- 1Soil and Fertilizer Institute, Sichuan Academy of Agricultural Sciences, Chengdu, China
- 2School of Food and Biological Engineering, Chengdu University, Chengdu, China
- 3College of Life Sciences, Henan Agricultural University, Zhengzhou, China
In the present study, the mitogenome of Tuber calosporum was assembled and analyzed. The mitogenome of T. calosporum comprises 15 conserved protein-coding genes, two rRNA genes, and 14 tRNAs, with a total size of 287,403 bp. Fifty-eight introns with 170 intronic open reading frames were detected in the T. calosporum mitogenome. The intronic region occupied 69.41% of the T. calosporum mitogenome, which contributed to the T. calosporum mitogenome significantly expand relative to most fungal species. Comparative mitogenomic analysis revealed large-scale gene rearrangements occurred in the mitogenome of T. calosporum, involving gene relocations and position exchanges. The mitogenome of T. calosporum was found to have lost several tRNA genes encoding for cysteine, aspartate, histidine, etc. In addition, a pair of fragments with a total length of 32.91 kb in both the nuclear and mitochondrial genomes of T. calosporum was detected, indicating possible gene transfer events. A total of 12.83% intragenomic duplications were detected in the T. calosporum mitogenome. Phylogenetic analysis based on mitochondrial gene datasets obtained well-supported tree topologies, indicating that mitochondrial genes could be reliable molecular markers for phylogenetic analyses of Ascomycota. This study served as the first report on mitogenome in the family Tuberaceae, thereby laying the groundwork for our understanding of the evolution, phylogeny, and population genetics of these important ectomycorrhizal fungi.
Introduction
The Tuber genus is a diversified lineage of truffle-forming fungi that produce hypogeous fruiting bodies. Truffles are regarded as prized food delicacies because of their unique flavors (Zhang et al., 2016; Caboni et al., 2020). As ectomycorrhizal fungus, Tuber species must form ectomycorrhiza with their host plants to complete their life cycles. It was reported that symbiosis with truffles and other ectomycorrhizal fungi could promote the growth of host plants and enhance the tolerance of host plants to pathogenic and abiotic stresses (Luo et al., 2009; Sebastiana et al., 2018; Zhang et al., 2019; Li X. et al., 2020). In return, plants provide carbon sources for truffles to grow and reproduce. The formation of this symbiotic relationship in nature plays an important role in maintaining the balance of forest ecosystem and promoting the carbon natural cycle (Franco et al., 2014; Corrales et al., 2018). Truffle, expecially T. melanosporum, has been used as model species to study the evolution, genetics, and ecological adaptation of ectomycorrhizal fungi (Martin et al., 2010; Murat et al., 2018b; Zarivi et al., 2018). Genome analyses revealed peculiar features of truffles, such as heterothallism, few genes coding lignocellulose-degrading enzymes, were closely related to the ectomycorrhizal life patterns of truffles (Martin et al., 2010; Rubini et al., 2011; Murat et al., 2018a, b). However, the mitochondrial gene characteristics of truffles are still unknown, which limits our comprehensive understanding of the genetic information and evolution of truffles. Tuber calosporum was found in southwest China, which was described by Wan et al. (2016). Tuber calosporum lives in soil under mixed forest with Pinus yunnanensis as dominant species. Phylogenetic analysis found that the T. calosporum belonged to the Macrosporum group (Wan et al., 2016).
As additional genetic component of eukaryotes cells, the mitochondrial genome (mitogenome) has been reported playing an important regulatory role in the process of stress resistance, growth and development, aging, and death (Ding et al., 2019; Luevano-Martinez et al., 2019). In addition, mitogenome features, such as the several available molecular markers, uniparental inheritance, have promoted the mitogenome becoming a powerful tool for studying the phylogeny and evolution of eukaryotic species (Andersen and Balding, 2018; Fourie et al., 2018; Li et al., 2018b, 2020a). The repetitive sequence, intron information, tRNA structure, and gene arrangement of the mitogenome also provide useful information for understanding the evolution of species (du Toit et al., 2017; Li et al., 2018a, 2020b). However, compared to the available mitogenomes of animals (>9,000 mitogenomes in database), the available mitogenome of fungi (<700 mitogenomes in database) is far from enough and is even less than the studied nuclear genomes of fungi (>5,000 genomes in database). So far, only two complete mitogenomes from Pezizales have been published in the NCBI database, including Pyronema omphalodes (Nowrousian, 2016) and Morchella importuna (Liu et al., 2019). No mitogenome of Tuberaceae has been reported. The rapid development of the next-generation sequencing (NGS) technology and the third-generation sequencing technology (Giordano et al., 2017; Zascavage et al., 2019) provides us with the possibility to obtain complex fungal mitogenomes, which promotes our understanding of fungal evolution and phylogeny.
In the present study, the complete mitogenome of Tuber calosporum was sequenced using the NGS technology and successfully assembled. The aims of this study are (1) to reveal the features of T. calosporum mitogenomes and the similarities or variations between Pezizales mitogenomes; and (2) to analyze the phylogenetic status of Tuber calosporum in Ascomycota based on a combined mitochondrial gene set. As the first reported mitogenome in the Tuberaceae, the mitogenome of T. calosporum will help to understand the phylogeny and evolution of truffles and provide reference for the acquisition of more truffle mitogenomes.
Materials and Methods
Assembly and Annotations of Mitogenome
The raw sequencing data of T. calosporum were obtained from the 90 mushroom genome sequencing project (Li H. et al., 2018), under the following Sequence Read Archive (SRA) accession numbers: SRR5804115 and SRR5804116. The raw sequencing data of T. calosporum was generated by the Illumina HiSeq 4000 platform, and a total of 22.5 Gbp data were obtained. A series of quality control steps were conducted to obtain clean reads from the raw sequencing data, including removal of adapters (Schubert et al., 2016) and filtering sequences with low quality value. The obtained clean reads were used to assemble the complete mitogenome of T. calosporum by SPAdes 3.9.0 (Bankevich et al., 2012). The software MITObim V1.9 (Hahn et al., 2013) was used to fill in the gaps between the contigs obtained in the previous step. Since organelle sequences usually have more copies than nuclear gene sequences, the coverage is generally higher than that of nuclear genome sequences when assembled. In addition, we also used MIRA and NOVO Plasty to test the assembly of this study. All the software obtained mitochondrial sequences identical to this study, which proves that the mitogenome obtained in the present study is reliable. The obtained complete mitogenome of T. calosporum was further annotated according to methods we previously described (Li et al., 2018a). Briefly, the protein-coding genes (PCGs), introns, rRNA genes, and tRNA genes of the T. calosporum mitogenome were initially annotated using the MITOS (Bernt et al., 2013) and MFannot (Valach et al., 2014), using the Mold, Protozoan, and Coelenterate Mitochondrial Code (genetic code 4). Then the PCGs were predicted or modified by using the NCBI Open Reading Frame Finder (Coordinators, 2017) and further annotated by BLASTP searches against the NCBI non-redundant protein sequence database (Bleasby and Wootton, 1990). Intron-exon borders of PCGs were verified using exonerate v2.2 (Slater and Birney, 2005). The tRNA genes in the T. calosporum mitogenome were also predicted by the tRNAscan-SE v1.3.1 (Lowe and Chan, 2016). The OGDraw v1.2 software (Lohse et al., 2013) was used to draw graphical maps of the T. calosporum mitogenome.
Sequence and Repetitive Elements Analyses of the T. calosporum Mitogenome
The base composition of the T. calosporum mitogenome was calculated using DNASTAR Lasergene v7.11. Strand asymmetries of the T. calosporum mitogenome and other related mitogenomes were assessed according to the following formulas: AT skew = [A – T] / [A + T] and GC skew = [G – C] / [G + C] (Li et al., 2019a). To identify whether there were interspersed repeats or intragenomic duplications of large fragments throughout the T. calosporum mitogenome, we conducted BLASTN searches (Chen et al., 2015) of the mitogenome against itself based on an E-value of <10–10. In addition, Tandem Repeats Finder (Benson, 1999) was used to detect tandem repeats (>10 bp in length) in the T. calosporum mitogenome. REPuter (Kurtz et al., 2001) was used to identify forward (direct), reverse, complemented, and palindromic (reverse complement) repeats in the T. calosporum mitogenome. We also conducted BLASTN searches of the T. calosporum mitogenome against its published nuclear genome (QFET00000000.1) (Li H. et al., 2018) to identify if there were gene segments naturally transferring between nuclear and mitochondrial genomes.
Comparative Mitogenome and Phylogenetic Analyses
The genome sizes, base composition, gene numbers, intron numbers, gene content between different Pezizales mitogenomes, and the largest mitogenome in Basidiomycota (Rhizoctonia solani) were compared to assess variations or conservativeness of mitogenomes. To investigate the phylogenetic status of T. calosporum in the Ascomycota phylum, we constructed a phylogenetic tree of 104 species based on the combined mitochondrial gene set (15 core PCGs + two rRNA genes) (Li et al., 2018d). Rhizoctonia solani (Losada et al., 2014) and Blastosporella zonata (Nieuwenhuis et al., 2019) from the Basidiomycota phylum and Chytriomyces confervae (van de Vossenberg et al., 2018) from Chytridiomycota were set as outgroups. MAFFT v7.037 software (Katoh et al., 2019) was used to align individual mitochondrial genes. Then the aligned mitochondrial genes were concatenated into a combined mitochondrial gene set using SequenceMatrix v1.7.8 (Vaidya et al., 2011). A partition homogeneity test was used to detect potential phylogenetic conflicts among different mitochondrial genes. PartitionFinder 2.1.1 (Lanfear et al., 2017) was used to determine best-fit models of evolution and partitioning schemes for the mitochondrial gene set. Bayesian inference (BI) and maximum likelihood (ML) methods were used to construct phylogenetic trees. BI analysis was conducted using the MrBayes v3.2.6 (Ronquist et al., 2012) software and ML analysis was performed with RAxML v 8.0.0 (Stamatakis, 2014). When we conducted BI analysis, two independent runs with four chains (three heated and one cold) each were conducted simultaneously for 2 × 106 generations. Each run was sampled every 100 generations. We assumed that stationarity had been reached when the estimated sample size (ESS) was greater than 100, and the potential scale reduction factor (PSRF) approached 1.0. The first 25% samples were discarded as burn-in, and the remaining trees were used to calculate Bayesian posterior probabilities (BPP) in a 50% majority-rule consensus tree. Bootstrap values (BS) were assessed through an ultrafast bootstrap approach with 10,000 replicates.
Data Availability
The complete mitogenome of T. calosporum was deposited in the GenBank database under the accession number MT028548.
Results
Mitogenome Features and Composition
The complete mitogenome of T. calosporum was composed of circular DNA molecules with a size of 287,403 bp (Figure 1). The GC content was 29.92%. Both the AT skew and GC skew were positive in the T. calosporum mitogenome (Table 1). We detected two pairs of overlapping ORFs in the mitogenome of T. calosporum, one of which located across the neighboring genes orf228 and orf211 (−10 bp) and the other of which was located between orf62 and orf84 (−28 bp) (Supplementary Table 1). The length of the intergenic sequences ranged from 0 to 6,501 bp, and the longest intergenic sequence was located across the neighboring genes orf66 and orf213 gene. Intronic regions occupied the largest proportion of the T. calosporum mitogenome, reaching 69.41% (Figure 2). Intergenic region was the second largest region, accounting for 16.76%. The protein coding region accounted for 10.20% of the entire mitogenome. The RNA genes (including tRNAs and rRNAs) were 10,421 bp long in total, accounting for 3.63% of the whole mitogenome. Comparative mitogenomic analysis indicated that intron gain was the primary factor that contributed to the size expansion of the T. calosporum mitogenome. Compared with the other three mitogenomes, the protein coding region and intergenic region reduced in T. calosporum.
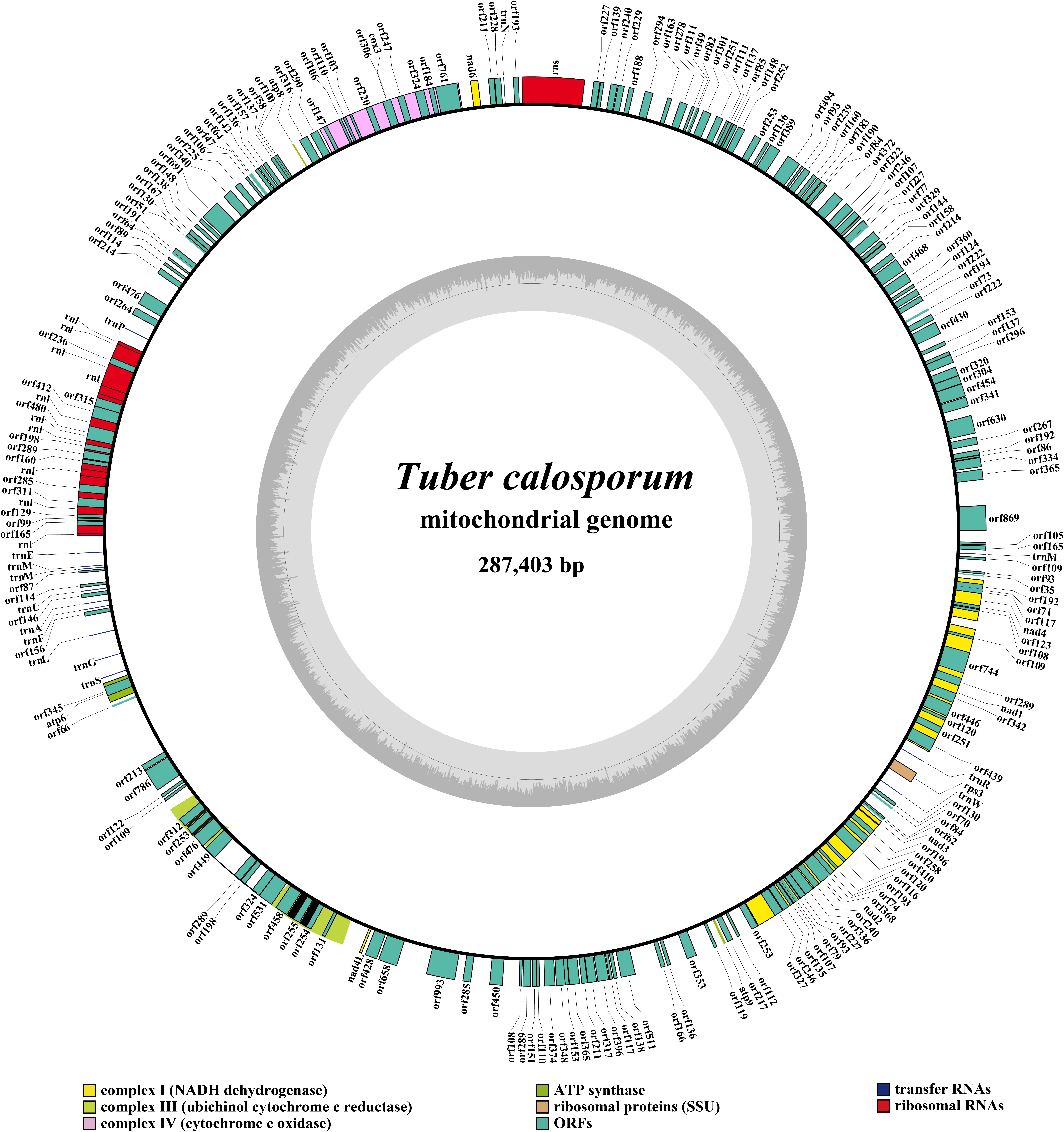
Figure 1. Circular map of the mitochondrial genome of Tuber calosporum. Genes are represented by different colored blocks.
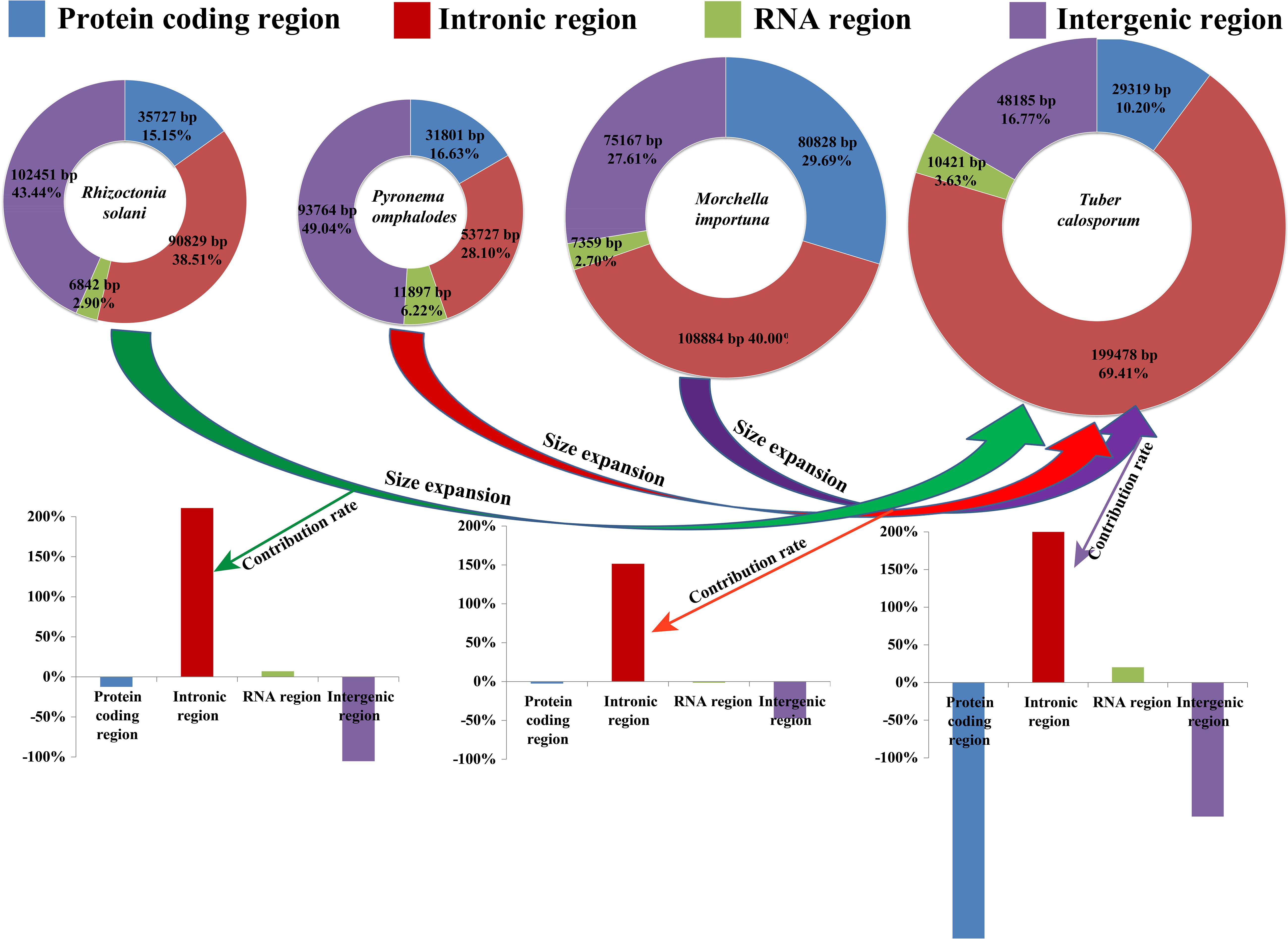
Figure 2. The protein-coding, intronic, intergenic, and RNA gene region proportions of the entire mitochondrial genomes of Tuber calosporum, Pyronema omphalodes, Morchella importuna, and Rhizoctonia solani. The bottom panel shows the contribution of different gene regions to the size expansion of the T. calosporum mitogenome.
Protein Coding Genes, tRNAs, rRNAs, and Codon Analysis
A total of 43 free standing (non-intronic) protein coding genes (PCGs) were detected in the mitogenome of T. calosporum, including 14 core PCGs for energy metabolism, one rps3 gene for transcriptional regulation, eight PCGs containing the LAGLIDADG homing endonuclease domain, 10 PCGs with the GIY-YIG homing endonuclease domain, three genes encoding DNA-directed RNA polymerase, and seven PCGs with unknown functions (Supplementary Table 1). A total of 58 introns were detected in the T. calosporum mitogenome, which were distributed in atp6, cob, cox1, cox2, cox3, nad1, nad2, nad4, nad5, and rnl genes. One hundred and seventy intronic open reading frames (ORFs) were detected in these introns, including 110 intronic ORFs containing LAGLIDADG homing endonuclease domain, 56 ORFs containing GIY-YIG homing endonuclease domain, one ORF encoding reverse transcriptase/maturase, and 3 ORFs with unknown functions.
The mitogenome of T. calosporum contained 2 rRNA genes, namely the small subunit ribosomal RNA (rns), and the large subunit ribosomal RNA (rnl) (Supplementary Table 1). Thirteen tRNA genes were detected in the T. calosporum mitogenome, which predicted structures resembled the classical clover leaf folding (Figure 3). The mitochondrial genome of T. calosporum lost trnC, trnD, trnH, trnI, trnK, trnQ, trnT, trnV, and trnY, which predicted function is the transport of cysteine, aspartate, histidine, isoleucine, lysine, glutamine, threonine, valine, and tyrosine, respectively. The mitogenome of T. calosporum contained two tRNAs with different anticodons coding for leucine and three tRNAs with the same anticodon coding for methionine. The length of individual tRNAs ranged from 71 to 88 bp.
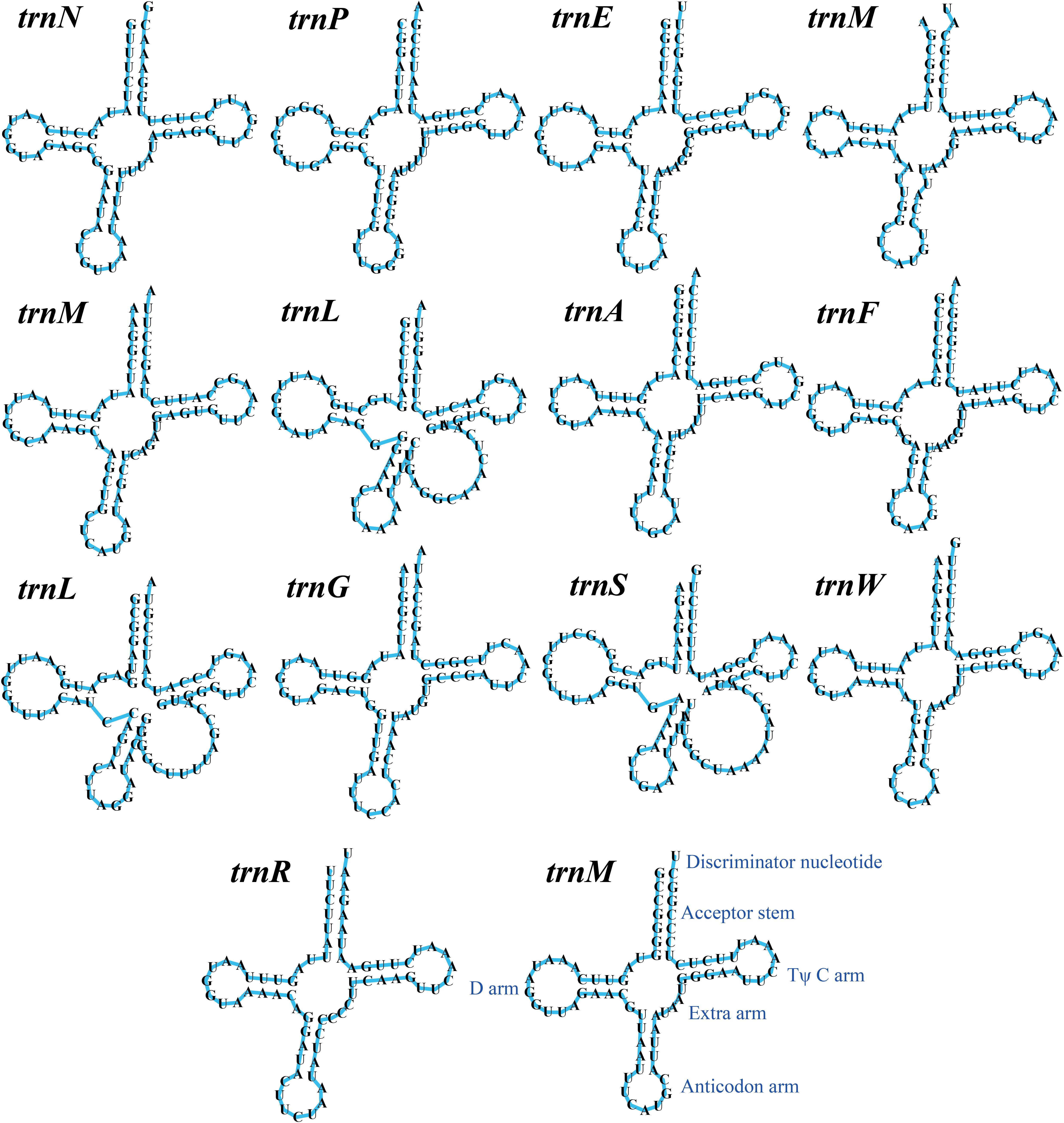
Figure 3. Putative secondary structures of the 14 tRNA genes identified in the mitochondrial genome of Tuber calosporum. All genes are shown in order of occurrence in the mitochondrial genome of T. calosporum starting from trnN.
Codon usage analysis indicated that the most frequently used codons in the mitogenome of T. calosporum were AAA (for lysine; Lys), TTT (for phenylalanine; Phe), TTA (for leucine; Leu), AAT (for asparagine; Asn), ATT (for isoleucine; Ile), and TAT (for Tyrosine; Tyr) (Figure 4 and Supplementary Table 2). The frequent use of A and T in codon contributed to the high AT content in the T. calosporum mitogenome (average: 70.08%).
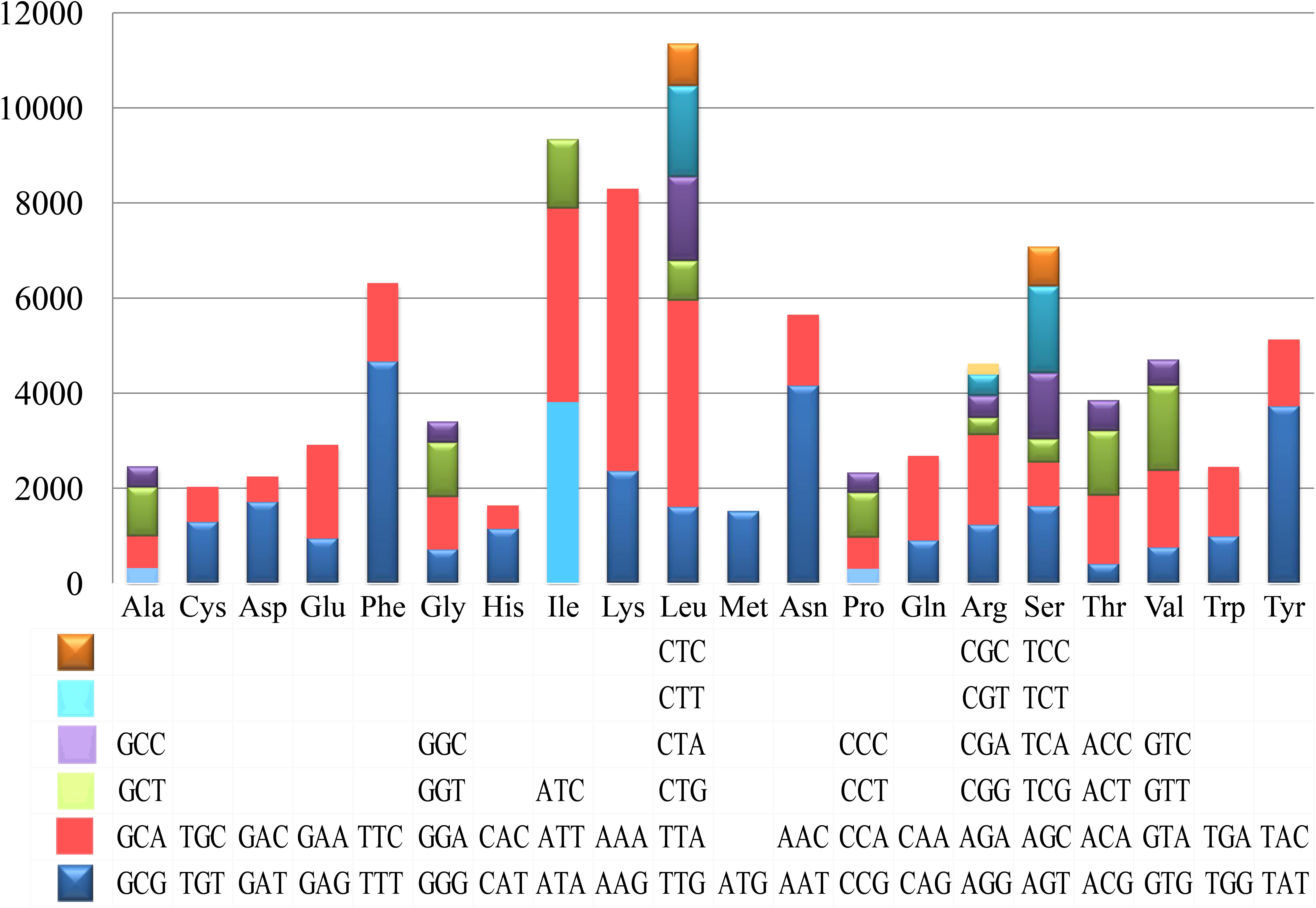
Figure 4. Codon usage in the mitochondrial genomes of Tuber calosporum. Frequency of codon usage is plotted on the y-axis.
Repetitive Elements Analysis
We conducted BLASTN searches of the T. calosporum mitogenome against itself and identified 181 repetitive sequences in the T. calosporum mitogenome (Supplementary Table 3). The size of these repeats ranged from 35 to 999 bp, with pair-wise nucleotide similarities ranging from 74.68 to 100%. The longest repeats were detected in the third intron of cox3 gene and also in the intergenic region of trnM and cox1. Repetitive sequences detected by BLASTN searches accounted for 12.83% of the T. calosporum mitogenome. Through Tandem Repeats Finder, we detected 15 tandem repeats (Supplementary Table 4), which accounted for 0.097% of the entire mitogenome. The longest tandem sequence (77 bp) was detected in the thirteenth intron of the cox1 gene. Based on REPuter, a total of 50 forward repeats, accounting for 1.18% of the whole mitogenome, were detected (Supplementary Table 5).
We conducted BlastN searches of the T. calosporum mitogenome against its nuclear genome (Li H. et al., 2018), and identified 109 aligned fragments between the mitogenome and nuclear genome, with a total length of 32.91 kb (Supplementary Table 6). The length of these aligned fragments ranged from 102 to 1,360 bp, with sequence identities between 73.08 and 94.63%. The largest aligned fragment was found located in the sixth and seventh introns of nad5 gene and encompassed the seventh exon of nad5 gene. The second largest aligned fragments were located in the third intron of cox3 gene, and also in the intergenic region between trnE and trnM, with a length of 999 bp. The presence of large fragments aligned between the mitochondrial and nuclear genomes of the T. calosporum mitogenome indicated that genetic transfer between mitochondrial and nuclear genome has occurred in the evolution of T. calosporum.
Comparative Mitogenomics
Gene orders of the three Pezizales mitogenomes varied greatly between different species (Figure 5). We observed four gene relocations, two gene position exchanges, and one gene duplication event in the three Pezizales mitogenomes. Gene relocations involved box I, box IV, box V, and box VI, which harbored atp6, atp8, nad4L, nad5, rps3, and nad1. Gene position exchanges involved box II and box III, which harbored nad4 and cob genes. The M. importuna mitogenome (Liu et al., 2019) had one duplication of atp8 gene. Gene migration, transposition, and duplication observed in Pezizales mitogenomes indicated that frequent gene rearrangements occurred during the mitochondrial evolution of Pezizales species.
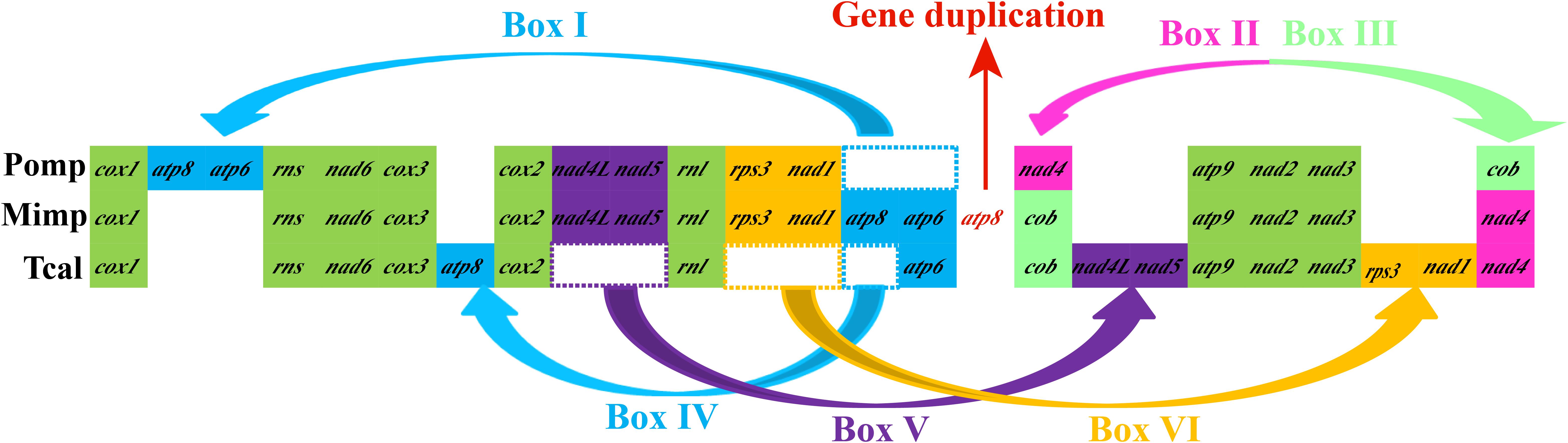
Figure 5. Mitochondrial gene arrangement analysis of 3 Pezizales mitogenomes. 15 core protein coding genes and 2 rRNA genes were included in the gene arrangement, starting from cox1 gene.
The mitogenome of T. calosporum was 5.57 and 50.32% larger than the two species from Pezizales, M. importuna (Liu et al., 2019) and P. omphalodes (Nieuwenhuis et al., 2019), respectively (Table 1). The GC content of the T. calosporum mitogenome was the lowest among Pezizales mitogenomes. Both the AT and GC skews were larger in T. calosporum mitogenome than in other two Pezizales mitogenomes, indicating high preferences for As and Gs in the T. calosporum mitogenome. The number of PCGs in T. calosporum was between M. importuna (the highest) and P. omphalodes (the lowest). However, the T. calosporum contained the greatest number of introns and intronic ORFs among the Pezizales mitogenomes detected. Two rRNA genes were detected in all the three Pezizales species. In addition, 14–31 tRNA genes were detected in the three Pezizales species. The mitogenome of T. calosporum had lost some of tRNA genes compared with the other two Pezizales mitogenomes.
Phylogenetic Analysis
We obtained well-supported and identical tree topologies using maximum likelihood (ML) and bayesian inference (BI) methods based on the combined mitochondrial gene set (15 core PCGs + two rRNA genes) (Figure 6). All major clades within the trees had good support values (BPP ≥ 0.97; BS ≥ 98). Based on the phylogenetic analysis, the 101 Ascomycota species could be divided into 24 major clades, corresponding to the orders Diaporthales, Sordariales, Glomerellales, Chaetothyriales, Eurotiales, Onygenales, Xylariales, Microascales, Hypocreales, etc. The clade related to Pezizales was recovered as [P. omphalodes+ (M. importuna + T. calosporu)]. The result indicated that T. calosporum mitogenome had a close relationship with M. importuna. The phylogenetic analysis showed that mitochondrial genes were effective molecular markers for phylogenetic analysis of Ascomycota species.
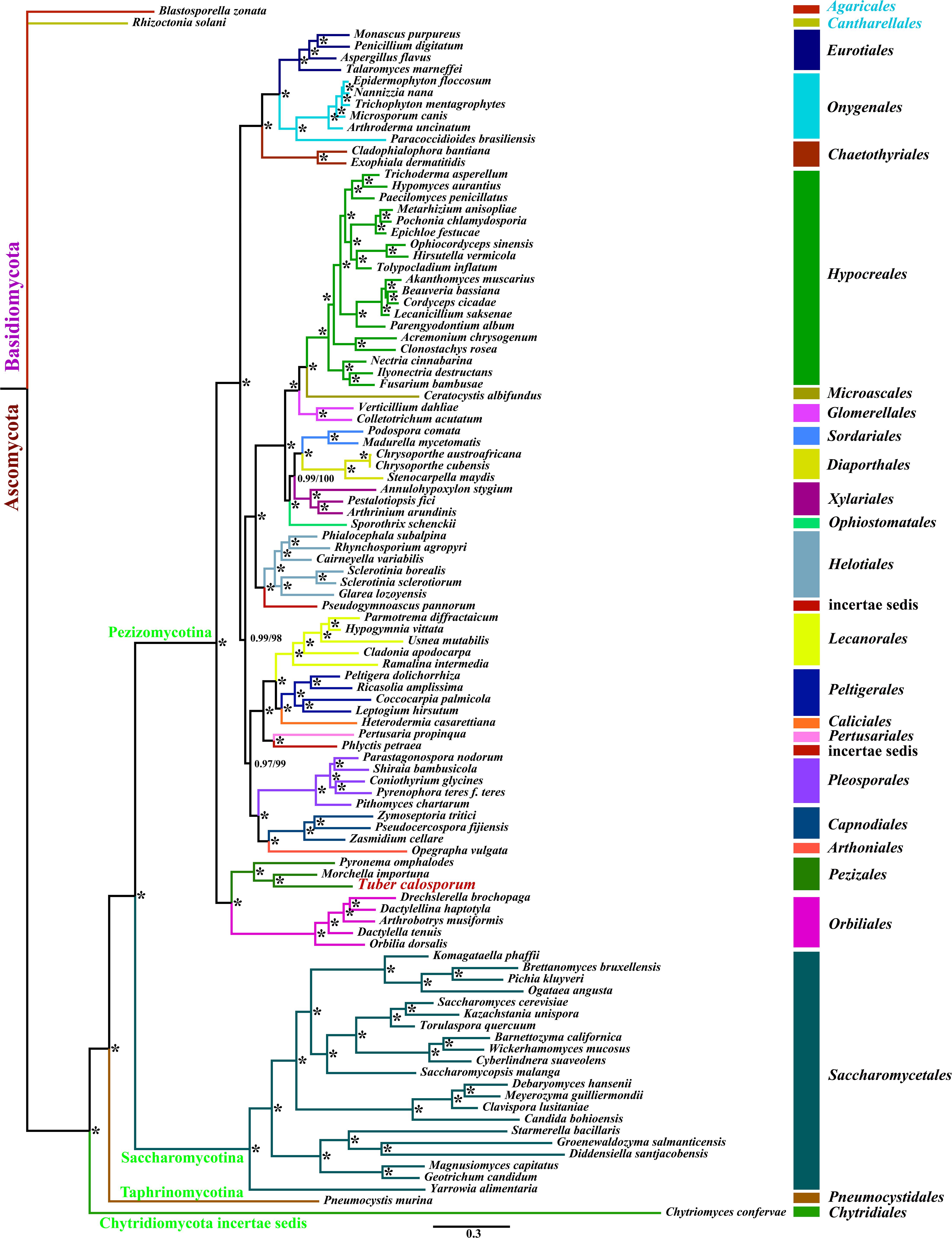
Figure 6. Molecular phylogeny of 104 fungal species based on Bayesian inference (BI) and Maximum Likelihood (ML) analyses of 15 protein coding genes and two rRNA genes. Support values are bayesian posterior probabilities (before slash) and bootstrap values (after slash). The asterisk indicates that the BPP and BS values are 1 and 100, respectively. Species and NCBI accession numbers for genomes used in the phylogenetic analysis are provided in Supplementary Table 6.
Discussion
The mitogenome was reported to have been derived from alphabacteria through endosymbiosis (Lang et al., 1999). In the long-term evolution, most of the ancient mitochondrial genes have been integrated into the nuclear genome, which is considered to have many advantages (Adams et al., 2002; Adams and Palmer, 2003). However, some genes also remain in the mitogenome for local control of oxidative phosphorylation and transcriptional regulation (Allen, 2015). Many physiological activities of eukaryotes need to be completed under the joint control of nuclear genome and mitogenome. The mitogenome size is more variable in fungi than in animals, which is believed to be closely related to the accumulation of repeat sequences, introns, plasmid related regions, and horizontal transferring genes (Himmelstrand et al., 2014; Mardanov et al., 2014). Up to now, the largest mitogenomes of Ascomycota and Basidiomycota are M. importuna (Liu et al., 2019) and R. solani (Losada et al., 2014), respectively. Accumulations of repeat sequences and introns contributed to the expansion of the two mitogenomes. In the present study, we obtained the mitogenome of T. calosporum. Compared with M. importuna and R. solani, the mitogenome of T. calosporum contained more introns and intronic ORFs. In addition, the intronic region accounted for 69.41% of the whole genome of T. calosporum, which was much higher than that of M. importuna and R. solani. The results indicated that intron gain was the primary factor contributing to the significant expansion of T. calosporum mitogenome, while the protein-coding region and intergenic region were reduced in T. calosporum mitogenome compared with the other three mitogenomes.
The GC content of mitogenomes varies between different species, which is thought to be affected by biases of reconstitution-related DNA repair, selection and mutation bias (Li et al., 2018c, 2019b). In the present study, we found the GC content of T. calosporum was significantly lower than the other two Pezizales mitogenomes reported. According to the second parity rule, each base in the complementary DNA strand exists at an approximately equal frequency if there is no mutation or selection bias (Chen et al., 2014). However, we found excesses of Gs and As (but not Cs and Ts) in the replication leading strands of Pezizales mitogenomes. Both the AT and GC skews were larger in T. calosporum mitogenome than in other 2 Pezizales mitogenomes, indicating unique evolutionary characteristics of the T. calosporum mitogenome.
Previous studies have shown that mitochondrial gene transfer to nuclear genome is a trend, which is considered having several advantages (Adams and Palmer, 2003). However, there were still a small number of genes that were transferred from nuclei to mitogenomes (Zhao et al., 2018). Natural gene transfer between nuclear and mitochondrial genomes showed great effects on species differentiation and functional evolution of mitogenomes (Adams et al., 2002). In the present study, large aligned fragments were observed between nuclear and mitochondrial genomes of T. calosporum, indicating gene fragments may have transferred between mitochondrial and nuclear genomes of T. calosporum in the process of evolution. Several non-conserved PCGs were also identified in the mitogenomes of T. calosporum, which encoded homing endonucleases, DNA polymerase, and other proteins with unknown function. These results suggest that there are still a number of undiscovered proteins in the T. calosporum mtiogenome that warrant future investigation. Homing endonucleases initiate transfer of introns, inteins, and themselves by generating strand breaks in cognate alleles that lack the intervening sequence, as well as in additional ectopic sites that broaden the range of intron and intein mobility (Stoddard, 2005). Interestingly, we found that the mitogenome of T. calosporum lost tRNA genes encoding for cysteine, aspartate, histidine, isoleucine, lysine, glutamine, threonine, valine, and tyrosine. Previous studies indicated that tRNA import and superwobble was the functional replacement for tRNA genes loss in mitogenomes (Rogalski et al., 2008; Salinas et al., 2008; Saunier et al., 2014; Warren and Sloan, 2020). Mitochondrial tRNA import has been experimentally documented in several organisms (Salinas et al., 2008). In contrast to mitochondrial protein import, tRNA import has a polyphyletic evolutionary origin (Schneider and Marechal-Drouard, 2000). Each organism recruits distinct housekeeping proteins to direct mitochondrial import (Salinas et al., 2008), but the overall process of mitochondrial protein import is conserved (Baker et al., 2007). ‘Superwobble’ in which a tRNA species with an unmodified U in the wobble position reads all four nucleotides in the third codon position, indicating that a reduced tRNA set could still suffice in some species (Rogalski et al., 2008). The functional replacement of tRNA gene loss and the effect to the synthesis of mitochondrial protein in T. calosporum needs to be further studied.
Mitochondrial gene arrangement can provide useful information for understanding the origin and evolution of species (Zheng et al., 2018; Li et al., 2020c), because mitochondrial genes of all species derived from a common ancestor (Lang et al., 1999). Mitochondrial gene rearrangements in animals have been studied extensively, and several models have been proposed to explain these rearrangement events (Boore, 1999; Perseke et al., 2008). Plant mitochondrial gene orders are highly variable owing to high recombination rates of their mitogenomes (Galtier, 2011; Liu et al., 2011). However, compared to other lineages, fungal mitochondrial arrangements have been less investigated, despite their importance in the global ecosystem (Hamari et al., 2001; Aguileta et al., 2014). In the present study, large-scale gene rearrangements were observed in the three Pezizales mitogenomes, which involved gene relocations, position exchanges, and duplication. Previous studies found that gene rearrangements in fungi were closely related to recombination and accumulation of repetitive sequences in fungal mitogenomes (Aguileta et al., 2014). Interestingly, we found the three Pezizales mitogenomes contained rich content of repeat sequences (over 12% of each mitogenomes). The accumulation of repeat sequences may lead to variable gene arrangements in the three Pezizales mitogenomes.
The Tuber genus is a group of important ectomycorrhizal fungi, which comprises numerous recognized species (Wan et al., 2016; Guevara-Guerrero et al., 2018; Polemis et al., 2019). Limited morphological features and overlapping of some features render it difficult to differentiate some Tuber species accurately. Reliable molecular markers are important tools for accurate identification and classification of Tuber species (Fan et al., 2016; Gryndler et al., 2017; Qiao et al., 2018), also for understanding origin of Pezizales species. Mitochondrial genes have been widely used in phylogeny and population genetic study of animals, plants, and some classes of fungi (Dai et al., 2018; Doyle et al., 2018; Li et al., 2019c; Wang et al., 2020). However, the number of known mitogenomes in Pezizales is very limited, which limits our understanding of the origin and evolution of Pezizales species. In this study, we obtained a well-supported phylogenetic tree based on combined mitochondrial gene set using two phylogenetic inference methods, which divided 101 Ascomycota species into 24 independent clades. The present study indicated that mitochondrial genes are reliable molecular markers to reconstruct phylogeny of Ascomycota.
Data Availability Statement
The complete mitogenome of T. calosporum was deposited in the GenBank database under the accession number MT028548.
Author Contributions
QL and XL conceived and designed the experiments. LL, XW, ZB, WT, XH, LY, and BZ analyzed the data. QL and XL wrote and reviewed the manuscript. All authors contributed to the article and approved the submitted version.
Funding
This work was supported by the National Natural Science Foundation of China (No. 31900079), Sichuan Mushroom Innovation Team (SCCXTD-2021-07), and the project of Sichuan Academy of Agricultural Sciences (2020BJRC003).
Conflict of Interest
The authors declare that the research was conducted in the absence of any commercial or financial relationships that could be construed as a potential conflict of interest.
Supplementary Material
The Supplementary Material for this article can be found online at: https://www.frontiersin.org/articles/10.3389/fmicb.2020.591453/full#supplementary-material
Footnotes
References
Adams, K. L., and Palmer, J. D. (2003). Evolution of mitochondrial gene content: gene loss and transfer to the nucleus. Mol. Phylogenet. Evol. 29, 380–395. doi: 10.1016/s1055-7903(03)00194-5
Adams, K. L., Qiu, Y. L., Stoutemyer, M., and Palmer, J. D. (2002). Punctuated evolution of mitochondrial gene content: high and variable rates of mitochondrial gene loss and transfer to the nucleus during angiosperm evolution. Proc. Natl. Acad. Sci. U.S.A. 99, 9905–9912. doi: 10.1073/pnas.042694899
Aguileta, G., de Vienne, D. M., Ross, O. N., Hood, M. E., Giraud, T., Petit, E., et al. (2014). High variability of mitochondrial gene order among fungi. Genome Biol. Evol. 6, 451–465. doi: 10.1093/gbe/evu028
Allen, J. F. (2015). Why chloroplasts and mitochondria retain their own genomes and genetic systems: colocation for redox regulation of gene expression. Proc. Natl. Acad. Sci. U.S.A. 112, 10231–10238. doi: 10.1073/pnas.1500012112
Andersen, M. M., and Balding, D. J. (2018). How many individuals share a mitochondrial genome? PLoS Genet. 14:e1007774. doi: 10.1371/journal.pgen.1007774
Baker, M. J., Frazier, A. E., Gulbis, J. M., and Ryan, M. T. (2007). Mitochondrial protein-import machinery: correlating structure with function. Trends Cell Biol. 17, 456–464. doi: 10.1016/j.tcb.2007.07.010
Bankevich, A., Nurk, S., Antipov, D., Gurevich, A. A., Dvorkin, M., Kulikov, A. S., et al. (2012). SPAdes: a new genome assembly algorithm and its applications to single-cell sequencing. J. Comput. Biol. 19, 455–477. doi: 10.1089/cmb.2012.0021
Benson, G. (1999). Tandem repeats finder: a program to analyze DNA sequences. Nucleic Acids Res. 27, 573–580. doi: 10.1093/nar/27.2.573
Bernt, M., Donath, A., Juhling, F., Externbrink, F., Florentz, C., Fritzsch, G., et al. (2013). MITOS: improved de novo metazoan mitochondrial genome annotation. Mol. Phylogenet. Evol. 69, 313–319. doi: 10.1016/j.ympev.2012.08.023
Bleasby, A. J., and Wootton, J. C. (1990). Construction of validated, non-redundant composite protein sequence databases. Protein Eng. 3, 153–159. doi: 10.1093/protein/3.3.153
Boore, J. L. (1999). Animal mitochondrial genomes. Nucleic Acids Res. 27, 1767–1780. doi: 10.1093/nar/27.8.1767
Caboni, P., Scano, P., Sanchez, S., Garcia-Barreda, S., Corrias, F., and Marco, P. (2020). Multi-platform metabolomic approach to discriminate ripening markers of black truffles (Tuber melanosporum). Food Chem. 319:126573. doi: 10.1016/j.foodchem.2020.126573
Chen, H., Sun, S., Norenburg, J. L., and Sundberg, P. (2014). Mutation and selection cause codon usage and bias in mitochondrial genomes of ribbon worms (Nemertea). PLoS One 9:e85631. doi: 10.1371/journal.pone.0085631
Chen, Y., Ye, W., Zhang, Y., and Xu, Y. (2015). High speed BLASTN: an accelerated MegaBLAST search tool. Nucleic Acids Res. 43, 7762–7768. doi: 10.1093/nar/gkv784
Coordinators, N. R. (2017). Database resources of the national center for biotechnology information. Nucleic Acids Res. 44, D7–D19. doi: 10.1093/nar/gkv1290
Corrales, A., Henkel, T. W., and Smith, M. E. (2018). Ectomycorrhizal associations in the tropics - biogeography, diversity patterns and ecosystem roles. New Phytol. 220, 1076–1091. doi: 10.1111/nph.15151
Dai, L. S., Kausar, S., Abbas, M. N., and Wang, T. T. (2018). Complete sequence and characterization of the Ectropis oblique mitochondrial genome and its phylogenetic implications. Int. J. Biol. Macromol. 107, 1142–1150. doi: 10.1016/j.ijbiomac.2017.09.093
Ding, Y., Teng, Y. S., Zhuo, G. C., Xia, B. H., and Leng, J. H. (2019). The mitochondrial tRNAHis G12192A mutation may modulate the clinical expression of deafness-associated tRNAThr G15927A mutation in a chinese pedigree. Curr. Mol. Med. 19, 136–146. doi: 10.2174/1566524019666190308121552
Doyle, J. M., Bell, D. A., Bloom, P. H., Emmons, G., Fesnock, A., Katzner, T. E., et al. (2018). Andrew DeWoody J: new insights into the phylogenetics and population structure of the prairie falcon (Falco mexicanus). BMC Genomics 19:233. doi: 10.1186/s12864-018-4615-z
du Toit, Z., du Plessis, M., Dalton, D. L., Jansen, R., Paul Grobler, J., and Kotze, A. (2017). Mitochondrial genomes of African pangolins and insights into evolutionary patterns and phylogeny of the family Manidae. BMC Genomics 18:746. doi: 10.1186/s12864-017-4140-5
Fan, L., Zhang, P. R., Yan, X. Y., and Li, Y. (2016). Phylogenetic analyses of Chinese Tuber species that resemble T. borchii reveal the existence of the new species T. hubeiense and T. wumengense. Mycologia 108, 354–362. doi: 10.3852/14-349
Fourie, G., Van der Merwe, N. A., Wingfield, B. D., Bogale, M., Wingfield, M. J., and Steenkamp, E. T. (2018). Mitochondrial introgression and interspecies recombination in the Fusarium fujikuroi species complex. IMA Fungus 9, 37–48. doi: 10.5598/imafungus.2018.09.01.04
Franco, A. R., Sousa, N. R., Ramos, M. A., Oliveira, R. S., and Castro, P. M. (2014). Diversity and persistence of ectomycorrhizal fungi and their effect on nursery-inoculated Pinus pinaster in a post-fire plantation in Northern Portugal. Microb. Ecol. 68, 761–772. doi: 10.1007/s00248-014-0447-9
Galtier, N. (2011). The intriguing evolutionary dynamics of plant mitochondrial DNA. BMC Biol. 9:61. doi: 10.1186/1741-7007-9-61
Giordano, F., Aigrain, L., Quail, M. A., Coupland, P., Bonfield, J. K., Davies, R. M., et al. (2017). De novo yeast genome assemblies from MinION, PacBio and MiSeq platforms. Sci. Rep. 7:3935.
Gryndler, M., Smilauer, P., Stovicek, V., Novakova, K., Hrselova, H., and Jansa, J. (2017). Truffle biogeography-A case study revealing ecological niche separation of different Tuber species. Ecol. Evol. 7, 4275–4288. doi: 10.1002/ece3.3017
Guevara-Guerrero, G., Bonito, G., Smith, M. E., Healy, R., Grupe, A. C. II, Cazares, E., et al. (2018). Tuber aztecorum sp. nov., a truffle species from Mexico belonging to the Maculatum clade (Tuberaceae, Pezizales). MycoKeys 30, 61–72. doi: 10.3897/mycokeys.30.22887
Hahn, C., Bachmann, L., and Chevreux, B. (2013). Reconstructing mitochondrial genomes directly from genomic next-generation sequencing reads–a baiting and iterative mapping approach. Nucleic Acids Res. 41:e129. doi: 10.1093/nar/gkt371
Hamari, Z., Juhasz, A., Gacser, A., Kucsera, J., Pfeiffer, I., and Kevei, F. (2001). Intron mobility results in rearrangement in mitochondrial DNAs of heterokaryon incompatible Aspergillus japonicus strains after protoplast fusion. Fungal Genet. Biol. 33, 83–95. doi: 10.1006/fgbi.2001.1272
Himmelstrand, K., Olson, A., Brandstrom Durling, M., Karlsson, M., and Stenlid, J. (2014). Intronic and plasmid-derived regions contribute to the large mitochondrial genome sizes of Agaricomycetes. Curr. Genet 60, 303–313. doi: 10.1007/s00294-014-0436-z
Katoh, K., Rozewicki, J., and Yamada, K. D. (2019). MAFFT online service: multiple sequence alignment, interactive sequence choice and visualization. Brief Bioinform. 20, 1160–1166. doi: 10.1093/bib/bbx108
Kurtz, S., Choudhuri, J. V., Ohlebusch, E., Schleiermacher, C., Stoye, J., and Giegerich, R. (2001). REPuter: the manifold applications of repeat analysis on a genomic scale. Nucleic Acids Res. 29, 4633–4642. doi: 10.1093/nar/29.22.4633
Lanfear, R., Frandsen, P. B., Wright, A. M., Senfeld, T., and Calcott, B. (2017). PartitionFinder 2: new methods for selecting partitioned models of evolution for molecular and morphological phylogenetic analyses. Mol. Biol. Evol. 34, 772–773.
Lang, B. F., Gray, M. W., and Burger, G. (1999). Mitochondrial genome evolution and the origin of eukaryotes. Annu. Rev. Genet. 33, 351–397. doi: 10.1146/annurev.genet.33.1.351
Li, H., Wu, S., Ma, X., Chen, W., Zhang, J., Duan, S., et al. (2018). The genome sequences of 90 mushrooms. Sci. Rep. 8:9982.
Li, Q., Chen, C., Xiong, C., Jin, X., Chen, Z., and Huang, W. (2018a). Comparative mitogenomics reveals large-scale gene rearrangements in the mitochondrial genome of two Pleurotus species. Appl. Microbiol. Biotechnol. 102, 6143–6153. doi: 10.1007/s00253-018-9082-6
Li, Q., He, X., Ren, Y., Xiong, C., Jin, X., Peng, L., et al. (2020a). Comparative mitogenome analysis reveals mitochondrial genome differentiation in ectomycorrhizal and asymbiotic amanita species. Front. Microbiol. 11:1382. doi: 10.3389/fmicb.2020.01382
Li, Q., Ren, Y., Shi, X., Peng, L., Zhao, J., Song, Y., et al. (2019a). Comparative mitochondrial genome analysis of two ectomycorrhizal fungi (Rhizopogon) reveals dynamic changes of intron and phylogenetic relationships of the subphylum agaricomycotina. Int. J. Mol. Sci. 20:5167. doi: 10.3390/ijms20205167
Li, Q., Ren, Y., Xiang, D., Shi, X., Zhao, J., Peng, L., et al. (2020b). Comparative mitogenome analysis of two ectomycorrhizal fungi (Paxillus) reveals gene rearrangement, intron dynamics, and phylogeny of basidiomycetes. IMA Fungus 11:12.
Li, Q., Wang, Q., Chen, C., Jin, X., Chen, Z., Xiong, C., et al. (2018b). Characterization and comparative mitogenomic analysis of six newly sequenced mitochondrial genomes from ectomycorrhizal fungi (Russula) and phylogenetic analysis of the Agaricomycetes. Int. J. Biol. Macromol. 119, 792–802. doi: 10.1016/j.ijbiomac.2018.07.197
Li, Q., Wang, Q., Jin, X., Chen, Z., Xiong, C., Li, P., et al. (2019b). Characterization and comparative analysis of six complete mitochondrial genomes from ectomycorrhizal fungi of the Lactarius genus and phylogenetic analysis of the Agaricomycetes. Int. J. Biol. Macromol. 121, 249–260. doi: 10.1016/j.ijbiomac.2018.10.029
Li, Q., Wang, X., Chen, X., and Han, B. (2018c). Complete mitochondrial genome of the tea looper caterpillar, Ectropis obliqua (Lepidoptera: Geometridae) with a phylogenetic analysis of Geometridae. Int. J. Biol. Macromol. 114, 491–496. doi: 10.1016/j.ijbiomac.2018.02.038
Li, Q., Xiang, D., Wan, Y., Wu, Q., Wu, X., Ma, C., et al. (2019c). The complete mitochondrial genomes of five important medicinal Ganoderma species: features, evolution, and phylogeny. Int. J. Biol. Macromol. 139, 397–408. doi: 10.1016/j.ijbiomac.2019.08.003
Li, Q., Yang, L., Xiang, D., Wan, Y., Wu, Q., Huang, W., et al. (2020c). The complete mitochondrial genomes of two model ectomycorrhizal fungi (Laccaria): features, intron dynamics and phylogenetic implications. Int. J. Biol. Macromol. 145, 974–984. doi: 10.1016/j.ijbiomac.2019.09.188
Li, Q., Yang, M., Chen, C., Xiong, C., Jin, X., Pu, Z., et al. (2018d). Characterization and phylogenetic analysis of the complete mitochondrial genome of the medicinal fungus Laetiporus sulphureus. Sci. Rep. 8:9104.
Li, X., Ye, L., Zhang, X., Tan, H., and Li, Q. (2020). Root-tip cutting and uniconazole treatment improve the colonization rate of Tuber indicum on Pinus armandii seedlings in the greenhouse. Microb. Biotechnol. 13, 535–547. doi: 10.1111/1751-7915.13511
Liu, W., Cai, Y., Zhang, Q., Chen, L., Shu, F., Ma, X., et al. (2019). The mitochondrial genome of Morchella importuna (272.2 kb) is the largest among fungi and contains numerous introns, mitochondrial non-conserved open reading frames and repetitive sequences. Int. J. Biol. Macromol. 143, 373–381. doi: 10.1016/j.ijbiomac.2019.12.056
Liu, Y., Xue, J. Y., Wang, B., Li, L., and Qiu, Y. L. (2011). The mitochondrial genomes of the early land plants Treubia lacunosa and Anomodon rugelii: dynamic and conservative evolution. PLoS One 6:e25836. doi: 10.1371/journal.pone.0025836
Lohse, M., Drechsel, O., Kahlau, S., and Bock, R. (2013). OrganellarGenomeDRAW–a suite of tools for generating physical maps of plastid and mitochondrial genomes and visualizing expression data sets. Nucleic Acids Res. 41, W575–W581.
Losada, L., Pakala, S. B., Fedorova, N. D., Joardar, V., Shabalina, S. A., Hostetler, J., et al. (2014). Mobile elements and mitochondrial genome expansion in the soil fungus and potato pathogen Rhizoctonia solani AG-3. FEMS Microbiol. Lett. 352, 165–173. doi: 10.1111/1574-6968.12387
Lowe, T. M., and Chan, P. P. (2016). tRNAscan-SE On-line: integrating search and context for analysis of transfer RNA genes. Nucleic Acids Res. 44, W54–W57.
Luevano-Martinez, L. A., Caldeira da Silva, C. C., Nicastro, G. G., Schumacher, R. I., Kowaltowski, A. J., and Gomes, S. L. (2019). Mitochondrial alternative oxidase is determinant for growth and sporulation in the early diverging fungus Blastocladiella emersonii. Fungal Biol. 123, 59–65. doi: 10.1016/j.funbio.2018.11.005
Luo, Z. B., Janz, D., Jiang, X., Gobel, C., Wildhagen, H., Tan, Y., et al. (2009). Upgrading root physiology for stress tolerance by ectomycorrhizas: insights from metabolite and transcriptional profiling into reprogramming for stress anticipation. Plant Physiol. 151, 1902–1917. doi: 10.1104/pp.109.143735
Mardanov, A. V., Beletsky, A. V., Kadnikov, V. V., Ignatov, A. N., and Ravin, N. V. (2014). The 203 kbp mitochondrial genome of the phytopathogenic fungus Sclerotinia borealis reveals multiple invasions of introns and genomic duplications. PLoS One 9:e107536. doi: 10.1371/journal.pone.0107536
Martin, F., Kohler, A., Murat, C., Balestrini, R., Coutinho, P. M., Jaillon, O., et al. (2010). Perigord black truffle genome uncovers evolutionary origins and mechanisms of symbiosis. Nature 464, 1033–1038. doi: 10.1038/nature08867
Murat, C., Kuo, A., Barry, K. W., Clum, A., Dockter, R. B., Fauchery, L., et al. (2018a). Draft genome sequence of tuber borchii vittad., a whitish edible truffle. Genome Announc. 6:e00537-18. doi: 10.1128/genomeA.00537-18
Murat, C., Payen, T., Noel, B., Kuo, A., Morin, E., Chen, J., et al. (2018b). Pezizomycetes genomes reveal the molecular basis of ectomycorrhizal truffle lifestyle. Nat. Ecol. Evol. 2, 1956–1965. doi: 10.1038/s41559-018-0710-4
Nieuwenhuis, M., van de Peppel, L. J. J., Bakker, F. T., Zwaan, B. J., and Aanen, D. K. (2019). Enrichment of G4DNA and a large inverted repeat coincide in the mitochondrial genomes of termitomyces. Genome Biol. Evol. 11, 1857–1869. doi: 10.1093/gbe/evz122
Nowrousian, M. (2016). Complete mitochondrial genome sequence of the pezizomycete pyronema confluens. Genome Announc. 4:e00355-16. doi: 10.1128/genomeA.00355-16
Perseke, M., Fritzsch, G., Ramsch, K., Bernt, M., Merkle, D., Middendorf, M., et al. (2008). Evolution of mitochondrial gene orders in echinoderms. Mol. Phylogenet. Evol. 47, 855–864. doi: 10.1016/j.ympev.2007.11.034
Polemis, E., Konstantinidis, G., Fryssouli, V., Slavova, M., Tsampazis, T., Nakkas, V., et al. (2019). Tuberpulchrosporum sp. nov., a black truffle of the Aestivum clade (Tuberaceae, Pezizales) from the Balkan peninsula. MycoKeys 47, 35–51. doi: 10.3897/mycokeys.47.32085
Qiao, P., Tian, W., Liu, P., Yu, F., Chen, J., Deng, X., et al. (2018). Phylogeography and population genetic analyses reveal the speciation of the Tuber indicum complex. Fungal Genet. Biol. 113, 14–23. doi: 10.1016/j.fgb.2018.02.001
Rogalski, M., Karcher, D., and Bock, R. (2008). Superwobbling facilitates translation with reduced tRNA sets. Nat. Struct. Mol. Biol. 15, 192–198. doi: 10.1038/nsmb.1370
Ronquist, F., Teslenko, M., van der Mark, P., Ayres, D. L., Darling, A., Hohna, S., et al. (2012). MrBayes 3.2: efficient Bayesian phylogenetic inference and model choice across a large model space. Syst. Biol. 61, 539–542.
Rubini, A., Belfiori, B., Riccioni, C., Tisserant, E., Arcioni, S., Martin, F., et al. (2011). Isolation and characterization of MAT genes in the symbiotic ascomycete Tuber melanosporum. New Phytol. 189, 710–722.
Salinas, T., Duchene, A. M., and Marechal-Drouard, L. (2008). Recent advances in tRNA mitochondrial import. Trends Biochem. Sci. 33, 320–329.
Saunier, A., Garcia, P., Becquet, V., Marsaud, N., Escudie, F., and Pante, E. (2014). Mitochondrial genomes of the Baltic clam Macoma balthica (Bivalvia: Tellinidae): setting the stage for studying mito-nuclear incompatibilities. BMC Evol. Biol. 14:259. doi: 10.1186/s12862-014-0259-z
Schneider, A., and Marechal-Drouard, L. (2000). Mitochondrial tRNA import: are there distinct mechanisms? Trends Cell Biol. 10, 509–513.
Schubert, M., Lindgreen, S., and Orlando, L. (2016). AdapterRemoval v2: rapid adapter trimming, identification, and read merging. BMC Res. Notes 9:88. doi: 10.1186/s13104-016-1900-2
Sebastiana, M., da Silva, A. B., Matos, A. R., Alcantara, A., Silvestre, S., and Malho, R. (2018). Ectomycorrhizal inoculation with Pisolithus tinctorius reduces stress induced by drought in cork oak. Mycorrhiza 28, 247–258.
Slater, G. S., and Birney, E. (2005). Automated generation of heuristics for biological sequence comparison. BMC Bioinformatics 6:31. doi: 10.1186/1471-2105-6-31
Stamatakis, A. (2014). RAxML version 8: a tool for phylogenetic analysis and post-analysis of large phylogenies. Bioinformatics 30, 1312–1313.
Vaidya, G., Lohman, D. L., and Meier, R. (2011). SequenceMatrix: concatenation software for the fast assembly of multi-gene datasets with character set and codon information. Cladistics 27, 171–180.
Valach, M., Burger, G., Gray, M. W., and Lang, B. F. (2014). Widespread occurrence of organelle genome-encoded 5S rRNAs including permuted molecules. Nucleic Acids Res. 42, 13764–13777.
van de Vossenberg, B., Brankovics, B., Nguyen, H. D. T., van Gent-Pelzer, M. P. E., Smith, D., Dadej, K., et al. (2018). The linear mitochondrial genome of the quarantine chytrid Synchytrium endobioticum; insights into the evolution and recent history of an obligate biotrophic plant pathogen. BMC Evol. Biol. 18:136. doi: 10.1186/s12862-018-1246-6
Wan, S. P., Wang, X. H., Zheng, Y., and Yu, F. Q. (2016). Tuber shidianense and T-calosporum, two new truffle species from southwest China. Mycoscience 57, 393–399.
Wang, X., Song, A., Wang, F., Chen, M., Li, X., Li, Q., et al. (2020). The 206 kbp mitochondrial genome of Phanerochaete carnosa reveals dynamics of introns, accumulation of repeat sequences and plasmid-derived genes. Int. J. Biol. Macromol. 162, 209–219.
Warren, J. M., and Sloan, D. B. (2020). Interchangeable parts: the evolutionarily dynamic tRNA population in plant mitochondria. Mitochondrion 52, 144–156.
Zarivi, O., Cesare, P., Poma, A. M., Colafarina, S., Bonfigli, A., Kohler, A., et al. (2018). The genomic tool-kit of the truffle Tuber melanosporum programmed cell death. Cell Death Discov. 4:32.
Zascavage, R. R., Thorson, K., and Planz, J. V. (2019). Nanopore sequencing: an enrichment-free alternative to mitochondrial DNA sequencing. Electrophoresis 40, 272–280.
Zhang, N., Chen, H., Sun, B., Mao, X., Zhang, Y., and Zhou, Y. (2016). Comparative analysis of volatile composition in chinese truffles via GC x GC/HR-TOF/MS and electronic nose. Int. J. Mol. Sci. 17:412.
Zhang, X., Ye, L., Kang, Z., Zou, J., Zhang, X., and Li, X. (2019). Mycorrhization of Quercus acutissima with Chinese black truffle significantly altered the host physiology and root-associated microbiomes. PeerJ 7:e6421.
Zhao, N., Wang, Y., and Hua, J. (2018). The roles of mitochondrion in intergenomic gene transfer in plants: a source and a pool. Int. J. Mol. Sci. 19:547.
Keywords: Tuber, mitochondria, phylogenetic analysis, gene rearrangements, evolution 3
Citation: Li X, Li L, Bao Z, Tu W, He X, Zhang B, Ye L, Wang X and Li Q (2020) The 287,403 bp Mitochondrial Genome of Ectomycorrhizal Fungus Tuber calosporum Reveals Intron Expansion, tRNA Loss, and Gene Rearrangement. Front. Microbiol. 11:591453. doi: 10.3389/fmicb.2020.591453
Received: 04 August 2020; Accepted: 09 November 2020;
Published: 09 December 2020.
Edited by:
Raffaella Balestrini, Institute for Sustainable Plant Protection, Italian National Research Council, ItalyReviewed by:
Claudia Riccioni, National Research Council, Institute of Biosciences and Bioresources, ItalyIlias Kappas, Aristotle University of Thessaloniki, Greece
Copyright © 2020 Li, Li, Bao, Tu, He, Zhang, Ye, Wang and Li. This is an open-access article distributed under the terms of the Creative Commons Attribution License (CC BY). The use, distribution or reproduction in other forums is permitted, provided the original author(s) and the copyright owner(s) are credited and that the original publication in this journal is cited, in accordance with accepted academic practice. No use, distribution or reproduction is permitted which does not comply with these terms.
*Correspondence: Qiang Li, leeq110@126.com; Xu Wang, xuwang@henau.edu.cn
†Present address: Qiang Li, School of Food and Biological Engineering, Chengdu University, Chengdu, China