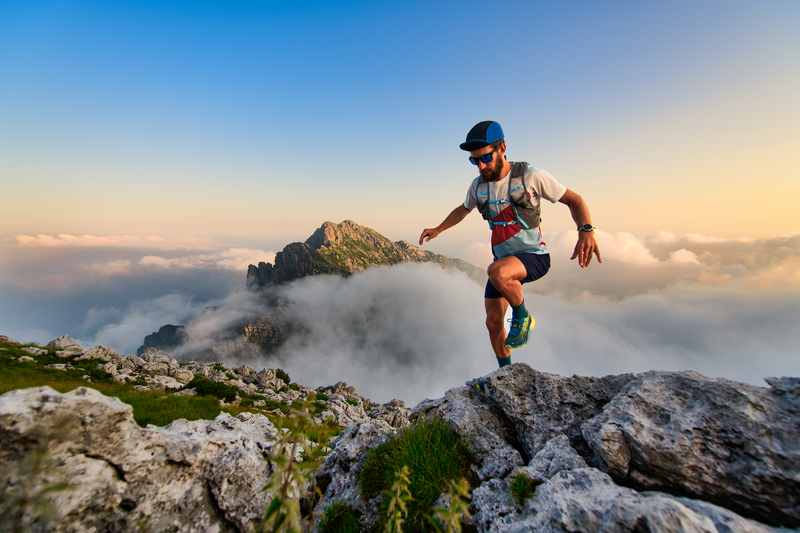
95% of researchers rate our articles as excellent or good
Learn more about the work of our research integrity team to safeguard the quality of each article we publish.
Find out more
ORIGINAL RESEARCH article
Front. Microbiol. , 13 January 2021
Sec. Microbial Symbioses
Volume 11 - 2020 | https://doi.org/10.3389/fmicb.2020.590111
This article is part of the Research Topic Diversity of Beetles and Associated Microorganisms View all 22 articles
Bark beetles (sensu lato) colonize woody tissues like phloem or xylem and are associated with a broad range of micro-organisms. Specific fungi in the ascomycete orders Hypocreales, Microascales and Ophistomatales as well as the basidiomycete Russulales have been found to be of high importance for successful tree colonization and reproduction in many species. While fungal mutualisms are facultative for most phloem-colonizing bark beetles (sensu stricto), xylem-colonizing ambrosia beetles are long known to obligatorily depend on mutualistic fungi for nutrition of adults and larvae. Recently, a defensive role of fungal mutualists for their ambrosia beetle hosts was revealed: Few tested mutualists outcompeted other beetle-antagonistic fungi by their ability to produce, detoxify and metabolize ethanol, which is naturally occurring in stressed and/or dying trees that many ambrosia beetle species preferentially colonize. Here, we aim to test (i) how widespread beneficial effects of ethanol are among the independently evolved lineages of ambrosia beetle fungal mutualists and (ii) whether it is also present in common fungal symbionts of two bark beetle species (Ips typographus, Dendroctonus ponderosae) and some general fungal antagonists of bark and ambrosia beetle species. The majority of mutualistic ambrosia beetle fungi tested benefited (or at least were not harmed) by the presence of ethanol in terms of growth parameters (e.g., biomass), whereas fungal antagonists were inhibited. This confirms the competitive advantage of nutritional mutualists in the beetle’s preferred, ethanol-containing host material. Even though most bark beetle fungi are found in the same phylogenetic lineages and ancestral to the ambrosia beetle (sensu stricto) fungi, most of them were highly negatively affected by ethanol and only a nutritional mutualist of Dendroctonus ponderosae benefited, however. This suggests that ethanol tolerance is a derived trait in nutritional fungal mutualists, particularly in ambrosia beetles that show cooperative farming of their fungi.
Filamentous fungi are generally known to be common symbionts of bark and ambrosia beetles (Curculionidae: Scolytinae and Platypodinae). While many species use beetles for dissemination with apparently little benefits for their vectors (Birkemoe et al., 2018; Seibold et al., 2019) other fungi are essential for the beetles like nutritional or tree-defenses-detoxifying mutualists as well as tree-defenses-stimulating fungi (e.g., Six, 2003; Lieutier et al., 2009; Kandasamy et al., 2019; Biedermann and Vega, 2020). The most famous nutritional mutualisms are those between xylem-colonizing ambrosia beetles and various “ambrosia fungus” species in the ascomycete orders Hypocreales, Microascales and Ophiostomatales as well as phloem-colonizing Dendroctonus bark beetles and their basidiomycete and ascomycete mutualists in the orders Russulales and Ophiostomatales (Barras and Perry, 1972; Six and Paine, 1998; Six and Klepzig, 2004; Harrington, 2005; Kirkendall et al., 2015; Biedermann and Vega, 2020). All these are truly agricultural mutualisms, because they evolve active care of the beetles for their fungal “crops” and involve more or less species-specific partnerships. Indeed, within the majority of the ambrosia beetle – fungus relationships, the beetles are essential for the survival of their mutualistic fungi as they would be overgrown by fungal competitors if the beetles are not present (Kirkendall et al., 2015; Nuotclà et al., 2019). Up to date, these mutualisms are unique for beetles and comparable to the advanced fungi culturing systems of attine ants and fungus-farming termites (Farrell et al., 2001; Six, 2003; Müller et al., 2005; Biedermann and Vega, 2020).
The nutritional mutualisms of ambrosia beetles and certain Dendroctonus spp. as well as some Ips sp. are known for quite a while (Batra, 1963; Francke-Grosmann, 1965, 1966, 1967; Six, 2003; Vissa and Hofstetter, 2017), but more recent research shows that other bark beetle species also depend on fungal associates mainly through detoxification of insect-repelling tree-chemistry (like in the case of fungal symbionts of the European spruce bark beetle Ips typographus; Kandasamy et al., 2016, 2019; Wadke et al., 2016; Zhao et al., 2019). While nutritionally important fungal mutualists are typically vertically transmitted within mycetangia (i.e., fungus spore carrying and selecting organs), these organs are rather rare in non-nutritional beetle-fungus associations (Francke-Grosmann, 1956, 1967; Batra, 1963; Six, 2003; Hulcr and Stelinski, 2017; Skelton et al., 2020; Biedermann and Vega, 2020). How mutualisms are maintained in the latter case is poorly known and supposedly involves vertical transmission through the gut or the surface of the exoskeleton of the insects (Six, 2003; Harrington, 2005). Even less understood is how the beetles maintain the dominance of certain fungi within their tunnel systems and how they suppress ubiquitous competitor fungi and beetle pathogens (henceforth termed antagonistic fungi).
So far, three evolutionary mechanisms are known by which a host (e.g., a bark beetle) can maintain a mutualism with a beneficial symbiont (e.g., an ambrosia fungus): (i) Partner choice, in which a host is actively selecting a specific symbiont, (ii) partner fidelity, where symbionts are vertically transmitted from one host generation to the next, and (iii) the theoretical and empirically hardly studied mechanism of competition-based screening, in which environmental filters created by the host select for the preferred symbiont (Archetti et al., 2011; Scheuring and Yu, 2012; Foster et al., 2017). Environmental screening of mutualistic ambrosia fungi utilizing ethanol within woody substrate (that most ambrosia beetles preferentially colonize) has been recently discovered by Ranger et al. (2018). These authors show that ethanol strengthens the competitive ability of mutualistic ambrosia fungi over other fungal antagonists, because ambrosia fungi are able to detoxify ethanol and use it as a carbon source, whereas the antagonists are strongly inhibited in their growth by even small amounts of ethanol, which is typically an antimicrobial compound (McGovern et al., 2004; Tunc et al., 2007). Moreover, mutualistic ambrosia beetle fungi are known to produce ethanol and other alcohols themselves (Kuhns et al., 2014; Kandasamy et al., 2016), giving them the possibility to enrich the colonized woody substrate with ethanol and thus maintain their dominance even after the production by the dying plant cells ceases (Kimmerer and Kozlowski, 1982). The ethanol production of ambrosia fungi can thus be compared with other similar defensive mechanisms used by other microorganisms to protect themselves and their animal hosts (Cardoza et al., 2006; Scott et al., 2008; Six, 2013; Flórez et al., 2015; Ranger et al., 2018).
Ethanol generally plays a crucial role for the attraction of ambrosia beetles (i.e., it is a kairomone) as it allows them to detect suitable hosts like stressed or recently dead trees (Graham, 1968; Kühnholz et al., 2001; Ranger et al., 2015). In fact, ethanol is not solely present in stressed trees but is commonly found also in healthy trees within both xylem and phloem (Kimmerer and Stringer, 1988; MacDonald and Kimmerer, 1991; Kozlowski, 1992; Kelsey et al., 2014). Its content is known to increase as soon as the tree is stressed (e.g., flood stress, mechanical damage) (Kimmerer and Kozlowski, 1982; MacDonald and Kimmerer, 1991; Ranger et al., 2013) and is thereby functioning as a cue for ambrosia beetles to recognize defense deficient trees. The phloem-colonizing bark beetles, which are the evolutionary ancestors of ambrosia beetles, still prefer similarly deficient host trees. On the contrary, however, they usually use tree volatiles and/or aggregation pheromones (produced de novo) other than ethanol to detect their preferred hosts (Vité et al., 1972; Wood, 1982; Kirkendall et al., 2015; Kandasamy et al., 2016; Biedermann et al., 2019). So currently it is unknown whether ethanol plays a role in the bark beetle system by preferentially fostering the growth of their fungal mutualists.
Ambrosia beetle fungi are not unique in their ability to produce ethanol. In fact, the ability of yeasts and filamentous fungi to produce ethanol is relatively widespread in certain fungal groups (e.g., plant-colonizing fungi like Fusarium and Rhizopus; the brewer’s yeast Saccharomyces cerevisiae; saprobic and soil-colonizing fungi like Aspergillus) (Schneider and Jeffries, 1989; Singh and Kumar, 1991; Singh et al., 1992; Wainwright et al., 1994; Skory et al., 1997; Ferreira et al., 2014) and has an important role in biotechnologically used fermentation processes. While many of these taxa are able to create their own defended niche by metabolizing, producing and accumulating ethanol in their environment, they are typically found free-living and not in mutualisms with insect hosts (Thomson et al., 2005; Dashko et al., 2014; Zhou et al., 2017). The only other insects known to be associated with an ethanol-producing fungus (i.e., yeast) are Drosophila species, which benefit, like the ambrosia beetles, from the defensive abilities of their symbionts (McKenzie and Parsons, 1972; Becher et al., 2012; Christiaens et al., 2014). Some fungal mutualists of bark beetles like Endoconidiophora polonica and Grosmannia penicillata (associated with Ips typographus), are as well known to produce several different alcohols (Kandasamy et al., 2019), but whether they metabolize, produce and use them to defend themselves like the ambrosia beetle mutualists remains unstudied.
Nutritional fungus mutualisms have evolved independently in at least eleven lineages of wood-boring weevils (Coleoptera: Curculionidae: Scolytinae and Platypodinae) with at least five lineages of ascomycetes (Ophiostomataceae, Nectriaceae, Bionectriaceae, Saccharomycetaceae, Ceratocystidaceae) as well as two lineages of basidiomycetes (Peniophoraceae, Meruliaceae) (Alamouti et al., 2009; Li et al., 2014; Hulcr et al., 2015; Bateman et al., 2017; Hulcr and Stelinski, 2017; Biedermann and Vega, 2020). The ancestral habit of these weevils is phloem and most lineages transitioned to colonize xylem after the origin of the nutritional mutualism (which made it possible to live on the nutrient-poor xylem), possibly because of the high competition within the phloem (Kirkendall et al., 2015; Biedermann and Vega, 2020). Currently, only a few ambrosia beetle fungal mutualists in the Ophiostomataceae and Ceratocystidaceae have been investigated for their affinity to ethanol (Ranger et al., 2018) and it remains unknown how widespread this trait is for the other lineages of ambrosia fungi as well as and in particular for the ancestral fungal symbionts of bark beetles.
Some bark and ambrosia beetles are severe pests, causing high economic damages in forests and plantations worldwide (Kühnholz et al., 2001; Hulcr and Dunn, 2011; La Spina et al., 2013; Ranger et al., 2015; Biedermann et al., 2019). Therefore, research on the physiology of their fungal mutualists is very important to understand the ecology of these beetle-fungus interactions, which may help at some point to develop new management tools that target the fungal mutualists. Here, we examined 10 fungal species from four convergently evolved clades of bark and ambrosia beetle associated fungal symbionts, including five nutritional and two tree-chemistry-detoxifying mutualists and one symbiont with unknown role as well as 2 ubiquitous antagonistic fungi for their ability to grow on ethanol-containing substrate. Two free-living, non-mutualistic filamentous fungi in the same lineages were included to test for phylogenetic effects of ethanol tolerance. Our aims were (i) to test the ethanol tolerance of previously not-tested clades of bark and ambrosia beetle fungal mutualists in the basidiomycete Russulales and the ascomycete Hypocreales and Ophiostomatales (see Figure 1) and (ii) to compare it between bark beetle (sensu stricto) and ambrosia beetle fungal partners. The latter can help to understand the evolution of ethanol tolerance because ambrosia beetle fungal mutualists are descendants of bark beetle fungal mutualists, which have not been investigated for their tolerance toward ethanol so far. Finally, we aim to test (iii) how widespread the ethanol sensitivity is in antagonists of bark and ambrosia beetles and free-living fungi in the same clades of known beetle mutualists.
Figure 1. Phylogenetic placement based on 49 fungal LSU sequences. Black-colored species were examined within this study (N = 12), while species in gray act as a fungal outgroup (N = 37). The LSU region of the following fungi was sequenced by us and used for the phylogenetic analysis (strain IDs in brackets): Ophiostoma bicolor (P22), Grosmannia penicillata (2), Endoconidiophora polonica (F5), Beauveria bassiana (P13), Entomocorticium sp. (P8), Entomocorticium dendroctoni (P163), and Fusarium euwallaceae (P170). Sequences from all other species were obtained from NCBI GenBank. For accession numbers and further information of each individual strain see Supplementary Tables 1, 2. Ophiostomatales were divided into their four sub-clades that are currently hypothesized to have evolved independently as nutritional mutualists of ambrosia beetles (Biedermann and Vega, 2020). All examined species were classified into (i) ambrosia beetle fungi, (ii) bark beetle fungi, and (iii) free-living fungi. Triangles denote significant effects on fungal biomass (decrease, increase or not significant; p < 0.05) between the 0 and 1% EtOH treatments. Nutritional mutualists of bark and ambrosia beetles are underlined. Due to its abundant sporulation we could not receive comparable data from Beauveria bassiana (indicated with *) and excluded it from all further analyses.
In this study, we used common fungal mutualists/symbionts of widespread bark and ambrosia beetles from the three ascomycete orders [Microascales (Ceratocystidaceae), Ophiostomatales (Ophiostomataceae), Hypocreales (Nectriaceae)] and one basidiomycete order [Russulales (Peniophoraceae)]. Additionally, we used two common fungal antagonists of bark and ambrosia beetles in the two ascomycete orders Hypocreales (Cordycipitaceae) and Sordariales (Chaetomiaceae). Finally, we included the nematophagous fungus Esteya vermicola in the Ophiostomatales (Ophiostomataceae) as well as the basidiomycete Entomocorticium sp. (see Lehenberger et al., 2018) in the Russulales (Peniophoraceae), which served as non-beetle-associated, free-living, phylogenetic controls (for an overview of all strains see Table 1 and Supplementary Table S1). We included all fungal species within a phylogenetic replacement that aimed to (i) visualize the independently evolved phylogenetic lineages of all tested species and to (ii) indicate each classification as well as (iii) our current findings.
Table 1. Overview of the twelve fungal isolates used in this study and additional information on their classification, beetle host(s), association, phylogenetic order and literature.
A stock collection of all these fungal isolates in glycerol (80%) and glycerol/peptone (80%/1%; Roth, Germany) is constantly maintained at −80°C in our lab in Freiburg, Germany. Origins of the fungal isolates are given in Supplementary Table 1. At the beginning of the experiment, we first revived fungi on malt extract agar plates (MEA: 3% malt extract, 0.5% soy peptone, 2% agar, pH = 5.5–6; Sigma-Aldrich, Germany) and stored them at 5°C until they were sub-cultured once more on MEA (25°C, 60% RH) for 5 (fast growers) to 14 (slow growers) days (Supplementary Table 3) until the experiment was started for each fungus individually.
We first homogenized fungal samples (pure biomass) by grinding in liquid nitrogen and then proceeded with DNA extraction using the NORGEN Biotek Corp. fungi/yeast genomic DNA isolation kit following the manufacturer’s protocol. Sequences of the large subunit (LSU) of the ribosomal RNA gene were primarily used for the identification and phylogenetic placement of isolates (Figure 1; Supplementary Table 1). For LSU amplification, we used the common primers LROR-F (GTACCCGCTGAACTTAAGC) and LR5-R (ATCCTGAGGGAAACTTCG) (Vilgalys and Hester, 1990; Rehner and Samuels, 1994), which amplify a region of approximately 830 to 880 bp.
Because some samples repeatedly failed to amplify using the LSU primers, we barcoded them using the internal transcribed spacer (ITS) of the ribosomal RNA or the beta-tubulin gene (βT). The ITS region was amplified using primers ITS1-F (TCCGTAGGTGAACCTGCGG) and ITS4-R (TCCTCCGCTTATTGATATGC) (White et al., 1990) and with an annealing temperature of 54.5°C. The ITS region finally contained approximately 580 bp. For amplification of βT, we used the primers T10-F (ACGATAGGTTCACCTCCAGAC) and Bt2b-R (ACCCTCAGTGTAGTGACCCTTGGC) (Glass and Donaldson, 1995; O’Donnell and Cigelnik, 1997) and 57.5°C as annealing temperature. Sequence lengths contained around 300 to 400 bp.
For all PCR reactions, we used a similar master-mix for LSU, βT and ITS barcoding [Master-mix for 50 μl: 25 μl 2× phusion high-fidelity PCR master mix with GC buffer (Thermo ScientificTM, Germany), 2.5 μl forward primer 1 (10 μM, Eurofins Genomics, Germany) 2.5 μl reverse primer 2 (10 μM, Eurofins Genomics, Germany), 18 μl ddH20, 2 μl template (usually 1:10 diluted)]. For all reactions we applied the following PCR conditions: 98°C for 30 s, followed by 35 cycles at 98°C for 10 s, 55.5°C, 54.5°C or 57.5°C (for LSU, ITS, and βT, respectively) for 30 s and 72°C for 20 s, ending with 72°C for 10 min and ending with a storage temperature of 5°C.
DNA-purification was done using the Wizard® SV gel and PCR clean-up system (Promega, Germany) after performing gel electrophoresis. Sanger-sequencing was performed by Eurofins (Eurofins Genomics, Germany). To verify each sequence quality, we used the “SnapGene® Viewer” 3.2 (SnapGene software, from GSL Biotech; available at snapgene.com) and manually corrected automatically deduced sequences, if necessary. Fungal species were finally identified using BLASTn at NCBI (Altschul et al., 1990). All obtained fungal sequences were uploaded to the NCBI GenBank database (the accession numbers are included in Supplementary Table 1).
Sequences of the LSU for seven of our fungal sequences were used for maximum likelihood analyses and construction of a phylogenetic tree (Supplementary Table 1). NCBI GenBank entries were used for the remaining five sequences of studied fungi as well as for 37 fungal outgroup sequences (Supplementary Table 2). Individual sequences were prepared using GeneDoc 2.7 (Nicholas, 1997) or MEGA 7.0 (Kumar et al., 2016) and were additionally compared with the received output from Clustal Omega (Madeira et al., 2019). The phylogenetic analyses were done with the IQ-Tree-Web-Server (Nguyen et al., 2015; Trifinopoulos et al., 2016) using the ultrafast bootstrap analysis (Minh et al., 2013). Based on the web server FindModel (Posada and Crandall, 1998, available at1), we chose the generalized time reversible (GTR) model plus gamma (+ G) with rate heterogeneity (rate categories – 4) and combined it with 8.000 bootstrap alignments as our substitution model. FigTree2 was used for visualization of the phylogenetic tree.
The phylogenetic relationship of tested fungi is already known (see Rollins et al., 2001; Alamouti et al., 2009; Dreaden et al., 2014; Vanderpool et al., 2018), therefore we did not include it within the results. The aim of the phylogenetic reconstruction was to give an overview over the different examined clades with our choice of fungi as well as to visually summarize our findings in an appealing way (see Figure 1).
We examined the influence of five ethanol (EtOH) concentrations in MEA culturing media (0, 1, 2, 3, 5 vol/vol) on biomass, area and density of 12 fungal strains. The methods closely followed those reported in Ranger et al. (2018). Ethanol (99.8%, Sigma-Aldrich, Germany) was added to the MEA media at around 55°C (just before it solidified) to reduce its evaporation. Immediately after the media had cooled down in the petri dishes, we added a sterile cellophane membrane (6 × 6 cm, NeoLab, Germany) and a plug of the pre-cultured fungal mycelium (Ø 3 mm, using a cork borer) to the center of each plate. We used eight replicates per fungus for each of the five EtOH treatments, for a total of 40 plates per fungus, which were sealed with parafilm and subsequently incubated in dark at 25°C and 60% RH. Images of the colonies were taken every second day (Sony alpha 5000, 12 × in macro mode) through the closed lid of the petri dish. Only at the last day of the experiment the lid was removed before taking the picture (see Figure 2 and Supplementary Figure 1). All images were analyzed for surface area using ImageJ software (version 1.52a). The experiment was terminated for each fungal species separately and as soon as one replicate (independent of the EtOH treatment) reached an edge of the cellophane membrane. For individual incubation times see Supplementary Table 3. If single replicates were contaminated, we excluded them from our analyses (Supplementary Table 3). After termination of the experiment and taking of the picture, the entire fungal biomass was collected from each cellophane membrane, dried individually at 50°C for up to 14 days (dry biomass) in a drying oven and was then weighed. To calculate the density (mg/mm2) of fungal mycelia, the determined dry weight (mg) was divided by measured surface area (mm2).
Figure 2. Representative images of seven studied fungi growing on MEA treated with five investigated ethanol concentrations (0, 1, 2, 3, and 5% vol/vol). (a): ambrosia beetle fungi = Ambrosiella hartigii, Raffaelea sulphurea; (b): bark beetle fungi = Grosmannia penicillata, Endoconidiophora polonica; (c): pathogens/free-living = Chaetomium globosum, Esteya vermicola, Entomocorticium sp. For incubation times and further information of each individual strain see Supplementary Table 3. The five remaining fungal species are displayed in Supplemantary Figure 1.
Effects of EtOH concentration on dry biomass, surface area and density for each fungal species were visualized with ggplot in R (version 1.2.5033) using the package ggplot2 (Wickham, 2016). One-way-ANOVAs (log10 transformed data; Biomass in dependence of ethanol concentration) were conducted to detect differences in the biomass between the 0 and 1%, and the 0 and 2% EtOH treatments for each fungus using the package rcompanion (CRAN.R-project.org/package = rcompanion). We added the received ANOVA output for each examined fungus to the Anova Output.
Ethanol enriched substrate had a strong effect on the majority of examined fungal species. Three different reactions of tested fungi toward EtOH could be distinguished: (I) Fungal biomass was positively affected (Figure 3A) (II) not affected (Figure 3A) or (III) negatively affected (Figure 3B) by medium containing 1% ethanol in comparison to the absence of ethanol. Fungal species were only associated with pattern I or III, if a significant difference between the 0 and 1% EtOH treatment could be detected. In case we did not detect any significances, fungi were generally associated with pattern II, even though some species showed a slight non-significant increase or decrease of biomass.
Figure 3. Fungal biomass in mg (dry-weight) as a function of the EtOH (% v/v) treatment (0, 1, 2, 3, and 5% vol/vol). Fungi were classified into (A) positively affected (Ambrosiella hartigii, Entomocorticium dendroctoni, Esteya vermicola) and not affected (Raffaelea canadensis, R. sulphurea Fusarium euwallaceae) by the 1% EtOH treatment or (B) negatively affected (Chaetomium globosum, Endoconidiophora polonica, Entomocorticium sp. (E.sp.), Grosmannia penicillata, Ophiostoma bicolor) by the 1% EtOH treatment. With one exception (E. vermicola) all species in the first group are nutritional mutualists of bark and ambrosia beetles, whereas all species in the second group are either plant-defenses-detoxifying mutualists of bark beetles, free-living or antagonistic species. (AB) denotes ambrosia beetle associates and (BB) bark beetle associates. Means and standard deviations are given for each EtOH treatment. Significant differences between the 0% and 1% and 2% EtOH treatments are given: *p < 0.05, **p < 0.01, ***p < 0.001. Additionally, we added (+) and (–) to indicate a significant increase, respectively, decrease of fungal biomass. N = 8 replicates per fungal species.
Within the Microascales, we observed significantly more fungal biomass at 1% EtOH (pattern I) for the nutritional mutualist of the ambrosia beetle Anisandrus dispar, Ambrosiella hartigii (p = 0.0008, Figure 2a) while the detoxifying mutualist of the bark beetle Ips typographus, Endoconidiophora polonica (p = 2.23e-10, Figure 2b) produced significantly fewer biomass and thus showed pattern III. In the Opiostomatales, the non-beetle-associated, endophyte Esteya vermicola (p = 0.008, Figure 2c) clearly showed pattern I while there was no significant increase in biomass but a general tolerance toward ethanol (pattern II) for the two nutritional mutualists of the ambrosia beetle Xyleborinus saxesenii, Raffaelea sulphurea (p = 0.65, Figure 2a) and R. canadensis (p = 0.35, Supplementary Figure 1B), (mean biomass for both was higher at 1% EtOH, but not statistically significant). The tree-defenses-detoxifying mutualist of I. typographus, Grosmannia penicillata (p = 0.154, Figure 2b) showed a tolerance toward the 1% EtOH treatment, even though mean biomass decreased with increasing EtOH concentrations (no significance at 1% EtOH (pattern II), but rather similar to pattern III). Another symbiont with unknown role in I. typographus, Ophiostoma bicolor (p = 4.01e-11, Supplementary Figure 1C), was highly negatively affected by EtOH (pattern III). The only Sordariales, the antagonistic bark and ambrosia beetle symbiont Chaetomium globosum (p = 1.83e-06, Figure 2c) produced significantly fewer biomass (pattern III). Within the Russulales, the supposedly free-living, endophytic Entomocorticium sp. (isolated from Trypodendron lineatum) shows pattern III (p = 1.73e-06, Figure 2c), while the nutritional mutualist of the bark beetle Dendroctonus ponderosae, Entomocorticium dendroctoni shows pattern I (p = 0.00003, Supplementary Figure 1C). In the Hypocreales, the only fungal species which was tolerating EtOH even up to 2% (pattern II) is the nutritional mutualist of the ambrosia beetle Euwallacea fornicatus, Fusarium euwallaceae (p = 0.82, Supplementary Figure 1B). Within the same group, the generally entomopathogenic fungus Beauveria bassiana (Supplementary Figure 1A) showed an enormous standard deviation due to its abundant sporulation, which made it impossible to compare the biomass of this species at different ethanol concentrations as spores easily spread all over the plate. Its growth patterns should be treated with care and are thus only presented in Supplementary Table 3 and Supplementary Figure 1A. All examined fungi produced less biomass above 2 to 3% EtOH compared to the 0% EtOH treatment. This effect was particularly present in the bark beetle symbionts (E. polonica, O. bicolor, G. penicillata, E. dendroctoni), the antagonist C. globosum and the endophyte Entomocorticium sp., while the remaining ambrosia beetle mutualists were less strongly affected. The strongest effect could be observed at the 5% EtOH media, on which only ambrosia beetle mutualists as well as the free-living endophyte E. vermicola produced substantial amounts of biomass (see Figure 2, Supplementary Figure 1 and Supplementary Table 3). Results from fungal surface area as well as the calculated density in relation to the amount of EtOH in the media can be found in the Supplementary Results.
Our findings showed that the examined obligate and nutritional ambrosia beetle mutualists (A. hartigii, R. canadensis, R. sulphurea) as well as the obligate and nutritional bark beetle mutualist (E. dendroctoni) benefit or at least are not harmed (F. euwallaceae) by the presence of low (1–2%) concentrations of ethanol. Most fascinating, this tolerance of ethanol by mutualistic fungi is apparently not restricted to a specific lineage of ambrosia beetle fungi (Figure 1), but must have evolved convergently in different orders of fungi, such as the ascomycete Microascales, Ophiostomatales and Hypocreales as well as the basidiomycete Russulales. Interestingly, the majority of non-nutritional, plant-defenses-detoxifying bark beetle fungi (E. polonica, G. penicillata, O. bicolor) and the fungal antagonist of bark and ambrosia beetles C. globosum strongly decrease in biomass in the presence of ethanol. Thus, we confirm and further expand the findings of Ranger et al. (2018) (that were based on just a handful of fungi) to some more lineages of independently evolved bark and ambrosia beetle symbionts with beneficial to antagonistic roles. Our findings show that non-nutritional mutualists of bark beetles (which have never been investigated for their ethanol tolerance) are sensitive to even small amounts of ethanol. This is fascinating because at least in the Ophiostomatales and Microascales these fungi are ancestral to ambrosia beetle mutualists, suggesting that ethanol tolerance convergently evolved repeatedly along with the xylem-boring and fungus-farming habit of ambrosia beetles. Interestingly, all symbionts of bark and ambrosia beetles can tolerate or benefit by the presence of 1–2% ethanol if they are nutritionally beneficial to their obligately dependent beetle host. This is particularly interesting because we have pairs of ethanol benefiting/tolerant vs. sensitive fungal species in almost every fungal order that we tested. It indicates that ethanol tolerance is a derived trait in bark and ambrosia beetle nutritional mutualists that are transmitted in mycetangia and actively farmed by their hosts. Furthermore, the ethanol preference means that these fungi (as well as their hosts) are adapted to colonize stressed or recently dead trees (Moeck, 1970; Miller and Rabaglia, 2009; Ranger et al., 2011, 2013, 2015, 2018; Reding et al., 2011).
While the group of bark and ambrosia beetles are best known by their tree-killing species, the majority of species actually colonizes highly stressed or recently dead host trees (Batra, 1963; Beaver et al., 1989; Kühnholz et al., 2001; Hulcr and Dunn, 2011; Kirkendall et al., 2015; Ranger et al., 2015). On the tree, they differ in the localization of their tunnels – xylem for ambrosia and phloem for bark beetles – and their association with fungi that are typically nutritionally important for ambrosia beetles and detoxify (or induce) tree defenses for bark beetles (but see exceptions in some Dendroctonus and Ips spp.) (Batra, 1963, 1967; Francke-Grosmann, 1963, 1966; Whitney et al., 1987; Kirkendall et al., 2015; Hulcr and Stelinski, 2017; Vissa and Hofstetter, 2017; Kandasamy et al., 2019). As we discussed above, ambrosia beetles are generally associated with ethanol tolerant or benefiting fungi, whereas bark beetle fungi are typically sensitive to ethanol. This is also reflected by the attraction to ethanol during tree-host finding only of ambrosia beetles, but not of bark beetles. Studies show that ethanol can be found in both xylem and phloem tissue (Kimmerer and Stringer, 1988; MacDonald and Kimmerer, 1991; Kelsey et al., 2014) and ethanol is even enriched in the plant tissues during bark beetle attacks (Kelsey et al., 2014). However, it is unclear if phloem of dying trees is generally lower in ethanol (e.g., due to evaporation) or if bark and ambrosia beetles differ in host substrate preferences. Also, it is possible that both bark and ambrosia beetles have to be exposed to ethanol in their breeding substrate, but that bark beetle symbionts (e.g., bacteria) are capable of detoxifying the phloem. A variety of yeasts, bacteria and filamentous fungi have been isolated from bark beetles (Six, 2013) and many of them have detoxifying capabilities (Dowd, 1989, 1992; Skrodenyte-Arbaciauskiene et al., 2006; Hammerbacher et al., 2013; Um et al., 2013; Ramadhar et al., 2014; Wadke et al., 2016; Zhao et al., 2019).
All fungi showing a tolerance (R. sulphurea, R. canadensis, F. euwallacea, G. penicillata) or even an increase in biomass at 1% ethanol (E. vermicola, A. hartigii, E. dendroctoni, Figure 3A) might indicate an elevated activity of alcohol dehydrogenases (ADHs; referring to the ADH experiment in Ranger et al., 2018). These enzymes allow organisms to detoxify ethanol and even consume it as a carbon source, which can lead to an increase in biomass in the presence of EtOH, as we found in some species (significant increase: E. vermicola, A. hartigii, E. dendroctoni; not significant, but higher mean biomass: R. canadensis, R. sulphurea, F. euwallaceae). The presence and high activity of ADHs has already been supported by Ranger et al. (2018) for the ambrosia beetle fungal mutualists Ambrosiella grosmanniae and Raffaelea canadensis, while ADHs were only poorly present in Ambrosiella roeperi and not detectable within the yeast-like fungus Ascoidea sp. and the fungal antagonist Aspergillus sp. Ranger et al. (2018) found a significant increase in biomass at 1% ethanol for R. canadensis that we did not find.
Our findings regarding the sensitivity of plant-defenses-detoxifying mutualists of bark beetles toward ethanol (pattern III) in contrast to the insensitivity of nutritional mutualists of bark and ambrosia beetles (pattern I and II) should be interpreted with care, however. Here, we tested only a small fraction of fungi, with only one nutritional mutualist of a bark beetle and no plant-defenses-detoxifying mutualists of ambrosia beetles. Therefore, it is currently unclear whether the ethanol sensitivity that is certainly more common in bark beetle symbionts is related to the phloem habitat or the beneficial physiology of the fungi to the beetles.
In the Hypocreales, the fourth lineage of ambrosia beetle fungal mutualists, F. euwallaceae, was neither facilitated nor harmed by EtOH up to 2% relative to the control (Figure 3A). This indicates that this fungus can tolerate EtOH, which is already known for the genus Fusarium; for example, F. oxysporum is known to produce ethanol (Ueng and Gong, 1982; Christakopoulos et al., 1989). This might indicate that the insensitivity toward ethanol had been present at the origin of the ambrosia beetle-fungus mutualism in the Hypocreales and did not evolve anew as hypothesized for the other lineages (see above). The endophytic ascomycete E. vermicola (Ophiostomatales) (Figure 3A) can also metabolize ethanol, similar to ambrosia beetle fungal mutualists. This is especially interesting as this fungus is not associated with any beetle (Liou et al., 1999), but is phylogenetically closely related to the ambrosia beetle fungal mutualist R. sulphurea (Figure 1). This could mean that ethanol tolerance is present in some free-living fungi, or even that E. vermicola has been previously associated with beetles. Close examination of the phylogeny of the relatives of E. vermicola and R. sulphurea and their EtOH sensitivities have to be conducted to answer this question.
Quite unique for filamentous fungal species is the high tolerance (reflected by the ability to produce some biomass) of some ambrosia beetle mutualists (A. hartigii, R. canadensis, and F. euwallaceae) as well as some free-living fungi (E. vermicola and Entomocorticium sp.) toward even the highest tested EtOH concentration (5%). (Figures 2a,c and Supplementary Figure 1B). This tolerance is close to the naturally occurring, wild-type strains of yeasts (up to 6% ethanol by certain species in the genus Saccharomyces, Candida, Fabospora, Kluyveromyces, Kloeckera) and of specific filamentous fungi (3–7% by certain species in the genus Rhizopus and Fusarium) used for producing ethanol and above the typical limits of alcohol tolerance in other fungal species (Gao and Fleet, 1988; Singh and Kumar, 1991; Singh et al., 1992; Skory et al., 1997; Banat et al., 1998; Pina et al., 2004; Benjaphokee et al., 2012; Ferreira et al., 2014; Lam et al., 2014; Paschos et al., 2015; Ruchala et al., 2020). In biotechnology, ethanol tolerance and production of fungi can be increased by culture dependent methods (e.g., pH, temperature, medium, carbon-source), genetic modifications and artificial selection (e.g., Gao and Fleet, 1988; Skory et al., 1997; Pina et al., 2004; Benjaphokee et al., 2012; Lam et al., 2014; Ruchala et al., 2020). The extraordinary tolerance of some of our tested fungi in combination with the known ethanol production of ambrosia beetle fungi makes them quite interesting for biotechnological purposes (i.e., second-generation biofuels made from plant biomass). Moreover, as we show here, their tolerance toward ethanol evolved apparently several times independently in unrelated fungal lineages, so it might be fruitful for biotechnological purposes to investigate whether the tolerance is always enabled by the same physiological mechanisms.
Here, we show that not only specific ambrosia beetles, but many ambrosia beetle species and even a Dendroctonus bark beetle rely on fungal partners that detoxify and metabolize ethanol. This competitive ability of their mutualistic partners may allow the beetles to indirectly select their partners by biological screening through the ethanol-containing substrate they choose for boring their galleries (Scheuring and Yu, 2012; Ranger et al., 2018). By contrast, most non-nutritional bark beetle fungal mutualists lack the ability to tolerate ethanol. Further studies are necessary to test whether ethanol is generally absent in most of the phloem colonized by bark beetles or is degraded by other symbionts in the microbiome of the beetles.
All obtained fungal sequences were uploaded to the NCBI GenBank database (the accession numbers are included in Supplementary Table 1).
ML and PB designed the study and wrote the manuscript. ML isolated and sequenced all examined fungi, analyzed data, did statistics, plotted results, and constructed the phylogenetic tree. MB and ML did the ethanol-based culturing. All authors contributed to the article and approved the submitted version.
This project was founded by the German Research Foundation (DFG) (Emmy Noether grant number BI 1956/1-1 to PB) and was supported by the Open Access Publication Fund of the University of Würzburg.
The authors declare that the research was conducted in the absence of any commercial or financial relationships that could be construed as a potential conflict of interest.
We thank Dr. Richard Hofstetter (Northern Arizona University, NAU, School of Forestry, Arizona, United States: Entomocorticium dendroctoni), Dr. Dineshkumar Kandasamy (Max Planck Institute for Chemical Ecology, Department of Biochemistry, Plant-Pathogen Interactions, Jena, Germany: Ophiostoma bicolor, Grosmannia penicillata, Endoconidiophora polonica) and Dr. Zvi Mendel (Agricultural Research Organization ARO, The Volcani Center, Department of Entomology, Nematology and Chemistry, Bet Dagan, Israel: Fusarium euwallaceae) for sharing their fungal isolates with us.
The Supplementary Material for this article can be found online at: https://www.frontiersin.org/articles/10.3389/fmicb.2020.590111/full#supplementary-material
Supplementary Figure 1 | Representative images of five studied fungi growing on MEA treated with five investigated ethanol concentrations (0, 1, 2, 3, and 5% vol/vol). (A): entomopathogens = Beauveria bassiana; (B): ambrosia beetle fungi = Raffaelea canadensis, Fusarium euwallaceae; (C): bark beetle fungi = Entomocorticium dendroctoni, Ophiostoma bicolor. For incubation times and further information on each individual strain see Supplementary Table 3. The seven other fungal species are displayed in Figure 1.
Supplementary Figure 2 | (A) = Surface area in mm2 and (B) = density in mg/mm2 based on EtOH treatment (0, 1, 2, 3, 5 vol/vol) of fungi positively affected (Ambrosiella hartigii, Entomocorticium dendroctoni, Esteya vermicola) and not affected (Raffaelea canadensis, R. sulphurea, Fusarium euwallaceae) in dry weight (see Figure 3) by the 1% compared to the 0% EtOH treatment. Means and standard deviations are given. N = 8 replicates per fungal species.
Supplementary Figure 3 | (A) = Surface area in mm2 and (B) = density in mg/mm2 based on the EtOH treatments (0, 1, 2, 3, 5%) of fungi negatively affected (Chaetomium globosum, Endoconidiophora polonica, Entomocorticium sp. (E.sp.), Grosmannia penicillata, Ophiostoma bicolor) in dry weight (see Figure 3) by the 1% compared to the 0% EtOH treatment. Means and standard deviations are given. N = 8 replicates per fungal species.
Alamouti, S. M., Tsui, C. K. M., and Breuil, C. (2009). Multigene phylogeny of filamentous ambrosia fungi associated with ambrosia and bark beetles. Mycol. Res. 113, 822–835. doi: 10.1016/j.mycres.2009.03.003
Altschul, S. F., Gish, W., Miller, W., Myers, E. W., and Lipman, D. (1990). Basic local alignment search tool. J. Mol. Biol. 215, 403–410.
Archetti, M., Scheuring, I., Hoffman, M., Frederickson, M. E., Pierce, N. E., and Yu, D. W. (2011). Economic game theory for mutualism and cooperation. Ecol. Lett. 14, 1300–1312. doi: 10.1111/j.1461-0248.2011.01697.x
Banat, I. M., Nigam, P., Singh, D., Marchant, and McHale, A. P. (1998). Ethanol production at elevated temperatures and alcohol concentrations: Part I–yeasts in general. World J. Microbiol. Biotechnol. 14, 809–821.
Barras, S., and Perry, T. (1972). Fungal symbionts in the prothoracic mycangium of Dendroctonus frontalis (Coleoptera: Scolytidae). Zeitschrift für Angewandte Entomol. 71, 95–104. doi: 10.1111/j.1439-0418.1972.tb01724.x
Bateman, C., Huang, Y. T., Simmons, D. R., Kasson, M. T., Stanley, E. L., and Hulcr, J. (2017). Ambrosia beetle Premnobius cavipennis (Scolytinae: Ipini) carries highly divergent ascomycotan ambrosia fungus, Afroraffaelea ambrosiae gen. nov. et sp. nov.(Ophiostomatales). Fungal Ecol. 25, 41–49. doi: 10.1016/j.funeco.2016.10.008
Batra, L. R. (1963). Ecology of ambrosia fungi and their dissemination by beetles. Trans. Kansas Acad. Sci. 66, 213–236. doi: 10.2307/3626562
Batra, L. R. (1967). Ambrosia fungi: a taxonomic revision, and nutritional studies of some species. Mycologia 59, 976–1017. doi: 10.1080/00275514.1967.12018485
Beaver, R. A., Wilding, N., Collins, N., Hammond, P., and Webber, J. (1989). “Insect-fungus relationships in the bark and ambrosia beetles,” in Proceedings of the Insect-fungus interactions. 14th Symposium of the Royal Entomological Society of London in collaboration with the British Mycological Society, (London: Academic Press), 121–143. doi: 10.1016/b978-0-12-751800-8.50011-2
Becher, P. G., Flick, G., Rozpedowska, E., Schmidt, A., Hagman, A., Lebreton, S., et al. (2012). Yeast, not fruit volatiles mediate Drosophila melanogaster attraction, oviposition and development. Funct. Ecol. 26, 822–828. doi: 10.1111/j.1365-2435.2012.02006.x
Benjaphokee, S., Hasegawa, D., Yokota, D., Asvarak, T., Auesukaree, C., Sugiyama, M., et al. (2012). Highly efficient bioethanol production by a Saccharomyces cerevisiae strain with multiple stress tolerance to high temperature, acid and ethanol. New Biotechnol. 29, 379–386. doi: 10.1016/j.nbt.2011.07.002
Biedermann, P. H., and Vega, F. E. (2020). Ecology and evolution of insect–fungus mutualisms. Annu. Rev. Entomol. 65, 431–455. doi: 10.1146/annurev-ento-011019-024910
Biedermann, P. H. W., Müller, J., Grégoire, J. C., Gruppe, A., Hagge, J., Hammerbacher, A., et al. (2019). Bark beetle population dynamics in the anthropocene: challenges and solutions. Trends Ecol. Evol. 34, 914–924. doi: 10.1016/j.tree.2019.06.002
Birkemoe, T., Jacobsen, R. M., Sverdrup-Thygeson, A., and Biedermann, P. H. W. (2018). “Insect-fungus interactions in dead wood systems,” in Saproxylic Insects, ed. M. D. Ulyshen (Berlin: Springer), 377–427. doi: 10.1007/978-3-319-75937-1_12
Cardoza, Y. J., Klepzig, K. D., and Raffa, K. F. (2006). Bacteria in oral secretions of an endophytic insect inhibit antagonistic fungi. Ecol. Entomol. 31, 636–645. doi: 10.1111/j.1365-2311.2006.00829.x
Christakopoulos, P., Macris, B., and Kekos, D. (1989). Direct fermentation of cellulose to ethanol by Fusarium oxysporum. Enzyme Microbial Technol. 11, 236–239. doi: 10.1016/0141-0229(89)90098-7
Christiaens, J. F., Franco, L. M., Cools, T. L., De Meester, L., Michiels, J., Wenseleers, T., et al. (2014). The fungal aroma gene atf1 promotes dispersal of yeast cells through insect vectors. Cell Reports 9, 425–432. doi: 10.1016/j.celrep.2014.09.009
Dashko, S., Zhou, N., Compagno, C., and Piškur, J. (2014). Why, when, and how did yeast evolve alcoholic fermentation? FEMS Yeast Res. 14, 826–832. doi: 10.1111/1567-1364.12161
Davidson, R. W. (1955). Wood-staining fungi associated with bark beetles in engelmann spruce in colorado. Mycologia 47, 58–67. doi: 10.1080/00275514.1955.12024429
De Beer, Z. W., Duong, T., Barnes, I., Wingfield, B. D., and Wingfield, M. J. (2014). Redefining ceratocystis and allied genera. Stud. Mycol. 79, 187–219. doi: 10.1016/j.simyco.2014.10.001
Dowd, P. F. (1989). In situ production of hydrolytic detoxifying enzymes by symbiotic yeasts in the cigarette beetle (Coleoptera: Anobiidae). J. Econ. Entomol. 82, 396–400. doi: 10.1093/jee/82.2.396
Dowd, P. F. (1992). Insect fungal symbionts–a promising source of detoxifying enzymes. J. Ind. Microbiol. 9, 149–161. doi: 10.1007/bf01569619
Dreaden, T. J., Davis, J. M., De Beer, Z. W., Ploetz, R. C., Soltis, P. S., Wingfield, M. J., et al. (2014). Phylogeny of ambrosia beetle symbionts in the genus Raffaelea. Fungal Biol. 118, 970–978. doi: 10.1016/j.funbio.2014.09.001
Farrell, B. D., Sequeira, A. S., O’Meara, B. C., Normark, B. B., Chung, J. H., and Jordal, B. H. (2001). The evolution of agriculture in beetles (Curculionidae: Scolytinae and Platypodinae). Evolution 55, 2011–2027. doi: 10.1111/j.0014-3820.2001.tb01318.x
Ferreira, J. A., Lennartsson, P. R., and Taherzadeh, M. J. (2014). Production of ethanol and biomass from thin stillage using food-grade zygomycetes and ascomycetes filamentous fungi. Energies 7, 3872–3885. doi: 10.3390/en7063872
Flórez, L. V., Biedermann, P. H. W., Engl, T., and Kaltenpoth, M. (2015). Defensive symbioses of animals with prokaryotic and eukaryotic microorganisms. Natural Product Reports 32, 904–936. doi: 10.1039/c5np00010f
Foster, K. R., Schluter, J., Coyte, K. Z., and Rakoff-Nahoum, S. (2017). The evolution of the host microbiome as an ecosystem on a leash. Nature 548, 43–51. doi: 10.1038/nature23292
Francke-Grosmann, H. (1956). Hautdrüsen als träger der pilzsymbiose bei ambrosiakäfern. Zeitschrift für Morphologie und Ökologie der Tiere 45, 275–308. doi: 10.1007/bf00430256
Francke-Grosmann, H. (1963). Die Übertragung der Pilzflora bei dem Borkenkäfer Ips acuminatus. Z. Angew. Entomol. 52, 355–361. doi: 10.1111/j.1439-0418.1963.tb02046.x
Francke-Grosmann, H. (1965). Ein Symbioseorgan bei dem Borkenkäfer Dendroctonus frontalis Zimm. (Coleoptera Scolytidae). Naturwis-senschaften 52, 143. doi: 10.1007/bf00638532
Francke-Grosmann, H. (1966). Über Symbiosen von xylo-mycetophagen und phloeophagen Scolytoidea mit holzbewohnenden Pilzen. Material und Organismen 1, 501–522.
Francke-Grosmann, H. (1967). “Ectosymbiosis in wood-inhabiting insects,” in Associations of Invertebrates, Birds, Ruminants, and Other Biota, ed. S. M. Henry (London: Acad. Press), 141–205. doi: 10.1016/b978-1-4832-2758-0.50010-2
Freeman, S., Sharon, M., Maymon, M., Mendel, Z., Protasov, A., Aoki, T., et al. (2013). Fusarium euwallaceae sp. nov.–a symbiotic fungus of Euwallacea sp., an invasive ambrosia beetle in Israel and California. Mycologia 105, 1595–1606. doi: 10.3852/13-066
Gao, C., and Fleet, G. H. (1988). The effects of temperature and pH on the ethanol tolerance of the wine yeasts, Saccharomyces cerevisiae, Candida stellata and Kloeckera apiculata. J. Appl. Bacteriol. 65, 405–409. doi: 10.1111/j.1365-2672.1988.tb01909.x
Glass, N. L., and Donaldson, G. C. (1995). Development of primer sets designed for use with the PCR to amplify conserved genes from filamentous ascomycetes. Appl. Environ. Microbiol. 61, 1323–1330. doi: 10.1128/aem.61.4.1323-1330.1995
Goidanich, G. (1936). II genre di Ascorniceti ‘Grosmanni’G. Goid. Bolletino della Stazione di Patologia vegetale di Roma 16, 26–40.
Graham, K. (1968). Anaerobic induction of primary chemical attractancy for ambrosia beetles. Can. J. Zool. 46, 905–908. doi: 10.1139/z68-127
Hammerbacher, A., Schmidt, A., Wadke, N., Wright, L. P., Schneider, B., Bohlmann, J., et al. (2013). A common fungal associate of the spruce bark beetle metabolizes the stilbene defenses of norway spruce. Plant Physiol. 162, 1324–1336. doi: 10.1104/pp.113.218610
Harrington, T., Aghayeva, D., and Fraedrich, S. (2010). New combinations in Raffaelea, Ambrosiella, and Hyalorhinocladiella, and four new species from the Redbay ambrosia beetle, Xyleborus glabratus. Mycotaxon 111, 337–361. doi: 10.5248/111.337
Harrington, T. C. (2005). “Ecology and evolution of mycophagous bark beetles and their fungal partners,” in Ecological and Evolutionary Advances in Insect-Fungal Associations, eds F. E. Vega and M. Blackwell (Oxford: Oxford University Press), 257–291.
Hulcr, J., Atkinson, T. H., Cognato, A. I., Jordal, B. H., and McKenna, D. D. (2015). “Morphology, taxonomy, and phylogenetics of bark beetles,” in Bark Beetles, eds F. Vega and R. Hofstetter (Orlando, FL: Elsevier), 41–84. doi: 10.1016/b978-0-12-417156-5.00002-2
Hulcr, J., and Dunn, R. R. (2011). The sudden emergence of pathogenicity in insect–fungus symbioses threatens naive forest ecosystems. Proc. R. Soc. B Biol. Sci. 278, 2866–2873. doi: 10.1098/rspb.2011.1130
Hulcr, J., and Stelinski, L. L. (2017). The ambrosia symbiosis: from evolutionary ecology to practical management. Annu. Rev. Entomol. 62, 285–303. doi: 10.1146/annurev-ento-031616-035105
Kandasamy, D., Gershenzon, J., Andersson, M. N., and Hammerbacher, A. (2019). Volatile organic compounds influence the interaction of the Eurasian spruce bark beetle (Ips typographus) with its fungal symbionts. ISME J. 13, 1788–1800. doi: 10.1038/s41396-019-0390-3
Kandasamy, D., Gershenzon, J., and Hammerbacher, A. (2016). Volatile organic compounds emitted by fungal associates of conifer bark beetles and their potential in bark beetle control. J. Chem. Ecol. 42, 952–969. doi: 10.1007/s10886-016-0768-x
Kelsey, R. G., Gallego, D., Sánchez-Garcá, F., and Pajares, J. (2014). Ethanol accumulation during severe drought may signal tree vulnerability to detection and attack by bark beetles. Can. J. Forest Res. 44, 554–561. doi: 10.1139/cjfr-2013-0428
Kimmerer, T. W., and Kozlowski, T. T. (1982). Ethylene, ethane, acetaldehyde, and ethanol production by plants under stress. Plant Physiol. 69, 840–847. doi: 10.1104/pp.69.4.840
Kimmerer, T. W., and Stringer, M. A. (1988). Alcohol dehydrogenase and ethanol in the stems of trees. Plant Physiol. 87, 693–697. doi: 10.1104/pp.87.3.693
Kirkendall, L. R., Biedermann, P. H. W., and Jordal, B. H. (2015). “Evolution and diversity of bark and ambrosia beetles,” in Bark Beetles, eds F. E. Vega and R. W. Hofstetter (London: Academic Press), 85–156. doi: 10.1016/b978-0-12-417156-5.00003-4
Kozlowski, T. (1992). Carbohydrate sources and sinks in woody plants. Bot. Rev. 58, 107–222. doi: 10.1007/bf02858600
Kühnholz, S., Borden, J. H., and Uzunovic, A. (2001). Secondary ambrosia beetles in apparently healthy trees: adaptations, potential causes and suggested research. Integrated Pest Manage. Rev. 6, 209–219.
Kuhns, E. H., Tribuiani, Y., Martini, X., Meyer, W. L., Peña, J., Hulcr, J., et al. (2014). Volatiles from the symbiotic fungus Raffaelea lauricola are synergistic with Manuka lures for increased capture of the Redbay ambrosia beetle Xyleborus glabratus. Agricult. Forest Entomol. 16, 87–94. doi: 10.1111/afe.12037
Kumar, S., Stecher, G., and Tamura, K. (2016). Mega7: molecular evolutionary genetics analysis version 7.0 for bigger datasets. Mol. Biol. Evol. 33, 1870–1874. doi: 10.1093/molbev/msw054
La Spina, S., De Cannière, C., Dekri, A., and Grégoire, J. C. (2013). Frost increases beech susceptibility to Scolytine ambrosia beetles. Agricult. Forest Entomol. 15, 157–167. doi: 10.1111/j.1461-9563.2012.00596.x
Lam, F. H., Ghaderi, A., Fink, G. R., and Stephanopoulos, G. (2014). Engineering alcohol tolerance in yeast. Science 346, 71–75. doi: 10.1126/science.1257859
Lehenberger, M., Benz, J. P., Müller, J., and Biedermann, P. H. W. (2018). Trypodendron domesticum (Linne) und Trypodendron lineatum (Olivier) (Curculionidae; Scolytinae) als potentielle Vektoren von xylobionten und sapro-xylobionten Pilzen. Mitteilungen der Deutschen Gesellschaft für Allgemeine und Angewandte Entomologie 21, 279–282.
Li, Q., Zhang, G., Guo, H., He, G., and Liu, B. (2014). Euwallacea fornicatus, an important pest insect attacking Acer buergerianum. Forest Pest Dis. 33, 25–27.
Lieutier, F., Yart, A., and Salle, A. (2009). Stimulation of tree defenses by Ophiostomatoid fungi can explain attack success of bark beetles on conifers. Ann. Forest Sci. 66, 801–801. doi: 10.1051/forest/2009066
Liou, J., Shih, J., and Tzean, S. (1999). Esteya, a new nematophagous genus from Taiwan, attacking the pinewood nematode (Bursaphelenchus xylophilus). Mycol. Res. 103, 242–248. doi: 10.1017/s0953756298006984
MacDonald, R. C., and Kimmerer, T. W. (1991). Ethanol in the stems of trees. Physiol. Plant 82, 582–588. doi: 10.1034/j.1399-3054.1991.820415.x
Madeira, F., Park, Y. M., Lee, J., Buso, N., Gur, T., Madhusoodanan, N., et al. (2019). The EMBL-EBI search and sequence analysis tools APIs in 2019. Nucleic Acids Res. 47, W636–W641.
McGovern, P. E., Zhang, J., Tang, J., Zhang, Z., Hall, G. R., Moreau, R. A., et al. (2004). Fermented beverages of pre-and proto-historic China. Proc. Natl. Acad. Sci. U.S.A. 101, 17593–17598. doi: 10.1073/pnas.0407921102
McKenzie, J., and Parsons, P. (1972). Alcohol tolerance: an ecological parameter in the relative success of Drosophila melanogaster and Drosophila simulans. Oecologia 10, 373–388. doi: 10.1007/bf00345738
Miller, D. R., and Rabaglia, R. J. (2009). Ethanol and (-)-α-pinene: attractant kairomones for bark and ambrosia beetles in the southeastern us. J. Chem. Ecol. 35, 435–448. doi: 10.1007/s10886-009-9613-9
Minh, B. Q., Nguyen, M. A. T., and Haeseler, A. (2013). Ultrafast approximation for phylogenetic bootstrap. Mol. Biol. Evol. 30, 1188–1195. doi: 10.1093/molbev/mst024
Moeck, H. A. (1970). Ethanol as the primary attractant for the ambrosia beetle Trypodendron lineatum (Coleoptera: Scolytidae). Can. Entomol. 102, 985–995. doi: 10.4039/ent102985-8
Müller, U. G., Gerardo, N. M., Aanen, D. K., Six, D. L., and Schultz, T. R. (2005). The evolution of agriculture in insects. Annu. Rev. Ecol. Evol. Syst. 36, 563–595.
Nguyen, L. T., Schmidt, H. A., Haeseler, A. V., and Minh, B. Q. (2015). IQ-tree: a fast and effective stochastic algorithm for estimating maximum likelihood phylogenies. Mol. Biol. Evol. 32, 268–274. doi: 10.1093/molbev/msu300
Nicholas, K. B. (1997). GeneDoc: a Tool for Editing and Annoting Multiple Sequence Alignments. Available online at: https://genedoc.software.informer.com (accessed December 22, 2020).
Nuotclà, J. A., Biedermann, P. H. W., and Taborsky, M. (2019). Pathogen defence is a potential driver of social evolution in ambrosia beetles. Proc. R. Soc. B 286:20192332. doi: 10.1098/rspb.2019.2332
O’Donnell, K., and Cigelnik, E. (1997). Two divergent intragenomic rDNA ITS2 types within a monophyletic lineage of the fungus Fusarium are non-orthologous. Mol. Phylogenet. Evol. 7, 103–116. doi: 10.1006/mpev.1996.0376
Paschos, T., Xiros, C., and Christakopoulos, P. (2015). Ethanol effect on metabolic activity of the ethalogenic fungus Fusarium oxysporum. BMC Biotechnol. 15:15.
Pina, C., Santos, C., Couto, J. A., and Hogg, T. (2004). Ethanol tolerance of five non-Saccharomyces wine yeasts in comparison with a strain of Saccharomyces cerevisiae—influence of different culture conditions. Food Microbiol. 21, 439–447. doi: 10.1016/j.fm.2003.10.009
Posada, D., and Crandall, K. A. (1998). Modeltest: testing the model of DNA substitution. Bioinformatics (Oxford, England) 14, 817–818. doi: 10.1093/bioinformatics/14.9.817
Ramadhar, T. R., Beemelmanns, C., Currie, C. R., and Clardy, J. (2014). Bacterial symbionts in agricultural systems provide a strategic source for antibiotic discovery. J. Antibiotics 67, 53–58. doi: 10.1038/ja.2013.77
Ranger, C. M., Biedermann, P. H. W., Phuntumart, V., Beligala, G. U., Gosh, S., Palmquist, D. E., et al. (2018). Symbiont selection via alcohol benefits fungus farming by ambrosia beetles. Proc. Natl. Acad. Sci. U.S.A. 115, 4447–4452. doi: 10.1073/pnas.1716852115
Ranger, C. M., Reding, M. E., Gandhi, K. J., Oliver, J. B., Schultz, P. B., Cañas, L., et al. (2011). Species dependent influence of (-)-α-pinene on attraction of ambrosia beetles (Coleoptera: Curculionidae: Scolytinae) to ethanol-baited traps in nursery agroecosystems. J. Econ. Entomol. 104, 574–579. doi: 10.1603/ec10243
Ranger, C. M., Reding, M. E., Schultz, P. B., and Oliver, J. B. (2013). Influence of flood-stress on ambrosia beetle host-selection and implications for their management in a changing climate. Agricult. Forest Entomol. 15, 56–64. doi: 10.1111/j.1461-9563.2012.00591.x
Ranger, C. M., Schultz, P. B., Frank, S. D., Chong, J. H., and Reding, M. E. (2015). Non-native ambrosia beetles as opportunistic exploiters of living but weakened trees. PLoS One 10:e0131496. doi: 10.1371/journal.pone.0131496
Reding, M. E., Schultz, P. B., Ranger, C. M., and Oliver, J. B. (2011). Optimizing ethanol-baited traps for monitoring damaging ambrosia beetles (Coleoptera: Curculionidae, Scolytinae) in ornamental nurseries. J. Econ. Entomol. 104, 2017–2024. doi: 10.1603/ec11119
Rehner, S. A., and Samuels, G. J. (1994). Taxonomy and phylogeny of Gliocladium analysed from nuclear large subunit ribosomal DNA sequences. Mycol. Res. 98, 625–634. doi: 10.1016/s0953-7562(09)80409-7
Rollins, F., Jones, K. G., Krokene, P., Solheim, H., and Blackwell, M. (2001). Phylogeny of asexual fungi associated with bark and ambrosia beetles. Mycologia 93, 991–996. doi: 10.2307/3761761
Ruchala, J., Kurylenko, O. O., Dmytruk, K. V., and Sibirny, A. A. (2020). Construction of advanced producers of first-and second-generation ethanol in Saccharomyces cerevisiae and selected species of non-conventional yeasts (Scheffersomyces stipitis, Ogataea polymorpha). J. Industrial Microbiol. Biotechnol. 47, 109–132. doi: 10.1007/s10295-019-02242-x
Scheuring, I., and Yu, D. W. (2012). How to assemble a beneficial microbiome in three easy steps. Ecol. Lett. 15, 1300–1307. doi: 10.1111/j.1461-0248.2012.01853.x
Schneider, H., and Jeffries, T. W. (1989). Conversion of pentoses to ethanol by yeasts and fungi. Crit. Rev. Biotechnol. 9, 1–40. doi: 10.3109/07388558909040614
Scott, J. J., Oh, D. C., Yuceer, M. C., Klepzig, K. D., Clardy, J., and Currie, C. R. (2008). Bacterial protection of beetle-fungus mutualism. Science 322, 63–63. doi: 10.1126/science.1160423
Seibold, S., Müller, J., Baldrian, P., Cadotte, M. W., Štursová, M., Biedermann, P. H. W., et al. (2019). Fungi associated with beetles dispersing from dead wood – Let’s take the beetle bus! Fungal Ecol. 39, 100–108. doi: 10.1016/j.funeco.2018.11.016
Singh, A., and Kumar, P. (1991). Fusarium oxysporum: status in bioethanol production. Crit. Rev. Biotechnol. 11, 129–147. doi: 10.3109/07388559109040619
Singh, A., Kumar, P., and Schügerl, K. (1992). “Bioconversion of cellulosic materials to ethanol by filamentous fungi,” in Enzymes and Products from Bacteria Fungi and Plant Cells. Advances in Biochemical Engineering/Biotechnology, Vol. 45, (Berlin, Heidelberg: Springer), 29–55. doi: 10.1007/BFb0008755
Six, D. L. (2013). The bark beetle holobiont: why microbes matter. J. Chem. Ecol. 39, 989–1002. doi: 10.1007/s10886-013-0318-8
Six, D. L., and Klepzig, K. D. (2004). Dendroctonus bark beetles as model systems for studies on symbiosis. Symbiosis 37, 207–232.
Six, D. L., and Paine, T. D. (1998). Effects of mycangial fungi and host tree species on progeny survival and emergence of Dendroctonus ponderosae (Coleoptera: Scolytidae). Environ. Entomol. 27, 1393–1401. doi: 10.1093/ee/27.6.1393
Skelton, J., Loyd, A., Smith, J. A., Blanchette, R. A., Held, B. W., and Hulcr, J. (2020). Fungal symbionts of bark and ambrosia beetles can suppress decomposition of pine sapwood by competing with wood-decay fungi. Fungal Ecol. 45:100926. doi: 10.1016/j.funeco.2020.100926
Skory, C. D., Freer, S. N., and Bothast, R. J. (1997). Screening for ethanol-producing filamentous fungi. Biotechnol. Lett. 19, 203–206.
Skrodenyte-Arbaciauskiene, V., Buda, V., Radžiute, S., and Stunženas, V. (2006). Myrcene-resistant bacteria isolated from the gut of phytophagous insect Ips typographus. Ekologija 4, 1–6.
Thomson, J. M., Gaucher, E. A., Burgan, M. F., De Kee, D. W., Li, T., Aris, J. P., et al. (2005). Resurrecting ancestral alcohol dehydrogenases from yeast. Nature Genet. 37, 630–635. doi: 10.1038/ng1553
Trifinopoulos, J., Nguyen, L. T., von Haeseler, A., and Minh, B. Q. (2016). W-IQ-TREE: a fast online phylogenetic tool for maximum likelihood analysis. Nucleic Acids Res. 44, W232–W235.
Tunc, S., Chollet, E., Chalier, P., Preziosi-Bello, L., and Gontard, N. (2007). Combined effect of volatile antimicrobial agents on the growth of Penicillium notatum. Int. J. Food Microbiol. 113, 263–270. doi: 10.1016/j.ijfoodmicro.2006.07.004
Ueng, P. P., and Gong, C. (1982). Ethanol production from pentoses and sugar-cane bagasse hemicellulose hydrolysate by Mucor and Fusarium species. Enzyme Microbial Technol. 4, 169–171. doi: 10.1016/0141-0229(82)90111-9
Um, S., Fraimout, A., Sapountzis, P., Oh, D. C., and Poulsen, M. (2013). The fungus-growing termite Macrotermes natalensis harbors bacillaene-producing Bacillus sp. that inhibit potentially antagonistic fungi. Sci. Rep. 3, 1–7. doi: 10.3109/19401736.2014.961142
Vanderpool, D., Bracewell, R. R., and McCutcheon, J. P. (2018). Know your farmer: ancient origins and multiple independent domestications of ambrosia beetle fungal cultivars. Mol. Ecol. 27, 2077–2094. doi: 10.1111/mec.14394
Vilgalys, R., and Hester, M. (1990). Rapid genetic identification and mapping of enzymatically amplified ribosomal DNA from several Cryptococcus species. J. Bacteriol. 172, 4238–4246. doi: 10.1128/jb.172.8.4238-4246.1990
Vissa, S., and Hofstetter, R. W. (2017). “The role of mites in bark and ambrosia beetle-fungal interactions,” in Insect Physiology and Ecology, ed. V. D. C. Shields (Rijeka, Croatia: InTech), 135–156. doi: 10.5772/67619
Vité, J. P., Bakke, A., and Renwick, J. A. A. (1972). Pheromones in Ips (Coleoptera: Scolytidae): occurrence and production. Can. Entomol. 104, 1967–1975. doi: 10.4039/ent1041967-12
Vuillemin, P. M. (1912). Beauveria, nouveau genre de Verticilliacées. Bull. Soc. Botanique de France 59, 34–40. doi: 10.1080/00378941.1912.10832379
Wadke, N., Kandasamy, D., Vogel, H., Lah, L., Wingfield, B. D., Paetz, C., et al. (2016). The bark-beetle-associated fungus, Endoconidiophora polonica, utilizes the phenolic defense compounds of its host as a carbon source. Plant Physiol. 171, 914–931.
Wainwright, M., Ali, T. A., and Killham, K. (1994). Anaerobic growth of fungal mycelium from soil particles onto nutrient-free silica gel. Mycol. Res. 98, 761–762. doi: 10.1016/s0953-7562(09)81051-4
White, T. J., Bruns, T., Lee, S. J. W. T., and Taylor, J. (1990). “Amplification and direct sequencing of fungal ribosomal RNA genes for phylogenetics,” in PCR Protocols: a Guide to Methods and Applications, Vol. 18, eds M. A. Innis, D. H. Gelfand, J. J. Sninsky, and T. J. White (New York, NY: Academic Press), 315–322. doi: 10.1016/b978-0-12-372180-8.50042-1
Whitney, H., Bandoni, R., and Oberwinkler, F. (1987). Entomocorticium dendroctoni gen. et sp. nov. (Basidiomycotina), a possible nutritional symbionte of the mountain pine beetle in lodgepole pine in British Columbia. Can. J. Bot. 65, 95–102. doi: 10.1139/b87-013
Wood, D. L. (1982). The role of pheromones, kairomones, and allomones in the host selection and colonization behavior of bark beetles. Annu. Rev. Entomol. 27, 411–446. doi: 10.1146/annurev.en.27.010182.002211
Zhao, T., Kandasamy, D., Krokene, P., Chen, J., Gershenzon, J., and Hammerbacher, A. (2019). Fungal associates of the tree-killing bark beetle, Ips typographus, vary in virulence, ability to degrade conifer phenolics and influence bark beetle tunneling behavior. Fungal Ecol. 38, 71–79. doi: 10.1016/j.funeco.2018.06.003
Keywords: ambrosia fungi, bark and ambrosia beetles, symbiont selection, ethanol, detoxification, Ips typographus
Citation: Lehenberger M, Benkert M and Biedermann PHW (2021) Ethanol-Enriched Substrate Facilitates Ambrosia Beetle Fungi, but Inhibits Their Pathogens and Fungal Symbionts of Bark Beetles. Front. Microbiol. 11:590111. doi: 10.3389/fmicb.2020.590111
Received: 31 July 2020; Accepted: 16 December 2020;
Published: 13 January 2021.
Edited by:
Michael Poulsen, University of Copenhagen, DenmarkReviewed by:
Caterina Villari, University of Georgia, United StatesCopyright © 2021 Lehenberger, Benkert and Biedermann. This is an open-access article distributed under the terms of the Creative Commons Attribution License (CC BY). The use, distribution or reproduction in other forums is permitted, provided the original author(s) and the copyright owner(s) are credited and that the original publication in this journal is cited, in accordance with accepted academic practice. No use, distribution or reproduction is permitted which does not comply with these terms.
*Correspondence: Maximilian Lehenberger, bWF4aW1pbGlhbi5sZWhlbmJlcmdlckB1bmktd3VlcnpidXJnLmRl
†ORCID: Maximilian Lehenberger, orcid.org/0000-0001-9097-9715; Peter H. W. Biedermann, orcid.org/0000-0003-4234-5659
Disclaimer: All claims expressed in this article are solely those of the authors and do not necessarily represent those of their affiliated organizations, or those of the publisher, the editors and the reviewers. Any product that may be evaluated in this article or claim that may be made by its manufacturer is not guaranteed or endorsed by the publisher.
Research integrity at Frontiers
Learn more about the work of our research integrity team to safeguard the quality of each article we publish.