseudaminic acid onto the polar flagellin of Aeromonas caviae Sch3N. Microbiologyopen 1, 149–160. doi: 10.1002/mbo3.19
Parker, J. L., Lowry, R. C., Couto, N. A., Wright, P. C., Stafford, G. P., and Shaw, J. G. (2014). Maf-dependent bacterial flagellin glycosylation occurs before chaperone binding and flagellar T3SS export. Mol. Microbiol. 92, 258–272. doi: 10.1111/mmi.12549
Parker, J. L., and Shaw, J. G. (2011). Aeromonas spp. clinical microbiology and disease. J. Infec. 62, 109–118. doi: 10.1016/j.jinf.2010.12.003
Persson, K., Ly, H. D., Dieckelmann, M., Wakarchuk, W. W., Withers, S. G., and Strynadka, N. C. J. (2001). Crystal structure of the retaining galactosyltransferase LgtC from Neisseria meningitidis in complex with donor and acceptor sugar analogs. Nat. Struct. Biol. 8, 166–175. doi: 10.2210/pdb1ga8/pdb
Rabaan, A. A., Gryllos, I., Tomás, J. M., and Shaw, J. G. (2001). Motility and the polar flagellum are required for Aeromonas caviae adherence to Hep-2 cells. Infect. Immun. 69, 4257–4267. doi: 10.1128/IAI.69.7.4257-4267.2001
Riley, D. R., Angiuoli, S. V., Crabtree, J., Dunning Hotopp, J. C., and Tettelin, H. (2012). Using Sybil for interactive comparative genomics of microbes on the web. Bioinformatics 28, 160–166. doi: 10.1093/bioinformatics/btr652
Ringenberg, M. A., Steenbergen, S. M., and Vimr, E. R. (2003). The first committed step in the biosynthesis of sialic acid by Escherichia coli K1 does not involve a phosphorylated N-acetylmannosamine intermediate. Mol. Microbiol. 50, 961–975. doi: 10.1046/j.1365-2958.2003.03741.x
Rubires, X., Saigi, F., Piqué, N., Climent, N., Merino, S., Albertí, S., et al. (1997). A gene (wbbL) from Serratia marcescens N28b (O4) complements the rfb-50 mutation of Escherichia coli K-12 derivatives. J. Bacteriol. 179, 7581–7586. doi: 10.1128/JB.179.23.7581-7586.1997
Sambrook, J., Fritsch, E. F., and Maniatis, T. (1989). Molecular Cloning: A Laboratory Manual. 2nd ed. New York, NY: Cold Spring Harbor Laboratory, Cold Spring Harbor.
Sanger, F., Nicklen, S., and Coulson, A. R. (1977). DNA sequencing with chain-terminating inhibitors. Proc. Natl. Acad. Sci. U.S.A. 74, 5463–5467. doi: 10.1073/pnas.74.12.5463
Schäffer, C., and Messner, P. (2017). Emerging facets of prokaryotic glycosylation. FEMS Microbiol. Rev. 41, 49–91. doi: 10.1093/femsre/fuw036
Schirm, M., Kalmokoff, M., Aubry, A., Thibault, P., Sandoz, M., and Logan, S. M. (2004). Flagellin from Listeria monocytogenes is glycosylated with beta-O-linked N-acetylglucosamine. J. Bacteriol. 186, 6721–6727. doi: 10.1128/JB.186.20.6721-6727.2004
Seshadri, R., Joseph, S. W., Chopra, A. K., Sha, J., Shaw, J. G., Graf, J., et al. (2006). Genome Sequence of Aeromonas hydrophila ATCC 7966T: jack of all trades. J. Bacteriol. 188, 8272–8282. doi: 10.1128/JB.00621-06
Sievers, F., Wilm, A., Dineen, D., Gibson, T. J., Karplus, K., Li, W., et al. (2011). Fast, scalable generation of high-quality protein multiple sequence alignments using Clustal Omega. Mol. Syst. Biol. 7:539. doi: 10.1038/msb.2011.75
Szymanski, C. M., Logan, S. M., Linton, D., and Wren, B. W. (2003). Campylobacter- a tale of two protein glycosylation systems. Trends Microbiol. 11, 233–238. doi: 10.1016/S0966-842X(03)00079-9
Szymanski, C. M., and Wren, B. W. (2005). Protein glycosylation in bacterial mucosal pathogens. Nat. Rev. Microbiol. 3, 225–237. doi: 10.1038/nrmicro1100
Tabei, S. M., Hitchen, P. G., Day-Williams, M. J., Merino, S., Vart, R., Pang, P. C., et al. (2009). An Aeromonas caviae genomic island is required for both O-antigen lipopolysaccharide biosynthesis and flagellin glycosylation. J. Bacteriol. 191, 2851–2863. doi: 10.1128/JB.01406-08
Tan, F. Y. Y., Tang, C. M., and Exley, R. M. (2015). Sugar coating: bacterial protein glycosylation and host-microbe interactions. Trends Biochem. Sci. 40, 342–350. doi: 10.1016/j.tibs.2015.03.016
Tomás, J. M. (2012). The main Aeromonas pathogenic factors. ISRN Microbiol. 2012:256261. doi: 10.5402/2012/256261
Twine, S. M., Paul, C. J., Vinogradov, E., McNally, D. J., Brisson, J. R., Mullen, J. A., et al. (2008). Flagellar glycosylation in Clostridium botulinum. FEBS J. 275, 4428–4444. doi: 10.1111/j.1742-4658.2008.06589.x
Twine, S. M., Reid, C. W., Aubry, A., McMullin, D. R., Fulton, K. M., Austin, J., et al. (2009). Motility and flagellar glycosylation in Clostridium difficile. J. Bacteriol. 191, 7050–7062. doi: 10.1128/JB.00861-09
Wass, M. N., Kelley, L. A., and Sternberg, M. J. E. (2010). 3DLigandSite: predicting ligand-binding sites using similar structures. Nucleic Acids Res. 38, W469–W473. doi: 10.1093/nar/gkq406
Wilhelms, M., Fulton, K. M., Twine, S. M., Tomás, J. M., and Merino, S. (2012). Differential glycosylation of polar and lateral flagellins in Aeromonas hydrophila AH-3. J. Biol. Chem. 287, 27851–27862. doi: 10.1074/jbc.M112.376525
Wilhelms, M., Molero, R., Shaw, J. G., Tomás, J. M., and Merino, S. (2011). Transcriptional hierarchy of Aeromonas hydrophila polar-flagellum genes. J. Bacteriol. 193, 5179–5990. doi: 10.1128/JB.05355-11
Keywords: Aeromonas, polar flagellum, motility, glycosylation island, glycosyltransferases
Citation: Forn-Cuní G, Fulton KM, Smith JC, Twine SM, Mendoza-Barberà E, Tomás JM and Merino S (2021) Polar Flagella Glycosylation in Aeromonas: Genomic Characterization and Involvement of a Specific Glycosyltransferase (Fgi-1) in Heterogeneous Flagella Glycosylation. Front. Microbiol. 11:595697. doi: 10.3389/fmicb.2020.595697
Received: 21 September 2020; Accepted: 21 December 2020;
Published: 18 January 2021.
Edited by:
Zhaomin Yang, Virginia Tech, United StatesReviewed by:
Wenlong Cai, University of California, Berkeley, United StatesGraciela Castro Escarpulli, Instituto Politécnico Nacional de México (IPN), Mexico
Copyright © 2021 Forn-Cuní, Fulton, Smith, Twine, Mendoza-Barberà, Tomás and Merino. This is an open-access article distributed under the terms of the Creative Commons Attribution License (CC BY). The use, distribution or reproduction in other forums is permitted, provided the original author(s) and the copyright owner(s) are credited and that the original publication in this journal is cited, in accordance with accepted academic practice. No use, distribution or reproduction is permitted which does not comply with these terms.
*Correspondence: Susana Merino, c21lcmlubyYjeDAwMDQwO3ViLmVkdQ==