- 1Shanghai Universities Key Laboratory of Marine Animal Taxonomy and Evolution, Key Laboratory of Exploration and Utilization of Aquatic Genetic Resources, National Demonstration Center for Experimental Fisheries Science Education, Shanghai Ocean University, Shanghai, China
- 2Department of Evolution, Ecology and Behaviour, Biosciences Building, University of Liverpool, Liverpool, United Kingdom
- 3Institute of Zoology, Zoological Society of London, London, United Kingdom
- 4MRC Centre for Global Infectious Disease Analysis, Imperial College London, London, United Kingdom
Free-living eukaryotic microbes may reduce animal diseases. We evaluated the dynamics by which micrograzers (primarily protozoa) apply top-down control on the chytrid Batrachochytrium dendrobatidis (Bd) a devastating, panzootic pathogen of amphibians. Although micrograzers consumed zoospores (∼3 μm), the dispersal stage of chytrids, not all species grew monoxenically on zoospores. However, the ubiquitous ciliate Tetrahymena pyriformis, which likely co-occurs with Bd, grew at near its maximum rate (r = 1.7 d–1). A functional response (ingestion vs. prey abundance) for T. pyriformis, measured using spore-surrogates (microspheres) revealed maximum ingestion (Imax) of 1.63 × 103 zoospores d–1, with a half saturation constant (k) of 5.75 × 103 zoospores ml–1. Using these growth and grazing data we developed and assessed a population model that incorporated chytrid-host and micrograzer dynamics. Simulations using our data and realistic parameters obtained from the literature suggested that micrograzers could control Bd and potentially prevent chytridiomycosis (defined as 104 sporangia host–1). However, simulated inferior micrograzers (0.7 × Imax and 1.5 × k) did not prevent chytridiomycosis, although they ultimately reduced pathogen abundance to below levels resulting in disease. These findings indicate how micrograzer responses can be applied when modeling disease dynamics for Bd and other zoosporic fungi.
Introduction
Although the traditional microbial food web (i.e., prokaryotes and protists, sensu Azam et al. (1983) is well-established as a driver of aquatic productivity (Fenchel, 1987), fungi are only now being appreciated as integral aquatic microbes. A dominant group of fungi, the chytrids, are parasites of phytoplankton, zooplankton, and vertebrates (Kagami et al., 2014), and zoospores, the chytrid dispersal stage, are an appropriate size for protozoan grazers and are highly nutritious, containing essential fatty acids and sterols (Fenchel, 1987; Gleason et al., 2008; Kagami et al., 2014; Gerphagnon et al., 2018; Frenken et al., 2020a). Hence, through top-down control micrograzers within the microbial food web have the potential to reduce the likelihood or severity of, or even prevent, disease outbreaks caused by these pathogens (Kagami et al., 2014; Grossart and Rojas-Jimenez, 2016; Frenken et al., 2019). Here, by developing and parameterizing a population model we explore the dynamics by which microbial grazers may control the chytrid Batrachochytrium dendrobatidis, a panzootic pathogen of amphibians that is argued to have caused the greatest loss of biodiversity attributed to any disease (Scheele et al., 2019).
Batrachochytrium dendrobatidis (henceforth, Bd) infects amphibian hosts through the dispersal of motile, 3–5 μm zoospores (Figure 1). The environmental pool of zoospores is instrumental in driving infection dynamics, as these can accrue in a dose-dependent manner (Garner et al., 2009), with for some hosts the size of the zoospore pool influencing long-term consequences for population survival or extinction (Briggs et al., 2010). It follows that processes that reduce the zoospore-pool should reduce the probability and intensity of disease outbreaks. Consumption of zoospores by naturally occurring micrograzers has been suggested to result in losses sufficient to reduce infections. Experiments show that some micrograzers may reduce the likelihood of Bd infections, and field data indicate a negative relationship between potential-grazer abundance and both the prevalence of infection and host mortality from disease (Woodhams et al., 2008; Buck et al., 2011; Gleason et al., 2014; Schmeller et al., 2014). There is now a need to build on these observations and investigate in more depth the dynamics by which consumers may impact on Bd.
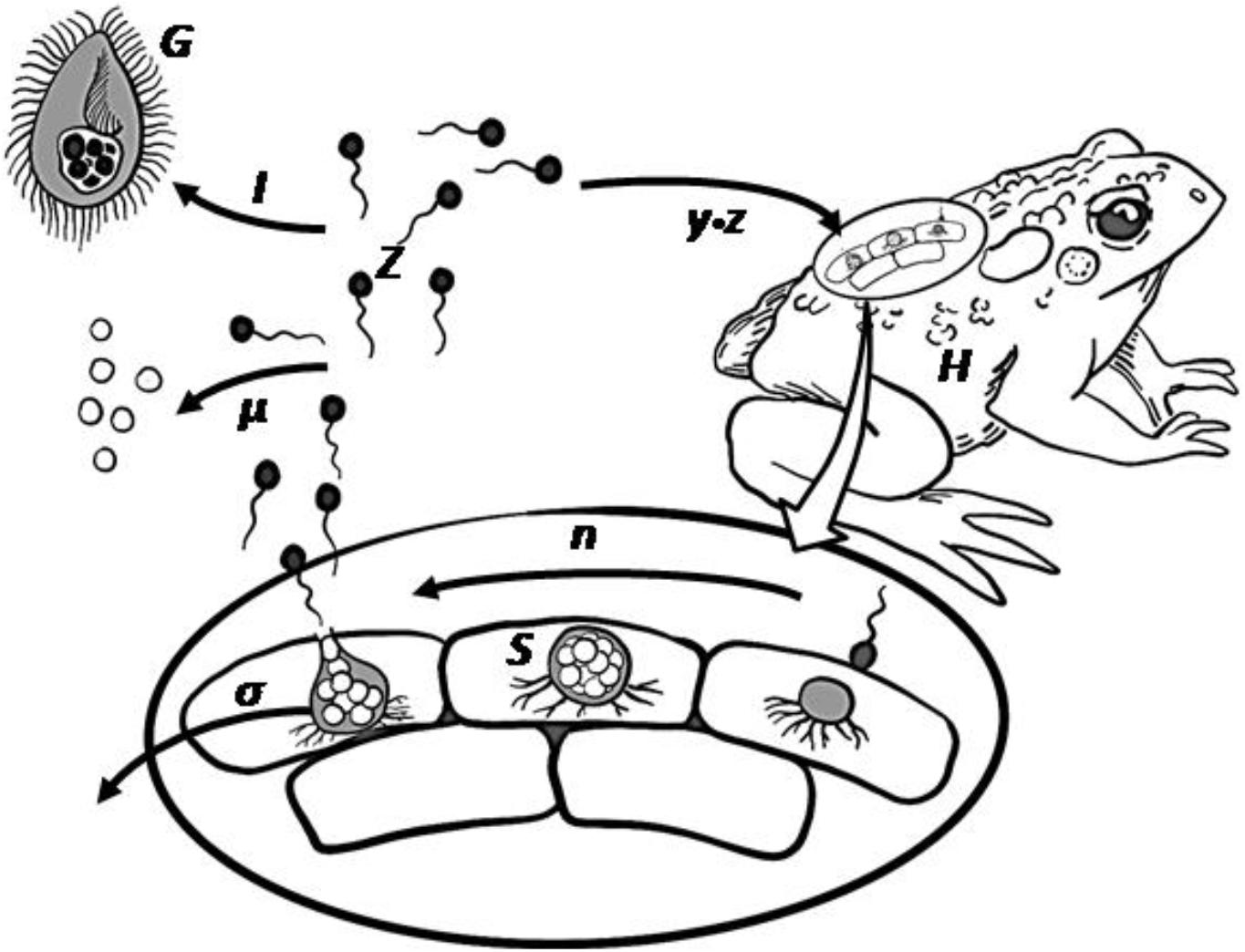
Figure 1. Bd infectious life cycle including the potential grazing pressure by micrograzers. Zoospores (Z) move using a flagellum, and on contact infect the amphibian host (H). Within the host epidermal cells, a spore then forms a sporangium (S) that releases further zoospores through asexual reproduction (n), after which the sporangium dies (σ). Released zoospores may die naturally (μ) or be ingested (I) by micrograzers (G).
Studies investigating the spores-grazer link, the “mycoloop,” have shown that multiple micrograzers can survive and grow on chytrid zoospores, including rotifers and copepods (Kagami et al., 2007, 2014; Schmeller et al., 2014). However, most work to date on the consumption of Bd zoospores has focused on large zooplankton, especially cladocerans (Buck et al., 2011; Hamilton et al., 2012; Searle et al., 2013; Maier et al., 2016). However, experiments on cladocerans have used unrealistically high micrograzer abundances (>10–100 times higher than natural levels), and at natural levels large zooplankton seem to have little impact on Bd infections (Buck et al., 2016). Micrograzers, in contrast, are abundant in shallow waters and are often near the bottom of ponds where infected hosts (e.g., benthic, grazing tadpoles) spend time resting and grazing on the substrate (Dionne, 1969; Beiswenger, 1977; Thurnheer and Reyer, 2001). Furthermore, as many protozoa have generation times on the order of hours, by reproducing asexually when zoospores are abundant, micrograzer populations may increase several fold, consuming zoospores as they are released from the host. We, therefore, argue that protozoa will be more important than cladocerans in reducing the abundance of chytrid zoospores. This is supported by Schmeller et al. (2014) who, using mesocosms, showed the ciliate Paramecium can significantly reduce the number of hosts infected with Bd by up to 65% when it is introduced at naturally occurring abundances.
We also suggest that the main impact of micrograzers on Bd spore-load will be in the water directly surrounding the host, where zoospores will be most abundant. Field studies suggest that in water bodies where Bd occurs, zoospore densities in the water column are low, ranging from ∼0.5 to 500 L–1 (Kirshtein et al., 2007; Walker et al., 2007). In contrast, zoospores are shed from hosts at up to 250 zoospores min–1 (Maguire et al., 2016), surviving only ∼1–3 days (Woodhams et al., 2008). Additionally, zoospores mostly disperse on the order of 1 cm (Piotrowski et al., 2004), demonstrating chemotaxis toward keratinised skin over this distance (Moss et al., 2008; Garmyn et al., 2012). Although these laboratory-based rates will be dependent on environmental factors such as temperature (Woodhams et al., 2008), they suggest that the limited movement and survival of the rapidly produced zoospores will lead to dense aggregations in localized regions around the host. Recognizing the likelihood of these local abundances and the well-established density-dependent feeding and growth responses of micrograzers (Fenchel, 1987), in this study we focused attention on the impact of micrograzers on Bd dynamics around the host. We achieved this by: first, investigating a range of potential micrograzers, determining which survived on a diet of only Bd zoospores and if they grew at their maximum rates when fed saturating prey concentrations – we then concentrated on those species that grew; second, measuring ingestion and growth rates of a common, widely distributed species that thrives on Bd; and third, using these data, developing and exploring a model that couples the Bd life-cycle with micrograzer-control on zoospores. In doing so, we indicate the dynamics by which micrograzers may reduce Bd populations – potentially preventing disease – and provide a mechanism by which chytrid-diseases can be incorporated into microbial food web models.
Materials and Methods
Culture Maintenance
Batrachochytrium dendrobatidis (Bd) cultures (strain #262 IA 9′13, Imperial College London) were maintained at 18°C (at which all experiments were conducted) on H-broth medium (500 mL: 5 g Tryptone and 16 g glucose) and were regularly transferred (every ∼5 days) to maintain exponential growth. Bacterial growth was prevented by the addition of antibiotics (Ampicillin at 100 μg ml–1; Kanamycin at 50 μg ml–1; Chloramphenicol at 34 μg ml–1). Note, antibiotics may have small detrimental effects on protozoan swimming speed (Wu et al., 1996), but we assume here that under saturating food conditions they will have a negligible impact on growth. Micrograzers were obtained from Sciento (Manchester, United Kingdom): the ciliates Blepharisma sp., Oxytricha sp., Paramecium aurelia, Paramecium caudatum, Stentor coeruleus, Tetrahymena pyriformis, and Urocentrum turbo and the rotifers Brachionus calyciflorus and Philodina sp. Tetrahymena pyriformis was maintained axenically for extended periods on H-broth. All other species were maintained prior to experiments on a natural assemblage of bacteria in Chalkley’s medium enriched with cereal grains, as provided by Sciento (Chalkley, 1930).
Assessing Growth of Micrograzer Species on Bd Zoospores
We tested the hypothesis that Bd zoospores were of nutritional benefit to the micrograzer. To do so, we compared potential maximum growth (i.e., at expected saturating prey concentrations, that may be reached near the host Weisse, 2017) on zoospores to when no food was available (i.e., when grazers would be starving). We also compared growth on zoospores to maximal rate of growth of the micrograzers, obtained from literature estimates (Figure 2).
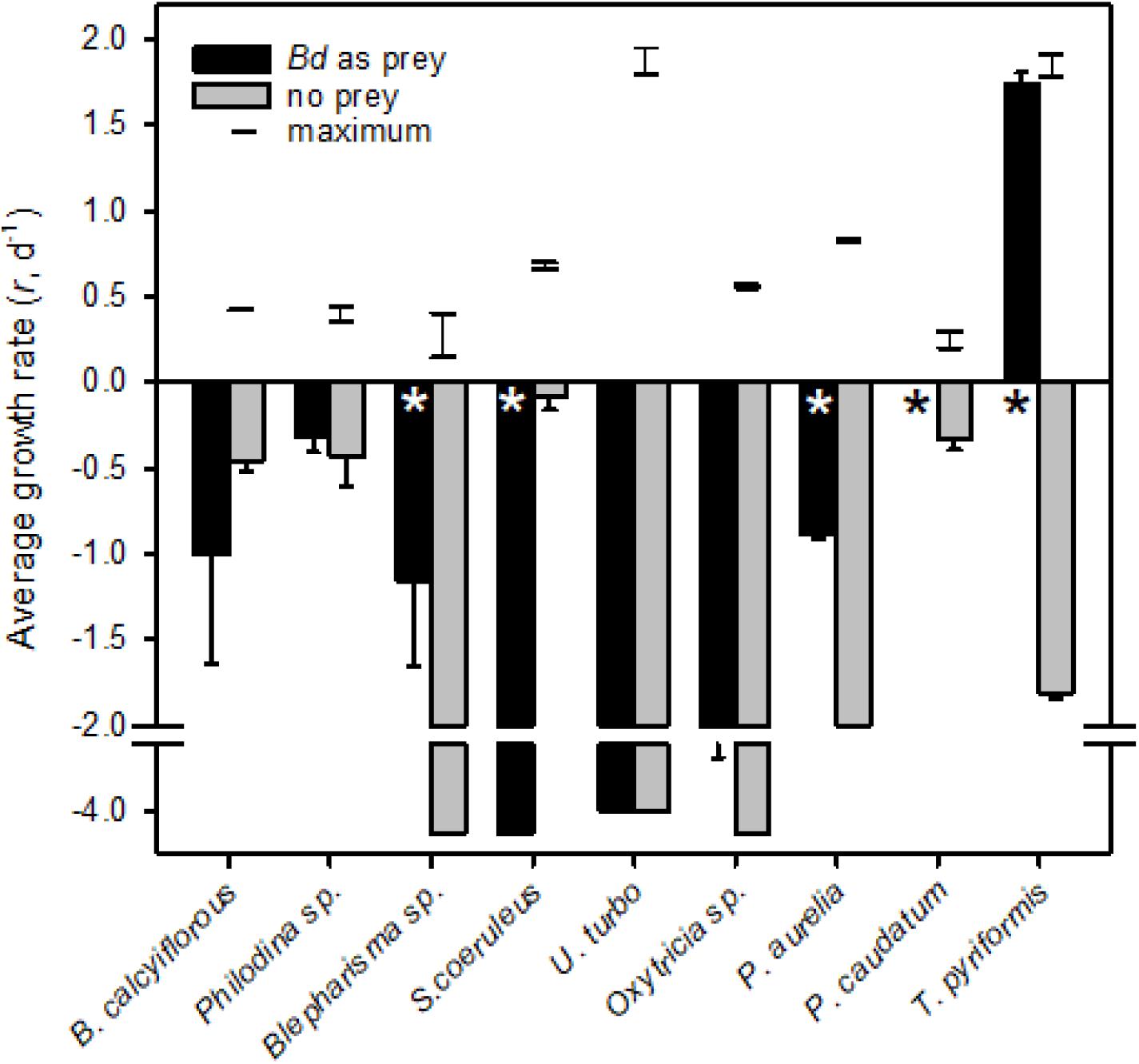
Figure 2. Average growth rates (r, d− 1) for micrograzers fed Bd (black) or no prey (gray); error bars are one standard error, and * indicates where significant (α = 0.05) differences occurred between fed and unfed treatments. All grazers exhibited a constant growth or death rate except from the two Paramecium species which we expand on in Figure 3. Note that for P. caudatum growth rate was zero when fed Bd. The horizontal lines connected by a vertical line represent the range of predicted maximum growth rate (i.e., food saturated on suitable prey) at 18°C, for each species. Maximum growth rate data were obtained from various sources at a range of temperatures (Kasturi Bai et al., 1969; Finlay, 1977; Taylor, 1978; Jackson and Berger, 1984; Inamori et al., 1990; Weithoff and Walz, 1995; Weisse et al., 2013; Salt et al., 2017) and were converted to rates at 18°C by two methods, either assuming a Q10 of 2 or that growth rate varies linearly with temperature at a rate of 0.07 r (d− 1) °C− 1 (Montagnes et al., 2003).
Prior to introducing micrograzers, Bd was isolated from its growth medium to ensure that the medium was not a source of nutrients for the micrograzers. To do so, a Bd suspension (in exponential phase) was centrifuged (50 ml tubes, 5000 rpm, 6 min), the supernatant removed, and the pellet resuspended in autoclaved Volvic® mineral water to a concentration >1.50 × 105 ml–1 (determined microscopically). Resuspended cultures were examined microscopically (40×) to ensure zoospores were motile. Bacterial growth was prevented with antibiotics, as above.
To assess growth rate, we followed our previous methods (Montagnes, 1996). Micrograzers (9 species) were passed 5 times through autoclaved Volvic® water to remove bacteria. Then 5 to 8 individuals, dependent on micrograzer size, were added to a 10 ml well containing the Bd suspension. Parallel treatments containing only sterile Volvic® water were used to assess mortality rate in the absence of prey, i.e., starvation rates. All treatments (i.e., species incubations with or without Bd) were replicated in triplicate (i.e., three 10 ml wells). To assess growth rate (r, d–1), the number of gazers in each well was determined microscopically, using a dissection microscope, after 2 or 3 days (depending on the observed change in abundance). Then, new Bd suspensions were prepared (as above), and micrograzers were transferred to these, maintaining Bd abundance. If micrograzer numbers decreased (net death occurred) over the incubation, then all individuals were transferred, but if numbers increased (net growth occurred) then the initial number was transferred. Cells death was estimated by their disappearance (Montagnes, 1996). This procedure was continued for 14 days or until all micrograzers had died. Cultures were routinely checked under a compound microscope (100×), to ensure there was no bacterial contamination.
When numbers increased between transfers, growth rate (r, d–1) was determined over each incubation period, as r = ln(Nt/N0)/t, where N0 and Nt are the micrograzer abundance on the initial and final day, respectively, and t is the incubation period (2 or 3 days); to determine growth rate across all transfers (up to 14 days), the average of these was obtained. When micrograzer numbers decreased between transfers, mortality rates (–r, d–1) were determined as slope of ln numbers over the entire incubation period. To assess if growth (or death) rate differed between treatments (i.e., with or without Bd) a two tailed t-test was conducted (α = 0.05).
Quantifying the Functional Response (Ingestion vs. Prey Abundance) of Tetrahymena Grazing on Bd
Our study focused on Tetrahymena pyriformis as: (1) it grew rapidly on Bd zoospores (see section “Results”) and therefore clearly consumed and assimilated zoospores; (2) it is a model organism for which there are substantial data (see section “Discussion”); and (3) it is common, globally, in habitats where Bd may occur (see section “Discussion”). Prior to determining ingestion rate, T. pyriformis was acclimated with zoospores for >10 h. To do so, the ciliates were first removed from H-broth by centrifugation (50 ml tubes, 8000 rpm, 8 min) and then resuspended in 10 ml of autoclaved Volvic® water. To obtain only zoospores, a centrifuged Bd culture (as above) was filtered through a 5 μm Nitex® mesh. Zoospores were then added to the water containing ciliates, to a total volume of 20 ml (resulting in ∼106 zoospores ml–1), with antibiotics (as above). This acclimation had no negative effects on the ciliates: after 10 h, zoospore abundance had substantially decreased and ciliate abundance increased (indicating the ciliates were feeding and growing), the ciliates behaved similarly to when grown on H-broth (i.e., similar swimming pattern), and their cell size and shape were similar to when grown on H-broth.
To determine ingestion rate on spore-sized particles, 3 μm fluorescent polymer microspheres (henceforth beads, Fluoro-MaxTM, Thermo Fisher Scientific, United States) acted as a surrogate for Bd zoospores which are 3–5 μm (Berger et al., 2005). Bead concentrations, ∼8 × 103 ml–1 to 106 ml–1 (see section “Results”), were prepared in autoclaved Volvic® water and vortexed prior to use, ensuring mono-dispersion. An aliquot (0.5 ml) of the acclimated ciliate culture (>30 micrograzers) was added to 1 ml of Volvic® water with beads, at various concentrations [with more measurements at low abundances (Montagnes and Berges, 2004), see section “Results”], and incubated for 5 or 10 min, depending on the bead concentration (preliminary experiments deemed these to be appropriate incubation periods). Incubations were terminated by fixing cells with ethanol (final concertation 70%). The average number of beads ingested per ciliate (>30 cells) was determined via fluorescent microscopy and was subsequently used to determine ingestion rate (I, prey d–1) at each prey concentration.
The relationship between ingestion rate and zoospore abundance (Z ml–1), was determined by fitting a Type II functional response to the data: I = Imax∗Z/(k + Z), where Imax (Z min–1) is the maximum ingestion rate and k is the half saturation constant (Z ml–1). The response was fit using the Marquardt-Levenberg algorithm (SigmaPlot, Systat, Germany); this algorithm is appropriate for describing such biological data sets (Berges et al., 1994).
Modeling Micrograzer Impacts on Bd Populations
To assess the dynamics by which grazing pressure impacts on Bd infection populations we developed and applied the following model that couples a reduced version of the Bd-load model (Briggs et al., 2010) with the Rosenzweig-MacArthur predator-prey model (Turchin, 2003). Data for T. pyriformis were used to represent micrograzers (see the Discussion for a justification to focus on this species). Following logic outlined in the Introduction, the model describes the infection load on a single host and, as a proxy for the waters surrounding the host, only considers a volume of 10 ml around that host, where zoospores and micrograzers reside. As a metric to predict chytridiomycosis, it assumes that a sporangia load of 104 per host results in host mortality (Briggs et al., 2010), with the recognition that this will vary between hosts and Bd strains (Fisher et al., 2009; Kilpatrick et al., 2010). It does not include reduction of spore numbers by emigration as zoospores are unlikely to disperse far before dying (Piotrowski et al., 2004), and we assume through chemokinesis, micrograzers remain near their food source (Leick and Hellung-Larsen, 1992; Montagnes et al., 2008; Walker et al., 2010). The model is described by the following equations,
where for Eqs. 1, 2, S is the number of sporangia ml–1 (note for per host measurements this value is multiplied by 10); Z is the zoospore abundance (ml–1); y is the per capita spore-host encounter rate; v is the fractional likelihood of spore infection per encounter; σ is the per capita sporangia mortality rate; η is the per sporangia spore-release rate; and μ is the per capita spore mortality rate (see Table 1).
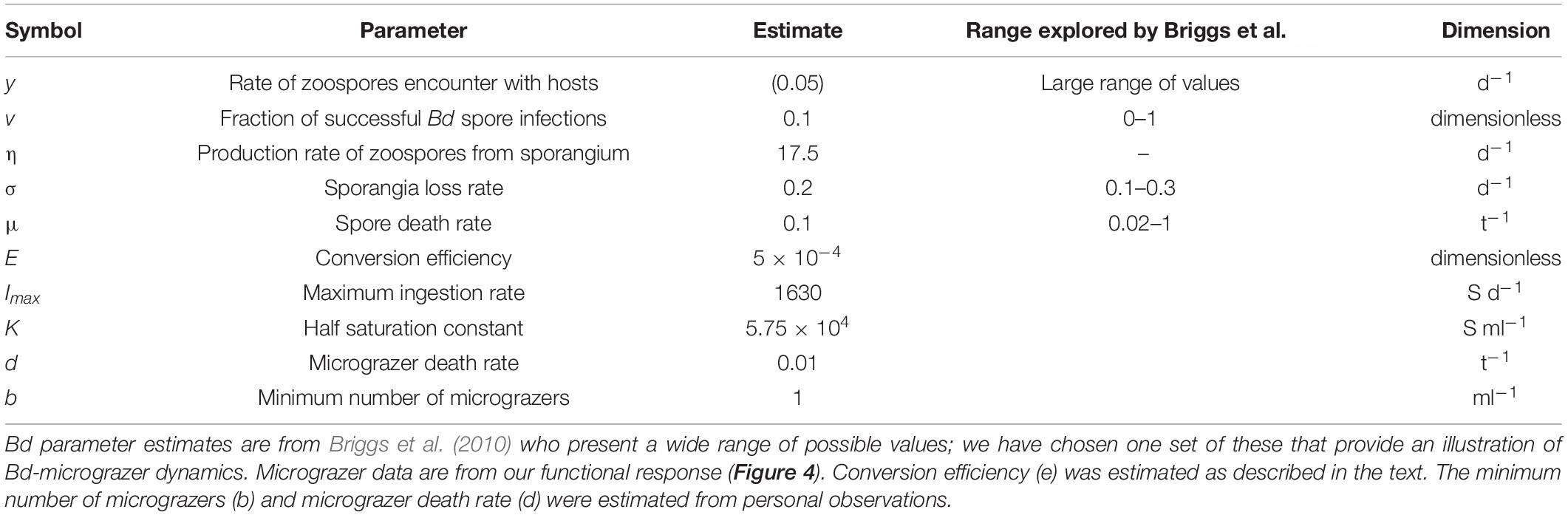
Table 1. Parameters used in the Bd-grazer model (Eq. 1–3), to assess temporal dynamics of Bd and grazer abundances (Figures 5, 6).
Then, Eqs. 2, 3 were coupled to include spore loss by micrograzers (G), where grazing (I) is dictated by the functional response (see Tetrahymena pyriformis ingestion, above); e is the abundance-based conversion efficiency, determined assuming a biomass-based estimate of e is ∼0.1 (Montagnes et al., 2019) and biovolumes of Bd zoospores and T. pyriformis; and d is the micrograzer per capita death rate. We assume here that Bd zoospores are not the only potential food source for the micrograzers, and so incorporate a minimum micrograzer abundance (b) that implicitly assumes that in the absence of zoospores the micrograzer population is maintained by the presence of other potential food sources; hence we model potential increases in micrograzer abundance over and above this minimum, dependent on consumption of Bd zoospores. Estimates of d and b are based on our unpublished observations when Tetrahymena was exposed to poor food conditions. Table 1 summarizes the above parameters and the estimates used.
All model runs (100 d) were initiated with 10 sporangia host–1 (1 sporangium ml–1), 100 zoospores ml–1, and 1 micrograzer ml–1 (again assumed to be the minimal population size, maintained by other resources in the environment). For Bd, we applied parameter values that were within the range explored by Briggs et al. (2010) (Table 1).
We first performed simulations to assess the ability of T. pyriformis to control Bd. Then, we assessed the extent to which micrograzers that are inferior to T. pyriformis could control Bd, through exploration of micrograzer parameter space. Inferior micrograzers had reduced maximum ingestion rate (up to 0.5 × Imax of T. pyriformis) and increased half saturation constant (up to 2 × k of T. pyriformis); see Figure 4 for an indication of the range of these responses. To quantify the impact of micrograzers on Bd, we examined the maximum abundance (over the 100 days) and the abundances at 50 and 100 days of S, Z, and G.
Results
Assessing Growth of Micrograzer Species on Bd
All of the micrograzers can be maintained in laboratory cultures, with maximum growth rates ranging from ∼0.4 to 2 d–1 (Figure 2), and all died when maintained on water alone, indicating their relative mortality rates when starved (Figure 2). When fed Bd, micrograzers exhibited four distinct responses (Figure 2): (1) for the ciliate Stentor coeruleus the death rate was significantly (and substantially) higher than in water alone; (2) for the ciliates Urocentrum turbo, Blepharisma sp., and Oxytricha sp. and the rotifer Philodina sp. there was no significant difference between death rate with or without Bd; (3) for the rotifer Brachionus calyciflorus growth rate initially increased (i.e., after 48 h) followed by death, and for the ciliates Paramecium aurelia and P. caudatum (Figure 3) the growth rate was initially positive when Bd was present followed by a negative growth rate as time progressed – on average over the incubation P. aurelia exhibited negative growth while P. caudatum exhibited zero growth (Figures 3, 4) for the ciliate Tetrahymena pyriformis there was a sustained positive growth rate (Figure 2), which was significantly higher than the negative growth rate on water alone; this growth rate of ∼1.7 ± 0.23 (SE) d–1 was equal to that when the ciliate was grown axenically on H-broth (unpublished data) and near its maximum rate under any conditions.
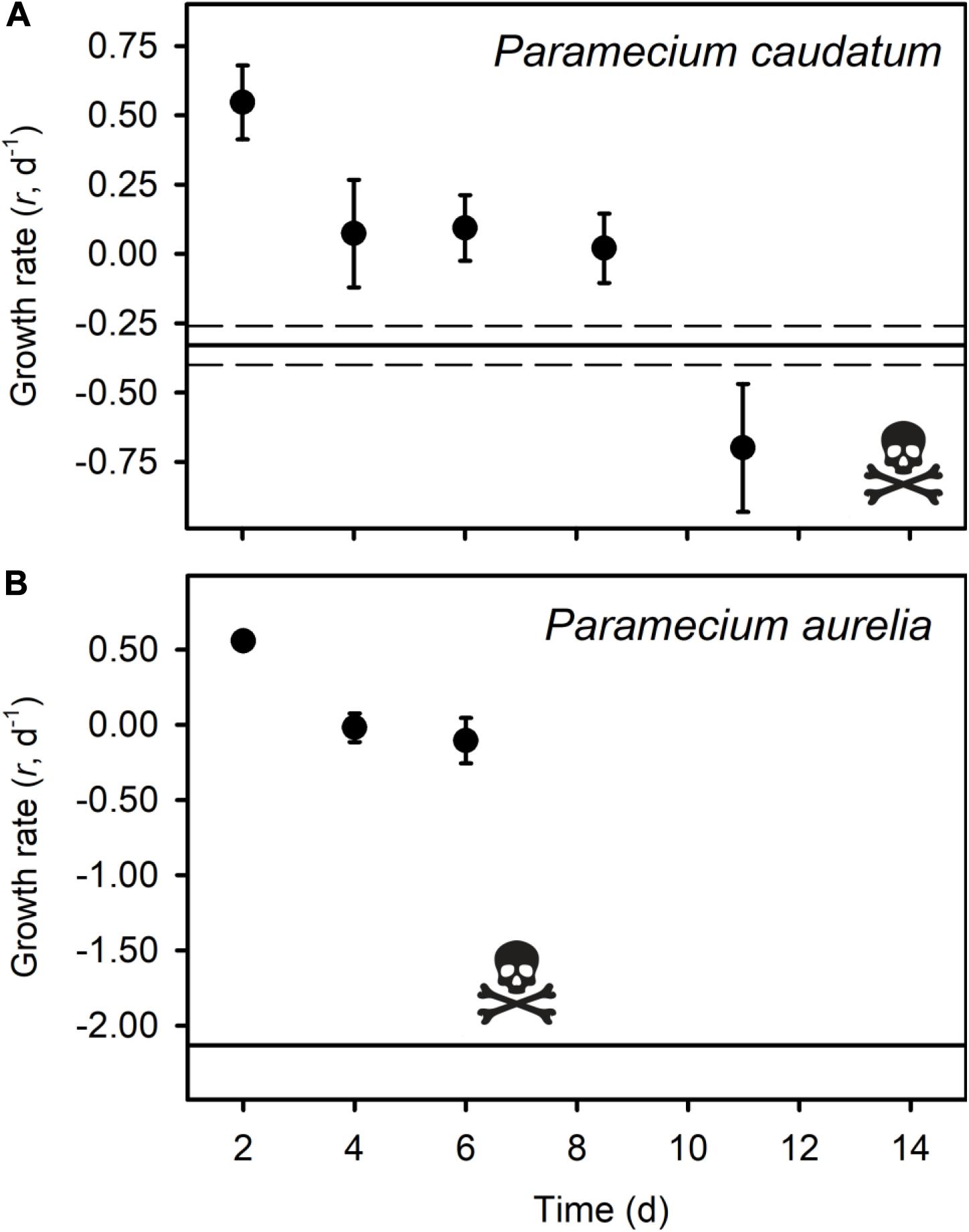
Figure 3. Average growth rates (r d– 1) of three replicates of Paramecium caudatum (A) and Paramecium aurelia (B) in the Bd treatment, with standard error bars. The skull and crossbones indicate the time point where all individuals had died. The solid black line represents the average death rate of the micrograzers when no prey were present, and the dotted black line indicates the standard error of the control groups.
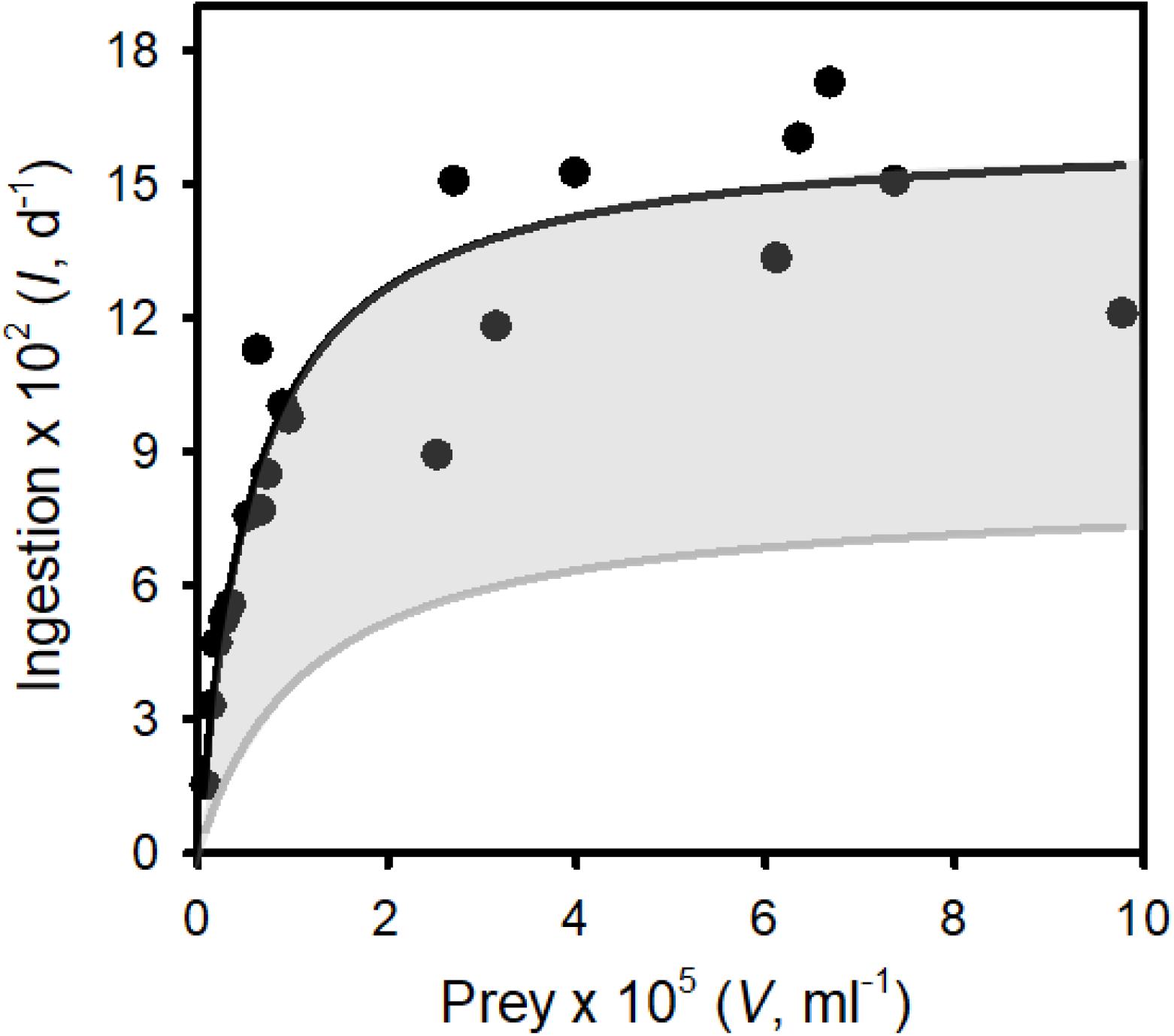
Figure 4. The functional response: ingestion rates of Tetrahymena pyriformis on surrogate zoospores (prey) vs. prey concentration. Points are ingestion rates at defined prey abundances. The solid line represents the best fit of a Type II functional response to the data (see Results for the parameter estimates). The gray region represents the range of functional responses used to assess the ability of “inferior micrograzers” to control Bd (i.e., reduced maximum ingestion rate and increased half saturation constant; see Methods, Modeling micrograzer impacts on Bd populations).
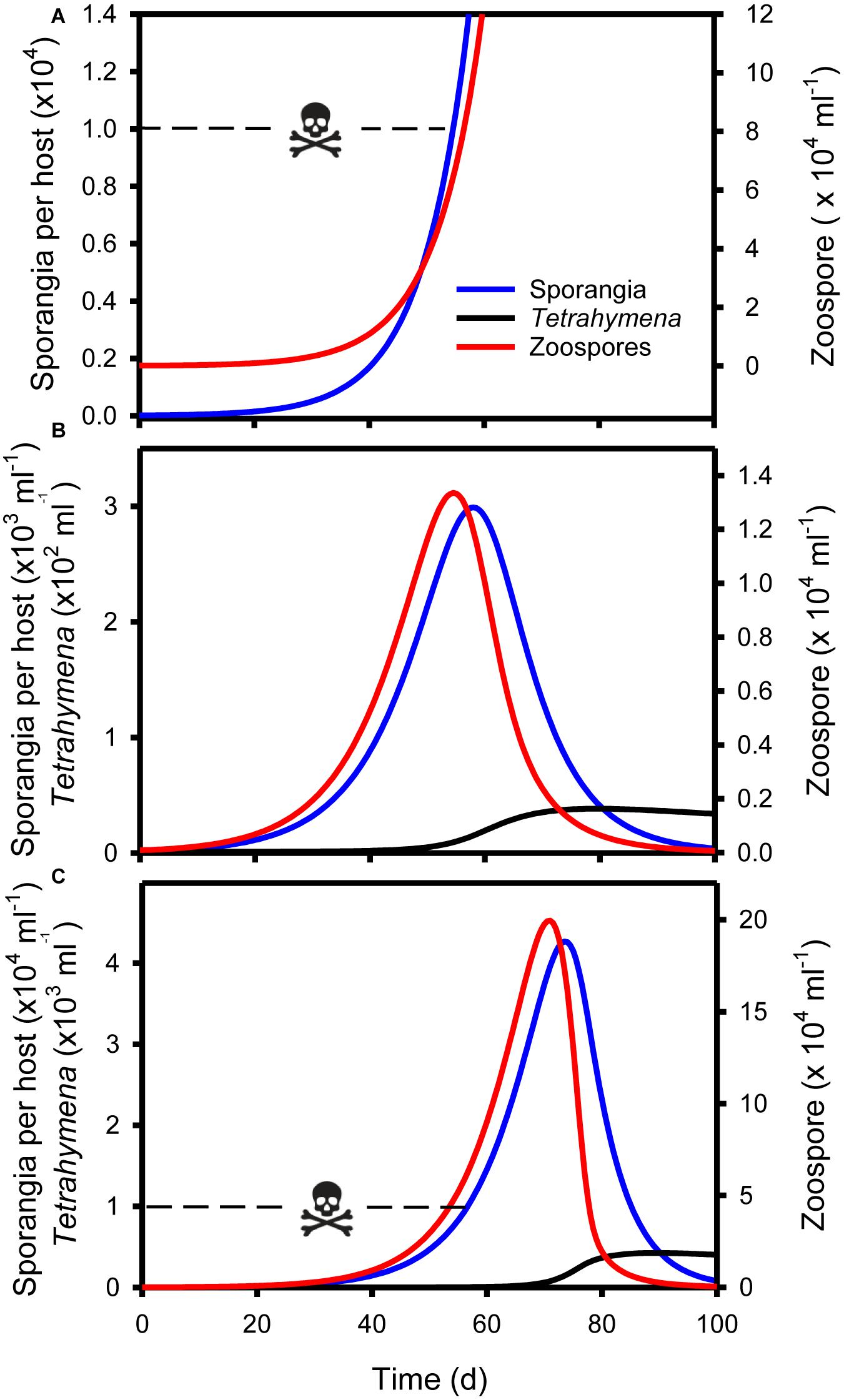
Figure 5. Simulations of micrograzer (T. pyriformis) control of Bd, based on Eq. 1–3 and parameters presented in Table 1. (A) Bd (zoospore and sporangia) dynamics in the absence of micrograzers, indicating that by ∼55 days sporangia per host approach lethal limits [skull and crossbones, 104 sporangia per host Briggs et al. (2010)]. (B) Bd and micrograzer dynamics, indicating control of zoospores and sporangia, maintaining sporangia numbers below the lethal limit. (C) Bd and micrograzer dynamics based on an inferior micrograzer to T. pyriformis (0.5 × Imax; 2 × k presented in Table 1), indicating the micrograzers inability to prevent host death at ∼55 days (skull and crossbones) but its ability to ultimately reduce Bd levels by 100 days.
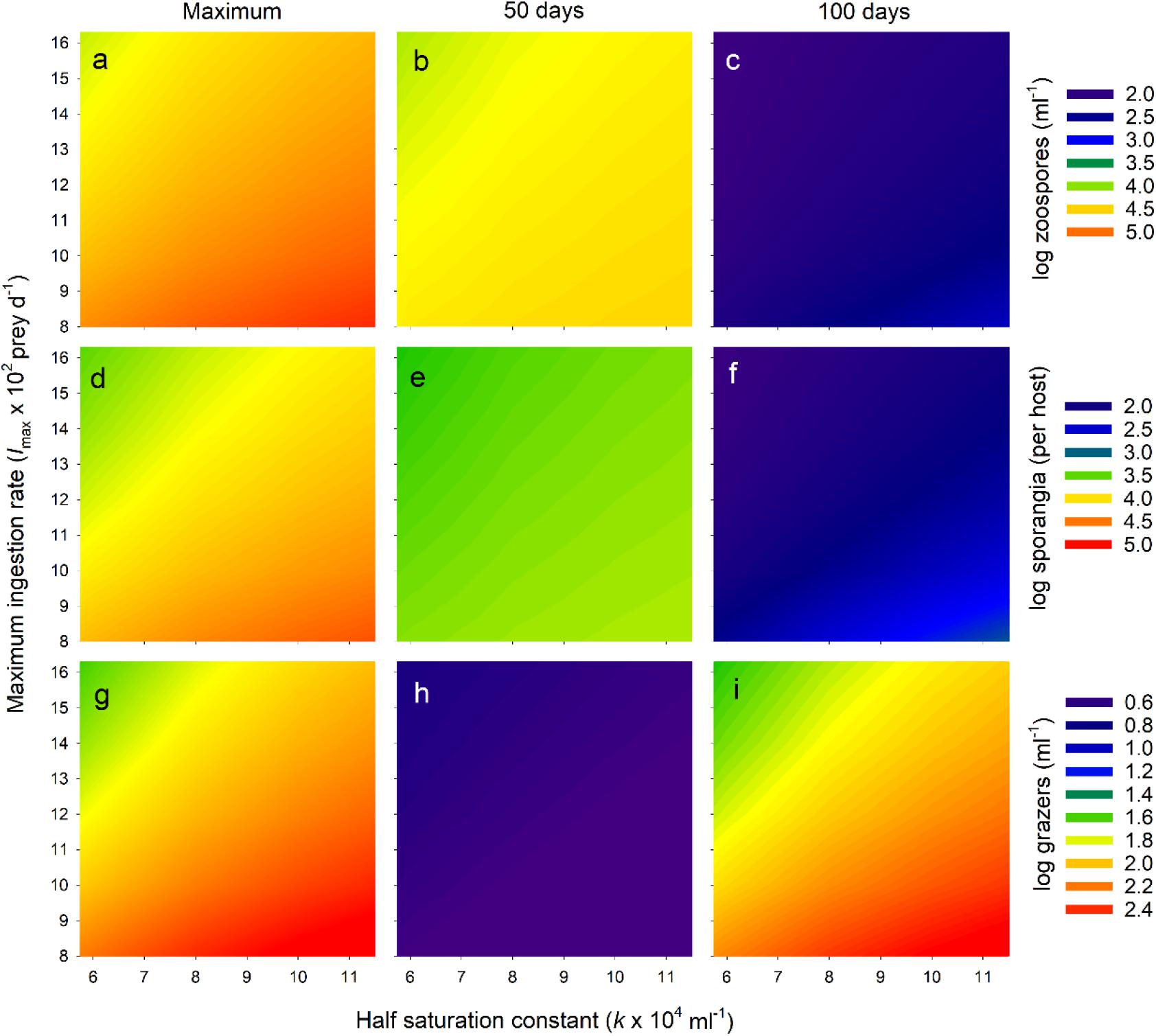
Figure 6. Exploration of Bd-micrograzer dynamics (Eq. 1–3), through varying two key micrograzer parameters: the half saturation constant (k) and the maximum ingestion rate (Imax); see Methods for details. To characterize dynamics, we provide log numbers of zoospores (a–c), sporangia (d–f), and micrograzers (g–i). Note that for each of these, abundance is presented as a colour-map, with the associated colour-key to the right of the associated three panels. To indicate trends, for each stage (zoospores, sporangia, grazers), we only present three abundances: the maximum number reached over the 100 days (Maximum), the number at 50 days (50 days), and the number at 100 days (100 days), presented in the first, middle and last columns, respectively.
Quantifying the Functional Response of Tetrahymena Grazing on Bd
As T. pyriformis grew on zoospores alone it was clear that this ciliate ingested, digested, and assimilated Bd zoospores. Zoospores were also observed in T. pyriformis, in the food vacuoles of the ciliate, under 40 × magnification, further supporting their consumption. Ingestion rate using beads as a surrogate for Bd zoospores followed a typical Type II (rectangular hyperbolic) functional response (Figure 4, adjusted R2 = 0.82), with Imax = 1.63 × 103 ± 98 (SE) prey d–1 and k = 5.75 × 103 ± 1.38 × 103 (SE) prey ml–1.
Modeling Micrograzer Impacts on Bd Populations
Control of Bd occurred when parameters for the micrograzer (T. pyriformis) were included in the model (Figure 5). In the absence of the micrograzer, sporangia per host reached lethal levels [>104 host–1 (Briggs et al., 2010)] by ∼55 days (Figure 5A). However, when micrograzers were included their population rose from 1 to ∼35 ml–1, with the result that sporangia were limited to a maximum of 3 × 103 per host (i.e., based on the assumption that 104 sporangia is a lethal limit, the host would survive), and Bd was virtually eradicated by 100 days (Figure 5B).
We then assessed the ability of micrograzers that were inferior to T. pyriformis to control Bd, through exploration of micrograzer parameter space: i.e., up to twice the half saturation (k) and half the maximum ingestion rate (Imax) of T. pyriformis (Figure 4). Figure 5C illustrates population dynamics when the most inferior micrograzer was included (highest half-saturation constant and lowest maximum ingestion rate): the general pattern remained similar to that when T. pyriformis parameters were applied, with the micrograzers controlling Bd over 100 days, but the abundance of zoospores, sporangia, and micrograzers were more than 10 times greater than the simulation including T. pyriformis, leading to predicted host death at ∼55 days and a peak in abundance at ∼70 days.
We then examined the pattern of the temporal dynamics across a wider range of parameter space (representing a range of predators-types) by reporting the maximum abundance and the abundances at 50 and 100 days of zoospores, sporangia, and micrograzers. Across all parameters explored, the micrograzer population provided top-down control of Bd, as over the entire range Bd was virtually eradicated by 100 days (Figures 6C,F). However, the quantitative levels and rates of control varied considerably with micrograzer efficiency: with reduced Imax and increased k, zoospores and sporangia reached higher maximum abundances (Figures 6A,D) and persisted longer (Figures 6B,E), indicating a decrease in the control of Bd. In particular, micrograzers with <0.7 Imax (∼103 prey d–1) and >1.5 × k (∼9 × 104 ml–1) were not capable of preventing sporangia per host exceeding lethal levels of 104 per host (the yellow-to-red region on Figure 6D). Decreased Imax and increased k also led to increases in micrograzer abundance (Figures 6G–I), in response to the increased spore levels available under these grazing regimes.
Discussion
Control of a range of diseases caused by zoosporic fungi may be achieved through consumption of zoospores (Kagami et al., 2014; Grossart and Rojas-Jimenez, 2016; Frenken et al., 2019). Here, we explore the dynamics by which micrograzers may controlling the amphibian disease chytridiomycosis, caused by Batrachochytrium dendrobatidis (Bd). Several micrograzers are known to consume Bd zoospores (Schmeller et al., 2014); we expand on this by indicating that Paramecium spp. grow on Bd zoospores for short periods and the ubiquitous ciliate Tetrahymena pyriformis maintains near maximal rate on zoospores alone. These findings provide essential information for modeling population dynamics. We then determine the functional response of T. pyriformis feeding on spore-sized prey, which is also required for population modeling. Finally, using our data and literature estimates, we develop and employ a novel model that couples the Bd life cycle with micrograzer-control on zoospores. This synthesis reveals the dynamics by which micrograzers may suppress Bd loads and argues that to predict Bd-host interactions it will be useful to consider embedding these into the larger microbial food web.
Micrograzer Growth on Bd
Micrograzers may consume Bd zoospores (Schmeller et al., 2014), and chytrid zoospores can be nutritious (Frenken et al., 2020a). All of the micrograzers we examined also could ingest Bd, but they exhibited a range of growth-responses (Figure 2). For one ciliate, S. coeruleus, Bd appeared to be toxic, possibly explaining reports that S. coeruleus does not reduce Bd viability (Schmeller et al., 2014), while other species seemed to obtain no nutritional benefit. However, several species benefited from ingesting Bd. Both Paramecium aurelia and P. caudatum initially grew, although this was not sustained (Figure 3), suggesting that while Bd is of some value, it may lack essential nutrients for these ciliates. In contrast, Tetrahymena pyriformis sustained positive growth, indicating that Bd can provide a complete diet for certain species. These observations are supported by previous work on ciliates: T. pyriformis and a closely related species, Colpidium striatum, also grow on yeast (Saccharomyces), while P. aurelia, and P. caudatum cannot, again possibly due to a lack of nutrients such as essential fatty acids and B-vitamins (Hirayama and Satuito, 1991; Pritchard et al., 2016).
Our analysis, therefore, suggest that not all micrograzers would be capable of or equally proficient at controlling Bd. However, with additional prey sources to sustain the consumers, there may be selective feeding on Bd. For instance, although some studies have shown Tetrahymena to be a non-selective feeder (Kirby et al., 2019) others show that T. pyriformis differentiates between bacterial prey, leading to a more efficient assimilation of prey biomass and a greater cell yield of ciliates (Thurman et al., 2010). Likely, in the mesocosm experiments conducted by Schmeller et al. (2014), where Paramecium controlled Bd, this ciliate’s diet was supplemented by naturally occurring bacteria. In fact, in our initial growth-experiments, where antibiotics were not included, bacteria grew, and Paramecium caudatum consumed zoospores in addition to bacteria and maintained extended positive growth (Supplementary Material 1). Our analysis here has focused on Bd as the sole food source and indicates that micrograzer dynamics (growth and ingestion) lead to control of Bd populations. These findings argue that Bd-host dynamics should now be examined in a wider food-web context, with mixed an assemblage of microgazers sustained by Bd and a wider range of natural food sources.
Tetrahymena Grazing on Bd
Globally, Tetrahymena is common in shallow waters, living near sediments, where it consumes bacteria and other microbes (Simon et al., 2007; Doerder, 2019). These are the same habitats that Bd occupies. Tetrahymena is also associated with amphibians where it may be an opportunistic ectoparasite (Thompson, 1958; Holz et al., 2015), but possibly also a consumer of Bd zoospores as they emerge from sporangia. Considering its habitat and ability to rapidly reproduce on Bd alone, we focused on T. pyriformis’ ingestion of Bd zoospores. In contradiction to Schmeller et al. (2014), attempts to stain Bd zoospores with calcofluor-white were not successful; calcofluor stains chitin (Hageage and Harrington, 1984), and although Bd sporangia have a chitin wall, zoospores do not (Berger et al., 2005). Therefore, this staining method seems inappropriate for Bd zoospores. We then explored vital stains (e.g., cell tracker green), but again we were not successful. Consequently, ingestion estimates relied on the uptake of fluorescent beads as surrogates for Bd, which may underestimate rates (e.g., Montagnes and Lessard, 1999). We, therefore, see our predictions as conservative, which we partially account for by examining slightly superior grazers (Figure 6). From our data, a clear Type II functional response was obtained for T. pyriformis (Figure 4), providing essential parameters for modeling Bd-micrograzer dynamics (see section “Methods”). To our knowledge, this is the first time a functional response on Bd sized particles has been obtained for any Tetrahymena species: the estimates of Imax and k are within the range of those obtained for other ciliates, although the k-values are on the lower end of the spectrum (Jürgens and Šimek, 2000; Gismervik, 2005; Pritchard et al., 2016), suggesting Tetrahymena has a high affinity for Bd size particles; our modeling, therefore, includes micrograzers that are inferior to Tetrahymena.
Modeling Micrograzer Impacts on Bd Populations
Empirical evidence suggests that Paramecium can reduce Bd infections, through examining end point estimates of host infection (Schmeller et al., 2014). Here we explore the temporal dynamics of such control and the potential for micrograzers to prevent host death. Through sensitivity analysis we examine a range of grazers from those with slightly higher grazing rates than Tetrahymena – reflecting potential discrimination of beads – to those substantially inferior – reflecting species that may not grow as well on Bd, such as Paramecium. Our analysis is reductionist and hence more qualitative than quantitative in its predictions. However, it clearly reveals that by applying plausible parameters for both the parasite and micrograzer, in a local environment, chytridiomycosis may at times be prevented and Bd virtually eradicated, or at least reduced to negligible levels (Figure 6). Critically, it suggests the time scales over which such dynamics may occur. Admittedly, we indicate that micrograzers that are inferior to T. pyriformis are less likely to prevent host death, yet they still, ultimately, reduce Bd populations to negligible numbers, potentially preventing further disease outbreaks (Figure 6). Our model, therefore, provides a mechanism to evaluate Bd-micrograzer dynamics, and its predictions strongly argue for the continued exploration of micrograzers in Bd research, specifically, and in the control of a range of diseases that spread through zoospores or other similarly sized dispersal stages (Kagami et al., 2014; Grossart and Rojas-Jimenez, 2016; Frenken et al., 2019).
To date, models of Bd-dynamics (Mitchell et al., 2008; Woodhams et al., 2008; Briggs et al., 2010; Louca et al., 2014) have not included estimates of spore loss by micrograzers. As indicated above, the modeling provided here is instructive and could benefit from elaboration. Given the ubiquity of protozoa in natural waters (Fenchel, 1987) and their potential impact (Figure 6; Kagami et al., 2014; Grossart and Rojas-Jimenez, 2016; Frenken et al., 2019), we suggest there is now a need for better parameterization of micrograzer-Bd responses. We suggest three main directions. First, micrograzers, and specifically Tetrahymena, exhibit chemosensory behavior (Leick and Hellung-Larsen, 1992); the extent to which protozoa are attracted to amphibian hosts and Bd requires evaluation. Second, as indicated above, the role of Bd as a supplement rather than a sole dietary component deserves attention. Finally, the Rosenzweig-MacArthur predator-prey model, which we used, has limitations. Model structures such as the independent response model (Fenton et al., 2010) that rely, independently, on growth and ingestion responses provide better predictions (Li and Montagnes, 2015). To this end, we suggest that both functional (ingestion) and numerical (growth) responses associated with Bd abundance are established for a range of micrograzers.
Future Directions for Microbial Ecology and Bd
Both Tetrahymena and Paramecium are common species in shallow waters (Görtz, 1988; Simon et al., 2007; Doerder, 2019) that, as we have shown, are capable of growth on Bd zoospores for limited to extended times. Undoubtedly, other protozoa will also consume and grow on Bd zoospores. We, therefore, suggest that the role of micrograzers is considered when evaluating Bd-dynamics and the dynamics of other zoosporic diseases. For instance, micrograzers may be important in ingesting chytrid spores that infect phytoplankton (Frenken et al., 2020b). However, planktonic levels of chytrid spores associated with phytoplankton blooms are on the order of 103 ml–1 (Frenken et al., 2020a), which is at the lowest end of the functional response of Tetrahymena (Figure 3), suggesting low ingestion rates for this and related taxa. Instead, other grazers may play greater roles, although the potential control of phytoplankton chytrid epidemics may generally be relatively low, except for the fastest growing, and smallest, grazers such as ciliates and rotifers (Frenken et al., 2020a).
Contributions of micrograzers to disease dynamics are also likely to have a highly site-specific component, due to their dependence on environmental factors (Doerder, 2019). For instance, chytridiomycosis is more prevalent at higher altitudes (Walker et al., 2010), which will often be both cooler and oligotrophic. While temperature may, in part, determine infection burdens (Cohen et al., 2019), there will likely be an interaction with the trophic status of the water. If in oligotrophic waters bacteria are reduced below levels sufficient to support ciliates (<106 ml–1), top-down control may be absent, and our analysis suggests that Bd may thrive, resulting in chytridiomycosis. Consequently, assessing the abundance of micrograzers in waters where chytridiomycosis occurs or is predicted seems warranted.
Finally, as both Tetrahymena and Paramecium are already common if not ubiquitous in aquatic environments and are simple and inexpensive to grow in large quantities (Görtz, 1988; Simon et al., 2007), they may be tractable target species for biomanipulation. We support previous suggestions (Schmeller et al., 2014) that by augmenting natural densities of these species, through addition or supplementary feeding, it may be possible to reduce zoospore densities for Bd in situ. However, such manipulations could have detrimental, ecosystem-changing effects (Symondson et al., 2002). Extensive evaluation of the role of microgazers in a wider context is, therefore, required before implementation of such approaches.
Data Availability Statement
The data supporting the conclusions of this article have been made available by the authors, without reservation. They can be found at: https://doi.org/10.6084/m9.figshare.13516577.v1.
Author Contributions
HF, JJ, and AH conducted the laboratory work, under the guidance of DM. HF, AF, DD, and DM devised and conducted the modeling. TG and MF provided specific guidance on host and chytrid biology, respectively. All authors provided essential input into the conceptualisation of the study, design of the experiments, contributed to writing, and revising the manuscript.
Conflict of Interest
The authors declare that the research was conducted in the absence of any commercial or financial relationships that could be construed as a potential conflict of interest.
Funding and Support
This work was conducted as part of postgraduate project (HF) at the University of Liverpool and was supported by funding provided to JJ through the Program for Training University Teachers by Shanghai Municipal Education Commission. AF and TG were funded by the NERC grant NE/N009800/1. MF was a CIFAR fellow with Bd research supported by NERC NE/K014455/1. Eleanor Ambrose, Philip Bahan, Valentina Iorio, and Sam Moss provided invaluable technical support. This manuscript has been released as a pre-print at BioRxiv, (Farthing et al., 2020).
Supplementary Material
The Supplementary Material for this article can be found online at: https://www.frontiersin.org/articles/10.3389/fmicb.2020.592286/full#supplementary-material
References
Azam, F., Fenchel, T., Field, J. G., Gray, J. S., Meyer-Reil, L. A., and Thingstad, F. (1983). The ecological role of water-column microbes in the sea. Mar. Ecol. Prog. Ser. 10, 257–263. doi: 10.3354/meps010257
Beiswenger, R. E. (1977). Diel patterns of aggregative behavior in tadpoles of Bufo americanus, in relation to light and temperature. Ecology 58, 98–108. doi: 10.2307/1935111
Berger, L., Hyatt, A. D., Speare, R., and Longcore, J. E. (2005). Life cycle stages of the amphibian chytrid Batrachochytrium dendrobatidis. Dis. Aquat. Organ. 68, 51–63. doi: 10.3354/dao068051
Berges, J. A., Montagnes, D. J. S., Hurd, C. L., and Harrison, P. J. (1994). Fitting ecological and physiological data to rectangular hyperbolae: a comparison of methods using monte carlo simulations. Mar. Ecol. Prog. Ser. 114, 175–183. doi: 10.3354/meps114175
Briggs, C. J., Knapp, R. A., and Vredenburg, V. T. (2010). Enzootic and epizootic dynamics of the chytrid fungal pathogen of amphibians. Proc. Natl. Acad. Sci. U.S.A. 107, 9695–9700. doi: 10.1073/pnas.0912886107
Buck, J. C., Rohr, J. R., and Blaustein, A. R. (2016). Effects of nutrient supplementation on host-pathogen dynamics of the amphibian chytrid fungus: a community approach. Freshw. Biol. 61, 110–120. doi: 10.1111/fwb.12685
Buck, J. C., Truong, L., and Blaustein, A. R. (2011). Predation by zooplankton on Batrachochytrium dendrobatidis: biological control of the deadly amphibian chytrid fungus? Biodivers. Conserv. 20, 3549–3553. doi: 10.1007/s10531-011-0147-4
Cohen, J. M., McMahon, T. A., Ramsay, C., Roznik, E. A., Sauer, E. L., Bessler, S., et al. (2019). Impacts of thermal mismatches on chytrid fungus Batrachochytrium dendrobatidis prevalence are moderated by life stage, body size, elevation and latitude. Ecol. Lett. 22, 817–825. doi: 10.1111/ele.13239
Dionne, J. C. (1969). Tadpole holes; a true biogenic sedimentary structure. J. Sediment. Res. B Stratigr. Glob. Stud. 39, 358–360. doi: 10.1306/74D71C6C-2B21-11D7-8648000102C1865D
Doerder, F. P. (2019). Barcodes reveal 48 new species of Tetrahymena, Dexiostoma, and Glaucoma: phylogeny, ecology, and biogeography of new and established species. J. Eukaryot. Microbiol. 66, 182–208. doi: 10.1111/jeu.12642
Farthing, H. N., Jiang, J., Henwood, A. J., Fenton, A., Garner, T. W. J., Daversa, D. R., et al. (2020). Microbial grazers may aid in controlling infections caused by aquatic zoosporic fungi. BioRxiv [Preprint]. doi: 10.1101/2020.02.03.931857
Fenchel, T. (1987). Ecology of Protozoa—The Biology of Free-Living Phagotrophic Protists. New York, NY: Springer Verlag.
Fenton, A., Spencer, M., and Montagnes, D. J. S. (2010). Parameterising variable assimilation efficiency in predator-prey models. Oikos 119, 1000–1010. doi: 10.1111/j.1600-0706.2009.17875.x
Finlay, B. J. (1977). The dependence of reproductive rate on cell size and temperature in freshwater ciliated protozoa. Oecologia 30, 75–81. doi: 10.1007/BF00344893
Fisher, M. C., Bosch, J., Yin, Z., Stead, D. A., Walker, J., Selway, L., et al. (2009). Proteomic and phenotypic profiling of the amphibian pathogen Batrachochytrium dendrobatidis shows that genotype is linked to virulence. Mol. Ecol. 18, 415–429. doi: 10.1111/j.1365-294X.2008.04041.x
Frenken, T., Agha, R., Schmeller, D. S., van West, P., and Wolinska, J. (2019). Biological concepts for the control of aquatic zoosporic diseases. Trends Parasitol. 35, 571–582. doi: 10.1016/j.pt.2019.04.003
Frenken, T., Miki, T., Kagami, M., Van de Waal, D. B., Van Donk, E., Rohrlack, T., et al. (2020a). The potential of zooplankton in constraining chytrid epidemics in phytoplankton hosts. Ecology 101:e02900. doi: 10.1002/ecy.2900
Frenken, T., Wolinska, J., Tao, Y., Rohrlack, T., and Agha, R. (2020b). Infection of filamentous phytoplankton by fungal parasites enhances herbivory in pelagic food webs. Limnol. Oceanogr. 65, 2618–2626. doi: 10.1002/lno.11474
Garmyn, A., Van Rooij, P., Pasmans, F., Hellebuyck, T., Van Den Broeck, W., Haesebrouck, F., et al. (2012). Waterfowl: potential environmental reservoirs of the chytrid fungus Batrachochytrium dendrobatidis. PLoS One 7:e35038. doi: 10.1371/journal.pone.0035038
Garner, T. W. J., Walker, S., Bosch, J., Leech, S., Marcus Rowcliffe, J., Cunningham, A. A., et al. (2009). Life history tradeoffs influence mortality associated with the amphibian pathogen Batrachochytrium dendrobatidis. Oikos 118, 783–791. doi: 10.1111/j.1600-0706.2008.17202.x
Gerphagnon, M., Agha, R., Martin-Creuzburg, D., Bec, A., Perriere, F., Rad-Menéndez, C., et al. (2018). Comparison of sterol and fatty acid profiles of chytrids and their hosts reveals trophic upgrading of nutritionally inadequate phytoplankton by fungal parasites. Environ. Microbiol. 21, 949–958. doi: 10.1111/1462-2920.14489
Gismervik, I. (2005). Numerical and functional responses of choreo-and oligotrich planktonic ciliates. Aquat. Microb. Ecol. 40, 163–173. doi: 10.3354/ame040163
Gleason, F. H., Kagami, M., Lefevre, E., and Sime-Ngando, T. (2008). The ecology of chytrids in aquatic ecosystems: roles in food web dynamics. Fungal Biol. Rev. 22, 17–25. doi: 10.1016/j.fbr.2008.02.001
Gleason, F. H., Lilje, O., Marano, A. V., Sime-Ngando, T., Sullivan, B. K., Kirchmair, M., et al. (2014). Ecological functions of zoosporic hyperparasites. Front. Microbiol. 5:244. doi: 10.3389/fmicb.2014.00244
Grossart, H. P., and Rojas-Jimenez, K. (2016). Aquatic fungi: targeting the forgotten in microbial ecology. Curr. Opin. Microbiol. 31, 140–145. doi: 10.1016/j.mib.2016.03.016
Hageage, G. J., and Harrington, B. J. (1984). Use of calcofluor white in clinical mycology. Lab. Med. 15, 109–112. doi: 10.1093/labmed/15.2.109
Hamilton, P. T., Richardson, J. M. L., and Anholt, B. R. (2012). Daphnia in tadpole mesocosms: trophic links and interactions with Batrachochytrium dendrobatidis. Freshw. Biol. 57, 676–683. doi: 10.1111/j.1365-2427.2011.02731.x
Hirayama, K., and Satuito, C. (1991). “The nutritional improvement of baker’s yeast for the growth of the rotifer, Brachionus plicatilis,” in Proceedings of the U.S.-Asia Workshop Rotifer and Microalgae Culture Systems January 28–31, eds W. Fulks and K. Main (Honolulu, HAW: The Oceanic Institute), 151–162.
Holz, P. H., Portas, T., Donahoe, S., Crameri, S., and Rose, K. (2015). Mortality in northern corroboree frog tadpoles (Pseudophryne pengilleyi) associated with Tetrahymena-like infection. Aust. Vet. J. 93, 295–297. doi: 10.1111/avj.12337
Inamori, Y., Kuniyasu, Y., Hayashi, N., Ohtake, H., and Sudo, R. (1990). Monoxenic and mixed cultures of the small metazoa Philodina erythrophthalma and Aeolosoma hemprichi isolated from a waste-water treatment process. Appl. Microbiol. Biotechnol. 34, 404–407.
Jackson, K. M., and Berger, J. (1984). Survival of ciliate protozoa under starvation conditions and at low bacterial levels. Microb. Ecol. 10, 47–59. doi: 10.1007/bf02011594
Jürgens, K., and Šimek, K. (2000). Functional response and particle size selection of Halteria cf. Grandinella, a common freshwater oligotrichous ciliate. Aquat. Microb. Ecol. 22, 57–68. doi: 10.3354/ame022057
Kagami, M., de Bruin, A., Ibelings, B. W., and Van Donk, E. (2007). Parasitic chytrids: their effects on phytoplankton communities and food-web dynamics. Hydrobiologia 578, 113–129. doi: 10.1007/s10750-006-0438-z
Kagami, M., Miki, T., and Takimoto, G. (2014). Mycoloop: chytrids in aquatic food webs. Front. Microbiol. 5:166. doi: 10.3389/fmicb.2014.00166
Kasturi Bai, A. R., Srihari, K., Shadaksharaswamy, M., and Jyothy, P. S. (1969). The effects of temperature on Blepharisma intermedium. J. Protozool. 16, 738–743. doi: 10.1111/j.1550-7408.1969.tb02336.x
Kilpatrick, A. M., Briggs, C. J., and Daszak, P. (2010). The ecology and impact of chytridiomycosis: an emerging disease of amphibians. Trends Ecol. Evol. 25, 109–118. doi: 10.1016/j.tree.2009.07.011
Kirby, A. E., Menta, B. W., and Corotto, F. (2019). Findings consistent with nonselective feeding in Tetrahymena pyriformis. Ga. J. Sci. 77:4.
Kirshtein, J. D., Anderson, C. W., Wood, J. S., Longcore, J. E., and Voytek, M. A. (2007). Quantitative PCR detection of Batrachochytrium dendrobatidis DNA from sediments and water. Dis. Aquat. Organ. 77, 11–15. doi: 10.3354/dao01831
Leick, V., and Hellung-Larsen, P. (1992). Chemosensory behaviour of Tetrahymena. Bioessays 14, 61–66. doi: 10.1002/bies.950140113
Li, J., and Montagnes, D. J. S. (2015). Restructuring fundamental predator-prey models by recognising prey-dependent conversion efficiency and mortality rates. Protist 166, 211–223. doi: 10.1016/j.protis.2015.02.003
Louca, S., Lampo, M., and Doebeli, M. (2014). Assessing host extinction risk following exposure to Batrachochytrium dendrobatidis. Proc. Biol. Sci. 281:20132783. doi: 10.1098/rspb.2013.2783
Maguire, C., DiRenzo, G. V., Tunstall, T. S., Muletz, C. R., Zamudio, K. R., and Lips, K. R. (2016). Dead or alive? Viability of chytrid zoospores shed from live amphibian hosts. Dis. Aquat. Organ. 119, 179–187. doi: 10.3354/dao02991
Maier, M. A., Uchii, K., Peterson, T. D., and Kagami, M. (2016). Evaluation of daphnid grazing on microscopic zoosporic fungi by using comparative threshold cycle quantitative PCR. Appl. Environ. Microbiol. 82, 3868–3874. doi: 10.1128/AEM.00087-16
Mitchell, K. M., Churcher, T. S., Garner, T. W., and Fisher, M. C. (2008). Persistence of the emerging pathogen Batrachochytrium dendrobatidis outside the amphibian host greatly increases the probability of host extinction. Proc. Biol. Sci. 275, 329–334. doi: 10.1098/rspb.2007.1356
Montagnes, D. J. S. (1996). Growth responses of planktonic ciliates in the genera Strobilidium and Strombidium. Mar. Ecol. Prog. Ser. 130, 241–254. doi: 10.3354/meps130241
Montagnes, D. J. S., Barbosa, A. B., Boenigk, J., Davidson, K., Jürgens, K., Macek, M., et al. (2008). Selective feeding behaviour of key free-living protists. Aquat. Microb. Ecol. 53, 83–98. doi: 10.3354/ame01229
Montagnes, D. J. S., and Berges, J. A. (2004). Determining parameters of the numerical response. Microb. Ecol. 48, 139–144. doi: 10.1007/s00248-003-9000-y
Montagnes, D. J. S., Kimmance, S., and Atkinson, D. (2003). Using Q10: can growth rates increase linearly with temperature? Aquat. Microb. Ecol. 32, 307–313. doi: 10.3354/ame032307
Montagnes, D. J. S., and Lessard, E. (1999). Population dynamics of the marine planktonic ciliate Strombidinopsis multiaris. Aquat. Microb. Ecol. 20, 167–181. doi: 10.3354/ame020167
Montagnes, D. J. S., Zhu, X., Gu, L., Sun, Y., Wang, J., Horner, R., et al. (2019). False exclusion: a case to embed predator performance in classical population models. Am. Nat. 194, 654–670. doi: 10.1086/705381
Moss, A. S., Reddy, N. S., Dortaj, I. M., and San Francisco, M. J. (2008). Chemotaxis of the amphibian pathogen Batrachochytrium dendrobatidis and its response to a variety of attractants. Mycologia 100, 1–5. doi: 10.3852/mycologia.100.1.1
Piotrowski, J. S., Annis, S. L., and Longcore, J. E. (2004). Physiology of Batrachochytrium dendrobatidis, a chytrid pathogen of amphibians. Mycologia 96, 9–15. doi: 10.2307/3761981
Pritchard, J. O., Porter, A. H., and Montagnes, D. J. S. (2016). Did gause have a yeast infection? J. Eukaryot. Microbiol. 63, 552–557. doi: 10.1111/jeu.12299
Salt, J. L., Bulit, C., Zhang, W., Qi, H., and Montagnes, D. J. S. (2017). Spatial extinction or persistence: landscape-temperature interactions perturb predator–prey dynamics. Ecography 40, 1177–1186. doi: 10.5061/dryad.p1442
Scheele, B. C., Pasmans, F., Skerratt, L. F., Berger, L., Martel, A., Beukema, W., et al. (2019). Amphibian fungal panzootic causes catastrophic and ongoing loss of biodiversity. Science 363, 1459–1463. doi: 10.1126/science.aav0379
Schmeller, D. S., Blooi, M., Martel, A., Garner, T. W., Fisher, M. C., Azemar, F., et al. (2014). Microscopic aquatic predators strongly affect infection dynamics of a globally emerged pathogen. Curr. Biol. 24, 176–180. doi: 10.1016/j.cub.2013.11.032
Searle, C. L., Mendelson, J. R. III, Green, L. E., and Duffy, M. A. (2013). Daphnia predation on the amphibian chytrid fungus and its impacts on disease risk in tadpoles. Ecol. Evol. 3, 4129–4138. doi: 10.1002/ece3.777
Simon, E. M., Nanney, D. L., and Doerder, F. P. (2007). “The Tetrahymena pyriformis complex of cryptic species,” in Protist Diversity and Geographical Distribution, eds W. Foissner and D. Hawksworth (Dordrecht: Springer), 131–146. doi: 10.1007/978-90-481-2801-3_10
Symondson, W. O. C., Sunderland, K. D., and Greenstone, M. H. (2002). Can generalist predators be effective biocontrol agents? Annu. Rev. Entomol. 47, 561–594. doi: 10.1146/annurev.ento.47.091201.145240
Taylor, W. D. (1978). Maximum growth rate, size and commonness in a community of bactivorous ciliates. Oecologia 36, 263–272. doi: 10.1007/BF00348052
Thompson, J. C. (1958). Experimental infections of various animals with strains of the genus Tetrahymena. J. Eukaryot. Microbiol. 5, 203–205. doi: 10.1111/j.1550-7408.1958.tb02552.x
Thurman, J., Parry, J. D., Hill, P. J., and Laybourn-Parry, J. (2010). The filter-feeding ciliates Colpidium striatum and Tetrahymena pyriformis display selective feeding behaviours in the presence of mixed, equally-sized, bacterial prey. Protist 161, 577–588. doi: 10.1016/j.protis.2010.04.001
Thurnheer, S., and Reyer, H. U. (2001). Diel patterns of aggregative behavior in tadpoles of Bufo americanus, in relation to light and temperature. Amphib. Reptil. 22, 21–32.
Turchin, P. (2003). Complex Population Dynamics: A Theoretical/Empirical Synthesis. Oxford: Princeton University Press.
Walker, S. F., Bosch, J., Gomez, V., Garner, T. W., Cunningham, A. A., Schmeller, D. S., et al. (2010). Factors driving pathogenicity vs. prevalence of amphibian panzootic chytridiomycosis in Iberia. Ecol. Lett. 13, 372–382. doi: 10.1111/j.1461-0248.2009.01434.x
Walker, S. F., Salas, M. B., Jenkins, D., Garner, T. W., Cunningham, A. A., Hyatt, A. D., et al. (2007). Environmental detection of Batrachochytrium dendrobatidis in a temperate climate. Dis. Aquat. Organ. 77, 105–112. doi: 10.3354/dao01850
Weisse, T. (2017). Functional diversity of aquatic ciliates. Eur. J. Protistol. 61, 331–358. doi: 10.1016/j.ejop.2017.04.001
Weisse, T., Moser, M., Scheffel, U., Stadler, P., Berendonk, T., Weithoff, G., et al. (2013). Systematics and species-specific response to pH of Oxytricha acidotolerans sp. nov. and Urosomoida sp. (Ciliophora, Hypotricha) from acid mining lakes. Eur. J. Protistol. 49, 255–271. doi: 10.1016/j.ejop.2012.08.001
Weithoff, G., and Walz, N. (1995). Influence of the filamentous cyanobacterium Planktothrix agardhii on population growth and reproductive pattern of the rotifer Brachionus calyciflorus. Hydrobiologia 313, 381–386. doi: 10.1007/978-94-009-1583-1_50
Woodhams, D. C., Alford, R. A., Briggs, C. J., Johnson, M., and Rollins-Smith, L. A. (2008). Life-history trade-offs influence disease in changing climates: strategies of an amphibian pathogen. Ecology 89, 1627–1639. doi: 10.1890/06-1842.1
Keywords: ciliates, disease, fungi, microbial loop, protozoa, Tetrahymena
Citation: Farthing HN, Jiang J, Henwood AJ, Fenton A, Garner TWJ, Daversa DR, Fisher MC and Montagnes DJS (2021) Microbial Grazers May Aid in Controlling Infections Caused by the Aquatic Zoosporic Fungus Batrachochytrium dendrobatidis. Front. Microbiol. 11:592286. doi: 10.3389/fmicb.2020.592286
Received: 06 August 2020; Accepted: 16 December 2020;
Published: 21 January 2021.
Edited by:
Jonathan P. Zehr, University of California, Santa Cruz, United StatesReviewed by:
Falk Hillmann, Leibniz Institute for Natural Product Research and Infection Biology, GermanyThijs Frenken, Netherlands Institute of Ecology (NIOO-KNAW), Netherlands
Copyright © 2021 Farthing, Jiang, Henwood, Fenton, Garner, Daversa, Fisher and Montagnes. This is an open-access article distributed under the terms of the Creative Commons Attribution License (CC BY). The use, distribution or reproduction in other forums is permitted, provided the original author(s) and the copyright owner(s) are credited and that the original publication in this journal is cited, in accordance with accepted academic practice. No use, distribution or reproduction is permitted which does not comply with these terms.
*Correspondence: David J. S. Montagnes, ZG1vbnRhZ0BsaXYuYWMudWs=
†These authors have contributed equally to this work and share first authorship