- 1Institut de Recherche Biomédicale des Armées, Département de Biologie des Agents Transmissibles, Unité de Bactériologie/UMR_MD1, Brétigny-sur-Orge, France
- 2Bacteriology Division, U.S. Army Medical Research Institute of Infectious Diseases (USAMRIID), Frederick, MD, United States
- 3Center for Genome Sciences, U.S. Army Medical Research Institute of Infectious Diseases (USAMRIID), Frederick, MD, United States
Francisella tularensis, the causative agent of tularemia, is capable of causing disease in a multitude of mammals and remains a formidable human pathogen due to a high morbidity, low infectious dose, lack of a FDA approved vaccine, and ease of aerosolization. For these reasons, there is concern over the use of F. tularensis as a biological weapon, and, therefore, it has been classified as a Tier 1 select agent. Fluoroquinolones and aminoglycosides often serve as the first line of defense for treatment of tularemia. However, high levels of resistance to these antibiotics has been observed in gram-negative bacteria in recent years, and naturally derived resistant Francisella strains have been described in the literature. The acquisition of antibiotic resistance, either natural or engineered, presents a challenge for the development of medical countermeasures. In this study, we generated a surrogate panel of antibiotic resistant F. novicida and Live Vaccine Strain (LVS) by selection in the presence of antibiotics and characterized their growth, biofilm capacity, and fitness. These experiments were carried out in an effort to (1) assess the fitness of resistant strains; and (2) identify new targets to investigate for the development of vaccines or therapeutics. All strains exhibited a high level of resistance to either ciprofloxacin or streptomycin, a fluoroquinolone and aminoglycoside, respectively. Whole genome sequencing of this panel revealed both on-pathway and off-pathway mutations, with more mutations arising in LVS. For F. novicida, we observed decreased biofilm formation for all ciprofloxacin resistant strains compared to wild-type, while streptomycin resistant isolates were unaffected in biofilm capacity. The fitness of representative antibiotic resistant strains was assessed in vitro in murine macrophage-like cell lines, and also in vivo in a murine model of pneumonic infection. These experiments revealed that mutations obtained by these methods led to nearly all ciprofloxacin resistant Francisella strains tested being completely attenuated while mild attenuation was observed in streptomycin resistant strains. This study is one of the few to examine the link between acquired antibiotic resistance and fitness in Francisella spp., as well as enable the discovery of new targets for medical countermeasure development.
Introduction
The facultative intracellular gram-negative bacterium Francisella tularensis, found ubiquitously across the northern hemisphere, is responsible for the zoonotic disease known as tularemia or more commonly “rabbit fever.” The disease is typically spread between hosts by arthropod vectors, namely through the bite of an infected mosquito or tick (Ellis et al., 2002). F. tularensis has a broad host range, as infections have been noted in an array of vertebrates including amphibians, birds, and mammals, though preferential colonization has been observed in lagomorphs in North America, mainly hares, and rabbits (Jellison and Parker, 1945; Hopla, 1974; Morner, 1992). The ulceroglandular form of tularemia is the most common, resulting in a primary ulcer at the inoculation site, followed by regional lymphadenopathy. Less common disease outcomes, depending on the route of exposure, include ocular, oropharyngeal, typhoidal, and pneumonic forms, with pneumonic tularemia having higher mortality rates (Hepburn and Simpson, 2008).
F. tularensis is comprised of two clinically important subspecies, subsp. tularensis (Type A) and subsp. holarctica (Type B), which are responsible for the majority of tularemia infections in humans. Type A strains are prevalent in North America and highly virulent, while Type B strains are present more widely across the Northern hemisphere and are typically less virulent. A third closely related subspecies, Francisella novicida, is only associated with brackish water and soil, and rarely causes disease in humans. F. novicida is commonly used as a laboratory surrogate since it has a high degree of genetic similarity to the virulent F. tularensis subspecies, is able to infect macrophages in vitro and cause disease in mice, and can be handled under BSL-2 conditions (Kingry and Petersen, 2014).
F. tularensis has garnered significant attention in recent years due to its concern as a potential biothreat agent. It has a low infectious dose, reported to be as low as a single bacterium, and is easy to obtain from the environment and aerosolize (Jones et al., 2005). Moreover, there is no United States (U.S.) Food and Drug Administration (FDA)-approved vaccine available, and the disease has an estimated mortality rate of 30–60% if left untreated (Pechous et al., 2009). Historically, bioweapons containing F. tularensis were developed during WWII by multiple biological weapons programs in the U.S., Japan, and the Soviet Union (Barras and Greub, 2014). A live vaccine strain (LVS), derived from F. holarctica, was developed by the Soviet Union and gifted to the U.S. and is also used as a surrogate strain. Currently, LVS is only available as an investigational vaccine to at-risk laboratory personnel (Oyston and Quarry, 2005). Moreover, its exact basis for loss of virulence is unknown, although probable genetic alterations have been identified (Rohmer et al., 2006), and concerns about its reversion to virulence and breakthrough in protection has prevented licensure by the FDA (Eigelsbach and Downs, 1961; Saslaw et al., 1961; Hornick and Eigelsbach, 1966). Complicating this threat, F. tularensis has the potential to acquire antibiotic resistance, either through natural selection or intentionally engineered, rendering current treatments ineffective (Loveless et al., 2010; Caspar et al., 2017; Chance et al., 2017; Heine et al., 2017). Current clinical, vector, and zoological surveillance efforts suggest emergence of resistance to clinically relevant antibiotics for F. tularensis strains is not typical or detected to date (Müller et al., 2013; Tomaso et al., 2017; Caspar et al., 2018). However, significant efforts are still required to i) characterize the fitness of such antibiotic resistant strains, and ii) identify novel targets for the development of vaccines and therapeutics to combat this threat.
The aminoglycosides, streptomycin and gentamicin, have been utilized historically to treat tularemia; however, issues with toxicity has kept their use mainly restricted to severe cases, while fluoroquinolones and tetracyclines are considered a first-line of defense against these infections (Hepburn and Simpson, 2008). Specifically, oral treatment with ciprofloxacin or doxycycline for at least 14 days is recommended in the event of a large-scale exposure for post-exposure prophylaxis (Dennis et al., 2001). However, relapse and treatment failure occurs in 10–15% of tularemia patients given fluoroquinolones (Johansson et al., 2001; Perez-Castrillon et al., 2001). Previous work by Sutera et al. (2014), showed that passaging of LVS and F. novicida on increasing concentrations of ciprofloxacin led to mutations within the topoisomerase and gyrase genes, the targets of ciprofloxacin, as well as cross-resistance to the related fluoroquinolones levofloxacin and moxifloxacin (Sutera et al., 2014). Most of the mutations were found within the QRDR (quinolone resistance-determining region) of gyrA/B and parC/E, encoding subunits of gyrase and topoisomerase enzymes, respectively. Similarly, a study focused on rapid detection of mutations leading to ciprofloxacin resistance, found that passaging of the fully virulent F. tularensis Schu S4 strain on ciprofloxacin gave rise to mutations in the QRDR of gyrA and parE genes (Loveless et al., 2010). Indeed, the presence of mutations in the QRDR in response to ciprofloxacin resistance is well established across diverse bacteria (Yoshida et al., 1990; Jacoby, 2005; Maruri et al., 2012). Streptomycin, typically used in severe cases of tularemia, is an aminoglycoside that inhibits bacterial protein synthesis by binding to the S12 protein of the 30S ribosomal subunit (Luzzatto et al., 1968; Parker, 2001). Like ciprofloxacin, resistance to streptomycin can occur via mutations in the rpsL allele encoding the ribosomal target, thereby altering the binding affinity of the drug.
The formation of a biofilm by bacterial cells is a well-known contributing factor to virulence and antibiotic resistance in many pathogenic species, often leading to recalcitrance and, subsequently, reoccurring infections (Costerton et al., 1999; Hall and Mah, 2017). Analysis of biofilm formation in recent years has revealed that F. novicida readily forms a robust biofilm on a variety of surfaces while the human pathogenic F. tularensis type A and B strains form either a weak or unsubstantial biofilm in vitro (Margolis et al., 2010; Mahajan et al., 2011; Champion et al., 2019). However, the importance of biofilm formation on virulence and in the treatment of tularemia is not well understood given the intracellular lifestyle of this pathogen. Previous work has shown F. novicida biofilms are resistant to antibiotics and that the enzymatic removal of the biofilm matrix is capable of re-sensitizing the cells embedded within the biofilm to antibiotics (Chung et al., 2014). In LVS, variation in surface polysaccharide and glycosylation has been shown to inhibit biofilm formation in wild-type cells, however, phase variation during extended incubations may remodel the cell surface to promote biofilm formation (Champion et al., 2019). While these studies have focused on the effects of biofilms on antibiotic treatment, little is currently known about how intrinsic antibiotic resistance affects biofilm formation in Francisella.
The emergence of multi-drug resistant bacteria has renewed the interest and urgency of understanding how the acquisition of antibiotic resistance affects virulence and alters the threat of pathogens, especially for bacterial biothreats. In this study, we selected for an antibiotic resistant panel of Francisella surrogates (F. novicida and LVS) in an effort to understand how resistance to ciprofloxacin and streptomycin affects Francisella fitness, which could help identify new druggable targets for medical countermeasures. Through step-wise serial passaging in the presence of these antibiotics, we show that high-level resistance to ciprofloxacin and streptomycin leads to a number of both on- and off-pathway mutations. On-pathway mutations were identified in the gyrase/topoisomerase genes and ribosomal genes, the targets of ciprofloxacin and streptomycin, respectively, as well as in genes encoding drug efflux proteins. Interestingly, many off-pathway mutations occurred in known or putative virulence factors, such as LPS and peptidoglycan biosynthesis genes. We also determined that ciprofloxacin resistant F. novicida strains were significantly impaired for biofilm formation compared to the parent strain, while streptomycin resistant F. novicida were not affected. Moreover, several ciprofloxacin resistant strains were reduced for replication in macrophages and exhibited significantly decreased virulence during intranasal infection of mice. In contrast, only mild attenuation was observed in several streptomycin resistant isolates. Here, we provide a thorough characterization of the growth, biofilm capacity, and fitness of naturally derived antibiotic resistant variants of F. novicida and LVS. These studies add to the growing body of knowledge on the relationship between resistance, biofilm, and virulence in bacterial species. More specifically, the mutations identified in these studies derived by serial passaging reveal genes that are involved in virulence and represent new targets to be explored for medical countermeasures to treat tularemia.
Materials and Methods
Bacterial Strains and Culture Conditions
Bacterial strains used in this study are listed in Table 1. F. novicida and LVS were routinely cultured on enriched chocolate agar plates (Thermo Fisher Scientific, Waltham, MA) at 37°C. For liquid culture, either brain heart infusion broth (BHI) supplemented with 1% IsoVitaleX (Becton-Dickinson, Cockeysville, MD) or Chamberlain’s Defined Medium (CDM) (Chamberlain, 1965).
Natural Selection of Antibiotic Resistant Non-select Agent Francisella Strains
The surrogates F. novicida U112 (Larson et al., 1955) and LVS (Saslaw et al., 1961) were obtained from the USAMRIID bacterial repository and used as the parental strains to derive either ciprofloxacin or streptomycin resistant isolates. Ciprofloxacin resistant derivative strains were obtained by sequentially passaging with the sub-inhibitory concentrations of 0.0125, 0.025, 0.05, 0.1, 0.2, 0.5, 1, 2, 4, 8, 16, 32, 64, 72, to 80 mg/L for F. novicida or 0.0125, 0.025, 0.05, 0.1, 0.2, 0.5, 1, 2, 3, 4, 8, 16, 32, 40, 48, 56, 64, 72, 80, 96, 104, to 128, mg/L for LVS. Streptomycin resistant derivative strains were obtained by sequentially passaging with the sub-inhibitory concentrations of 0.5, 1, 2, 4, 8, 16, 32, 64, 128, 256, to 512 mg/L for F. novicida and LVS. In all cases, the cultures were passaged by alternating between chocolate agar and supplemented BHI broth at 37°C. To confirm resistance stability the isolates were cultured in the absence of antibiotic for 10 passages and alternating agar and broth cultures at 37°C.
Generation of Growth Curves
Francisella strains were cultured for 24 h on chocolate agar and resuspended to an OD600 of 0.3 in phosphate buffered saline (PBS). Bacterial suspensions were then diluted 1–10 into either BHI + 1% IsoVitaleX or CDM in a 96-well plate. Growth assays were performed using an Infinite M200 Pro (Tecan Systems, San Jose, CA) microplate reader at 37°C with orbital shaking. All samples were performed in triplicate including medium controls to ensure sterility throughout the experiment as well as to calculate the absorbance of the cultures.
Minimum Inhibitory Concentration (MIC) Susceptibility Assays
LVS and F. novicida strains were pre-cultured for 4–6 h in BHI broth supplemented with 1% IsovitaleX, followed by plating onto chocolate agar and application of the E-test strip (bioMérieux, Inc., Durham, NC). E-tests were recorded after 24 h for F. novicida and 48 h for LVS. For each experiment, three separate E-tests were used, and the experiment was repeated three times.
Genome Sequencing and Analysis
Bacterial genomic DNA was sheared to 400 bp using Covaris LE220 Focused-ultrasonicator (Covaris Inc., Woburn, MA). End repair, A-tailing and ligation of barcoded Illumina adapters were performed on the Apollo 324 automated system, using Prep X Complete ILMN DNA Library Kit (WaferGen Biosystems, Fremont, CA). Libraries were enriched using KAPA Library Amplification Kit (KAPA Biosystems, Wilmington, MA) with 10 cycles of PCR. Libraries were then quantified by TapeStation (Agilent, Santa Clara, CA), normalized and pooled for sequencing. Final pool was quantified by qPCR using KAPA Library Quantification Kit (KAPA Biosystems, Wilmington, MA). Cluster amplification was performed on the cBot with the TruSeq PE Cluster Kit v3-cBot-HS (Illumina Inc., San Diego, CA). Clustered flow cell was sequenced on the HiSeq 2500 instrument, resulting in 2 × 100 bp reads.
Adapters were removed using Cutadapt v1.9dev1 (Martin, 2011), and reads were quality trimmed and filtered with Prinseq v0.20.3 (Schmieder and Edwards, 2011). Reads less than 70 bp in length after trimming and singletons were removed. Reads were aligned to either Francisella tularensis subsp novicida U112 (NZ_CP009633.1) or F. tularensis subsp holarctica LVS (NZ_CP009694.1) using Bowtie2 v2.1.0 (Langmead and Salzberg, 2012). For variant calling, we only utilized reads mapped in proper pairs and with mapping quality ≥ 20. Duplicate reads were removed with Picard v1.131 (”Picard Toolkit” 2019; GitHub Repository: Broad Institute) Variants were called using UnifiedGenotyper from GATK v3.5-0-g36282e4 with ploidy set to 1 and genotype likelihoods model set to BOTH (McKenna et al., 2010). Variant effects were annotated using SnpEff v4.2 (Cingolani et al., 2012). Repetitive or low-complexity regions of the genome were identified using custom scripts1 and variants called in those regions were filtered. Finally, mutations were confirmed by PCR analysis and Sanger sequencing. Data was deposited into the NCBI database under BioProject ID PRJNA645905.
Static Biofilm Assay
Bacterial suspensions in PBS were prepared as described above and diluted 1:10 into BHI medium + 1% IsoVitaleX in a 96-well plate. The plates were then incubated statically at 37°C for either 1, 3, 5, or 7 days. Peripheral wells were filled with sterile medium to minimize evaporation over the duration of the experiment. Before staining, the OD600 of each well was read to note cell density after which planktonic cells were removed by aspiration and the remaining biofilm was washed 3 times with PBS. When enzymes were used, the biofilm was washed twice with PBS and enzyme (DNase I, proteinase K, or sodium-meta-periodate; Millipore-Sigma, St. Louis, MO) was added at 250 μg/mL in 200 μL of PBS, incubated for 15′ at room temperature, and washed again twice with PBS. In all experiments, the biofilm was fixed for 15′ at room temperature in 100% ethanol. Biofilm was visualized by staining for 15′ with 0.1% crystal violet stain (Sigma-Aldrich, St. Louis, MO) dissolved in H2O (w/v) followed 3 PBS washes to remove excess stain. The remaining crystal violet was solubilized in 33% acetic acid (v/v) and the OD600 was obtained. In some instances, FM1-43 stain was used to fluorescently label cells within the biofilm. Where indicated, biofilms were stained for 30′ at room temperature using a final concentration of 100 μg/mL FM1-43 after which excess stain was removed by rinsing 3x with water. Fluorescent signal was then recorded using an Infinite M200 Pro (Tecan Systems, San Jose, CA) microplate reader at 472 nm ex/580 nm em set at a gain of 100. When necessary, samples were diluted to ensure OD600 or fluorescent readings were within the linear range of the instrument.
Macrophage Assays
Murine macrophage-like J774A.1 cells (American Type Culture Collection, Manassas, VA) were maintained in Dulbecco’s Modified Eagle’s medium (DMEM) containing 4.5 g/L glucose, L-glutamine and sodium pyruvate supplemented with 10% fetal bovine serum (FBS). Cells were seeded at approximately 2.5 × 105 into 24-well plates and cultured for 24 h at 37°C under 5% CO2 to allow a confluent monolayer to form. Prior to the experiment, F. novicida was resuspended from 18 h plates to an OD600 of ∼0.4 in PBS and diluted 1–5 in pre-warmed tissue culture medium to serve as a bacterial inoculum. Next, tissue culture medium was aspirated from the J774A.1 monolayer, replaced with 200 μL of inoculum (MOI of ∼100:1 confirmed by CFU determination) and incubated for 2 h. After incubation, extracellular bacteria were aspirated, wells were washed 3x with PBS and tissue culture medium containing 25 μg/mL gentamicin was used to replenish each well. At 4 and 24 h, the monolayer of J774A.1 cells was washed 3x with PBS and lysed with 200 μL of sterile water followed by scraping. Cells harvested were immediately suspended in 800 μL of PBS. This suspension was serial diluted and plated on chocolate agar to determine CFU recovery at each time point. For assays with LVS, a 96 well plate format was adapted following the methods described above with minor modifications. J774A.1 cells were seeded at approximately 8 × 104 cells per well in 80 μL. Bacteria were re-suspended for 18 h plates to an OD600 of ∼0.4 directly into tissue culture medium, diluted 1:5 into fresh tissue culture medium and 20 μL was added to each well as an inoculum. After 2 h of incubation, 10 mL of fresh tissue culture medium containing gentamicin was added directly to each well without washing at a final concentration of 25 μg/mL. At 4 and 24 h time points, J774A.1 cells were harvested as described above with the exception that 20 μL was used for lysis and immediately re-suspended into 80 μL of PBS. For both F. novicida and LVS, the average of three replicate wells was used to determined percent recovery in each experiment. CFU counts were averaged from two plates per well. Results shown were obtained from at least three independent experiments.
Animal Challenges
Six to eight weeks old female BALB/c mice (obtained from Charles River Laboratories, Frederick, MD) were challenged with LVS or F. novicida strains via the intranasal route. Strains were streaked onto chocolate agar and incubated for at 37°C for 2 days (LVS) or 24 h (F. novicida), after which the resulting growth was swabbed onto a fresh chocolate agar plate and incubated at 37°C for 24 h. Bacterial growth was resuspended in PBS and cell suspensions diluted to varying doses. Mice were anesthetized with 200 μl of ketamine, acepromazine, and xylazine injected intraperitoneally. These mice were then challenged by intranasal instillation with 50 μL of LVS or F. novicida suspended in PBS. Challenge doses were determined by serial dilution in PBS and plating on chocolate agar. Mice were monitored at least twice each day and mortality rates (or euthanasia when moribund) were recorded.
Ethics Statement
Challenged mice were observed at least twice daily for 21 days for clinical signs of illness. Humane endpoints were used during all studies, and mice were humanely euthanized when moribund according to an endpoint score sheet. Animals were scored on a scale of 0 ± 12: 0 ± 3 = no clinical signs; 4 ± 7 = clinical signs; increase monitoring; 8 ± 12 = distress; euthanize. Those animals receiving a score of 8 ± 12 were humanely euthanized by intraperitoneal injection with 200 μL pentobarbital sodium (Fatal Plus) followed by cervical dislocation. However, even with multiple checks per day, some animals died as a direct result of the infection. Animal research at The United States Army of Medical Research Institute of Infectious Diseases (USAMRIID) was conducted and approved under an Institutional Animal Care and Use Committee (USAMRIID IACUC) in compliance with the Animal Welfare Act, Public Health Service Policy, and other Federal statutes and regulations relating to animals and experiments involving animals. The facility where this research was conducted is accredited by the Association for Assessment and Accreditation of Laboratory Animal Care, International and adheres to principles stated in the Guide for the Care and Use of Laboratory Animals, National Research Council, 2011.
Statistics
All in vitro experiments described in this manuscript were performed independently at least 3 times. Intracellular replication data were analyzed by ANOVA with Dunnett’s post-hoc procedure on the ratio of 24–4 h CFU recovery time points, using SAS version 9.4. LD50 analysis was determined by the Bayesian probit analysis. Normality was assessed using a Shapiro-Wilk test.
Results
Generation of a Surrogate Panel of Antibiotic Resistant Francisella Strains
The acquisition of antibiotic resistance, either natural or engineered, presents a challenge for the development of medical countermeasures. As fluoroquinolones and aminoglycosides often serve as the first line of defense for treatment of tularemia, we sought to characterize a surrogate panel of antibiotic resistant Francisella strains in an effort to (1) assess the fitness of such strains; and (2) identify new targets to investigate for the development of vaccines or therapeutics. F. novicida U112 and LVS were selected as surrogates due to the relatedness to virulent forms of F. tularensis and the ability to work safely under BSL-2 laboratory conditions. To establish a panel of antibiotic resistant strains in these backgrounds, serial passaging in the presence of either ciprofloxacin or streptomycin was conducted in a step-wise manner by increasing the antibiotic concentration in each step (Figure 1A). A previous study compiling data from multiple antibiotic susceptibility studies encompassing > 800 F. tularensis strains, reported a range of 0.002–0.125 μg mL–1 for ciprofloxacin, with little variation between type A and type B isolates, while a range of 0.064–8 μg mL–1 was reported for streptomycin (Caspar and Maurin, 2017). We first tested the MIC values of WT F. novicida and LVS strains, then used sub-inhibitory concentrations of 1/4 of the WT MIC for the first selection step. 0.125 μg mL–1 ciprofloxacin was used to generate the ciprofloxacin-resistant (CipR) strain panel, and 0.5 μg mL–1 streptomycin was used to generate the streptomycin-resistant (StrepR) strain panel. Gradual increase of the antibiotic concentration should select for those genetic adaptations that would enable the cells to replicate in the presence of each respective antibiotic. Both F. novicida (Fn) and LVS had increased antibiotic resistance, although the number of passages required to obtain high levels of resistance were dependent on the antibiotic. Passaging of Fn and LVS on streptomycin quickly reached high MIC levels (>1024 μg mL–1), after 11 steps. In contrast, cultures in the presence of ciprofloxacin required an additional 3 steps and 6 steps for Fn and LVS, respectively, to achieve an MIC > 32 μg mL–1 (Figure 1B). To ensure resistance was stably maintained after selection, 10 CFU of the CipR and StrepR variants were sequentially passaged 10 times in alternating agar and broth cultures without the presence of the respective antibiotic and shown to retain resistance. No differences in growth in BHI/IsovitaleX liquid culture were observed for any of the CipR or StrepR strains tested compared to the parent (data not shown). It is from these antibiotic resistant strains that the surrogate panel was comprised for this study. Strains were designated as follows- Fn or LVS, Cip (ciprofloxacin-resistant) or Strep (streptomycin-resistant), followed by the concentration of antibiotic used in the final step of passaging and the colony number. For example, LVS Cip80-1 indicates LVS passaged on increasing ciprofloxacin to a final concentration of 80 μg mL–1, and the first independent colony isolated.
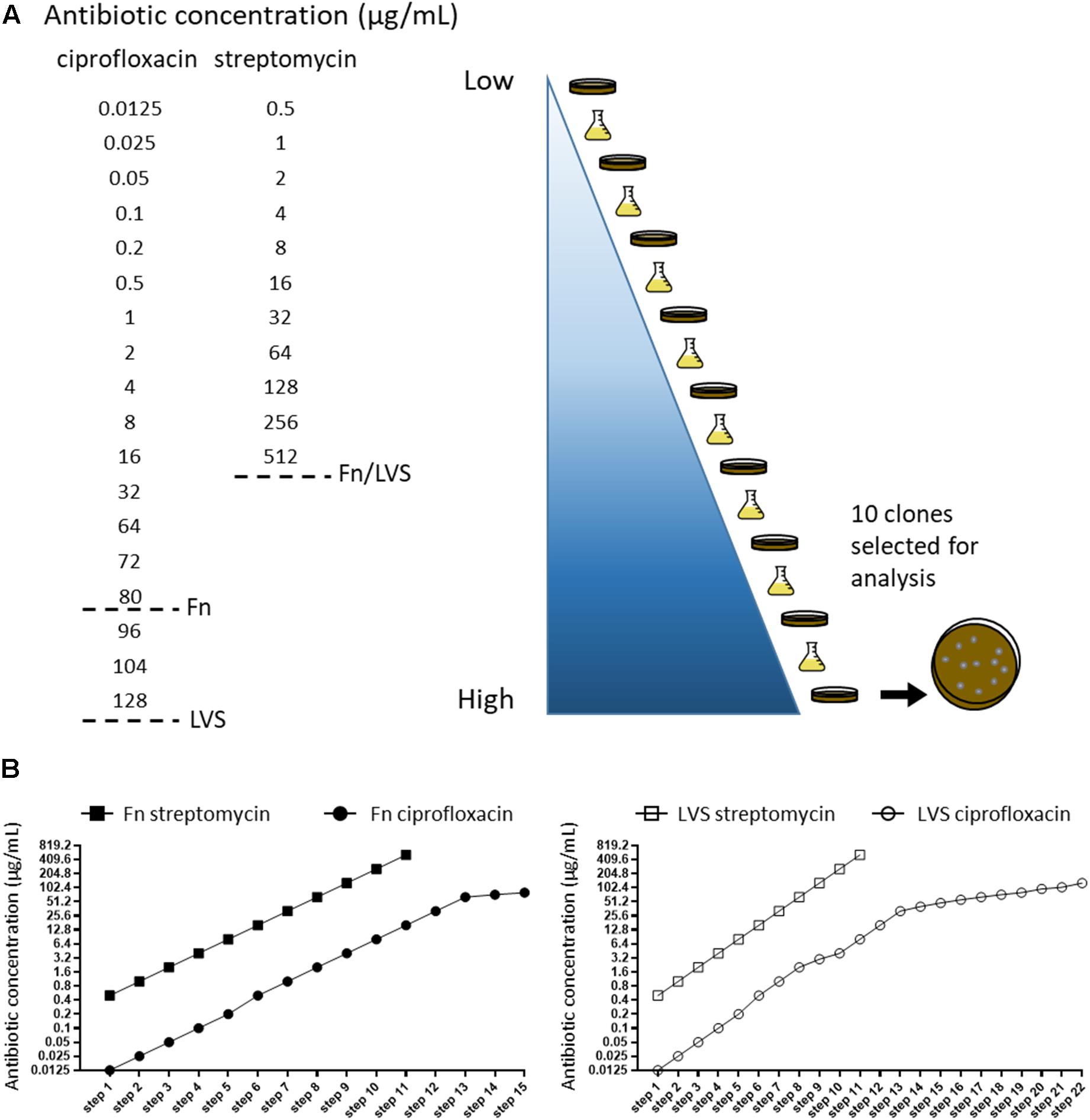
Figure 1. Construction of a surrogate panel of antibiotic resistant Francisella. (A) Schematic of experimental design. Serial passaging of LVS and Fn was performed on increasing concentrations of ciprofloxacin or streptomycin, alternating between broth and agar. Concentration gradients of each antibiotic are shown from low to high (light blue to dark blue), and dotted lines indicate endpoints for either Fn or LVS passaging. At each endpoint, 10 isolated colonies of either LVS or Fn CipR or StrepR were passaged 10 times without the presence of antibiotic to confirm resistance stability and sequenced. (B) Antibiotic concentrations used in each selection step of Fn (left graph) and LVS (right graph) during passaging with ciprofloxacin and streptomycin. Note that LVS required additional intermediate steps of selection on ciprofloxacin, resulting in the 22 selection steps shown.
MIC Determination of CipR and StrepR Resistant LVS and Fn Variants
CipR Variants
E-tests were carried out to assess final MIC levels of various antibiotics against each LVS or Fn variant. For ciprofloxacin, E-tests showed an MIC of 0.008 μg mL–1 for the parental Fn strain, and 0.006 μg mL–1 for the parental LVS strain. Fn CipR variants exhibited MIC levels of > 32 μg mL–1, the maximum concentration on the E-tests. In agreement, the LVS Cip128 clones had MIC levels > 32 μg mL–1 (Table 1). Additional fluoroquinolones including levofloxacin, ofloxacin, and gatifloxacin were also tested and showed similar patterns of increased resistance among CipR variants as compared to the parental strains (data not shown). CipR variants also exhibited increased resistance to the unrelated antibiotics doxycycline and chloramphenicol in both Fn and LVS (Table 1). Interestingly, all LVS CipR variants became more susceptible to polymyxin B, a membrane-destabilizing antibiotic, while the parent LVS remained resistant to polymyxin B (∼128-384 μg mL–1 compared to > 1024 μg mL–1 for parent LVS, Table 1). In contrast, the Fn CipR variants displayed slightly increased resistance to polymyxin B, 128 μg mL–1, compared to 96 μg mL–1 for WT.
StrepR Variants
E-tests for streptomycin showed MIC values of 2 and 8 μg mL–1 for parent LVS and Fn strains, respectively. In contrast, all LVS and Fn StrepR variants showed much higher levels of resistance to streptomycin, MIC > 1,024 μg mL–1, at least twofold greater than the concentration of streptomycin used for passaging. Unlike the CipR variants, the acquisition of StrepR did not grant cross protection to related antibiotics, including gentamicin, amikacin, tobramycin, and spectinomycin, as the sensitivities of these variants remained similar to the parental strains (data not shown). Additionally, sensitivity to polymyxin B was unaffected in both Fn and LVS StrepR variants compared to the parental strains (Table 1).
Genome Sequencing Reveals the Presence of Both On-Pathway and Off-Pathway Mutations in Surrogate Antibiotic Resistant Strains
To identify mutations acquired by Francisella during the development of antibiotic resistance, we performed whole genome sequencing on the surrogate strain panel. Sequencing revealed the presence of 49 unique mutations across 25 different genes throughout the surrogate panel, with 20 mutations harbored within CipR strains and 29 harbored within StrepR strains (Supplementary Table S1). Interestingly, the majority of mutations identified were within the LVS background, 41 mutations compared to 8 mutations in the Fn background (Figure 2A). The StrepR LVS isolates harbored about twice as many mutations as the CipR LVS isolates, 28 compared to 13. In contrast, the StrepR Fn isolates harbored only a single mutation, found within the 30S ribosomal protein S12, while CipR Fn isolates contained seven unique mutations (Figure 2A).
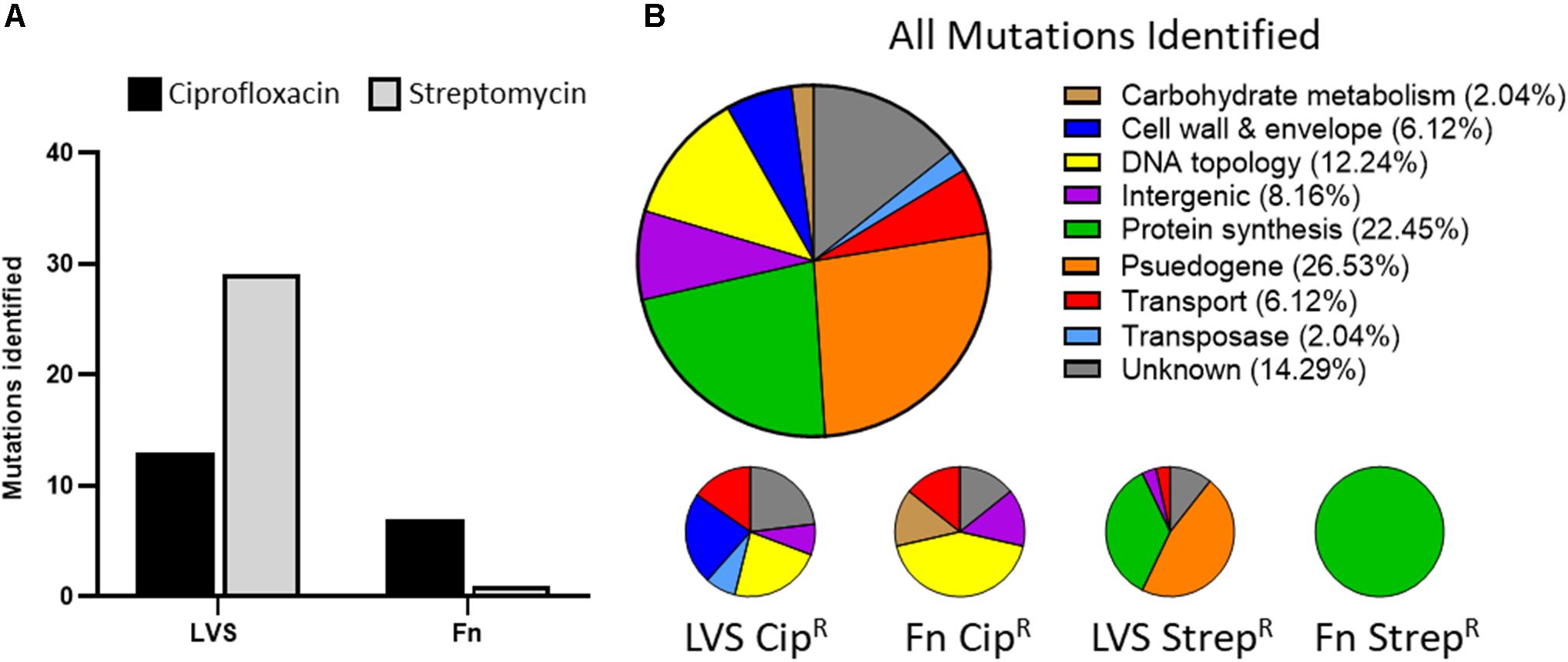
Figure 2. Characterization of mutations harbored within Francisella antibiotic resistant surrogates. (A) Number of mutations identified in CipR (black bars) and StrepR (gray bars) isolates of LVS and F. novicida. (B) Annotations of genes found to harbor mutations in all isolates (top pie chart) and LVS or Fn subsets (bottom pie charts) as determined by the prokka genome annotation tool. Percentages of each category are indicated in parentheses.
To ascribe functional classes to each of these mutations, we next performed Gene Ontology (GO)-term analysis which allowed the mutations and their respective genes to be grouped into nine categories. Figure 2B shows the proportion of mutations occurring in each functional category, with large percentage of mutations found in pseudogenes (∼27%), protein synthesis genes (∼22%), and genes of unknown function (∼14%). Other categories included proteins associated with cell wall and envelope, DNA topology, transport, and intergenic, each accounting for ∼6–12% of the total mutations. Lastly, carbohydrate metabolism and transposases represented ∼2% of the mutations identified. These categories are further broken down into four charts, representing the proportion of mutations found in each panel of strains (LVS CipR, Fn CipR, LVS StrepR, and Fn StrepR as shown in Figure 2B). The DNA topology, cell wall and envelope, and transposase mutations were exclusively contained in the CipR strains, while protein synthesis and pseudogene mutations were exclusively found in the StrepR strains. Notably, these categories can be further divided into on-pathway mutations, present in genes encoding proteins directly targeted by the antibiotic and off-pathway mutations, not known to be directly involved in antibiotic resistance. Supplementary Table S2 lists the patterns of mutations identified across all isolates within the CipR and StrepR panels.
On-Pathway Mutations (CipR)
Ciprofloxacin targets DNA replication through the inhibition of DNA gyrase and topoisomerase IV, thereby inhibiting cell division (LeBel, 1988). Consistent with on-pathway mutations, missense mutations were found in genes encoding each of these enzymes in both Fn and LVS CipR strains. All CipR variants of LVS and Fn harbored a single nucleotide polymorphism (SNP) in the gyrA allele, resulting in the amino acid substitution of threonine to isoleucine at position 83 of the protein (Table 2). The Fn CipR strains also contained a nearby SNP in gyrA, resulting in the amino acid change Asp87Tyr. The gyrB allele was only affected in LVS CipR strains, which had a Ser465Tyr mutation. Mutations were also identified in parC and parE genes, encoding topoisomerase IV subunits A and B, respectively. Unlike gyrA, which was mutated in both Fn and LVS, parC was only mutated in Fn CipR isolates (Gly81Asp), while parE was only mutated in LVS CipR isolates (Ser447Ile). As can be expected, repeated exposure to ciprofloxacin affected genes associated with antibiotic resistance, such as drug efflux pumps. These included an in-frame insertion in a gene encoding for a MexH family multidrug efflux RND transporter, a missense mutation in the gene encoding for an outer membrane efflux protein TolC resulting in Arg453Ser, and a Arg653His substitution in the gene encoding for the AcrB periplasmic accessory protein (Table 2). Interestingly, the gyrA/B and parC/E mutations were conserved across all colonies of Fn or LVS in which they were identified, while mutations outside of these genes were more scattered across the isolates.
Off-Pathway Mutations (CipR)
Mutations deemed off-pathway in CipR Fn strains were both frameshifts which likely resulted in the inactivation of the thiopurine S-methyltransferase (AW25_RS00910) and short-chain dehydrogenase/reductase (SDR) family oxidoreductase (AW25_RS02890) proteins (Table 3). Off-pathway mutations in CipR LVS isolates included a frameshift mutation in bamB, encoding a subunit of the beta-barrel assembly machinery, or BAM, complex. Additional mutations were found in genes involved in LPS biosynthesis and transport, wbtC (in-frame deletion) and lptE (frameshift), and peptidoglycan biosynthesis, slt (missense). A frameshift mutation was also identified in the gene encoding for DUF-3573 domain-containing protein FupA (AW21_RS06025). A single mutation was identified in the gene encoding for IS630 family transposase, and the remaining mutations were located in intergenic regions, classified as modifiers in this study, due to the potential for these mutations to modify the expression of downstream genes. In Fn, a cytosine to thymine SNP was identified 35 bp upstream of a IS5 family transposase. In LVS, a cytosine to adenine SNP occurred downstream of a Bcr/CflA family drug resistance efflux transporter, 52 bp upstream of a DUF3573 domain-containing membrane protein. Overall, the mutations found in the Fn CipR panel were present in every isolate, with the exception of a single nucleotide change in an intergenic region in the Cip80-10 strain (Supplementary Table S2). Similarly, the off-pathway mutations in the LVS CipR panel were conserved across all isolates except for those found in slt, lptE, and an I630 transposase, present in 3–4 isolates out of ten.
On-Pathway Mutations (StrepR):
Streptomycin inhibits translation by binding to the 30S ribosomal subunit halting protein synthesis. The 88th residue of this protein was previously found to be important for conferring resistance to streptomycin in Escherichia coli (Toivonen et al., 1999). Consistent with this finding, the LVS isolates harbored a missense mutation in rpsL (AW21_RS04995) resulting in a Lys88Arg substitution in the 30S ribosomal protein S12 (Table 2). Fn also had a mutated allele of rspL (AW25_RS08975) where a Lys43Arg substitution was identified. This mutation was the only one identified in all StrepR Fn strains sequenced. Conversely, the StrepR LVS isolates were some of the most diverse observed during the construction of the antibiotic resistant mutant panels (Table 2). Additional on-pathway mutations arose in the ribosomal RNA small subunit methyltransferase G gene (AW21_RS04075) of LVS StrepR strains. Interestingly, nine unique mutations were identified in this gene with only one being synonymous while the rest were missense mutations (Met4Leu, Leu31Pro, Gly79Val, Pro190Ser) or considered highly detrimental to a functional protein (two frameshifts; Asp6fs, Leu33fs and two stop codon introductions; Glu176∗, Glu196∗). Lastly, one missense mutation, resulting in Trp18Leu, was found in the gene encoding for the major facilitator superfamily (MFS) transporter (AW21_RS00170), a putative drug efflux protein.
Off-Pathway Mutations (StrepR LVS):
The StrepR Fn isolates in our panel only harbored a single mutation that was present in each isolate, the aforementioned missense mutation in rpsL (on-pathway), resulting in the substitution of Arg for Lys at position 88. Conversely, the StrepR LVS isolates harbored 28 unique mutations across a variety of loci. Interestingly, a single isolate Fn Strep512-1, harbored a stretch of SNPs and deletions in positions 457–474 of the gene encoding for the two-component sensor histidine kinase (AW21_RS06710). Since this gene is already annotated as a pseudogene, and the mutations occur after the premature stop codon, protein function is likely not impacted. The only two mutations suspected to impact protein function are missense mutations found in the genes encoding for the nicotinamide adenine dinucleotide (phosphate)/ flavin adenine dinucleotide NAD(P)/FAD-dependent oxidoreductase (AW21_RS09100) and hypothetical protein (AW21_RS08665). Unexpectedly, the remaining mutations occurred in pseudogenes or transposases annotated as pseudogenes, as well as a single mutation in an intergenic region (Table 3). These mutations are not likely to affect protein function. Unlike the CipR panel, the vast majority of off-pathway mutations in the StrepR isolates were unique, occurring in only one or a few isolates (Supplementary Table S2).
The Acquisition of Ciprofloxacin Resistance Alters the Ability of Fn to Form Biofilm in vitro
The formation of a biofilm signals the transition of a cell from a primarily planktonic lifestyle into a sessile community encased within an extracellular matrix. Life within a biofilm affords a cell protection from environmental stressors and has been attributed to enhanced resistance to oxidative stress and phagocytosis as well as resistance to antibiotics (Shirtliff et al., 2002; Yan and Bassler, 2019). To determine if biofilm formation was altered in the ciprofloxacin and streptomycin resistant strains used in this study, we focused on F. novicida antibiotic resistant strains as previous studies have reported that this subspecies readily forms biofilm in vitro on a variety of surfaces (Margolis et al., 2010; Zogaj et al., 2012; van Hoek, 2013). To assess biofilm formation, antibiotic resistant strains were grown statically in BHI with IsovitaleX for 3 days at 37°C and subsequently stained with crystal violet. In agreement with growth curve analysis when shaking, static incubation of antibiotic resistant strains yielded a similar optical density to wild-type (data not shown). Interestingly, crystal violet staining revealed that all CipR strains had approximately 50% of the biofilm compared to the parental wild-type strain (Figure 3A). This defect was not observed in StrepR strains, suggesting the phenotype is likely specific to mutations harbored in CipR strains and not an artifact of panel construction. Further, no differences were observed comparing individual strains to each other within the CipR or StrepR panel. Similar results were obtained using FM1-43 as a secondary method of biofilm staining (data not shown).
While Fn CipR strains failed to achieve wild-type levels of biofilm formation, we next wanted to explore the possibility that CipR strains may accrue biofilm at a slower rate giving the appearance of a biofilm defect at 3 days. To address this question, a time course experiment was performed by measuring biofilm formation at 1, 3, 5, and 7 days. Since individual strains within a panel shared a phenotype, Fn Cip80-10 and Fn Strep512-10 were chosen as representative strains for each resistance panel. The time course revealed that peak biofilm in Fn occurred at day 3 and gradually decreased after this time resulting in about 50% of the peak biofilm by day 7 (Figure 3B). Fn Strep512-10 mirrored wild-type and also peaked on day 3 with and decreased to day 7, suggesting that biofilm formation is not affected in these strains. Interestingly, the Fn Cip80-10 strain had a significant decrease in biofilm at both day 1 and 3 compared to wild-type (p < 0.01, one-way ANOVA). However, the observed decrease in biofilm did not occur in Fn Cip80-10 after day 3 resulting in similar levels of biofilm by day 7. These results suggest that biofilm development in Fn is a dynamic process as biofilm accrued until day 3, after which the overall amount of biofilm began to decrease, likely due to remodeling processes occurring as the biofilm matures. Further, the acquisition of ciprofloxacin resistance (including either on- or off- target mutations) alters both the ability of Fn biofilm to accrue and remodel as observed in wild-type cells.
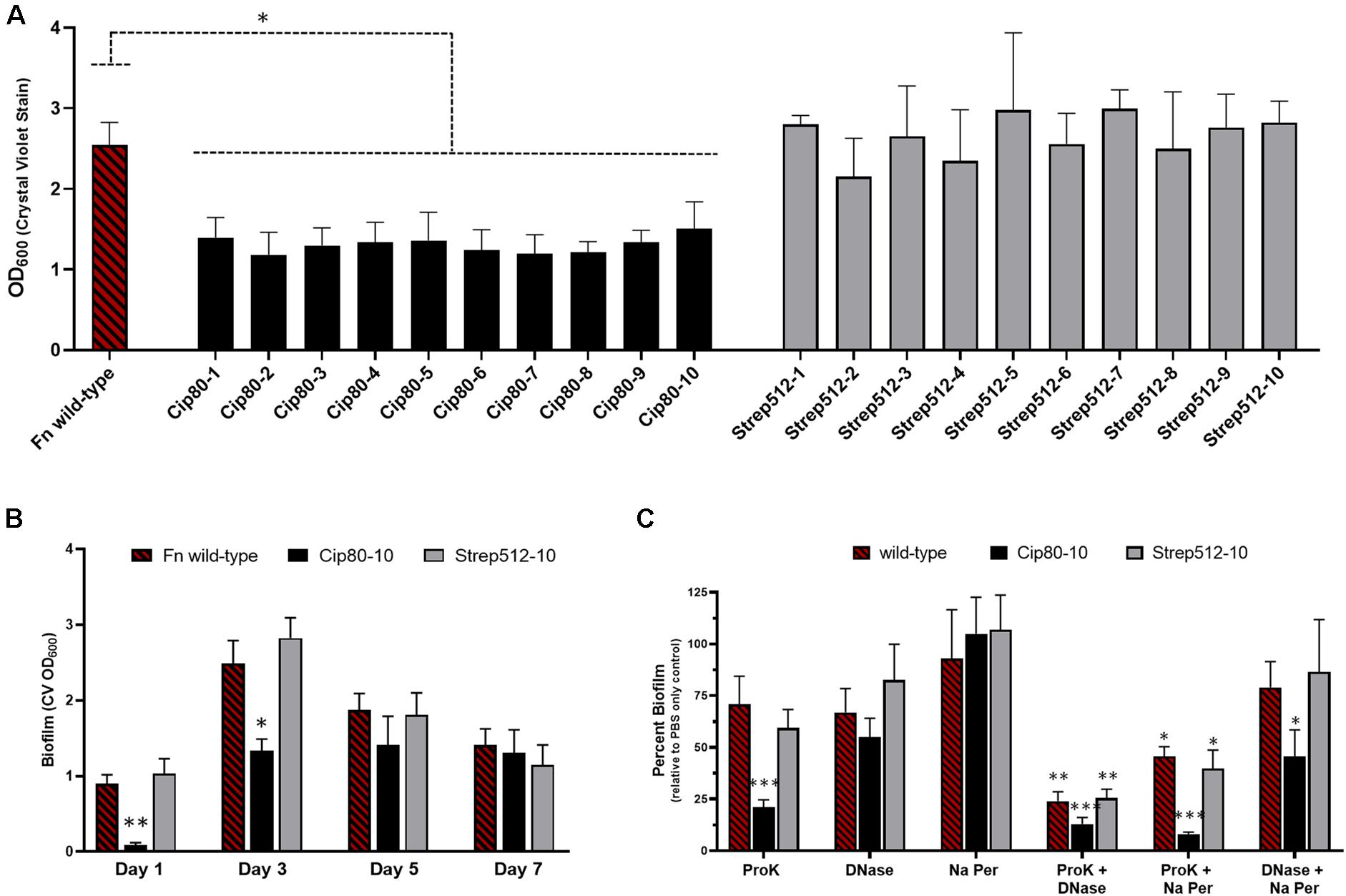
Figure 3. The acquisition of ciprofloxacin resistance delays the formation of biofilm and alters matrix composition. (A) Biofilm formation of the F. novicida CipR and StrepR surrogate strains as assessed by crystal violet staining after static growth for 3 days at 37°C. (B) Time course of biofilm formation for Fn WT compared to representative antibiotic resistant strains Cip80-10 and Strep512-10 as assessed by crystal violet staining after growth for 1, 3, 5, and 7 days. (C) Biofilm matrix composition analysis. Biofilms for Fn WT and representative CipR and StrepR strains were grown for 3 days and treated with proteinase K, DNase, sodium metaperiodate, or PBS as a control, then stained with crystal violet to assess biomass. Results are plotted as percent biofilm relative to PBS control. Significance was assessed using a one-way (A,B) or two-way ANOVA (C). ∗p < 0.05, ∗∗p < 0.01, ∗∗∗p < 0.001.
We hypothesized that the delayed onset of biofilm development and overall biofilm decrease could be due to an altered matrix composition synthesized by the CipR strains. Given that biofilm is often a mixed polymeric matrix consisting primarily of extracellular DNA, proteins, and polysaccharides, we hypothesized that the ratio of one of these macro-components may be diminished or absent from the matrix. To investigate the composition of the biofilm matrix, Fn biofilm was grown 3 days to achieve peak biofilm then subjected to treatment with the matrix degrading treatments with DNase I, proteinase K, and sodium-meta-periodate, and stained to assess the remaining biofilm. Proteinase K and DNase I had an equal effect on the Fn wild-type biofilm matrix, removing ∼25% of the biomass for each treatment compared to the PBS only treatment while sodium-meta-periodate had little to no effect (Figure 3C). The representative StrepR strain tested displayed no alterations in the biofilm matrix as the treatments yielded results that mirrored wild-type biofilm. Interestingly, proteinase K treatment removed ∼75% of the biofilm formed by the CipR10 mutant (p < 0.001, two-way ANOVA), while displaying a similar sensitivity to DNase I as observed in the wild-type biofilm. The susceptibility of the biofilm formed by the CipR mutant to proteinase K treatment suggests that the matrix components in this mutant are altered compared to the wild-type. Indeed, these results are further supported by combinatory treatment of the biofilm matrix with two enzymes simultaneously. In these experiments, the Fn wild-type biofilm was reduced by ∼75% when treated with proteinase K and DNase while the CipR strain was almost completely removed with less than 10% remaining (Figure 3C). These results suggest that polysaccharides do contribute to the Fn biofilm matrix, but likely are a minor component as treatment with both proteinase K and sodium-meta-periodate was able to remove more matrix material than proteinase K alone (25% proteinase K only compared to 50% proteinase K and Na-m-periodate). Additionally, combinatory treatment with DNase I and sodium-m-periodate shows an increase susceptibility of the CipR biofilm matrix to these treatments compared to wild-type, further suggesting that the matrix is altered in the CipR strains.
Ciprofloxacin Resistant Isolates Are Impaired for Intracellular Replication in Macrophages
The ability of Francisella to infect and replicate within macrophages and other host cell types is integral to its pathogenesis in humans and other mammals. In an effort to assess how the development of antibiotic resistance affects fitness in Francisella, we performed gentamicin protection assays with the CipR and StrepR surrogate panels using J774A.1 murine macrophage-like cells. Figure 4A shows the percent CFU recovery of F. novicida WT and two representative CipR and StrepR isolates, Fn Cip80-1 and Cip80-10, and Fn Strep512-1 and Strep512-10, respectively. Wild-type F. novicida exhibited an approximately 2 log increase in the CFU recovered from the 4 to 24 h time-point, with a percent increase of ∼15,000%. In contrast, the Fn CipR strains showed little increase in CFU at 24 h compared to 4 h, and the Fn StrepR strains had a percent CFU increase of ∼1,000%, as shown in Figure 4A. These experiments demonstrate that antibiotic resistant F. novicida variants are significantly attenuated for replication in macrophages (p < 0.05), a critical host cell reservoir, suggesting these isolates may also be attenuated during infection in vivo.
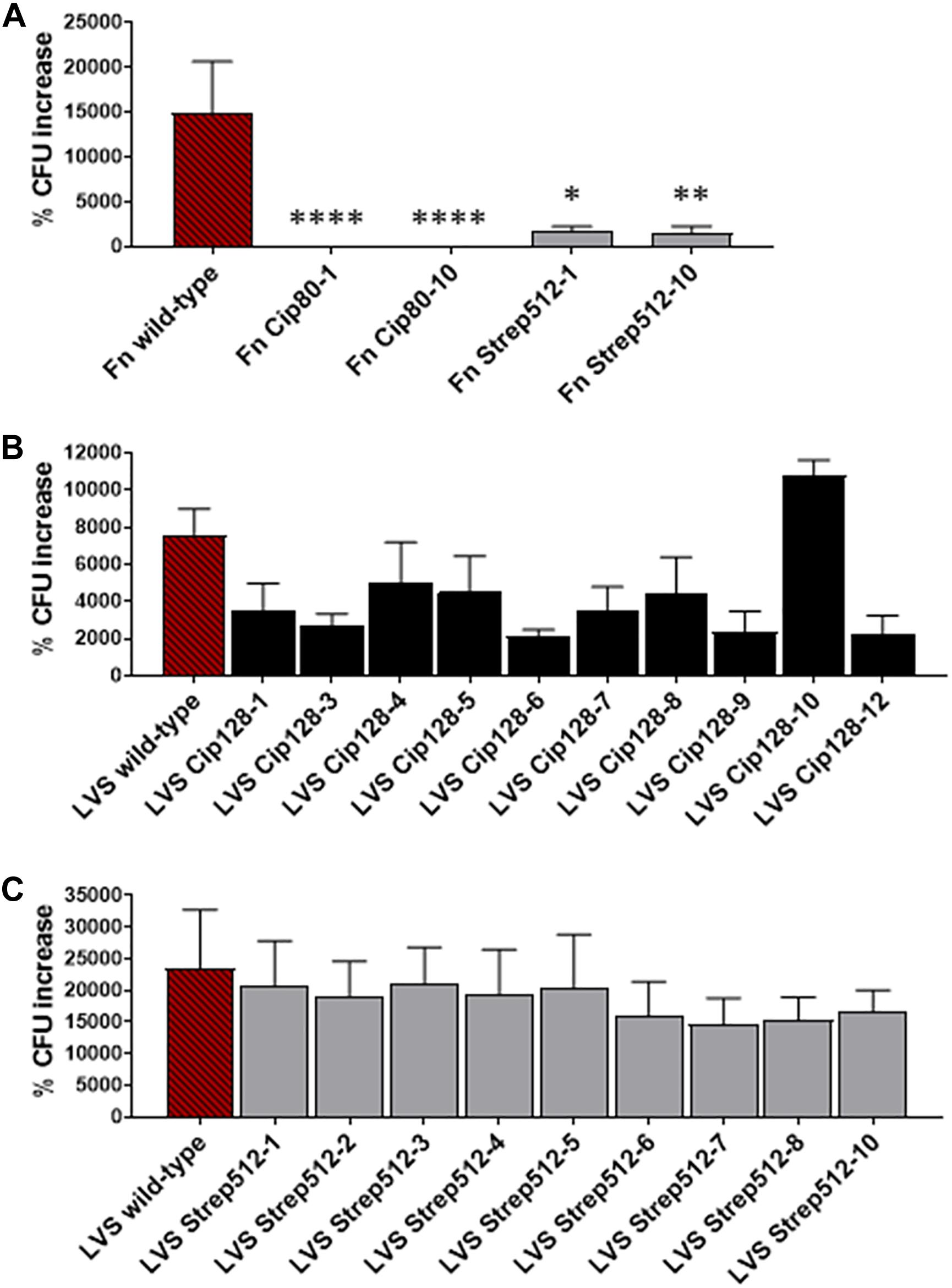
Figure 4. Intracellular replication of CipR and StrepR strains in J774A.1 murine macrophages. Gentamicin protection assays were used to assess intracellular replication of (A) F. novicida WT and two representative CipR and StrepR isolates, (B) the LVS CipR panel, and (C) the LVS StrepR panel. Macrophages were infected with F. novicida at an MOI ∼100 and cultured in the presence of gentamicin for 4 and 24 h, after which cells were lysed and plated for intracellular bacteria. % CFU recovery of each strain from 24 h compared to 4 h is shown in comparison to the parent F. novicida or LVS. Significance was determined using an ANOVA with Dunnett’s post-hoc procedure. ∗p < 0.05, ∗∗p < 0.01, ****p < 0.0001.
We next tested the entire LVS CipR and StrepR panel for replication in J774A.1 cells using the gentamicin protection assay. In this set of experiments, parent LVS exhibited a % CFU increase of ∼8,000. A general trend with a decrease % CFU recovery was observed with most of the CipR isolates ranging from ∼2,000 to 8,000% (Figure 4B), though not statistically significant. We observed no difference in CFU recovery for all of the LVS StrepR variants (Figure 4C), indicating the mutations acquired during streptomycin resistance acquisition likely have negligible impact on replication within a macrophage for LVS variants.
Ciprofloxacin and Streptomycin Resistant Francisella Are Significantly Attenuated in Mice
To determine how the acquisition of ciprofloxacin and streptomycin resistance affects the virulence of Francisella spp., the BALB/c mouse model of intranasal infection was utilized. A panel of Fn and LVS strains was selected for mouse challenges based upon their representative patterns of mutations, as well as their attenuation observed in the above macrophage studies. For Fn variants, which harbored the same mutations across isolates, Fn Cip80-1 and Cip80-10, and Fn Strep512-1 and Strep512-10 were chosen for challenges. For LVS variants, which exhibited more variation in patterns of mutation across isolates, LVS Cip128-3, and Cip128-12 strains were chosen to represent ciprofloxacin passaged strains, while LVS Strep512-1, 4, and 5, were chosen to represent streptomycin-passaged strains.
Both Fn Cip80-1 and Cip80-10 strains were significantly attenuated for virulence, with complete survival of mice at the tested doses of 23 CFU and 79 CFU, respectively, compared to the LD50 of ∼0.1 CFU for the parent WT strain (Figures 5A–C and Table 4). In contrast, the Fn Strep512-1 and Strep512-10 strains were slightly (but significantly) attenuated in mice (Figures 5D,E), but no delay in the median time to death was observed compared to the parent Fn strain.
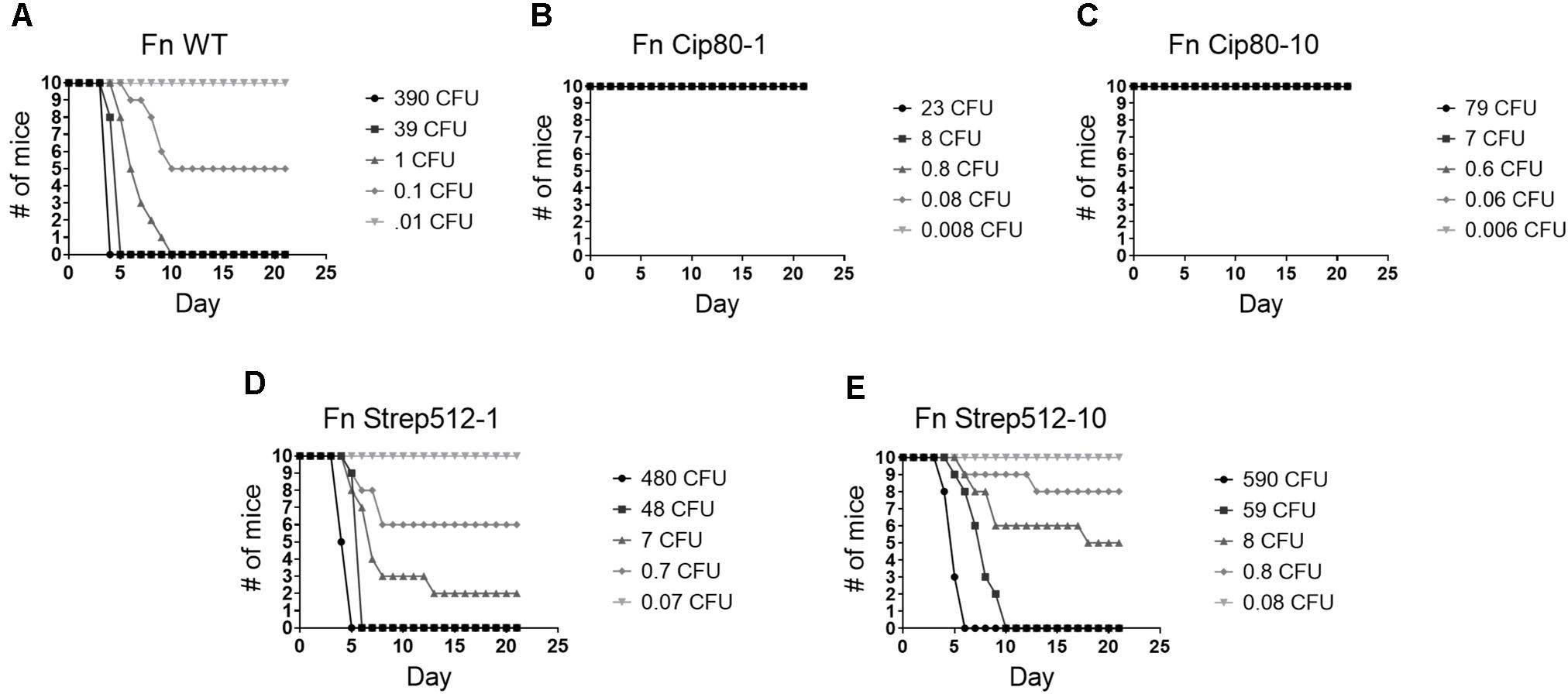
Figure 5. Virulence assessment of F. novicida parent (A) and select Fn CipR (B,C) and StrepR strains (D,E) in the BALB/c mouse model of intranasal infection. Mice were inoculated via the intranasal route with 50 μL of varying doses of each strain. Mice were then monitored over the course of 21 days and survival curves plotted. Serial dilutions of bacterial suspensions were plated to enumerate CFUs in challenge doses. LD50 values and median time-to-death were calculated and are shown in Table 4.
Virulence of CipR and StrepR variants followed a similar pattern in the LVS background. LVS Cip128-3 and Cip128-12 strains were significantly attenuated in the mice, with complete survival even at the highest doses tested, 64,000 CFU and 253,000 CFU, respectively, compared to the calculated LD50 of 172 CFU for WT (Figures 6A–C and Table 4). Of the LVS StrepR variants tested, Strep512-1, Strep512-4, and StrepR512-5 had significantly higher LD50 values compared to the parent strain; however, the median time to death for all StrepR variants was similar to WT (Figures 6D–F and Table 4). Overall, these results demonstrate that ciprofloxacin and streptomycin resistance leads to decreased virulence in Fn and LVS. Whether this attenuation is a direct result of antibiotic resistance, or due to the auxiliary mutations acquired during development of antibiotic resistance, is an interesting question that remains to be answered.
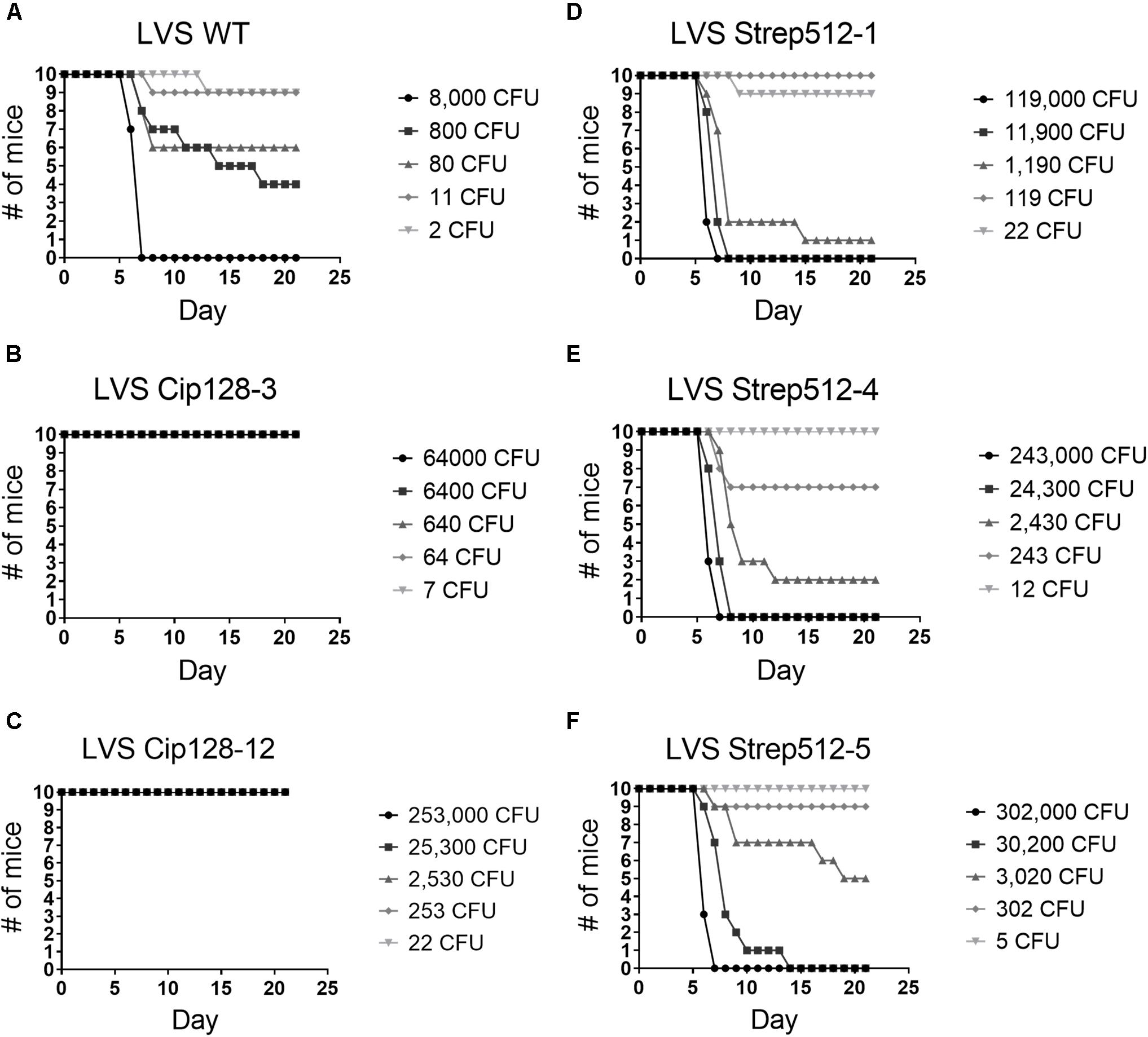
Figure 6. Virulence assessment LVS parent and select CipR and StrepR strains in the BALB/c mouse model of intranasal infection. Mice were infected and survival curves plotted. LD50 values and median time-to-death were calculated and are shown in Table 4.
Discussion
When cultured in increasing levels of ciprofloxacin or streptomycin, Fn and LVS were able to obtain high levels of resistance after 12 passages. Selecting isolated colonies from these populations allowed for the construction of a ciprofloxacin and streptomycin resistant strain panel featuring a diverse array of mutations across 20 Fn and 20 LVS surrogate strains. Whole genome sequencing revealed that these strains acquired both mutations directly involved in antibiotic resistance (on-pathway) as well as mutations in genes unrelated to antibiotic resistance (off-pathway). Interestingly, when deriving resistance by this natural selection method, many of these surrogate strains were attenuated in a mouse model, most apparent in the CipR strains, suggesting that the evolution of antibiotic resistance to fluoroquinolones and aminoglycosides may come at a cost for F. tularensis as virulence was decreased. However, we cannot speak to the fitness of resistant strains derived by other methods or strains passaged without antibiotic selection. With the current data in mind, some of these mutations harbored within these strains are of interest as potential targets for future medical countermeasures. For instance, some of the proteins encoded by the identified virulence factors could be used for future vaccine design as part of a subunit vaccine or in some version of Francisella mutation to be used as part of an attenuated live vaccine. Although, a stronger avenue for future biodefense needs, especially, for the enzymatic targets, would be drug design which is discussed in more detail below.
On-Pathway Mutations Discovered in Ciprofloxacin Resistant Strains
Both F. novicida and LVS passaged on ciprofloxacin acquired mutations within the DNA gyrase and topoisomerase IV subunit genes, products of which are known targets of this antibiotic. The subunits of DNA gyrase, gyrA, and gyrB, and those of the topoisomerase IV, parC, and parE, share significant sequence homology with a high conservation of amino acids in the quinolone resistance-determining region, or QRDR (Hooper, 2001). Loveless et al. reported several mutations in gyrA and parE of F. tularensis Schu S4 as a result of serial passaging on increasing concentrations of ciprofloxacin (Loveless et al., 2010). Whole genome sequencing of our ciprofloxacin-passaged isolates revealed two mutations in gyrA and one mutation in parC for Fn CipR strains, as well as one mutation in gyrA, one in gyrB, and one in parE for the LVS CipR strains. All of these mutations led to an amino acid change to the respective protein. Interestingly, the same Thr83Ile mutation was present in gyrA of both LVS and F. novicida CipR strains; this is a key residue known to be involved in fluoroquinolone resistance across both gram-positive and gram-negative bacteria (Hooper, 2001). This mutation was previously found in a ciprofloxacin-resistant derivative of a F. tularensis subsp. holarctica clinical isolate, as well as a F. tularensis Schu S4, LVS, and F. novicida strains passaged on ciprofloxacin (La Scola et al., 2008; Loveless et al., 2010; Sutera et al., 2014). The second mutation found in gyrA of the Fn CipR strains was Asp87Tyr, also previously identified as a hotspot for fluoroquinolone resistance in F. novicida and LVS, as well as in other species including Mycobacterium tuberculosis and E. coli (Matrat et al., 2008; Sutera et al., 2014; Jaing et al., 2016; Caspar et al., 2017). Notably, Jaing et al. (2016) observed gyrA mutations affecting amino acids 83 and 87 occurring in the same LVS isolate and showed that the presence of both mutations conferred higher levels of ciprofloxacin resistance than each individual mutation (Jaing et al., 2016). Interestingly, the study by Caspar et al. (2017) also found that F. novicida developed the first mutation in gyrB rather than gyrA upon passaging on increasing ciprofloxacin. Our study found the same Ser465Tyr mutation in gyrB; however it was only identified in LVS and not Fn CipR variants (Caspar et al., 2017). In most cases, the literature is consistent that gyrase is the primary target of ciprofloxacin, and topoisomerase is the secondary target; typically, a mutation in the gyrase and additional mutation in a topoisomerase gene is required to attain high levels of quinolone resistance. Our study supports this trend, but also adds evidence to suggest that gyrB may be the primary target in LVS, while gyrA may be the primary target in F. novicida. The order of mutations may be dependent on the strain or culture conditions used to derive resistance. Further studies utilizing a range of isolates could shed further light on how these mutations are acquired during antibiotic resistance development.
During passage of Fn on ciprofloxacin concentrations up to 80 μg mL–1, we observed high levels of resistance, as shown by E-tests (>32 vs. 0.008 for WT). This observation could possibly be explained by the order in which mutations occur in both the gyrase and topoisomerase genes. It has been shown that DNA gyrase is the primary target of quinolone action, and that parC-mediated resistance is only obtained in gyrA mutants at high fluoroquinolone concentrations (Drlica and Zhao, 1997). All of the Fn CipR variants in our study harbor two mutations in gyrA and a single mutation in parC. It is likely the gyrA mutations were acquired early during passaging, and subsequent passaging on higher concentrations led to the parC mutations, resulting in high levels of ciprofloxacin resistance. In general, the presence of two gyrA mutations and one parC mutation is associated with high levels of quinolone resistance (Vila et al., 1996). Conversely, the LVS CipR strains harbored a single gyrA mutation, Thr83Ile also found in Fn CipR, and two additional amino acid changes in gyrB and parE. In general, mutation of the serine at position 83 in E. coli isolates is associated with moderate levels of ciprofloxacin resistance (Drlica and Zhao, 1997). While QRDR mutations typically reduce the fitness of resistant organisms, it has been established that energetically favorable mutations occurring in the QRDR, mainly double-serine mutations, provide favorable fitness to bacteria during resistance development (Fuzi et al., 2017). The Thr83Ile mutation identified in gyrA of our study could provide a slight fitness advantage, while all other mutations are expected to cause a loss of fitness (Fuzi et al., 2017).
Ciprofloxacin Resistant Strains Harbor Multiple Mutations in Efflux Pumps
Several of the mutations found in our resistance panel occurred in genes encoding multidrug efflux pumps. Although not the targets of the antibiotics themselves, these mutations could be expected to alter resistance to either ciprofloxacin or streptomycin. Three mutations occurred in components of resistance-nodulation-cell division (RND) family efflux transporters. The RND efflux transporters are tripartate systems, composed of an outer membrane channel-forming protein, a secondary inner membrane transporter, and a periplasmic adaptor protein that bridges the two membrane proteins. The AcrAB-TolC system of E. coli is the most well characterized efflux system, in which TolC forms the outer membrane channel, AcrB functions as the inner membrane transporter, and AcrA is the periplasmic adaptor protein that connects the two (Du et al., 2014).
Previous studies focused on F. novicida ciprofloxacin resistance identified mutations in several RND efflux genes, including FTN_1610, encoding an RND efflux transporter, and FTN_1609, encoding the associated periplasmic adaptor subunit (Caspar et al., 2017; Siebert et al., 2019). In our study, we observed a single amino acid change in the outer membrane efflux protein TolC (encoded by AW21_RS03270) of the LVS CipR variants. The mutation resulted in an Arg453Ser substitution located in the outer membrane efflux protein (OEP) domain of tolC. The F. tularensis genome encodes two orthologs of TolC from E. coli, the aforementioned tolC gene and ftlC, both of which are highly conserved across all three subspecies. Gil et al. (2006) previously demonstrated that tolC was necessary for both drug resistance and virulence in a murine model of intradermal infection (Gil et al., 2006).
Additional mutations were identified in the acrA and acrB homologs upon passaging in ciprofloxacin. All Fn CipR variants contained an insertion of a histidine residue at position 263 of AcrA (AW25_RS01955). A BLAST search of this protein revealed ∼25% similarity to AcrA of E. coli, with the mutation present in the domain responsible for interaction with the AcrB subunit. All LVS CipR variants harbored an Arg653His mutation in AcrB (AW21_RS02275). BLAST search showed CmeB from Campylobacter jejuni as the closest Protein Data Bank (PDB) entry (97% query cover, ∼29% identity; 5T0O_A). Importantly, a cmeB mutant in C. jejuni was shown to have enhanced susceptibility to a variety of antibiotics including the fluoroquinolones, and ciprofloxacin specifically was shown to accumulate inside cells of the cmeB mutant, further providing support for a role of the Francisella AcrB protein in ciprofloxacin resistance (Pumbwe and Piddock, 2002). In contrast, a previous study developed an acrB mutant in LVS and showed that, while it was important for resistance to β-lactams and other antibiotics, ciprofloxacin resistance was unaffected (Bina et al., 2008). Moreover, it is unclear whether the AcrA/B subunits described above interact with the TolC or FtlC outer membrane components encoded in the Francisella genome, as they can switch adapter subunits.
For both the LVS and Fn CipR variants, mutations found in the efflux pump components could potentially contribute to the slightly higher MIC of doxycycline and chloramphenicol that we observed. These antibiotics would be substrates of these transporters. As fluoroquinolones are substrates of AcrAB-TolC system, mutations in these families of efflux pumps could help the Francisella strains to adapt themselves to higher concentrations of ciprofloxacin, resulting in a slight multidrug resistance.
Off-Pathway Mutations Discovered in Ciprofloxacin Resistant Strains
An interesting mutation that appeared in our study was the presence of a single bp insertion at position 107 in the gene encoding a DUF3573 domain-containing protein, causing a frameshift (AW21_RS06025). This is a unique fusion protein referred to as FupA/B, resulting from a deletion and recombination event between the adjacent genes fupA and fupB. FupA/B is necessary for iron acquisition in Francisella, and interestingly, multiple studies previously found mutations in fupA/B in floroquinolone-resistant Francisella derivatives (Jaing et al., 2016; Caspar et al., 2017; Chance et al., 2017; Siebert et al., 2019). Jaing et al. (2016) identified multiple fupA mutations in ciprofloxacin-resistant LVS isolates, two of which resulted in a premature stop codon and truncated protein product (Jaing et al., 2016). The recent study by Siebert et al. (2019) identified a single nucleotide insertion in the same location as our study, which occurred as a second step event after the development of mutations in the DNA gyrase. They further demonstrated that a fupA/B mutant exhibited a threefold increased MIC in comparison to WT, and this was accompanied by increased secretion of outer membrane vesicles and the formation of biofilm (Siebert et al., 2019). Our study showed a decreased biofilm capacity for all of the CipR Fn mutants. However, in agreement with Siebert et al., a screen of the LVS CipR and StrepR panel revealed increased biofilm formation by the CipR strains (Supplementary Figure S1). Perhaps the fupA frameshift mutation also identified in our study contributes to the increased outer membrane vesicle secretion and thus increased biofilm capacity.
Two LPS-related genes, wbtC and lptE, contained mutations resulting in truncated proteins in this study. The wbtC gene encodes the NAD-dependent epimerase involved in LPS biosynthesis, while lptE encodes an outer membrane lipoprotein that functions in complex with LptD to transport LPS across the outer membrane. Interestingly, the wbtQ and wbtH genes, also involved in LPS biosynthesis, were found to have mutations resulting in premature stop codons in ciprofloxacin-resistant F. novicida (Caspar et al., 2017). Moreover, our laboratory previously reported a mutation in the kdsD gene of a ciprofloxacin-resistant derivative of F. tularensis Schu S4, which resulted in growth defects, altered LPS profiles, and attenuation in mice (Chance et al., 2017). The high impact mutations reported here and by others support a potential role for the O-antigen in susceptibility to fluoroquinolones.
On-Pathway Mutations Discovered in Streptomycin Resistant Strains
Streptomycin functions by binding to the 30S subunit of the ribosome, thereby inhibiting protein synthesis (Luzzatto et al., 1968). In our study, both Fn and LVS passaged in streptomycin acquired a mutation in rpsL, the gene encoding the 30S ribosomal protein S12. Early publications characterizing high levels of streptomycin resistance in E. coli determined that mutation of lysine 42 to asparagine, threonine or arginine in the S12 protein conferred resistance (Funatsu and Wittmann, 1972). This finding was later extended to include lysine 43 in E. coli as well as an array of other bacteria after additional work revealed streptomycin interactions directly with the lysine residues at this location of the S12 protein (Sreevatsan et al., 1996; Barnard et al., 2010; Miskinyte and Gordo, 2013). While these amino acid substitutions of RpsL abolish the interaction with streptomycin, the polar substitutions of threonine and asparagine display a restrictive phenotype thereby increasing the fidelity of aminoacyl-tRNA (Sharma et al., 2007). Interestingly, the mutation in rpsL resulting in Lys43Arg was one of only two mutations identified in the Fn background. This finding is consistent with the reported non-restrictive phenotype of the Lys42Arg mutation in the S12 protein and may help explain the lack of mutational diversity observed in the Fn StrepR panel. Further, it is possible that the selection pressure applied by streptomycin forced the mutation of rpsL early during exposure, and this allele became fixed in the population during subsequent passaging (Heinemann, 1999; Vuilleumier et al., 2008; Baquero et al., 2013). Taken together, these findings potentially explain why relatively few mutations were identified in Fn despite serial passages in streptomycin.
Additional work has identified Lys88 in the S12 protein as a key residue responsible for spontaneous drug resistance in E. coli, as frequent mutations K88Q and K88R have been associated with streptomycin resistance (Springer et al., 2001; Benítez-Páez et al., 2014). Consistent with these studies, the K88R mutation in the S12 protein was identified in the LVS StrepR panel in our study. Interestingly, a stretch of single bp changes and deletions were observed in rsmG, encoding the ribosomal RNA small subunit methyltransferase G. The rsmG allele is annotated as a pseudogene in the LVS genome, and these mutations are located downstream of the premature stop codon, making it highly likely this protein is inactive in our strains. Multiple studies have recently demonstrated that loss of RsmG activity confers low-level streptomycin resistance that gives rise to mutations resulting in high-level resistance (Nishimura et al., 2007; Benítez-Páez et al., 2014). One of these synergistic mutations was identified as the K88R allele of the S12 protein (Benítez-Páez et al., 2014).
Several LVS StrepR variants were found to contain a Trp18Leu substitution in the MFS transporter. MFS transporters facilitate the transfer of a wide range of small molecules substrates across the cell membrane including amino acids, sugars, oxyanions, and large peptides. However, in bacteria, MFS transporters are mostly credited as nutrient transporters and drug efflux pumps (Schuldiner, 2018; Kumar et al., 2020). Previous work has shown that MFS transporters play an essential role in F. tularensis virulence by influencing nutrient acquisition within a host cell, and that inactivation of key MFS transporters results in attention in vivo in both Type A and B strains (Pérez et al., 2016; Balzano et al., 2018). Given the mild attenuation observed in the StrepR LVS strains, it is likely that this transporter functions as an efflux pump, though further work is required to identify the substrate of the MFS transporter identified in these strains.
Off-Pathway Mutations Discovered in Streptomycin Resistant Strains
No off-pathway mutations were found in the Fn StrepR surrogate panel. However, the LVS StrepR panel contained a multitude of off-pathway mutations, the majority of which were found in pseudogenes and transposases (discussed below). Two exceptions were mutations in the NAD(P)/FAD-dependent oxidoreductase and a hypothetical protein, neither of which have been previously reported to be involved in antibiotic resistance. Several LVS StrepR mutations were found in or nearby transposase genes, including four in IS630 family transposases and one in an IS5/IS1182 family transposase, all of which were annotated as pseudogenes. Moreover, the LVS CipR panel contained a frameshift in the gene encoding for an IS630 family transposase, similar to several LVS StrepR strains. The complete genome sequence of F. tularensis Schu S4 was reported to have an abundance of IS elements, the most common of which were IS630 family (ISFtu1) with 50 copies, and IS5 family (ISFtu2) with 16 copies (Larsson et al., 2005). It is possible that mutations frequently occurred in the IS elements because they are prevalent in the genome or that these regions tend to have higher mutation frequencies in general.
Ciprofloxacin Resistance Decreases the Biofilm Forming Capacity of Fn
The prevailing model is that biofilm formation allows Francisella to adapt and persist in an aquatic environment or vector reservoir (Mahajan et al., 2011; van Hoek, 2013). It is well established that biofilm enhances the ability of bacteria to cope with antibiotic stress (Chung et al., 2014; Siebert et al., 2019, 2020). Opposing this dogma, a link between quinolone resistance and biofilm forming capacity has been identified in several gram-negative bacteria including E. coli, Klebsiella pneumonia, Pseudomonas aeruginosa, and Salmonella enterica, with most studies reporting a decreased biofilm capacity as resistance to quinolones increases (Fàbrega et al., 2014; Cepas et al., 2018). In Salmonella, this has been attributed to decreased expression of type 1 fimbriae and the csg loci encoding curli, though Francisella appears to lack both of these systems (Vila and Soto, 2012; Fàbrega et al., 2014). Despite this, our results are consistent with these studies as we found that Fn CipR mutants displayed a delay in biofilm development and failed to reach wild-type levels. Ahmed and colleagues found that passaging P. aeruginosa in low-levels of ciprofloxacin either planktonically or in a biofilm resulted in CipR strains harboring different mutations, likely due to differences in selection pressures applied during a given lifestyle (Ahmed et al., 2018). Mutations identified in our study that overlap with Ahmed et al. (2018) were found in the Mex transporter family, gyrA and gyrB. Interestingly, the latter of these mutations were only identified in planktonically grown cultures, which is similar to how the strain panel in this study was constructed. Additional work in P. aeruginosa has suggested that these classic fluoroquinolone mutations in gyrA are permitted to occur due to secondary mutations in other genes, which in at least one instance has come at the cost of enzymes involved in cyclic-di-GMP signaling altering biofilm formation (Wong et al., 2012). Indeed, it has been convincingly demonstrated that cyclic-di-GMP is necessary for stimulating biofilm formation in F. novicida (Zogaj et al., 2012). In contrast, LVS lacks this signaling pathway, which likely accounts for its decreased biofilm capacity relative to F. novicida (Zogaj et al., 2012; van Hoek, 2013). With this in mind, further work is needed to investigate this possibility as our data show that Fn CipR strains can still form biofilm, but in a reduced capacity under an altered membrane composition.
Antibiotic Resistance Obtained by Natural Selection Leads to Attenuated Virulence in Fn and LVS
In our study, we sought to determine the effect of antibiotic resistance acquisition by serial passaging on virulence of the LVS and Fn strains using a macrophage replication assay and a murine model of intranasal infection. We showed that both CipR and StrepR F. novicida were significantly altered in replication in macrophages. However, CipR LVS strains had more variable levels of replication but most displayed a general trend for decreased intracellular replication. Interestingly, streptomycin resistance in LVS had no effect on replication in macrophages. Virulence studies using BALB/c mice showed similar patterns of attenuation as in the macrophage model, with Fn CipR isolates, and to a lesser extent Fn StrepR strains, showing significant attenuation. Similarly, the LVS CipR strains exhibited substantially more attenuation in mice, while the StrepR strains were mildly affected. Overall, these studies demonstrate that CipR strains were much more attenuated in macrophages and mice than StrepR strains, indicating the development of ciprofloxacin resistance obtained as described here may have broader impacts on virulence than that of streptomycin. A recent study demonstrated that deletion of TolC had significant impacts on both resistance profiles and virulence of F. tularensis SchuS4, suggesting a link between both antibiotic resistance and pathogenicity (Kopping et al., 2019). Similarly, the AcrB RND efflux protein from LVS was shown to be required for both β-lactam resistance and full virulence in mice (Bina et al., 2008). These studies support a dual role for multi-drug resistant efflux pumps in antibiotic resistance and virulence. The mutations in AcrA/B and TolC found in our study could account for some of the attenuation seen in the Fn and LVS CipR strains, although the strains in our panel contained single amino acid substitutions that increased resistance, as opposed to genetic deletions that increased antibiotic susceptibility of the strains. To our knowledge, this is one of the first studies to examine the link between naturally acquired antibiotic resistance and virulence in Francisella spp.
The acquisition of antibiotic resistance in bacteria is often accompanied by defects in fitness (Beceiro et al., 2013) which may explain the attenuation observed in our CipR and StrepR panel. However, this occurrence is highly species-dependent, with fluoroquinolone resistance coming at a cost to some organisms (i.e., Salmonella enterica and Acinetobacter baumannii), but not others (i.e., Pseudomonas aeruginosa and E. coli). The fitness cost also depends on the type of mutation. For example, it was shown in E. coli that streptomycin resistance acquired through mutations in ribosomal methyltransferases has no fitness cost, while ribosomal mutations come at a cost (Andersson and Levin, 1999; Gutierrez et al., 2012). In an elegant study, Marcusson et al. (2009) constructed 28 isogenic E. coli strains with combinations of up to five fluoroquinolone resistance mutations to examine the relationship between resistance and fitness. They found that fitness was affected by the nature of the mutations involved, rather than the number of mutations, and that it was possible for strains to gain high resistance levels with little compromise in fitness (Marcusson et al., 2009). Interestingly, a review by Fuzi et al. (2020), highlights that recent clonal expansion of diverse multi-drug resistant pathogens, including hospital-associated methicillin-resistant Staphylococcus aureus and extended spectrum β-lactamase-producing Klebsiella pneumoniae and E. coli, was likely driven by increased use of fluoroquinolones in recent decades (Fuzi et al., 2020). The studies summarized within demonstrate that fluoroquinolone resistance typically result in QRDR mutations or enhanced efflux activity that come at a fitness cost. However, major clones that had evolved energetically favorable QRDR mutations, were able to regain fitness and replace the minor clones (Fuzi et al., 2017, 2020).
It is currently unknown how these resistance mechanisms specifically affect fitness and virulence in Francisella spp. Alternatively, the different off-pathway mutations identified in CipR and StrepR strains could account for this. Indeed, several mutations were found in known or putative virulence factors, including Slt (cell wall biosynthesis), WbtC and LptE (LPS biosynthesis), and TolC (drug transport). It is important to note that the attenuation observed in some resistant isolates may be the result of passage in culture giving rise to attenuating mutations, rather than a result of the antibiotic selection itself. However, no overlapping mutations were found in CipR and StrepR strains, which suggests this is likely not the case. A recent investigation into the amplification of low frequency mutations during the passaging of F. tularensis without antibiotics further argues against this concept, since SNPs and small deletions were mainly found in capsule synthesis genes (Dwibedi et al., 2020). Further studies utilizing single mutants are required to determine the extent that the individual mutations contribute to attenuation.
Antibiotic Resistant Mutations for Identification of Future Drug Targets
A major strength of this study and other studies focused on deriving antibiotic resistant isolates is that off-target mutations are often important in cellular functions and can be sensitive to environmental stressors, like antibiotic selection, allowing for the identification of new drug targets. As a proof of principle, the slt gene identified in this study was found to be necessary for maintaining cell growth, morphology, and virulence and morphology of F. novicida (Bachert et al., 2019). Interestingly, the slt gene could be mutated in F. novicida, but not in LVS or F. tularensis Schu S4 strains, supporting slt as an essential gene in these species and an ideal therapeutic target (Bachert et al., 2019; Ireland et al., 2019). The mutation identified here likely does not inactivate the enzyme, given that it is essential in LVS, and that the mutation was found outside of the cluster of residues thought to be required for sugar binding and catalytic activity. Previous work in our laboratory also identified the presence of a frameshift mutation in the kdsD gene in a ciprofloxacin resistant variant of F. tularensis Schu S4. The kdsD gene encodes an enzyme involved in LPS biosynthesis, and a Schu S4 mutant was attenuated in mice (Chance et al., 2017). Current studies in our laboratory are aimed at assessing the therapeutic potential of KdsD. Numerous other studies have identified potential therapeutic targets for tularemia via the development of antibiotic resistant panels, including the FupA/B fusion protein (Siebert et al., 2019), WbtQ and WbtH proteins involved in LPS biosynthesis, and FTN_1029, encoding a protein involved in isoprenoid biosynthesis, necessary for cell wall synthesis and membrane fluidity (Caspar et al., 2017). These experiments enable us to understand how Francisella species and perhaps other gram-negative pathogens may respond to antibiotics and allow us to develop new strategies to overcome antibiotic resistance.
Data Availability Statement
The datasets presented in this study can be found in online repositories. The names of the repository/repositories and accession number(s) can be found below: https://www.ncbi.nlm.nih.gov/genbank/, SAMN15518680 SAMN15-518681 SAMN15518682 SAMN15518683 SAMN15518684 SA-MN15518685 SAMN15518686 SAMN15518687 SAMN15518688 SAMN15518689 SAMN15518690 SAMN15518691 SAMN155-18692 SAMN15518693 SAMN15518694 SAMN15518695 SA-MN15518696 SAMN15518697 SAMN15518698 SAMN155186-99 SAMN15518700 LSAMN15518701 SAMN15518702 SA-MN15518703 SAMN15518704 SAMN15518705 SAMN15518706 SAMN15518707 SAMN15518708 SAMN15518709.
Ethics Statement
Animal research at the United States Army Medical Research Institute of Infectious Diseases (USAMRIID) was approved and conducted under an Institutional Animal Care and Use Committee (USAMRIID IACUC) in compliance with the Animal Welfare Act, Public Health Service Policy, and other Federal statutes and regulations relating to animals and experiments involving animals. The facility where this research was conducted is accredited by the Association for Assessment and Accreditation of Laboratory Animal Care, International and adheres to principles stated in the Guide for the Care and Use of Laboratory Animals, National Research Council, 2011.
Author Contributions
FB, BB, KM, and JB contributed conception and design of the study and wrote the manuscript. FB, BB, KM, RT, GK, SL, CK, CC, JL, and JB participated in the experimentation and acquisition of data. FB, BB, KM, SL, JL, and JB were involved in the analysis or interpretation of data for the work. All authors contributed to manuscript revision, read and approved the submitted version.
Funding
This research was performed while BB and KM held an NRC Research Associate fellowship award and are currently supported by the CBD Research Associateship program sponsored by Defense Threat Reduction Agency (DTRA). The research described herein was sponsored by the DTRA JSTO-CBD (project number CB10645). Fabrice Biot performed this work at USAMRIID as part of the Engineer and Scientist Exchange Program.
Disclaimer
Opinions, interpretations, conclusions, and recommendations are those of the authors and are not necessarily endorsed by the U.S. Army.
Conflict of Interest
The authors declare that the research was conducted in the absence of any commercial or financial relationships that could be construed as a potential conflict of interest.
Acknowledgments
We thank Tara Kenny for culturing J774.1 cells and David Fetterer for performing the statistical analysis. We also thank Joshua Richardson for assisting with sequence submissions.
Supplementary Material
The Supplementary Material for this article can be found online at: https://www.frontiersin.org/articles/10.3389/fmicb.2020.593542/full#supplementary-material
Footnotes
References
Ahmed, M. N., Porse, A., Sommer, M. O. A., Høiby, N., and Ciofu, O. (2018). Evolution of antibiotic resistance in biofilm and planktonic Pseudomonas aeruginosa populations exposed to subinhibitory levels of ciprofloxacin. Antimicrob. Agents Chemother. 62: e00320-18.
Andersson, D. I., and Levin, B. R. (1999). The biological cost of antibiotic resistance. Curr. Opin. Microbiol. 2, 489–493. doi: 10.1016/s1369-5274(99)00005-3
Bachert, B. A., Biryukov, S. S., Chua, J., Rodriguez, S. A., and Toothman, R. G. Jr., et al. (2019). A Francisella novicida mutant, lacking the soluble lytic transglycosylase Slt, exhibits defects in both growth and virulence. Front. Microbiol. 10:1343. doi: 10.3389/fmicb.2019.01343
Balzano, P. M., Cunningham, A. L., Grassel, C., and Barry, E. M. (2018). Deletion of the major facilitator superfamily transporter fptB alters host cell interactions and attenuates virulence of type A Francisella tularensis. Infect. Immun. 86:e00832-17.
Baquero, F., Tedim, A. P., and Coque, T. M. (2013). Antibiotic resistance shaping multi-level population biology of bacteria. Front. Microbiol. 4:15. doi: 10.3389/fmicb.2013.00015
Barnard, A. M. L., Simpson, N. J. L., Lilley, K. S., and Salmond, G. P. C. (2010). Mutations in rpsL that confer streptomycin resistance show pleiotropic effects on virulence and the production of a carbapenem antibiotic in Erwinia carotovora. Microbiology 156, 1030–1039. doi: 10.1099/mic.0.034595-0
Barras, V., and Greub, G. (2014). History of biological warfare and bioterrorism. Clin. Microbiol. Infect. 20, 497–502. doi: 10.1111/1469-0691.12706
Beceiro, A., Tomás, M., and Bou, G. (2013). Antimicrobial resistance and virulence: a successful or deleterious association in the bacterial world? Clin. Microbiol. Rev. 26, 185–230. doi: 10.1128/cmr.00059-12
Benítez-Páez, A., Cárdenas-Brito, S., Corredor, M., Villarroya, M., and Armengod, M. E. (2014). Impairing methylations at ribosome RNA, a point mutation-dependent strategy for aminoglycoside resistance: the rsmG case. Biomedica 34(Suppl. 1), 41–49.
Bina, X. R., Lavine, C. L., Miller, M. A., and Bina, J. E. (2008). The AcrAB RND efflux system from the live vaccine strain of Francisella tularensis is a multiple drug efflux system that is required for virulence in mice. FEMS Microbiol. Lett. 279, 226–233. doi: 10.1111/j.1574-6968.2007.01033.x
Caspar, Y., Hennebique, A., and Maurin, M. (2018). Antibiotic susceptibility of Francisella tularensis subsp. holarctica strains isolated from tularaemia patients in France between 2006 and 2016. J. Antimicrob. Chemother. 73, 687–691. doi: 10.1093/jac/dkx460
Caspar, Y., and Maurin, M. (2017). Francisella tularensis susceptibility to antibiotics: a comprehensive review of the data obtained in vitro and in animal models. Front. Cell Infect. Microbiol. 7:122. doi: 10.3389/fcimb.2017.00122
Caspar, Y., Siebert, C., Sutera, V., Villers, C., Aubry, A., Mayer, C., et al. (2017). Functional characterization of the DNA gyrases in fluoroquinolone-resistant mutants of Francisella novicida. Antimicrob. Agents Chemother. 61:e02277-16. doi: 10.1128/AAC.02277-16
Cepas, V., López, Y., Muñoz, E., Rolo, D., Ardanuy, C., Martí, S., et al. (2018). Relationship between biofilm formation and antimicrobial resistance in Gram-negative bacteria. Microb. Drug Resist. 25, 72–79. doi: 10.1089/mdr.2018.0027
Chamberlain, R. E. (1965). Evaluation of live tularemia vaccine prepared in a chemically defined medium. Appl. Microbiol. 13, 232–235. doi: 10.1128/aem.13.2.232-235.1965
Champion, A. E., Catanzaro, K. C. F., Bandara, A. B., and Inzana, T. J. (2019). Formation of the Francisella tularensis biofilm is affected by cell surface glycosylation, growth medium, and a glucan exopolysaccharide. Sci. Rep. 9:12252.
Chance, T., Chua, J., Toothman, R. G., Ladner, J. T., Nuss, J. E., Raymond, J. L., et al. (2017). A spontaneous mutation in kdsD, a biosynthesis gene for 3 Deoxy-D-manno-Octulosonic Acid, occurred in a ciprofloxacin resistant strain of Francisella tularensis and caused a high level of attenuation in murine models of tularemia. PLoS One 12:e0174106. doi: 10.1371/journal.pone.0174106
Chung, M. C., Dean, S., Marakasova, E. S., Nwabueze, A. O., and Van Hoek, M. L. (2014). Chitinases are negative regulators of Francisella novicida biofilms. PLoS One 9:e93119. doi: 10.1371/journal.pone.0093119
Cingolani, P., Platts, A., Wang, Le, L., Coon, M., Nguyen, T., et al. (2012). A program for annotating and predicting the effects of single nucleotide polymorphisms, SnpEff: SNPs in the genome of Drosophila melanogaster strain w1118; iso-2; iso-3. Fly 6, 80–92. doi: 10.4161/fly.19695
Costerton, J. W., Stewart, P. S., and Greenberg, E. P. (1999). Bacterial biofilms: a common cause of persistent infections. Science 284, 1318–1322. doi: 10.1126/science.284.5418.1318
Dennis, D. T., Inglesby, T. V., Henderson, D. A., Bartlett, J. G., Ascher, M. S., Eitzen, E., et al. (2001). Tularemia as a biological weapon: medical and public health management. JAMA 285, 2763–2773. doi: 10.1001/jama.285.21.2763
Drlica, K., and Zhao, X. (1997). DNA gyrase, topoisomerase IV, and the 4-quinolones. Microbiol. Mol. Biol. Rev. 61, 377–392. doi: 10.1128/.61.3.377-392.1997
Du, D., Wang, Z., James, N. R., Voss, J. E., Klimont, E., Ohene-Agyei, T., et al. (2014). Structure of the AcrAB-TolC multidrug efflux pump. Nature 509, 512–515.
Dwibedi, C., Larsson, P., Ahlinder, J., Lindgren, P., Myrtennäs, K., Granberg, M., et al. (2020). Biological amplification of low frequency mutations unravels laboratory culture history of the bio-threat agent Francisella tularensis. Forensic Sci. Int. Genet. 45:102230. doi: 10.1016/j.fsigen.2019.102230
Eigelsbach, H. T., and Downs, C. M. (1961). Prophylactic effectiveness of live and killed tularemia vaccines. I. Production of vaccine and evaluation in the white mouse and guinea pig. J. Immunol. 87, 415–425.
Ellis, J., Oyston, P. C., Green, M., and Titball, R. W. (2002). Tularemia. Clin. Microbiol. Rev. 15, 631–646.
Fàbrega, A., Soto, S. M., Ballesté-Delpierre, C., Fernández-Orth, D., Jiménez, De Anta, M. T., et al. (2014). Impact of quinolone-resistance acquisition on biofilm production and fitness in Salmonella enterica. J. Antimicrob. Chemother. 69, 1815–1824. doi: 10.1093/jac/dku078
Funatsu, G., and Wittmann, H. G. (1972). Ribosomal proteins: XXXIII. Location of amino-acid replacements in protein S12 isolated from Escherichia coli mutants resistant to streptomycin. J. Mol. Biol. 68, 547–550.
Fuzi, M., Rodriguez Baño, J., and Toth, A. (2020). Global evolution of pathogenic bacteria with extensive use of fluoroquinolone agents. Front. Microbiol. 11:271. doi: 10.3389/fmicb.2020.00271
Fuzi, M., Szabo, D., and Csercsik, R. (2017). Double-serine fluoroquinolone resistance mutations advance major international clones and lineages of various multi-drug resistant bacteria. Front. Microbiol. 8:2261. doi: 10.3389/fmicb.2017.02261
Gil, H., Platz, G. J., Forestal, C. A., Monfett, M., Bakshi, C. S., Sellati, T. J., et al. (2006). Deletion of TolC orthologs in Francisella tularensis identifies roles in multidrug resistance and virulence. Proc. Natl. Acad. Sci. U.S.A. 103, 12897–12902. doi: 10.1073/pnas.0602582103
Gutierrez, B., Escudero, J. A., San Millan, A., Hidalgo, L., Carrilero, L., Ovejero, C. M., et al. (2012). Fitness cost and interference of Arm/Rmt aminoglycoside resistance with the RsmF housekeeping methyltransferases. Antimicrob. Agents Chemother. 56, 2335–2341. doi: 10.1128/aac.06066-11
Hall, C. W., and Mah, T. F. (2017). Molecular mechanisms of biofilm-based antibiotic resistance and tolerance in pathogenic bacteria. FEMS Microbiol. Rev. 41, 276–301. doi: 10.1093/femsre/fux010
Heine, H. S., Miller, L., Halasohoris, S., and Purcell, B. K. (2017). In vitro antibiotic susceptibilities of Francisella tularensis determined by broth microdilution following CLSI methods. Antimicrob. Agents Chemother. 61, e612–e617.
Heinemann, J. A. (1999). How antibiotics cause antibiotic resistance. Drug Discov. Today 4, 72–79. doi: 10.1016/s1359-6446(98)01294-x
Hepburn, M. J., and Simpson, A. J. (2008). Tularemia: current diagnosis and treatment options. Expert Rev. Anti Infect. Ther. 6, 231–240. doi: 10.1586/14787210.6.2.231
Hooper, D. C. (2001). Mechanisms of action of antimicrobials: focus on fluoroquinolones. Clin. Infect. Dis. 32(Suppl. 1), S9–S15.
Hornick, R. B., and Eigelsbach, H. T. (1966). Aerogenic immunization of man with live Tularemia vaccine. Bacteriol. Rev. 30, 532–538. doi: 10.1128/mmbr.30.3.532-538.1966
Ireland, P. M., Bullifent, H. L., Senior, N. J., Southern, S. J., Yang, Z. R., Ireland, R. E., et al. (2019). Global analysis of genes essential for Francisella tularensis Schu S4 growth in vitro and for fitness during competitive infection of Fischer 344 rats. J. Bacteriol. 201:e00630-18.
Jaing, C. J., Mcloughlin, K. S., Thissen, J. B., Zemla, A., Gardner, S. N., Vergez, L. M., et al. (2016). Identification of genome-wide mutations in ciprofloxacin-resistant F. tularensis LVS using whole genome tiling arrays and next generation sequencing. PLoS One 11:e0163458. doi: 10.1371/journal.pone.0163458
Jellison, W. L., and Parker, R. R. (1945). Rodents, rabbits and tularemia in North America: some zoological and epidemiological considerations. Am. J. Trop. s1-25, 349–362. doi: 10.4269/ajtmh.1945.s1-25.349
Johansson, A., Berglund, L., Sjostedt, A., and Tarnvik, A. (2001). Ciprofloxacin for treatment of tularemia. Clin. Infect. Dis. 33, 267–268. doi: 10.1086/321825
Jones, R. M., Nicas, M., Hubbard, A., Sylvester, M. D., and Reingold, A. (2005). The infectious dose of Francisella tularensis (tularemia). Appl. Biosaf. 10, 227–239.
Kingry, L. C., and Petersen, J. M. (2014). Comparative review of Francisella tularensis and Francisella novicida. Front. Cell Infect. Microbiol. 4:35. doi: 10.3389/fcimb.2014.00035
Kopping, E. J., Doyle, C. R., Sampath, V., and Thanassi, D. G. (2019). Contributions of TolC orthologs to Francisella tularensis Schu S4 multidrug resistance, modulation of host cell responses, and virulence. Infect. Immun. 87:e00823-18. doi: 10.1128/IAI.00823-18
Kumar, S., Lekshmi, M., Parvathi, A., Ojha, M., Wenzel, N., and Varela, M. F. (2020). Functional and structural roles of the major facilitator superfamily bacterial multidrug efflux pumps. Microorganisms 8:266. doi: 10.3390/microorganisms8020266
La Scola, B., Elkarkouri, K., Li, W., Wahab, T., Fournous, G., Rolain, J. M., et al. (2008). Rapid comparative genomic analysis for clinical microbiology: the Francisella tularensis paradigm. Genome Res. 18, 742–750. doi: 10.1101/gr.071266.107
Langmead, B., and Salzberg, S. L. (2012). Fast gapped-read alignment with Bowtie 2. Nat. Methods 9, 357–359. doi: 10.1038/nmeth.1923
Larson, C. L., Wicht, W., and Jellison, W. L. (1955). A new organism resembling P. tularensis isolated from water. Publ. Health Rep. 70, 253–258. doi: 10.2307/4589039
Larsson, P., Oyston, P. C., Chain, P., Chu, M. C., Duffield, M., Fuxelius, H. H., et al. (2005). The complete genome sequence of Francisella tularensis, the causative agent of tularemia. Nat. Genet. 37, 153–159.
LeBel, M. (1988). Ciprofloxacin: chemistry, mechanism of action, resistance, antimicrobial spectrum, pharmacokinetics, clinical trials, and adverse reactions. Pharmacotherapy 8, 3–33. doi: 10.1002/j.1875-9114.1988.tb04058.x
Loveless, B. M., Yermakova, A., Christensen, D. R., Kondig, J. P., Heine, H. S. III, Wasieloski, L. P., et al. (2010). Identification of ciprofloxacin resistance by SimpleProbe, high resolution melt and pyrosequencing nucleic acid analysis in biothreat agents: Bacillus anthracis, Yersinia pestis and Francisella tularensis. Mol. Cell Probes 24, 154–160. doi: 10.1016/j.mcp.2010.01.003
Luzzatto, L., Apirion, D., and Schlessinger, D. (1968). Mechanism of action of streptomycin in E. coli: interruption of the ribosome cycle at the initiation of protein synthesis. Proc. Natl. Acad. Sci. U.S.A. 60, 873–880. doi: 10.1073/pnas.60.3.873
Mahajan, U. V., Gravgaard, J., Turnbull, M., Jacobs, D. B., and Mcnealy, T. L. (2011). Larval exposure to Francisella tularensis LVS affects fitness of the mosquito Culex quinquefasciatus. FEMS Microbiol. Ecol. 78, 520–530. doi: 10.1111/j.1574-6941.2011.01182.x
Marcusson, L. L., Frimodt-Møller, N., and Hughes, D. (2009). Interplay in the selection of fluoroquinolone resistance and bacterial fitness. PLoS Pathog. 5:e1000541. doi: 10.1371/journal.ppat.1000541
Margolis, J. J., El-Etr, S., Joubert, L. M., Moore, E., Robison, R., Rasley, A., et al. (2010). Contributions of Francisella tularensis subsp. novicida chitinases and Sec secretion system to biofilm formation on chitin. Appl. Environ. Microbiol. 76, 596–608. doi: 10.1128/aem.02037-09
Martin, M. (2011). Cutadapt removes adapter sequences from high-throughput sequencing reads. EMBnet J. 17:3.
Maruri, F., Sterling, T. R., Kaiga, A. W., Blackman, A., Van Der Heijden, Y. F., Mayer, C., et al. (2012). A systematic review of gyrase mutations associated with fluoroquinolone-resistant Mycobacterium tuberculosis and a proposed gyrase numbering system. J. Antimicrob. Chemother. 67, 819–831. doi: 10.1093/jac/dkr566
Matrat, S., Aubry, A., Mayer, C., Jarlier, V., and Cambau, E. (2008). Mutagenesis in the alpha3alpha4 GyrA helix and in the Toprim domain of GyrB refines the contribution of Mycobacterium tuberculosis DNA gyrase to intrinsic resistance to quinolones. Antimicrob. Agents Chemother. 52, 2909–2914. doi: 10.1128/aac.01380-07
McKenna, A., Hanna, M., Banks, E., Sivachenko, A., Cibulskis, K., Kernytsky, A., et al. (2010). The Genome Analysis Toolkit: a MapReduce framework for analyzing next-generation DNA sequencing data. Genome Res. 20, 1297–1303. doi: 10.1101/gr.107524.110
Miskinyte, M., and Gordo, I. (2013). Increased survival of antibiotic-resistant Escherichia coli inside macrophages. Antimicrob. Agents Chemother. 57, 189–195. doi: 10.1128/aac.01632-12
Morner, T. (1992). The ecology of tularaemia. Rev. Sci. Tech. 11, 1123–1130. doi: 10.20506/rst.11.4.657
Müller, W., Hotzel, H., Otto, P., Karger, A., Bettin, B., Bocklisch, H., et al. (2013). German Francisella tularensis isolates from European brown hares (Lepus europaeus) reveal genetic and phenotypic diversity. BMC Microbiol. 13:61. doi: 10.1186/1471-2180-13-61
Nishimura, K., Hosaka, T., Tokuyama, S., Okamoto, S., and Ochi, K. (2007). Mutations in rsmG, encoding a 16S rRNA methyltransferase, result in low-level streptomycin resistance and antibiotic overproduction in Streptomyces coelicolor A3(2). J. Bacteriol. 189, 3876–3883. doi: 10.1128/jb.01776-06
Oyston, P. C., and Quarry, J. E. (2005). Tularemia vaccine: past, present and future. Antonie Van Leeuwenhoek 87, 277–281. doi: 10.1007/s10482-004-6251-7
Parker, J. (2001). “Streptomycin,” in Encyclopedia of Genetics, eds S. Brenner and J. H. Miller (New York, NY: Academic Press), 1890–1891.
Pechous, R. D., Mccarthy, T. R., and Zahrt, T. C. (2009). Working toward the future: insights into Francisella tularensis pathogenesis and vaccine development. Microbiol. Mol. Biol. Rev. 73, 684–711. doi: 10.1128/mmbr.00028-09
Pérez, N., Johnson, R., Sen, B., and Ramakrishnan, G. (2016). Two parallel pathways for ferric and ferrous iron acquisition support growth and virulence of the intracellular pathogen Francisella tularensis Schu S4. MicrobiologyOpen 5, 453–468. doi: 10.1002/mbo3.342
Perez-Castrillon, J. L., Bachiller-Luque, P., Martin-Luquero, M., Mena-Martin, F. J., and Herreros, V. (2001). Tularemia epidemic in northwestern Spain: clinical description and therapeutic response. Clin. Infect. Dis. 33, 573–576. doi: 10.1086/322601
Pumbwe, L., and Piddock, L. J. V. (2002). Identification and molecular characterisation of CmeB, a Campylobacter jejuni multidrug efflux pump. FEMS Microbiol. Lett. 206, 185–189. doi: 10.1111/j.1574-6968.2002.tb11007.x
Rohmer, L., Brittnacher, M., Svensson, K., Buckley, D., Haugen, E., Zhou, Y., et al. (2006). Potential source of Francisella tularensis live vaccine strain attenuation determined by genome comparison. Infect. Immun. 74, 6895–6906. doi: 10.1128/iai.01006-06
Saslaw, S., Eigelsbach, H. T., Prior, J. A., Wilson, H. E., and Carhart, S. (1961). Tularemia vaccine study. II. Respiratory challenge. Arch. Intern. Med. 107, 702–714. doi: 10.1001/archinte.1961.03620050068007
Schmieder, R., and Edwards, R. (2011). Quality control and preprocessing of metagenomic datasets. Bioinformatics 27, 863–864. doi: 10.1093/bioinformatics/btr026
Schuldiner, S. (2018). The Escherichia coli effluxome. Res. Microbiol. 169, 357–362. doi: 10.1016/j.resmic.2018.02.006
Sharma, D., Cukras, A. R., Rogers, E. J., Southworth, D. R., and Green, R. (2007). Mutational analysis of S12 protein and implications for the accuracy of decoding by the ribosome. J. Mol. Biol. 374, 1065–1076. doi: 10.1016/j.jmb.2007.10.003
Shirtliff, M. E., Mader, J. T., and Camper, A. K. (2002). Molecular interactions in biofilms. Chem. Biol. 9, 859–871. doi: 10.1016/s1074-5521(02)00198-9
Siebert, C., Lindgren, H., Ferré, S., Villers, C., Boisset, S., Perard, J., et al. (2019). Francisella tularensis: FupA mutation contributes to fluoroquinolone resistance by increasing vesicle secretion and biofilm formation. Emerg. Microbes Infect. 8, 808–822. doi: 10.1080/22221751.2019.1615848
Siebert, C., Villers, C., Pavlou, G., Touquet, B., Yakandawala, N., Tardieux, I., et al. (2020). Francisella novicida and F. philomiragia biofilm features conditionning fitness in spring water and in presence of antibiotics. PLoS One 15:e0228591. doi: 10.1371/journal.pone.0228591
Springer, B., Kidan, Y. G., Prammananan, T., Ellrott, K., Böttger, E. C., and Sander, P. (2001). Mechanisms of streptomycin resistance: selection of mutations in the 16S rRNA gene conferring resistance. Antimicrob. Agents Chemother. 45, 2877–2884. doi: 10.1128/aac.45.10.2877-2884.2001
Sreevatsan, S., Pan, X., Stockbauer, K. E., Williams, D. L., Kreiswirth, B. N., and Musser, J. M. (1996). Characterization of rpsL and rrs mutations in streptomycin-resistant Mycobacterium tuberculosis isolates from diverse geographic localities. Antimicrob. Agents Chemother. 40, 1024–1026. doi: 10.1128/aac.40.4.1024
Sutera, V., Levert, M., Burmeister, W. P., Schneider, D., and Maurin, M. (2014). Evolution toward high-level fluoroquinolone resistance in Francisella species. J. Antimicrob. Chemother. 69, 101–110. doi: 10.1093/jac/dkt321
Toivonen, J. M., Boocock, M. R., and Jacobs, H. T. (1999). Modelling in Escherichia coli of mutations in mitoribosomal protein S12: novel mutant phenotypes of rpsL. Mol. Microbiol. 31, 1735–1746. doi: 10.1046/j.1365-2958.1999.01307.x
Tomaso, H., Hotzel, H., Otto, P., Myrtennäs, K., and Forsman, M. (2017). Antibiotic susceptibility in vitro of Francisella tularensis subsp. holarctica isolates from Germany. J. Antimicrob. Chemother. 72, 2539–2543. doi: 10.1093/jac/dkx182
van Hoek, M. L. (2013). Biofilms: an advancement in our understanding of Francisella species. Virulence 4, 833–846. doi: 10.4161/viru.27023
Vila, J., Ruiz, J., Goñi, P., and De Anta, M. T. (1996). Detection of mutations in parC in quinolone-resistant clinical isolates of Escherichia coli. Antimicrob. Agents Chemother. 40, 491–493. doi: 10.1128/aac.40.2.491
Vila, J., and Soto, S. M. (2012). Salicylate increases the expression of marA and reduces in vitro biofilm formation in uropathogenic Escherichia coli by decreasing type 1 fimbriae expression. Virulence 3, 280–285. doi: 10.4161/viru.19205
Vuilleumier, S., Yearsley, J. M., and Perrin, N. (2008). The fixation of locally beneficial alleles in a metapopulation. Genetics 178:467. doi: 10.1534/genetics.107.081166
Wong, A., Rodrigue, N., and Kassen, R. (2012). Genomics of adaptation during experimental evolution of the opportunistic pathogen Pseudomonas aeruginosa. PLoS Genet. 8:e1002928. doi: 10.1371/journal.pgen.1002928
Yan, J., and Bassler, B. L. (2019). Surviving as a community: antibiotic tolerance and persistence in bacterial biofilms. Cell Host Microbe 26, 15–21. doi: 10.1016/j.chom.2019.06.002
Yoshida, H., Bogaki, M., Nakamura, M., and Nakamura, S. (1990). Quinolone resistance-determining region in the DNA gyrase gyrA gene of Escherichia coli. Antimicrob. Agents Chemother. 34, 1271–1272. doi: 10.1128/aac.34.6.1271
Keywords: Francisella, Francisella novicida, LVS, antimicrobial resistance, streptomycin, ciprofloxacin, tularemia, biofilm
Citation: Biot FV, Bachert BA, Mlynek KD, Toothman RG, Koroleva GI, Lovett SP, Klimko CP, Palacios GF, Cote CK, Ladner JT and Bozue JA (2020) Evolution of Antibiotic Resistance in Surrogates of Francisella tularensis (LVS and Francisella novicida): Effects on Biofilm Formation and Fitness. Front. Microbiol. 11:593542. doi: 10.3389/fmicb.2020.593542
Received: 10 August 2020; Accepted: 08 October 2020;
Published: 30 October 2020.
Edited by:
Miklos Fuzi, Semmelweis University, HungaryReviewed by:
Joseph Horzempa, West Liberty University, United StatesDouglas S Reed, University of Pittsburgh, United States
Copyright © 2020 Biot, Bachert, Mlynek, Toothman, Koroleva, Lovett, Klimko, Palacios, Cote, Ladner and Bozue. This is an open-access article distributed under the terms of the Creative Commons Attribution License (CC BY). The use, distribution or reproduction in other forums is permitted, provided the original author(s) and the copyright owner(s) are credited and that the original publication in this journal is cited, in accordance with accepted academic practice. No use, distribution or reproduction is permitted which does not comply with these terms.
*Correspondence: Joel A. Bozue, Sm9lbC5BLkJvenVlLmNpdkBtYWlsLm1pbA==
†These authors have contributed equally to this work
‡Present address: Jason T. Ladner, The Pathogen and Microbiome Institute, Northern Arizona University, Flagstaff, AZ, United States