- 1Department of Biochemistry and Molecular Biology, School of Basic Medical Sciences, Anhui Medical University, Hefei, China
- 2The Key Laboratory of Zoonoses of Anhui, Anhui Medical University, Hefei, China
- 3The Key Laboratory of Pathogen Biology of Anhui Province, Anhui Medical University, Hefei, China
- 4School of Nursing, Anhui Medical University, Hefei, China
Autophagy is an important homeostatic mechanism, in which lysosomes degrade and recycle cytosolic components. As a key defense mechanism against infections, autophagy is involved in the capture and elimination of intracellular parasites. However, intracellular parasites, such as Toxoplasma gondii, have developed several evasion mechanisms to manipulate the host cell autophagy for their growth and establish a chronic infection. This review provides an insight into the autophagy mechanism used by the host cells in the control of T. gondii and the host exploitation by the parasite. First, we summarize the mechanism of autophagy, xenophagy, and LC3-associated phagocytosis. Then, we illustrate the process of autophagy proteins-mediated T. gondii clearance. Furthermore, we discuss how the parasite blocks and exploits this process for its survival.
Introduction
Toxoplasma gondii (T. gondii) is an obligate intracellular protozoan parasite, which can infect warm-blooded animals and cause morbidity and mortality in humans and animals worldwide (Halonen and Weiss, 2013). T. gondii is unified as a single species in the genus Toxoplasma, although recent estimates based on genomic barcoding show a much greater diversity across continents. Most strains of Toxoplasma isolated in Europe and North America belong to three main clonal lineages known as Types I, II, and III strains, which have different virulence in mice and may cause different human sequelae (Darde et al., 1992; Howe and Sibley, 1995; Howe et al., 1997; Machacova et al., 2016). T. gondii strains in South America are genetically more diverse than those in North America and Europe. However, Chinese 1 strain was reported to be the dominant strain, differing from the three main clones previously reported in East Asia, particularly in China (Zhou et al., 2010; Chen et al., 2011; Wang et al., 2013; Li et al., 2014).
Toxoplasma gondii is transmitted through food-borne, animal-to-human, congenital, blood transfusion, and organ transplantation (Montoya and Liesenfeld, 2004; Chu et al., 2017; Yu et al., 2017). T. gondii can cause human congenital and acquired infection. The congenital form may be subclinical or manifest as destructive damage to the internal organs, eyes, and brain (Halonen and Weiss, 2013; Hampton, 2015). Available data suggest about a third of the world’s population have developed long lasting serum antibodies in response to previous subclinical infection. Most of the toxoplasmosis is asymptomatic (Halonen and Weiss, 2013; Parlog et al., 2014). In rare cases, acquired toxoplasmosis could cause serious, disseminated diseases such as meningoencephalitis, pneumonia, or myocarditis. In addition, acquired ocular disease generally occurs either alone as a result of reactivation from chronic infection, or as a complication of acute systemic disease, including newly acquired disease (Montoya and Remington, 1996; Montoya and Liesenfeld, 2004; Hampton, 2015). In the acute phase of infection, tachyzoites rapidly replicate and spread to various organs through the blood or lymphatic system. In the chronic phase, T. gondii forms tissue cysts (bradyzoites) in which the parasite can survive for a long time or even lifetime lurking in the brain, tissue, muscle, retina, etc. (Lyons et al., 2002; Halonen and Weiss, 2013; Li et al., 2016).
Autophagy, a well-conserved and tightly regulated process in eukaryotes, which is key for cellular homeostasis, cell survival, and degradation of damaged cytoplasmic components or harmful exogenous substrates (Levine and Kroemer, 2008; Wang et al., 2009). As a key defense mechanism against infections, it also involves catching parasites inside cells and then clearing them out (Besteiro, 2017). Moreover, autophagy is thought to be an integral component of the innate immune response, targeting intracellular parasites, and parasites in damaged vacuoles and phagosomes, limiting their growth (Huang and Brumell, 2014). Recent study has shown that 4-Hydroxybenzaldehyde restricts the intracellular growth of Toxoplasma by activating SIRT1-mediated autophagy in macrophages (Lee et al., 2020). However, intracellular pathogens have also evolved diverse mechanisms to avoid autophagy (Steele et al., 2015). In this review, we focus on recent progress on understanding interactions between T. gondii and host autophagy pathways.
Mechanisms of Autophagy
Autophagy is a conservative catabolism system in cells, in which double-membraned autophagosome engulfs cytoplasmic components and degrades them after fusion with lysosomes (Choi et al., 2013; Paulus and Xavier, 2015; Galluzzi et al., 2017). Autophagy is mainly dependent on autophagy (ATG) proteins leading to the formation of autophagosome and lysosomal degradation of autophagy substrates. This pathway involves several different steps, such as the formation of a cup-shaped double membrane capable of engulfing specific substances or chunks of cytoplasm; membrane elongation and closure to form autophagosomes; transport and fusion of autophagosomes and lysosomes; and finally, degradation of the enclosed cytoplasmic contents within the autophagosome and nutrient recycling (Nakatogawa et al., 2009; Parzych and Klionsky, 2014; Figure 1). Starvation and the mammalian target of rapamycin (mTOR) kinase inhibition induce autophagy to provide nutrients for the basic physiological processes of the cells and to maintain homeostasis. Nutrient deprived conditions induce autophagosome formation via the mTORcomplex 1 (mTORC1) inhibition in mammalian cells. The initiation complex of ULK1 activates the phosphatidylinositol 3-kinase (PI3K) complex of Beclin1-Vps34-Vps15-Atg14L (Deretic, 2011; Choi et al., 2014; Paulus and Xavier, 2015). The expansion or elongation of the phagophore need the involvement of two ubiquitin-like (UBL) conjugation systems. The first UBL conjugation system involves the formation of Atg12-Atg5-Atg16 complex. In yeast cells, Atg12 binds covalently to Atg5. This Atg12-Atg5 conjugation is highly dependent on Atg7 (E1 activating enzyme) and Atg10 (E2 activating enzyme). Then, Atg16 non-covalently binds to Atg5 forming the Atg12-Atg5-Atg16 complex, following the Atg12-Atg5 conjugation. The second UBL conjugation system involves the Atg8 or LC3 (in mammals) system. Atg8/LC3 is activated by the EI-like enzyme Atg7 and transferred to the E2-like enzyme Atg3. In the final step, phosphatidylethanolamine (PE) binds to the C-terminal glycine Atg8 to form LC3-phosphatidylethanolamine conjugate (LC3-II) and incorporated into the autophagosomal membrane (Hwang et al., 2012; Huang and Brumell, 2014; Biering et al., 2017; Choi et al., 2017; Wacker et al., 2017). The lysosome subsequently fuses with the outer membrane of the autophagosome to deliver their cytoplasmic cargo for degradation and recycling (Melzer et al., 2008; Paulus and Xavier, 2015; Selleck et al., 2015). Cytoplasmic cargoes are captured in double-membrane structure, called “autophagosomes,” which then mature into autolysosomes that degrade or otherwise eliminate captured cargoes. Another strategy employed by host cells to capture and degrade invading intracellular pathogens is xenophagy. It is a key defense mechanism against various infections involving the elimination intracellular pathogens such as protozoans, bacteria, and viruses (Mizushima et al., 2011; Paulus and Xavier, 2015; Galluzzi et al., 2017). Xenophagy is also considered as an innate component of cellular immune response.
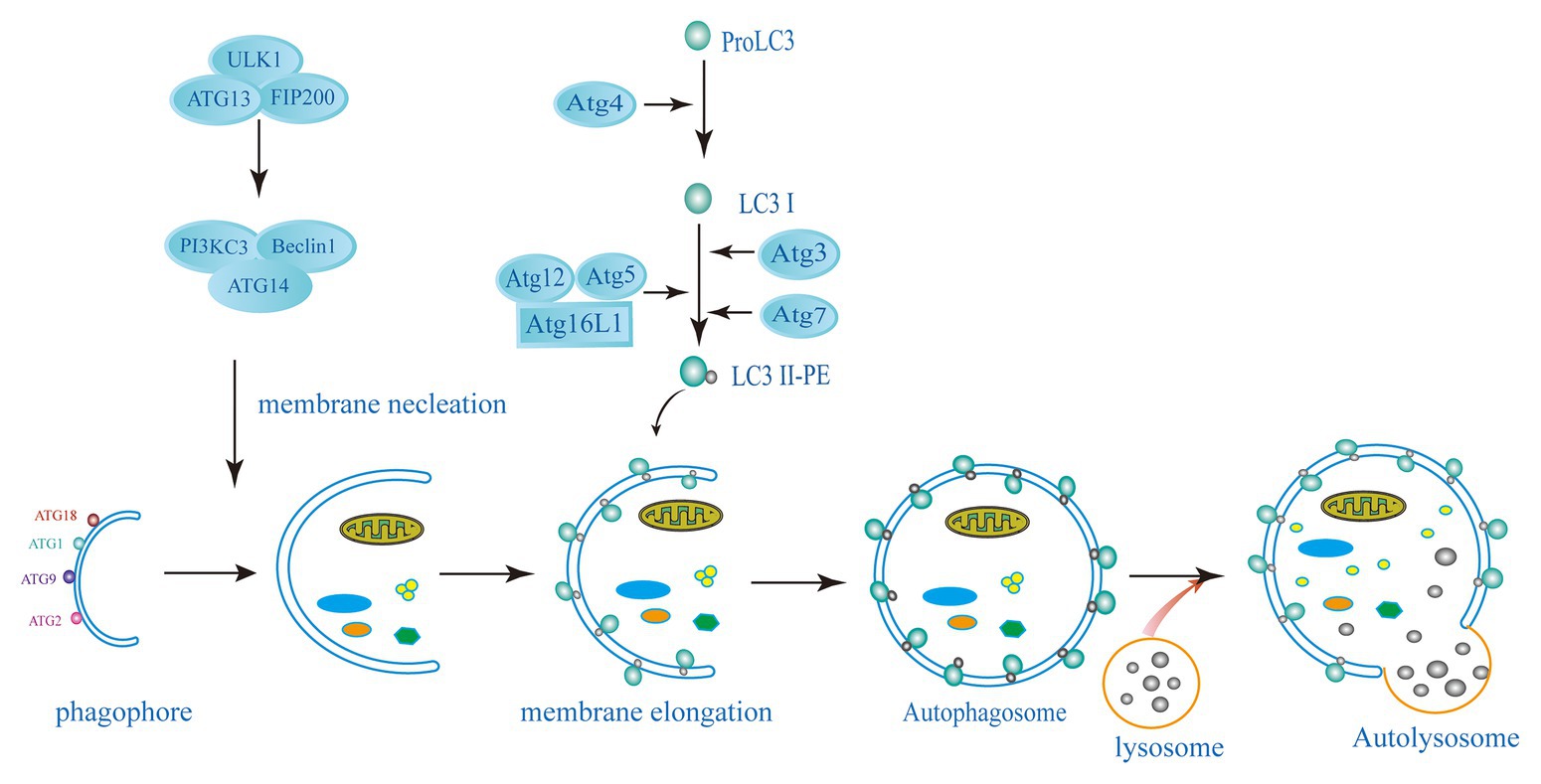
Figure 1. Schematic diagram of the autophagy pathway. The induction of autophagy causes the assembly and elongation of the phagophore and finally forms a structure that can surround the components in cytoplasm. The phagophore expands and develops into a double-membrane structure of autophagosomes. Targets in cytoplasm, like organelles, proteins, and microorganisms, are isolated. Autolysosome has formed by the fussing of autophagosome and lysosome, where hydrolytic enzymes in the autolysosome degrade cytoplasmic cargo. The left side shows the key proteins related to mammalian autophagy (ATG, autophagy-related: ATG3, ATG5, ATG7, ATG4, ATG12, ATG13, ATG14, ATG16L1, FIP200, ULK1, PI3KC3, Beclin1, LC3, and PE).
Autophagy proteins may be involved in certain cellular pathways leading to the elimination of intracellular pathogens but do not constitute autophagy. These cellular pathways are established under certain cellular conditions; some bypass proteins involved in elongation and closure (Atg7, Atg5, and LC3), while others bypass proteins necessary for initiation (ULK1) and nucleation (Beclin1; Steele et al., 2015; Galluzzi et al., 2017). LC3-associated phagocytosis (LAP) is an example of such a process, involves the recruitment of LC3 to single-membrane phagosomes, which surround intracellular pathogens or dead cells (Paulus and Xavier, 2015). This process is independent of the ULK1 initiation complex, while the PtdIns3K complex is important for LAP initiation (Martinez et al., 2015; Besteiro, 2019). During PAMP receptor activation, PI3PK complex is recruited to the phagosomal membrane (Ma et al., 2012). This complex lacks ATG14 but is composed of Rubicon and UV resistance-associated gene (UVRAG; Martinez et al., 2015). With Rubicon’s help, NADPH oxidase 2 (NOX2) then enters the phagosomes. Activation of NOX2 and PI3PK leads to the production reactive oxygen species (ROS) and initiate lipidation of the phagosomal membrane, respectively, and ultimately leading to the formation of LAPosome (LC3-decorated phagosome; Ueyama et al., 2011). Fusing with lysosomes to form a lytic compartment, the LAPosomes then degrades the cargo. Although the effect of LC3 on LAPosome is still unknown, some studies suggested that LC3 could accelerate its maturation by fusion with lysosomes (Martinez et al., 2015; Besteiro, 2019).
Mechanisms of Host Autophagy Proteins-Mediated T. Gondii Clearance
Toxoplasma gondii replicate in the host cell by forming a special endocytic vacuole, called parasitophorous vacuole (PV). The membrane of PV allows T. gondii to develop while protected from intracellular cytoplasmic defense mechanisms (Orlofsky, 2009; Portillo et al., 2017). Increasing literature indicates that T. gondii actively induces autophagic pathways in the infected host cells (Orlofsky, 2009; Wang et al., 2009; Chu et al., 2017; Saeij and Frickel, 2017; Subauste, 2019). Two types of immune response are required in the control of T. gondii: clearance of T. gondii by CD40-mediated autophagy and IFN-γ-induced the clearance of T. gondii through autophagy proteins. Both of them are essential for killing parasites within host cells.
Clearance of T. gondii by CD40-Mediated Autophagy
The parasitic vacuole is formed during the active invasion of T. gondii. Vacuolar membranes have been extensively modified by removing many proteins from host cells and inserting parasite-derived proteins into parasitic vacuoles. Once the PV is formed, its nonfusion properties will be unchanged (Subauste, 2009). So, whether there is a way to change the nonfusion properties of PV is the key question in the interaction between T. gondii and the immune system. Previous studies have demonstrated that this can be achieved via stimulation of CD40 (Andrade et al., 2006; Subauste et al., 2007b). In macrophages infected with T. gondii, stimulation with CD40 can induce the fusion of the PV with lysosomes in an autophagy-dependent manner.
As a member of the TNF receptor superfamily, CD40 expresses on various nonhematopoietic cells and APCs (Subauste, 2009; Van Grol et al., 2013). Several studies have demonstrated that CD40 triggers signaling pathways, which are upstream of ULK1 and Beclin1-PI3KC3 complexes to activate autophagy. CD40 stimulates autophagy via pathways involving CaMKKβ, AMP-activated protein kinase (AMPK), and ULK1, leading to kill T. gondii (Liu et al., 2016). The phosphorylation of Ser-555 ULK1 and autophagy mediated by ULK1 are caused by AMPK (Russell et al., 2013). CD40 can induce phosphorylation of Thr-172 AMPK mediated by CaMKKβ. Besides direct effects on the ULK1/2 complex, AMPK also inhibit mTORC1 by phosphorylating Raptor (a mTORC1 binding partner). CD40 also interacts with TRAF6 to stimulate TNF-α production in macrophages. Recruitment of TRAF6 in the cytoplasmic tail of CD40 enhances the expression of TNF-α. It causes phosphorylation of Bcl-2 at Ser-87 in JNK-dependent way, and binding Beclin 1 to PI3KC3 to induce autophagy (Subauste et al., 2007a; Liu et al., 2016). CD40 can also induce the death of T. gondii by lowering the level of P21 and upregulating the autophagy molecule Beclin 1 (Portillo et al., 2010; Figure 2). CD40 signaling triggers pathogen-lysosome fusion via the autophagic mechanism. ULK1/2 complexes and the Beclin1-PI3KC3 complex activation promote the formation and maturation of autophagosome by recruiting Atg proteins to the isolation membrane (Liu et al., 2016). The phagophore is produced by polymerization of Atg8 (LC3) and Atg12-Atg5 UBL conjugation systems (Kihara et al., 2001; Ohsumi, 2001). The isolation membrane envelops organelles and cytosol, which leads to forming the autophagosomes. Therefore, autophagosomes are recruited around the PV and fused with late endosomal lysosomes to kill T. gondii (Zhao et al., 2007; Subauste, 2009; Chu et al., 2017).
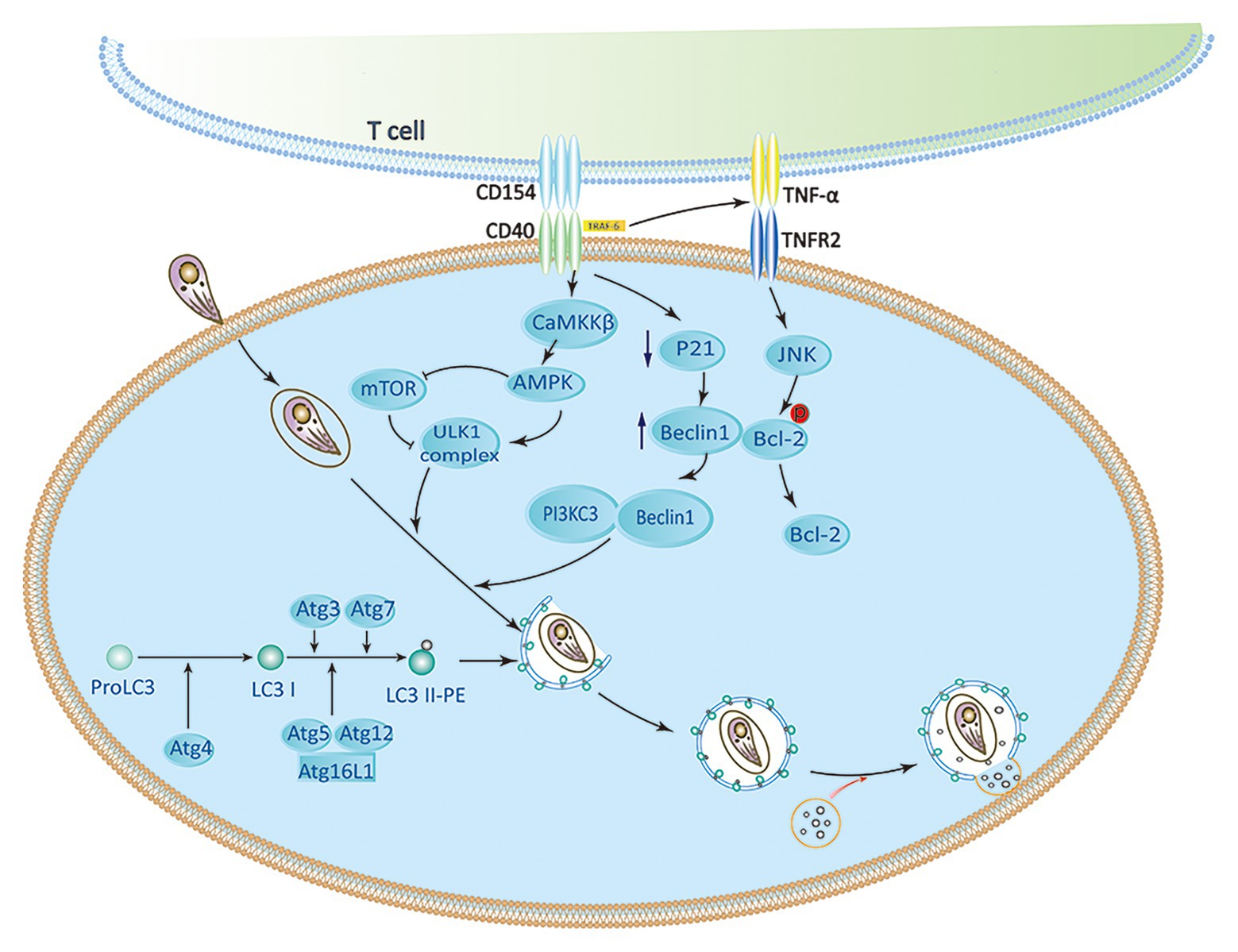
Figure 2. CD40 induces activation of autophagy signaling pathway. CaMKKβ-mediated Threonine-172 AMP-activated protein kinase (AMPK) phosphorylation can be induced by CD40, which leads to Serine-555 ULK1 phosphorylation and ULK1-mediated autophagy. CD40 may upregulate Beclin 1 protein levels by reducing p21. The accumulation of TRAF6 in the cytoplasmic tail of CD40 enhances TNF-α autocrine production, which leads to the phosphorylation of Bcl-2 at Serine 87 in JNK-dependent way and separation of Bcl-2 from Beclin1. ULK1, Beclin 1, PI3KC3, ATG5, ATG7, and lysosomal enzymes play an important role in killing of Toxoplasma gondii caused by CD40 (Subauste, 2009; Liu et al., 2016).
IFN-γ Restricts T. gondii Through Autophagy-Independent Effects of Autophagy Proteins
To restrict the infection, IFN-γ-stimulated murine cells are armed with effector molecules, such as the immunity-related p47GTPases (IRGs) and guanylate-binding proteins (GBPs; Feng et al., 2008). Studies have revealed that the LC3 conjugation system is necessary to recruit IRGs and GBPs to the parasitophorous vacuole membrane (PVM; Choi et al., 2014; Haldar et al., 2014; Ohshima et al., 2014; Lee et al., 2015). Atg5 is an important autophagic gene and is particularly useful for targeting IRGs and GBPs to PVM containing T. gondii. Without ATG5, IRGs and GBPs are usually induced by IFN-γ, and they form aggregates in the cytoplasm instead of targeting vacuoles containing pathogens (Zhao et al., 2008; Choi et al., 2014, 2017; Biering et al., 2017). Once these GTPases are recruited to the LC3-tagged PVM, the PVM surrounding parasites shows extensive vesiculation and blebbing with clusters of small vesicles in the purlieu of the vacuole. At these sites, IRGs localize to small vesicular forms with dense coats from the adjacent PVM, stripping the PV membrane of T. gondii. Denuded parasites are enveloped in autophagic vacuoles that eventually fuse with lysosomes and ultimately limit the growth of T. gondii growth (Ling et al., 2006; Zhao et al., 2008; Clough et al., 2016; Coppens, 2017). PVMs of type I virulent strains are much less intensely loaded with IRG proteins than those of type II or type III avirulent strains in single infection or co-infections (Khaminets et al., 2010). Although IRGs and GBPs participate in the disruption of parasite PVM, the molecular mechanisms of how these IFN-γ induced GTPases interact with the autophagic machinery are poorly understood (Melzer et al., 2008; Whitmarsh and Hunter, 2008; Choi et al., 2014; Biering et al., 2017; Figure 3).
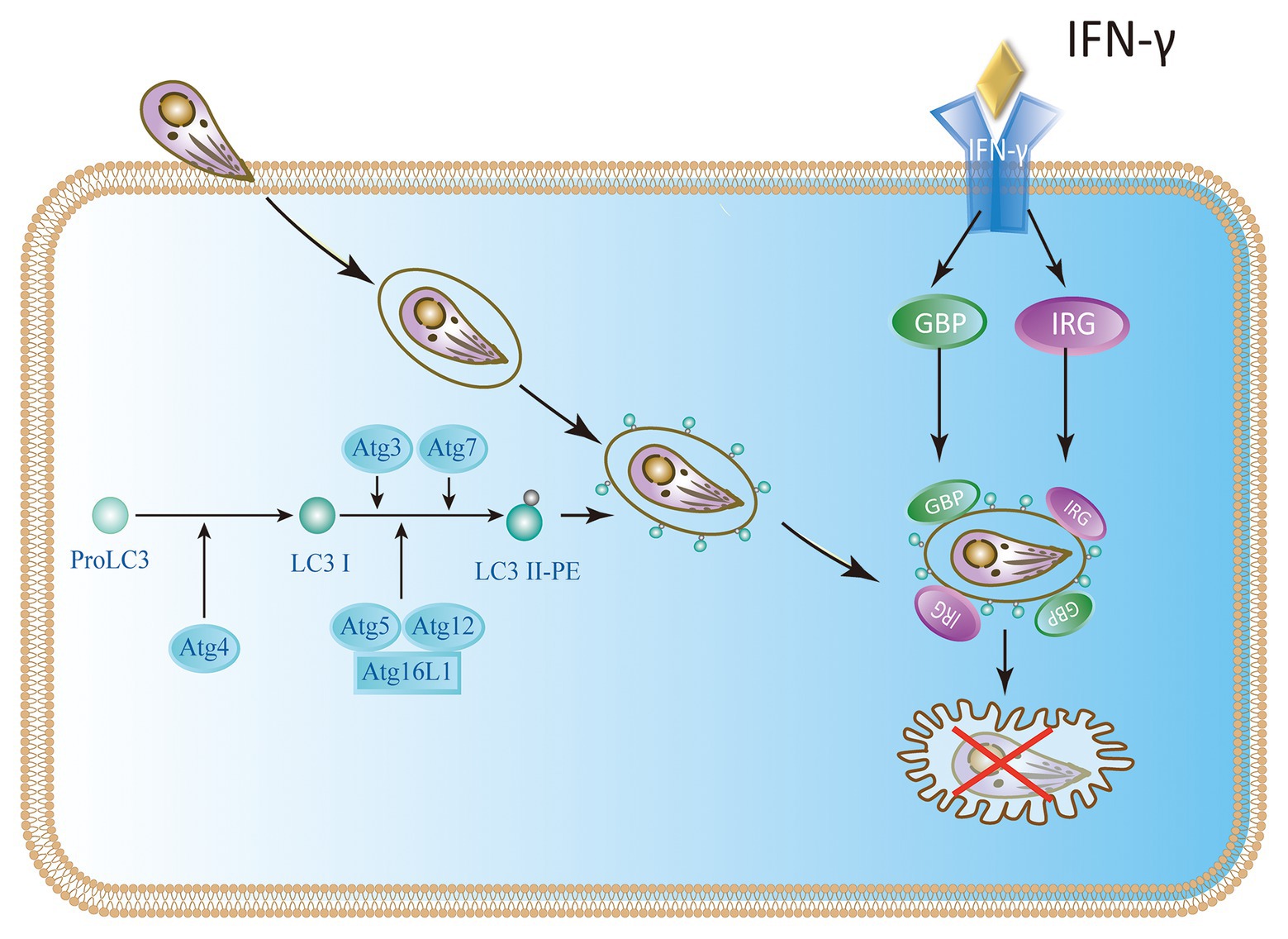
Figure 3. IFN-γ restricts the growth of T. gondii in mouse cells. In mice, the Atg proteins Atg7, Atg3, and the Atg12-Atg5-Atg16L1 complex play a key role in the delivery and binding of LC3 to the autophagosome membrane. They are also involved in targeting immunity-related p47GTPases (IRG) and guanylate-binding protein (GBP) to Toxoplasma parasitophorous vacuole membrane (PVM).
Although some studies have identified p47 IRGs as the primary IFN-γ-induced mechanism of anti-Toxoplasma infection in mice during the acute phase, there is a lack of multitude of IRGs in humans (Subauste, 2009; Clough et al., 2016; Krishnamurthy et al., 2017). Immunity-related GTPase family M protein (IRGM) and immunity-related GTPase cinema (IRGC) are the only two human IRGs. But neither of them are induced by IFN-γ. IRGM plays a role in autophagy and host resistance against Mycobacterium tuberculosis; but its role in toxoplasmosis has not been determined (Portillo et al., 2010; Deretic, 2011; Niedelman et al., 2013; Krishnamurthy et al., 2017). Furthermore, seven IFN-γ-inducible Guanylate binding proteins (GBPs) expressed in humans are involved in IFN-γ dependent clearance of pathogens such as Chlamydia and viruses. There is currently no evidence of GBP-mediated inhibition of T. gondii in humans (Ohshima et al., 2014). Selleck et al. identified new roles for ubiquitination and recruitment of autophagic adapters p62 and NDP52, which could control T. gondii replication in IFN-γ activated human cells (Choi et al., 2014; Lee et al., 2015; Selleck et al., 2015; Clough et al., 2016). The IFN-γ mediated ubiquitin-driven restriction pathways of Toxoplasma type II varies with cell type. Ubiquitin-targeted PVs found in HeLa cells do not acidify but instead restrict Toxoplasma type II by stunting growth. However, IFN-γ stimulated human umbilical vein endothelial cells (HUVEC) have been shown to restrict Toxoplasma in a manner independent of autophagy, where HUVEC exhibits vacuolar lysosomal acidification and subsequent parasite destruction. Thus, we conclude that the two different human cell types deploy the same initial defense molecules ubiquitin, p62 and NDP52 to similar numbers but diverge in the ultimate strategy (Clough et al., 2016; Saeij and Frickel, 2017). Neither of these mechanisms works in all cell types, suggesting the IFN-γ mediated control of T. gondii exists multiple overlapping pathways in human cells (Niedelman et al., 2013; Selleck et al., 2015; Bando et al., 2018). In addition, ATG16L1 and ATG7 are required to encapsulate PV in multiple host membranes. They do not destroy vacuoles or transmit parasite to lysosomes, but they limit nutrient absorption and inhibit parasite growth (Choi et al., 2014; Lee et al., 2015; Selleck et al., 2015). Therefore, IFN-γ mainly relies on ubiquitination and core autophagy to mediate membrane engulfment and cell growth restriction in human cells (Figure 4).
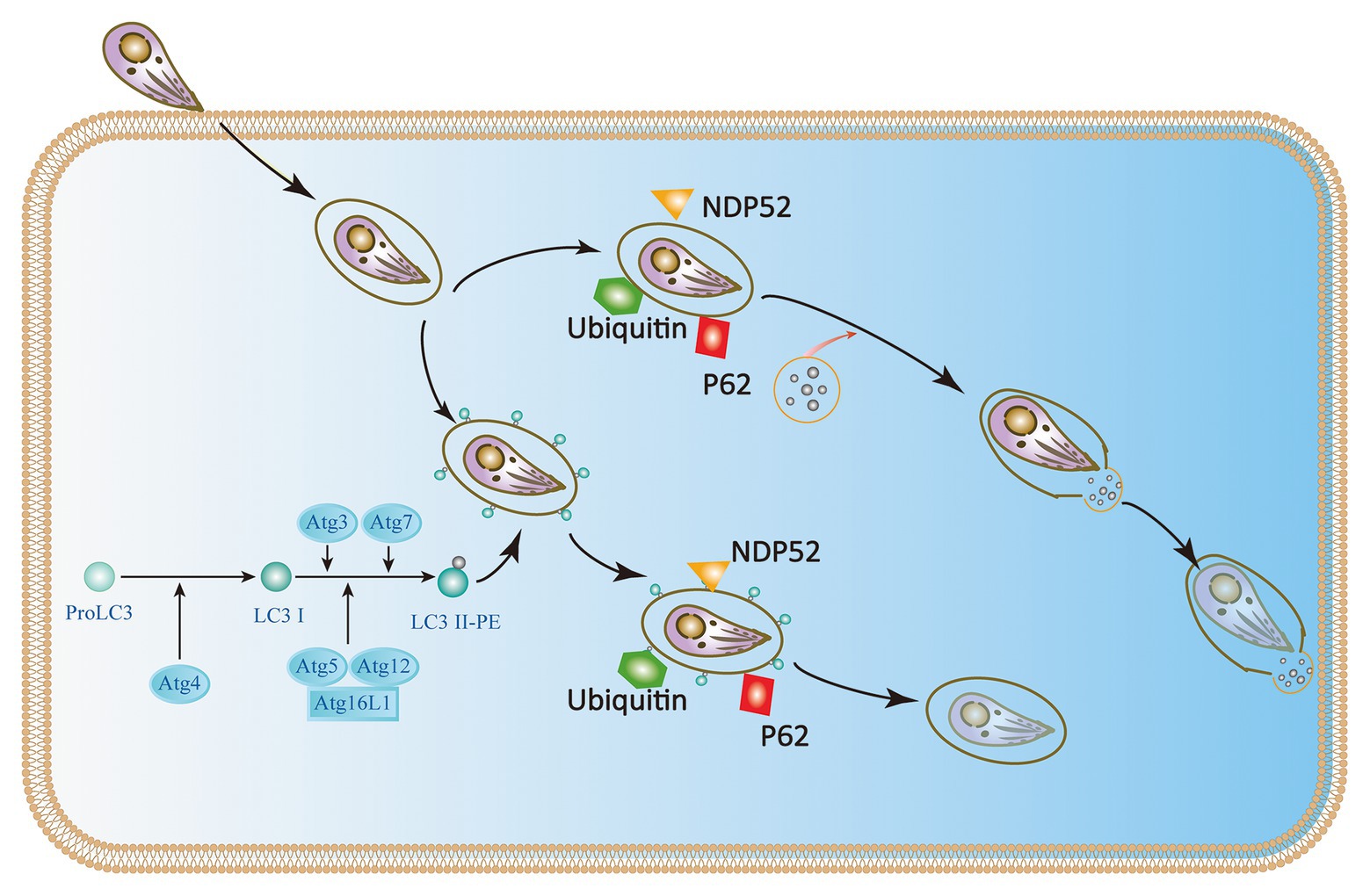
Figure 4. IFN-γ restricts the growth and proliferation of T. gondii in human cells. In HeLa cells, the Atg proteins Atg7, Atg3, and the Atg12-Atg5-Atg16L1 complex target ubiquitin to the Toxoplasma PVM, which causes the recruitment of p62 and NDP52 and subsequently LC3. The parasite is encapsulated in a double-membrane autophagy structure and cannot grow and replicate further. In human umbilical vein endothelial cells (HUVEC) cells, T. gondii encapsulated in parasitophorous vacuole (PV) is recognized by host cell immune effector factors (K63Ub, p62, and NDP52), which leads to lysosomal fusion and subsequent parasite destruction.
Host Cell Signaling Is Manipulated by Toxoplasma Gondii to Avoid Autophagy Targeting
Autophagy is mainly a process of sustaining life. It could not only coordinate the degradation and circulation of important macromolecules such as lipids and amino acids response to stress but also contribute to catching the intracellular parasites and transporting them for destruction (Bando et al., 2018). Avoiding lysosomal degradation is critical for the survival of T. gondii. Toxoplasma can interfere with the host autophagy machinery to escape targeting or even promote their intracellular growth by exploiting autophagy components (Wang et al., 2009; Deretic, 2011; Ohshima et al., 2014). Currently, it is still unclear how Toxoplasma interferes with autophagy.
Toxoplasma gondii activates host cell signaling cascade of the epidermal growth factor receptor (EGFR) by PI3K to avoid targeting autophagy mechanisms to parasitic vacuoles (Muniz-Feliciano et al., 2013; Coppens, 2017; Portillo et al., 2017). Parasites can induce the phosphorylation of EGFR in host cells by secreting several proteins containing multiple domains homologous to EGFR, such as MIC3 and MIC6. PI3K-mediated Akt phosphorylation can activate the autophagosome negative regulator mTORC1, which leads to reverse regulation of autophagy (Muniz-Feliciano et al., 2013; Coppens, 2017; Lopez Corcino et al., 2019). Thus, the Akt signaling pathway is critical for escaping host autophagy to promote parasite survival (Figure 5 I). The moving junction is characterized by the expression of RON4. The formation of the moving junction is accompanied by the activation of focal adhesion kinase (FAK) in the mammalian cell during the invasion. The activation of Src depends on FAK, and then causes the transactivation of EGFR. The activation of EGFR recruits STAT3 signaling to block the activation of key stimulators of autophagy, PKR, and eIF2α. They prevent T. gondii from becoming a target (Portillo et al., 2017). At the later stage of the intracellular phase of T. gondii, EGFR autophosphorylation is maintained through prolonged PKCα/PKCβ-Src signaling, which in turn promote the survival of Toxoplasma through Akt (Lopez Corcino et al., 2019; Figure 5 II).
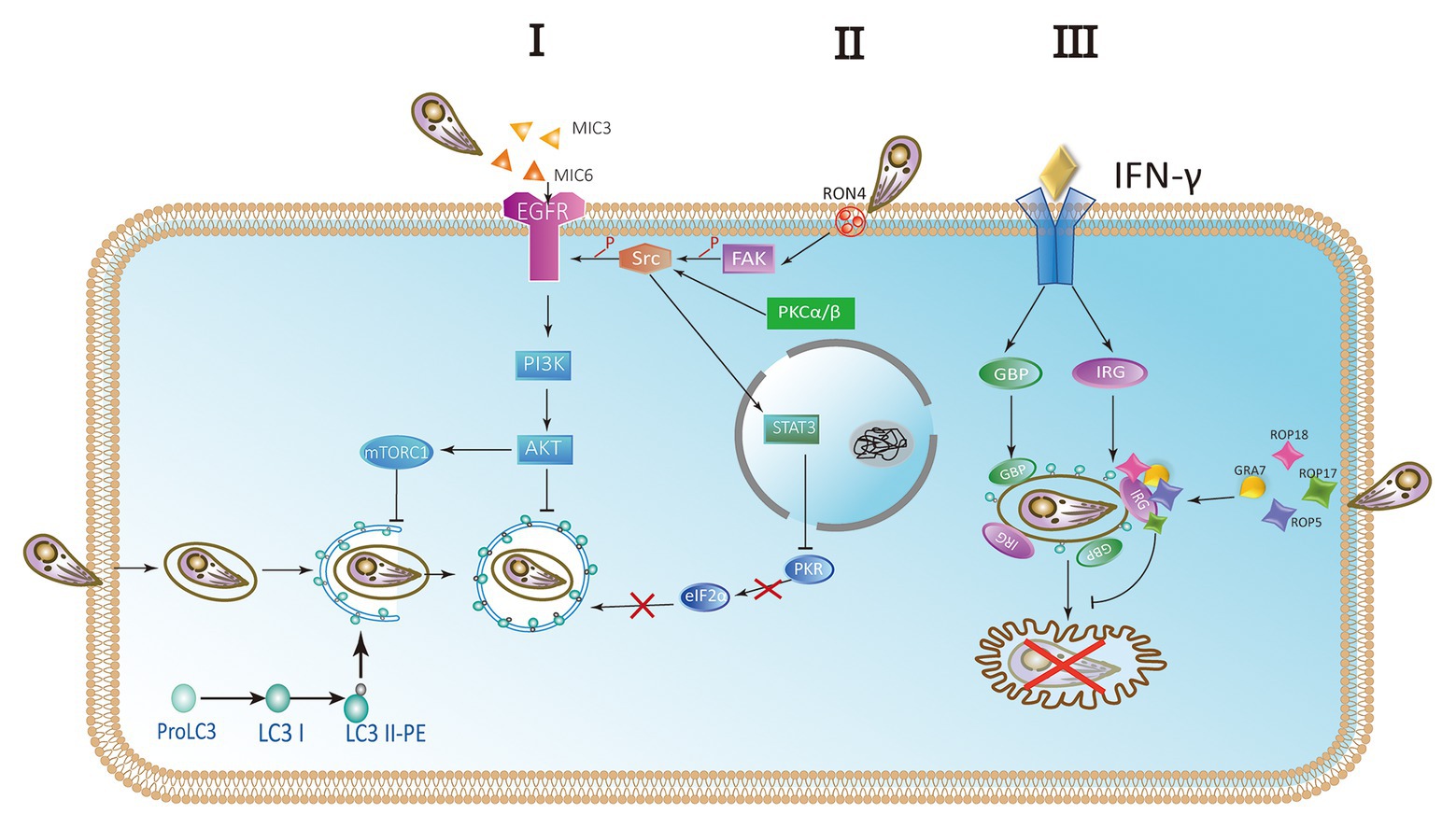
Figure 5. Parasites escape host autophagy destruction. (I) A hypothetical model of Toxoplasma controlling host autophagy by the epidermal growth factor receptor (EGFR)-Akt signaling pathway. MIC protein is released from micronemes upon invasion. MIC3 and MIC6 bind to the host EGFR through the EGF-like domain and are activated by phosphorylation. Phosphatidylinositol 3-kinase (PI3K)-induced Akt phosphorylation and Akt-dependent activation of mTORC1 block the autophagy pathway and signify T. gondii to survive. (II) During the invasion of host cells, RON4 expression was associated with focal adhesion kinase (FAK) activation. In turn, FAK activates Src leading to the transactivation of EGFR in a Src-dependent way. Furthermore, the STAT3 signaling pathway is facilitated to prevent PKR and eIF2a from activation. Blocking the signaling causes the activation of PKR and eIF2a, resulting in autophagic targeting and the killing of parasite. At later stages of the intracellular stage of T. gondii, autophosphorylation of EGFR is maintained through PKCα/PKCβ-Src signaling. (III) The complex of GRA7 and ROP18-ROP17-ROP5 phosphorylates IFN-γ-activated IRGs and GBPs permanently inactivates them to ensure the integrity of PVM and is conducive to the survival of parasite.
IFN-γ is a main effector for activating mammalian cell responses to T. gondii. Recruitment and loading of effector IRGs on PVM are induced by IFN-γ (Martens et al., 2005; Ling et al., 2006; Khaminets et al., 2010). The deposition of ubiquitin on the PVM is promoted by IRGs and subsequently the accumulation of p62-dependent GBP (Haldar et al., 2015). In mouse macrophages and fibroblasts activated by IFN-γ, LC3 is recruited to PVM and can target IRGs to PVM membrane (Park et al., 2016). Associating with the small GTPase ADP-ribosylation factor 1 (Arf1), Gate-16 mediates IRG recruitment (Sasai et al., 2017). Recruitment of IFN-γ-activated IRGs and GBPs resulted in the vesicle formation and rupture of PVM, resulting in the release of T. gondii into the cytoplasm and the death of T. gondii in mouse cells (Martens et al., 2005; Zhao et al., 2008, 2009; Choi et al., 2014; Ohshima et al., 2014). Rop18 is a rhoptry protein, also a polymorphic protein kinase. It mainly determines the virulence of parasite in mice (Taylor et al., 2006; Lei et al., 2014). Together with ROP17, ROP18 complexes mediate the protection from the IRG pathway. The phosphorylation ability of ROP18-ROP17 depends on the presence of virulent alleles of pseudokinase ROP5 (Behnke et al., 2011; Reese et al., 2011). GRA7 is a dense granule protein. It is also a part of the complex of ROP18-ROP17-ROP5 (Hermanns et al., 2016). Binding a conserved surface of IRG, ROP5 proteins promote IRG remain in an inactive GDP binding conformation. As a result, GTP-dependent activation of IRG is prevented. Simultaneously, threonines in the nucleotide-binding domain are exposed. Then ROP18 and ROP17 kinases phosphorylate threonines, resulting in permanent inactivation of IRG (Fleckenstein et al., 2012; Reese et al., 2014; Figure 5 III).
Concluding Remarks
In summary, autophagy is critical in maintaining cellular homeostasis and plays a key role in mechanism against a large number of intracellular pathogens including T. gondii as this pathway can degrade intracellular pathogens via autophagolysosomes. The interaction between T. gondii and host autophagy is a mutual process. However, T. gondii has developed complex evolutionary adaptation and evasion mechanisms to avoid host cell phagocytic recognition. Some PVM associated proteins (rhoptries and dense granules) are known to phosphorylate IRGs, inactivating IRGs. In recent years, the mechanism of T. gondii recognition and invasion has been extensively investigated. However, the mechanisms by which parasites antagonize these responses in different cell lines are still elusive. For instance, in mouse cells, IRGs contribute to immune control of T. gondii, but how it contributes to parasite control in human cells remains enigmatic. Understanding the mechanisms by which these parasites prevent the host’s innate immune defenses and escape autophagy may provide the basis to design new therapeutic strategies to treat toxoplasmosis.
Author Contributions
JD designed the work. MW and OC wrote the first draft of the manuscript. JS and YC did critical revision of the article. All authors contributed to the article and approved the submitted version.
Funding
This work was supported by the National Natural Science Foundation of China (81871674 and 81672046 to JD), the Academic and Technology Leaders reserve candidate Fund of Anhui Province (2016H080 to JD), and the key project of outstanding young talent support program in Anhui province university (gxyqZD2016047 to JD).
Conflict of Interest
The authors declare that the research was conducted in the absence of any commercial or financial relationships that could be construed as a potential conflict of interest.
Acknowledgments
The authors would like to thank members of our laboratories for many thoughtful discussions.
References
Andrade, R. M., Wessendarp, M., Gubbels, M. J., Striepen, B., and Subauste, C. S. (2006). CD40 induces macrophage anti-Toxoplasma gondii activity by triggering autophagy-dependent fusion of pathogen-containing vacuoles and lysosomes. J. Clin. Invest. 116, 2366–2377. doi: 10.1172/JCI28796
Bando, H., Sakaguchi, N., Lee, Y., Pradipta, A., Ma, J. S., Tanaka, S., et al. (2018). Toxoplasma effector TgIST targets host IDO1 to antagonize the IFN-gamma-induced anti-parasitic response in human cells. Front. Immunol. 9:2073. doi: 10.3389/fimmu.2018.02073
Behnke, M. S., Khan, A., Wootton, J. C., Dubey, J. P., Tang, K., and Sibley, L. D. (2011). Virulence differences in Toxoplasma mediated by amplification of a family of polymorphic pseudokinases. Proc. Natl. Acad. Sci. U. S. A. 108, 9631–9636. doi: 10.1073/pnas.1015338108
Besteiro, S. (2017). Autophagy in apicomplexan parasites. Curr. Opin. Microbiol. 40, 14–20. doi: 10.1016/j.mib.2017.10.008
Besteiro, S. (2019). The role of host autophagy machinery in controlling Toxoplasma infection. Virulence 10, 438–447. doi: 10.1080/21505594.2018.1518102
Biering, S. B., Choi, J., Halstrom, R. A., Brown, H. M., Beatty, W. L., Lee, S., et al. (2017). Viral replication complexes are targeted by LC3-guided interferon-inducible GTPases. Cell Host Microbe 22, 74.e77–85.e77. doi: 10.1016/j.chom.2017.06.005
Chen, Z. W., Gao, J. M., Huo, X. X., Wang, L., Yu, L., Halm-Lai, F., et al. (2011). Genotyping of Toxoplasma gondii isolates from cats in different geographic regions of China. Vet. Parasitol. 183, 166–170. doi: 10.1016/j.vetpar.2011.06.013
Choi, J., Biering, S. B., and Hwang, S. (2017). Quo vadis? Interferon-inducible GTPases go to their target membranes via the LC3-conjugation system of autophagy. Small GTPases 8, 199–207. doi: 10.1080/21541248.2016.1213090
Choi, J., Park, S., Biering, S. B., Selleck, E., Liu, C. Y., Zhang, X., et al. (2014). The parasitophorous vacuole membrane of Toxoplasma gondii is targeted for disruption by ubiquitin-like conjugation systems of autophagy. Immunity 40, 924–935. doi: 10.1016/j.immuni.2014.05.006
Choi, A. M., Ryter, S. W., and Levine, B. (2013). Autophagy in human health and disease. N. Engl. J. Med. 368, 1845–1846. doi: 10.1056/NEJMc1303158
Chu, J. Q., Jing, K. P., Gao, X., Li, P., Huang, R., Niu, Y. R., et al. (2017). Toxoplasma gondii induces autophagy and apoptosis in human umbilical cord mesenchymal stem cells via downregulation of Mcl-1. Cell Cycle 16, 477–486. doi: 10.1080/15384101.2017.1281484
Clough, B., Wright, J. D., Pereira, P. M., Hirst, E. M., Johnston, A. C., Henriques, R., et al. (2016). K63-linked ubiquitination targets Toxoplasma gondii for endo-lysosomal destruction in IFNgamma-stimulated human cells. PLoS Pathog. 12:e1006027. doi: 10.1371/journal.ppat.1006027
Coppens, I. (2017). How Toxoplasma and malaria parasites defy first, then exploit host autophagic and endocytic pathways for growth. Curr. Opin. Microbiol. 40, 32–39. doi: 10.1016/j.mib.2017.10.009
Darde, M. L., Riahi, H., Bouteille, B., and Pestre-Alexandre, M. (1992). Isoenzyme analysis of Hammondia hammondi and Toxoplasma gondii sporozoites. J. Parasitol. 78, 731–734.
Deretic, V. (2011). Autophagy in immunity and cell-autonomous defense against intracellular microbes. Immunol. Rev. 240, 92–104. doi: 10.1111/j.1600-065X.2010.00995.x
Feng, C. G., Zheng, L., Jankovic, D., Bafica, A., Cannons, J. L., Watford, W. T., et al. (2008). The immunity-related GTPase Irgm1 promotes the expansion of activated CD4+ T cell populations by preventing interferon-gamma-induced cell death. Nat. Immunol. 9, 1279–1287. doi: 10.1038/ni.1653
Fleckenstein, M. C., Reese, M. L., Konen-Waisman, S., Boothroyd, J. C., Howard, J. C., and Steinfeldt, T. (2012). A Toxoplasma gondii pseudokinase inhibits host IRG resistance proteins. PLoS Biol. 10:e1001358. doi: 10.1371/journal.pbio.1001358
Galluzzi, L., Baehrecke, E. H., Ballabio, A., Boya, P., Bravo-San Pedro, J. M., Cecconi, F., et al. (2017). Molecular definitions of autophagy and related processes. EMBO J. 36, 1811–1836. doi: 10.15252/embj.201796697
Haldar, A. K., Foltz, C., Finethy, R., Piro, A. S., Feeley, E. M., Pilla-Moffett, D. M., et al. (2015). Ubiquitin systems mark pathogen-containing vacuoles as targets for host defense by guanylate binding proteins. Proc. Natl. Acad. Sci. U. S. A. 112, E5628–E5637. doi: 10.1073/pnas.1515966112
Haldar, A. K., Piro, A. S., Pilla, D. M., Yamamoto, M., and Coers, J. (2014). The E2-like conjugation enzyme Atg3 promotes binding of IRG and Gbp proteins to chlamydia- and Toxoplasma-containing vacuoles and host resistance. PLoS One 9:e86684. doi: 10.1371/journal.pone.0086684
Halonen, S. K., and Weiss, L. M. (2013). Toxoplasmosis. Handb. Clin. Neurol. 114, 125–145. doi: 10.1016/B978-0-444-53490-3.00008-X
Hampton, M. M. (2015). Congenital toxoplasmosis: a review. Neonatal Netw. 34, 274–278. doi: 10.1891/0730-0832.34.5.274
Hermanns, T., Muller, U. B., Konen-Waisman, S., Howard, J. C., and Steinfeldt, T. (2016). The Toxoplasma gondii rhoptry protein ROP18 is an Irga6-specific kinase and regulated by the dense granule protein GRA7. Cell. Microbiol. 18, 244–259. doi: 10.1111/cmi.12499
Howe, D. K., Honore, S., Derouin, F., and Sibley, L. D. (1997). Determination of genotypes of Toxoplasma gondii strains isolated from patients with toxoplasmosis. J. Clin. Microbiol. 35, 1411–1414. doi: 10.1128/JCM.35.6.1411-1414.1997
Howe, D. K., and Sibley, L. D. (1995). Toxoplasma gondii comprises three clonal lineages: correlation of parasite genotype with human disease. J. Infect. Dis. 172, 1561–1566. doi: 10.1093/infdis/172.6.1561
Huang, J., and Brumell, J. H. (2014). Bacteria-autophagy interplay: a battle for survival. Nat. Rev. Microbiol. 12, 101–114. doi: 10.1038/nrmicro3160
Hwang, S., Maloney, N. S., Bruinsma, M. W., Goel, G., Duan, E., Zhang, L., et al. (2012). Nondegradative role of Atg5-Atg12/Atg16L1 autophagy protein complex in antiviral activity of interferon gamma. Cell Host Microbe 11, 397–409. doi: 10.1016/j.chom.2012.03.002
Khaminets, A., Hunn, J. P., Konen-Waisman, S., Zhao, Y. O., Preukschat, D., Coers, J., et al. (2010). Coordinated loading of IRG resistance GTPases on to the Toxoplasma gondii parasitophorous vacuole. Cell. Microbiol. 12, 939–961. doi: 10.1111/j.1462-5822.2010.01443.x
Kihara, A., Kabeya, Y., Ohsumi, Y., and Yoshimori, T. (2001). Beclin-phosphatidylinositol 3-kinase complex functions at the trans-Golgi network. EMBO Rep. 2, 330–335. doi: 10.1093/embo-reports/kve061
Krishnamurthy, S., Konstantinou, E. K., Young, L. H., Gold, D. A., and Saeij, J. P. (2017). The human immune response to Toxoplasma: autophagy versus cell death. PLoS Pathog. 13:e1006176. doi: 10.1371/journal.ppat.1006176
Lee, J., Choi, J. W., Han, H. Y., Kim, W. S., Song, H. Y., Byun, E. B., et al. (2020). 4-hydroxybenzaldehyde restricts the intracellular growth of Toxoplasma gondii by inducing SIRT1-mediated autophagy in macrophages. Korean J. Parasitol. 58, 7–14. doi: 10.3347/kjp.2020.58.1.7
Lee, Y., Sasai, M., Ma, J. S., Sakaguchi, N., Ohshima, J., Bando, H., et al. (2015). p62 plays a specific role in interferon-gamma-induced presentation of a Toxoplasma vacuolar antigen. Cell Rep. 13, 223–233. doi: 10.1016/j.celrep.2015.09.005
Lei, T., Wang, H., Liu, J., Nan, H., and Liu, Q. (2014). ROP18 is a key factor responsible for virulence difference between Toxoplasma gondii and Neospora caninum. PLoS One 9:e99744. doi: 10.1371/journal.pone.0099744
Levine, B., and Kroemer, G. (2008). Autophagy in the pathogenesis of disease. Cell 132, 27–42. doi: 10.1016/j.cell.2007.12.018
Li, X., Chen, D., Hua, Q., Wan, Y., Zheng, L., Liu, Y., et al. (2016). Induction of autophagy interferes the tachyzoite to bradyzoite transformation of Toxoplasma gondii. Parasitology 143, 639–645. doi: 10.1017/S0031182015001985
Li, M., Mo, X. W., Wang, L., Chen, H., Luo, Q. L., Wen, H. Q., et al. (2014). Phylogeny and virulence divergency analyses of Toxoplasma gondii isolates from China. Parasit. Vectors 7:133. doi: 10.1186/1756-3305-7-133
Ling, Y. M., Shaw, M. H., Ayala, C., Coppens, I., Taylor, G. A., Ferguson, D. J., et al. (2006). Vacuolar and plasma membrane stripping and autophagic elimination of Toxoplasma gondii in primed effector macrophages. J. Exp. Med. 203, 2063–2071. doi: 10.1084/jem.20061318
Liu, E., Lopez Corcino, Y., Portillo, J. A., Miao, Y., and Subauste, C. S. (2016). Identification of signaling pathways by which CD40 stimulates autophagy and antimicrobial activity against Toxoplasma gondii in macrophages. Infect. Immun. 84, 2616–2626. doi: 10.1128/IAI.00101-16
Lopez Corcino, Y., Gonzalez Ferrer, S., Mantilla, L. E., Trikeriotis, S., Yu, J. S., Kim, S., et al. (2019). Toxoplasma gondii induces prolonged host epidermal growth factor receptor signalling to prevent parasite elimination by autophagy: perspectives for in vivo control of the parasite. Cell. Microbiol. 21:e13084. doi: 10.1111/cmi.13084
Lyons, R. E., McLeod, R., and Roberts, C. W. (2002). Toxoplasma gondii tachyzoite-bradyzoite interconversion. Trends Parasitol. 18, 198–201. doi: 10.1016/s1471-4922(02)02248-1
Ma, J., Becker, C., Lowell, C. A., and Underhill, D. M. (2012). Dectin-1-triggered recruitment of light chain 3 protein to phagosomes facilitates major histocompatibility complex class II presentation of fungal-derived antigens. J. Biol. Chem. 287, 34149–34156. doi: 10.1074/jbc.M112.382812
Machacova, T., Ajzenberg, D., Zakovska, A., Sedlak, K., and Bartova, E. (2016). Toxoplasma gondii and Neospora caninum in wild small mammals: seroprevalence, DNA detection and genotyping. Vet. Parasitol. 223, 88–90. doi: 10.1016/j.vetpar.2016.04.018
Martens, S., Parvanova, I., Zerrahn, J., Griffiths, G., Schell, G., Reichmann, G., et al. (2005). Disruption of Toxoplasma gondii parasitophorous vacuoles by the mouse p47-resistance GTPases. PLoS Pathog. 1:e24. doi: 10.1371/journal.ppat.0010024
Martinez, J., Malireddi, R. K., Lu, Q., Cunha, L. D., Pelletier, S., Gingras, S., et al. (2015). Molecular characterization of LC3-associated phagocytosis reveals distinct roles for Rubicon, NOX2 and autophagy proteins. Nat. Cell Biol. 17, 893–906. doi: 10.1038/ncb3192
Melzer, T., Duffy, A., Weiss, L. M., and Halonen, S. K. (2008). The gamma interferon (IFN-gamma)-inducible GTP-binding protein IGTP is necessary for Toxoplasma vacuolar disruption and induces parasite egression in IFN-gamma-stimulated astrocytes. Infect. Immun. 76, 4883–4894. doi: 10.1128/IAI.01288-07
Mizushima, N., Yoshimori, T., and Ohsumi, Y. (2011). The role of Atg proteins in autophagosome formation. Annu. Rev. Cell Dev. Biol. 27, 107–132. doi: 10.1146/annurev-cellbio-092910-154005
Montoya, J. G., and Liesenfeld, O. (2004). Toxoplasmosis. Lancet 363, 1965–1976. doi: 10.1016/s0140-6736(04)16412-x
Montoya, J. G., and Remington, J. S. (1996). Toxoplasmic chorioretinitis in the setting of acute acquired toxoplasmosis. Clin. Infect. Dis. 23, 277–282. doi: 10.1093/clinids/23.2.277
Muniz-Feliciano, L., Van Grol, J., Portillo, J. A., Liew, L., Liu, B., Carlin, C. R., et al. (2013). Toxoplasma gondii-induced activation of EGFR prevents autophagy protein-mediated killing of the parasite. PLoS Pathog. 9:e1003809. doi: 10.1371/journal.ppat.1003809
Nakatogawa, H., Suzuki, K., Kamada, Y., and Ohsumi, Y. (2009). Dynamics and diversity in autophagy mechanisms: lessons from yeast. Nat. Rev. Mol. Cell Biol. 10, 458–467. doi: 10.1038/nrm2708
Niedelman, W., Sprokholt, J. K., Clough, B., Frickel, E. M., and Saeij, J. P. (2013). Cell death of gamma interferon-stimulated human fibroblasts upon Toxoplasma gondii infection induces early parasite egress and limits parasite replication. Infect. Immun. 81, 4341–4349. doi: 10.1128/iai.00416-13
Ohshima, J., Lee, Y., Sasai, M., Saitoh, T., Su Ma, J., Kamiyama, N., et al. (2014). Role of mouse and human autophagy proteins in IFN-gamma-induced cell-autonomous responses against Toxoplasma gondii. J. Immunol. 192, 3328–3335. doi: 10.4049/jimmunol.1302822
Ohsumi, Y. (2001). Molecular dissection of autophagy: two ubiquitin-like systems. Nat. Rev. Mol. Cell Biol. 2, 211–216. doi: 10.1038/35056522
Orlofsky, A. (2009). Toxoplasma-induced autophagy: a window into nutritional futile cycles in mammalian cells? Autophagy 5, 404–406. doi: 10.4161/auto.5.3.7807
Park, S., Choi, J., Biering, S. B., Dominici, E., Williams, L. E., and Hwang, S. (2016). Targeting by autophaGy proteins (TAG): targeting of IFNG-inducible GTPases to membranes by the LC3 conjugation system of autophagy. Autophagy 12, 1153–1167. doi: 10.1080/15548627.2016.1178447
Parlog, A., Harsan, L. A., Zagrebelsky, M., Weller, M., von Elverfeldt, D., Mawrin, C., et al. (2014). Chronic murine toxoplasmosis is defined by subtle changes in neuronal connectivity. Dis. Model. Mech. 7, 459–469. doi: 10.1242/dmm.014183
Parzych, K. R., and Klionsky, D. J. (2014). An overview of autophagy: morphology, mechanism, and regulation. Antioxid. Redox Signal. 20, 460–473. doi: 10.1089/ars.2013.5371
Paulus, G. L., and Xavier, R. J. (2015). Autophagy and checkpoints for intracellular pathogen defense. Curr. Opin. Gastroenterol. 31, 14–23. doi: 10.1097/MOG.0000000000000134
Portillo, J. C., Muniz-Feliciano, L., Lopez Corcino, Y., Lee, S. J., Van Grol, J., Parsons, S. J., et al. (2017). Toxoplasma gondii induces FAK-Src-STAT3 signaling during infection of host cells that prevents parasite targeting by autophagy. PLoS Pathog. 13:e1006671. doi: 10.1371/journal.ppat.1006671
Portillo, J. A., Okenka, G., Reed, E., Subauste, A., Van Grol, J., Gentil, K., et al. (2010). The CD40-autophagy pathway is needed for host protection despite IFN-gamma-dependent immunity and CD40 induces autophagy via control of P21 levels. PLoS One 5:e14472. doi: 10.1371/journal.pone.0014472
Reese, M. L., Shah, N., and Boothroyd, J. C. (2014). The Toxoplasma pseudokinase ROP5 is an allosteric inhibitor of the immunity-related GTPases. J. Biol. Chem. 289, 27849–27858. doi: 10.1074/jbc.M114.567057
Reese, M. L., Zeiner, G. M., Saeij, J. P., Boothroyd, J. C., and Boyle, J. P. (2011). Polymorphic family of injected pseudokinases is paramount in Toxoplasma virulence. Proc. Natl. Acad. Sci. U. S. A. 108, 9625–9630. doi: 10.1073/pnas.1015980108
Russell, R. C., Tian, Y., Yuan, H., Park, H. W., Chang, Y. Y., Kim, J., et al. (2013). ULK1 induces autophagy by phosphorylating Beclin-1 and activating VPS34 lipid kinase. Nat. Cell Biol. 15, 741–750. doi: 10.1038/ncb2757
Saeij, J. P., and Frickel, E. M. (2017). Exposing Toxoplasma gondii hiding inside the vacuole: a role for GBPs, autophagy and host cell death. Curr. Opin. Microbiol. 40, 72–80. doi: 10.1016/j.mib.2017.10.021
Sasai, M., Sakaguchi, N., Ma, J. S., Nakamura, S., Kawabata, T., Bando, H., et al. (2017). Essential role for GABARAP autophagy proteins in interferon-inducible GTPase-mediated host defense. Nat. Immunol. 18, 899–910. doi: 10.1038/ni.3767
Selleck, E. M., Orchard, R. C., Lassen, K. G., Beatty, W. L., Xavier, R. J., Levine, B., et al. (2015). A noncanonical autophagy pathway restricts Toxoplasma gondii growth in a strain-specific manner in IFN-gamma-activated human cells. MBio 6, e01157–e01115. doi: 10.1128/mBio.01157-15
Steele, S., Brunton, J., and Kawula, T. (2015). The role of autophagy in intracellular pathogen nutrient acquisition. Front. Cell. Infect. Microbiol. 5:51. doi: 10.3389/fcimb.2015.00051
Subauste, C. S. (2009). CD40, autophagy and Toxoplasma gondii. Mem. Inst. Oswaldo Cruz 104, 267–272. doi: 10.1590/s0074-02762009000200020
Subauste, C. S. (2019). Interplay between Toxoplasma gondii, autophagy, and autophagy proteins. Front. Cell. Infect. Microbiol. 9:139. doi: 10.3389/fcimb.2019.00139
Subauste, C. S., Andrade, R. M., and Wessendarp, M. (2007a). CD40-TRAF6 and autophagy-dependent anti-microbial activity in macrophages. Autophagy 3, 245–248. doi: 10.4161/auto.3717
Subauste, C. S., Subauste, A., and Wessendarp, M. (2007b). Role of CD40-dependent down-regulation of CD154 in impaired induction of CD154 in CD4+ T cells from HIV-1-infected patients. J. Immunol. 178, 1645–1653. doi: 10.4049/jimmunol.178.3.1645
Taylor, S., Barragan, A., Su, C., Fux, B., Fentress, S. J., Tang, K., et al. (2006). A secreted serine-threonine kinase determines virulence in the eukaryotic pathogen Toxoplasma gondii. Science 314, 1776–1780. doi: 10.1126/science.1133643
Ueyama, T., Nakakita, J., Nakamura, T., Kobayashi, T., Kobayashi, T., Son, J., et al. (2011). Cooperation of p40phox with p47phox for Nox2-based NADPH oxidase activation during Fcγ receptor (FcγR)-mediated phagocytosis mechanism for acquisition of p40phox phosphatidylinositol 3-phosphate (PI (3) P) binding. J. Biol. Chem. 286, 40693–40705. doi: 10.1074/jbc.M111.237289
Van Grol, J., Muniz-Feliciano, L., Portillo, J. A., Bonilha, V. L., and Subauste, C. S. (2013). CD40 induces anti-Toxoplasma gondii activity in nonhematopoietic cells dependent on autophagy proteins. Infect. Immun. 81, 2002–2011. doi: 10.1128/IAI.01145-12
Wacker, R., Eickel, N., Schmuckli-Maurer, J., Annoura, T., Niklaus, L., Khan, S. M., et al. (2017). LC3-association with the parasitophorous vacuole membrane of Plasmodium berghei liver stages follows a noncanonical autophagy pathway. Cell. Microbiol. 19:e12754. doi: 10.1111/cmi.12754
Wang, L., Chen, H., Liu, D., Huo, X., Gao, J., Song, X., et al. (2013). Genotypes and mouse virulence of Toxoplasma gondii isolates from animals and humans in China. PLoS One 8:e53483. doi: 10.1371/journal.pone.0053483
Wang, Y., Weiss, L. M., and Orlofsky, A. (2009). Host cell autophagy is induced by Toxoplasma gondii and contributes to parasite growth. J. Biol. Chem. 284, 1694–1701. doi: 10.1074/jbc.M807890200
Whitmarsh, R. J., and Hunter, C. A. (2008). Digest this! A role for autophagy in controlling pathogens. Cell Host Microbe 4, 413–414. doi: 10.1016/j.chom.2008.10.008
Yu, Y., Zhao, N., An, J., and Zhang, X. (2017). CCAAT/enhancer-binding protein beta mediates the killing of Toxoplasma gondii by inducing autophagy in nonhematopoietic cells. DNA Cell Biol. 36, 212–218. doi: 10.1089/dna.2016.3434
Zhao, Y., Ferguson, D. J., Wilson, D. C., Howard, J. C., Sibley, L. D., and Yap, G. S. (2009). Virulent Toxoplasma gondii evade immunity-related GTPase-mediated parasite vacuole disruption within primed macrophages. J. Immunol. 182, 3775–3781. doi: 10.4049/jimmunol.0804190
Zhao, Z., Fux, B., Goodwin, M., Dunay, I. R., Strong, D., Miller, B. C., et al. (2008). Autophagosome-independent essential function for the autophagy protein Atg5 in cellular immunity to intracellular pathogens. Cell Host Microbe 4, 458–469. doi: 10.1016/j.chom.2008.10.003
Zhao, Y., Wilson, D., Matthews, S., and Yap, G. S. (2007). Rapid elimination of Toxoplasma gondii by gamma interferon-primed mouse macrophages is independent of CD40 signaling. Infect. Immun. 75, 4799–4803. doi: 10.1128/IAI.00738-07
Keywords: Toxoplasma gondii, autophagy, xenophagy, LC3-associated phagocytosis, IFN-γ mediated pathogen elimination
Citation: Wu M, Cudjoe O, Shen J, Chen Y and Du J (2020) The Host Autophagy During Toxoplasma Infection. Front. Microbiol. 11:589604. doi: 10.3389/fmicb.2020.589604
Edited by:
Lihua Xiao, South China Agricultural University, ChinaReviewed by:
Carlos Subauste, Case Western Reserve University, United StatesVern B. Carruthers, University of Michigan, United States
Copyright © 2020 Wu, Cudjoe, Shen, Chen and Du. This is an open-access article distributed under the terms of the Creative Commons Attribution License (CC BY). The use, distribution or reproduction in other forums is permitted, provided the original author(s) and the copyright owner(s) are credited and that the original publication in this journal is cited, in accordance with accepted academic practice. No use, distribution or reproduction is permitted which does not comply with these terms.
*Correspondence: Ying Chen, Y3kuanVpY2VAYWxpeXVuLmNvbQ==; Jian Du, ZHVqYW5lQDE2My5jb20=
†These authors have contributed equally to this work