- 1Indian Institute of Science Education and Research, Pune, India
- 2INRAE, UMR 1332, Biologie du Fruit et Pathologie, University of Bordeaux, Bordeaux, France
Spiroplasmas are cell-wall-deficient helical bacteria belonging to the class Mollicutes. Their ability to maintain a helical shape in the absence of cell wall and their motility in the absence of external appendages have attracted attention from the scientific community for a long time. In this review we compare and contrast motility, shape determination and cytokinesis mechanisms of Spiroplasma with those of other Mollicutes and cell-walled bacteria. The current models for rod-shape determination and cytokinesis in cell-walled bacteria propose a prominent role for the cell wall synthesis machinery. These models also involve the cooperation of the actin-like protein MreB and FtsZ, the bacterial homolog of tubulin. However the exact role of the cytoskeletal proteins is still under much debate. Spiroplasma possess MreBs, exhibit a rod-shape dependent helical morphology, and divide by an FtsZ-dependent mechanism. Hence, spiroplasmas represent model organisms for deciphering the roles of MreBs and FtsZ in fundamental mechanisms of non-spherical shape determination and cytokinesis in bacteria, in the absence of a cell wall. Identification of components implicated in these processes and deciphering their functions would require genetic experiments. Challenges in genetic manipulations in spiroplasmas are a major bottleneck in understanding their biology. We discuss advancements in genome sequencing, gene editing technologies, super-resolution microscopy and electron cryomicroscopy and tomography, which can be employed for addressing long-standing questions related to Spiroplasma biology.
Introduction
Spiroplasmas are members of the class Mollicutes (cell-wall-deficient bacteria) and are characterized by their helical cell shape (Razin et al., 1973). They were discovered as mycoplasma-like helical organisms associated with citrus stubborn and corn stunt diseases (Granados et al., 1968; Laflèche and Bové, 1970; Davis and Worley, 1973). Over the years, many species of Spiroplasma have been isolated from a wide variety of hosts, mainly arthropods and plants (Clark et al., 1985; Saillard et al., 1987; Regassa and Gasparich, 2006), and have also been shown to be able to infect humans (Aquilino et al., 2015; Etienne et al., 2018). Spiroplasma cells exhibit chemotaxis (Daniels et al., 1980) and move by kinking motility (Shaevitz et al., 2005). The motility characteristics and distinct shape of the Spiroplasma cells make it an interesting model system to study the cell-wall independent mechanisms of cell division, shape determination and motility in bacteria.
Bacterial morphogenesis and motility have attracted considerable attention from researchers for many years. Indeed these processes play a vital role in survival and adaptation to environment, nutrient acquisition, division or host colonization (Yang et al., 2016; Raina et al., 2019). Major advances related to understanding shape determination and cell division have been made using cell-walled bacteria as models. These contain a peptidoglycan cell wall and include cocci (Figure 1A) and rod-shaped bacteria (Figure 1B). In rod-shaped bacteria, MreB and its isoforms (Figure 1B) are essential for guiding peptidoglycan synthesis machinery to distribute growth along the longitudinal surface (Jones et al., 2001; van den Ent et al., 2001; Carballido-López, 2006). Some rod-shaped bacteria without MreB maintain their rod-shape by polar growth (Figure 1C). Further, some bacteria have the ability to deviate from uniform growth of cell wall to attain modification in their rod shape, e.g., the crescent shape in Caulobacter crescentus (Figure 1D) and helical shape in Helicobacter pylori (Cabeen and Jacobs-wagner, 2005).
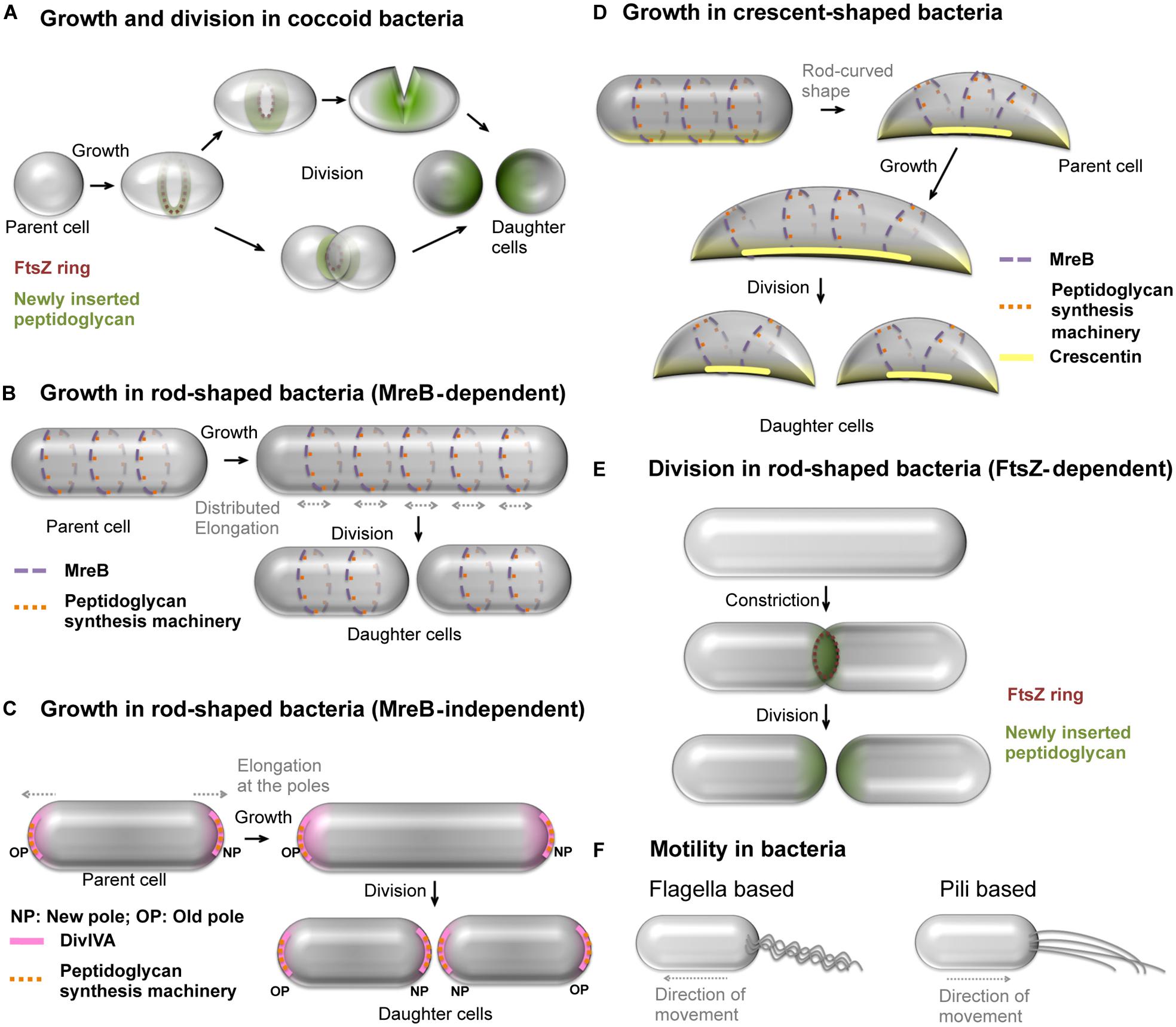
Figure 1. Growth, cell division and motility mechanisms in bacteria. (A) Spherical, cell-walled bacteria grow and divide by insertion of peptidoglycan in FtsZ-dependent manner. Alternatively, some spherical bacteria divide by constriction. (B) Some of the rod-shaped, cell-walled bacteria such as E. coli grow uniformly along the length by uniform insertion of peptidoglycan facilitated by MreB patches and divide to produce daughter cells. The dark blue and light blue colors represent patches of MreB, and associated peptidoglycan synthesis machinery shown as red and pink dots, on the membrane at the front and back of the cell, respectively. (C) M. smegmatis, a cell-walled bacterium exhibits MreB-independent polar growth by positioning the peptidoglycan synthesis machinery (orange dots) with the help of DivIVA (pink) at the cell poles. (D) Caulobacter crescentus attains crescent shape by asymmetric growth using crescentin polymers (yellow line) that prevent peptidoglycan insertion at the site of their location. The crescentin polymers are positioned by MreB and the former prevents insertion of new peptidoglycan in its vicinity. The MreB patches present at locations away from crescentin facilitate cell growth, thus leading to crescent shape. (E) The most predominant mechanism of cell division by formation and constriction of FtsZ ring at the mid-cell region in cell-walled bacteria. The FtsZ-assisted insertion of peptidoglycan at the mid-cell region results into septum closure and separation of daughter cells. (F) Well studied motility mechanisms using appendages in cell-walled bacteria include the (i) flagella dependent swimming and (ii) type IV pili-based twitching motility. See Miyata et al. (2020) for a detailed review on motility mechanisms in bacteria.
In cell-walled bacteria, FtsZ, a tubulin homolog, supports peptidoglycan insertion at the septum during cell division (Figures 1A,E). The positioning of FtsZ in a ring-like arrangement (Z-ring) at the mid-cell region is a crucial step in cell division. The Z-ring formed by multiple overlapping filaments is attached to the cell membrane through adapter proteins such as FtsA and/or ZipA (Hale and De Boer, 1997; Pichoff and Lutkenhaus, 2002). Recent results show that the division of cell-walled bacteria appears to be dependent on the assembly dynamics of FtsZ that guides septum synthesis (Bisson-Filho et al., 2017). However, mechanistic studies carried out on cell-walled bacteria may complicate the decoupling of the respective roles of the peptidoglycan and cytoskeletal proteins in both shape determination and cell division processes.
Spiroplasmas have a helical shape, distinct from those of most Mollicutes which are pleomorphic. Spiroplasmas possess the cytoskeletal proteins associated with rod-shape determination and cell division such as MreB, FtsZ, and FtsA. Hence, spiroplasmas serve as a cell-wall-less model system that possess essential cell division and shape determination machinery. However, the paucity of easy genetic modification tools for spiroplasmas has long hampered understanding of the mechanisms of morphogenesis and motility. The advent of genetic modification tools (Renaudin et al., 2014; Terahara et al., 2017), the increase in number of available genome sequences1 and improved imaging techniques open up avenues for understanding the molecular basis of morphology and cell division processes in bacteria using the Spiroplasma cell as a model system.
In this review, we compare and contrast information available on spiroplasmas with that on mycoplasmas (other Mollicutes which naturally lack a cell-wall), and cell-walled bacteria. Based on the comparison we bring out the open questions in understanding motility, shape determination and cell division of Spiroplasma cells. Further, we discuss tools and techniques available for understanding Spiroplasma biology and strategies for future developments.
Motility and Cell Division in Genus Mycoplasma
Motility in Mycoplasma Species
Motility mechanisms in bacteria typically involve appendages such as flagella and pili (Figure 1F; Miyata et al., 2020). Various motility mechanisms and components exist across species of Mycoplasma (Miyata, 2010; Balish, 2014; Garcıa-Morales et al., 2016; Miyata and Hamaguchi, 2016), especially well characterized in M. mobile, M. pneumoniae, and M. genitalium. All described mechanisms involve a multi-protein complex, termed attachment organelle (AO), localized at the cell neck in M. mobile (Uenoyama and Miyata, 2005) or at the leading pole in M. genitalium and M. pneumoniae (Balish et al., 2003; Hasselbring and Krause, 2007). The AO proteins of M. genitalium and M. pneumoniae are homologous to each other (Hasselbring et al., 2006). However the AO proteins of M. mobile and M. pneumoniae are not homologous (Balish, 2014), despite the phylogenetic proximity of these two Mycoplasma species. A characteristic of bacterial motility is chemotaxis driven by two-component systems. Chemotaxis has been reported in some Mycoplasma species (Kirchhoff et al., 1987), but genes encoding putative two-component systems have not been identified in Mycoplasma genomes yet.
Cell Division in Mycoplasma Species
Most mycoplasmas are pleiomorphic and do not encode mreB genes. Gene ftsZ is found in most but not all Mycoplasma genomes (Zhao et al., 2004; Lluch-Senar et al., 2010), suggesting that it may not be essential in some mycoplasmas. For instance, upon deletion of ftsZ gene, M. genitalium divides by a mechanism that involves motility machinery (Lluch-Senar et al., 2010). In M. pneumoniae, AO has been shown to be tightly linked to cell division. Partnering of FtsZ and motility machinery might be required for efficient division in motile mycoplasmas harboring an ftsZ gene (Balish, 2014). According to this model, FtsZ constricts the cell membrane and motility pulls the cells apart.
Spiroplasma Physiology
Cell Morphology
The elongated morphology of Spiroplasma cells ensures a large surface for nutrient import, and helical shape is well adapted for motility in semi-viscous media, a natural environment of spiroplasmas (arthropods’ fluids, plant phloem). Capacity to lose helical morphology appears also essential for some species because, upon entry into host cells, a drastic morphological change into coccoid shapes can occur (Fletcher et al., 1998; Duret et al., 2014).
Fibril (Williamson, 1974), a cytoskeletal protein (Williamson et al., 1991) with a molecular mass of about 59 kilodalton and unique to spiroplasmas is thought to be responsible for helical shape of spiroplasmas (Razin, 1978; Townsend and Archer, 1983). In vivo, fibril forms a ribbon of filaments (Charbonneau and Ghiorse, 1984; Schmitt et al., 1984; Townsend and Plaskitt, 1985). A flat ribbon bound at the cytoplasmic side of the cell membrane that spans the entire cell body has been visualized using electron microscopy (Figure 2A; Trachtenberg and Gilad, 2001). The ribbon links the two poles along the shortest helical path. Visualization of Spiroplasma cytoskeleton by electron cryotomography (ECT) revealed that filaments of fibril are indeed present close to the cell membrane (Kürner et al., 2005; Trachtenberg et al., 2008). Diffraction analysis of isolated fibrils suggested that conformational switches of the fibril could determine cell shape and helicity by varying the diameter of fibril tetramers in the ribbon (Trachtenberg and Gilad, 2001; Cohen-Krausz et al., 2011). However, this hypothesis will have to be experimentally assessed.
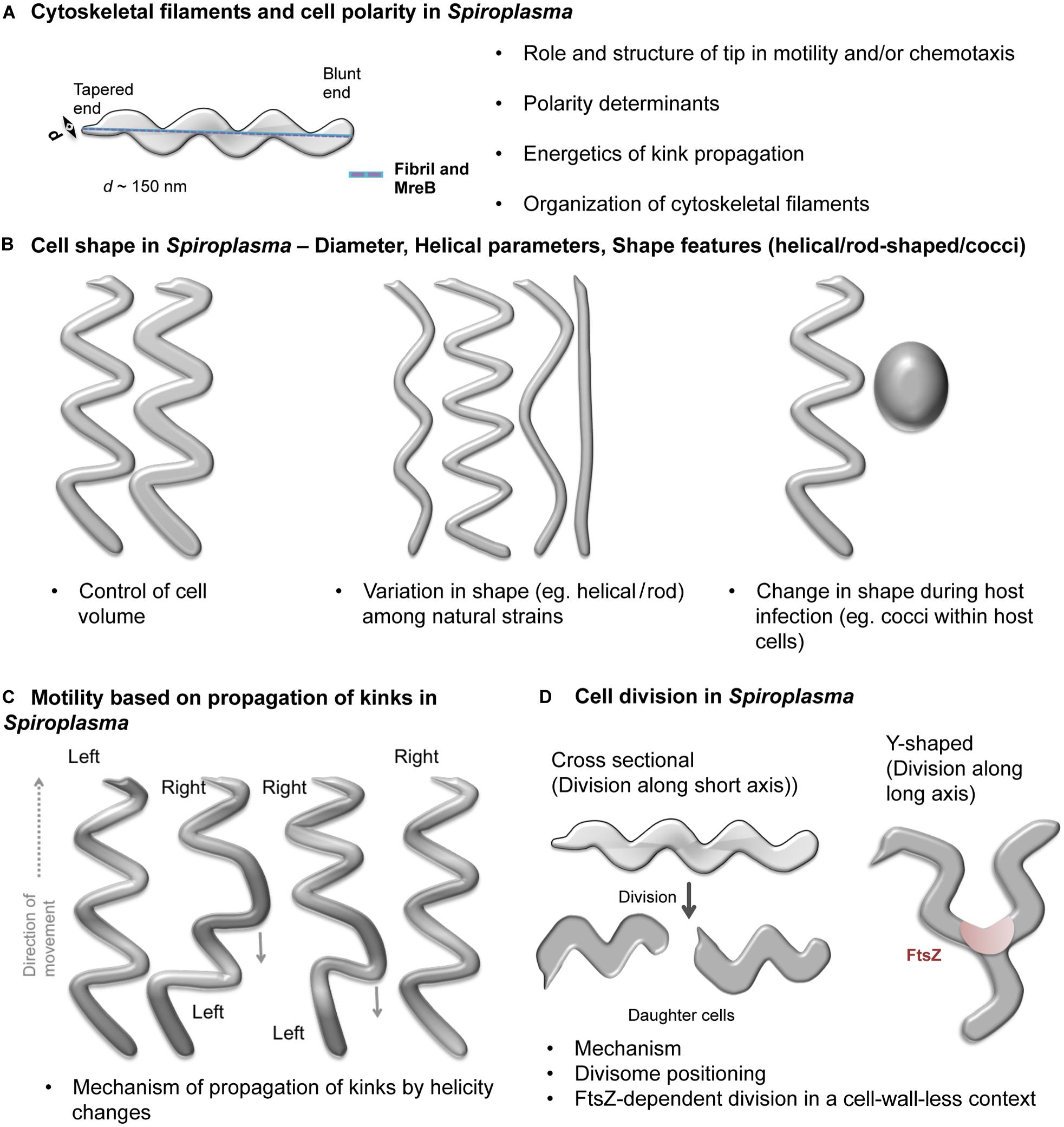
Figure 2. Schematic representations of open questions in Spiroplasma biology. Open questions in Spiroplasma biology include- (A) Organization of cytoskeletal filaments and cell polarity determinants. A Spiroplasma cell showing its characteristic helical shape, distinct poles (one is tapered while the other is blunt) and cytoskeletal ribbon (blue) connecting the two poles through the shortest path along the cell body is shown. (B) Factors conferring spherical, rod and helical shapes, determinants of helical pitch and cell lengths in Spiroplasma. (C) Kinking-based motility in Spiroplasma. The kink is introduced at one of the poles by change in handedness of the cell and traverses through the cell body. Portions of the cell on either side of the kink have opposite handedness. (D) Modes of cell division (cross sectional and Y-shaped) and factors favoring them. The bulleted text in the figures summarizes the open questions.
In addition to fibril, MreB filaments were also found to constitute the cytoskeletal ribbon of Spiroplasma cells. However, the organization of MreB and fibril filaments within the cytoskeleton is debated (Kürner et al., 2005; Trachtenberg et al., 2008). Spiroplasma species possess at least five paralogs of mreB gene (Ku et al., 2014). The functional relevance of the different MreB homologs has not yet been proven. However, the presence of 5-8 mreB genes in organisms with minimal genome suggests that these proteins may confer a selective advantage to spiroplasmas. In ECT studies on S. melliferum, the MreB filaments were identified on the basis of inter-filament spacing (Kürner et al., 2005). This information is insufficient to know if the filaments were made of one or more paralogs of MreB. It is unknown if one or more MreB paralogs act in association with fibril in Spiroplasma morphogenesis and if the MreBs perform overlapping functions (Harne et al., 2020).
Besides helicity, Spiroplasma cells also show polarity, with a tapered end (tip structure) associated to an electron dense core and a blunt or rounded end (Figure 2A; Garnier et al., 1981; Ammar et al., 2004). The tip structure seems to expose the adhesins required for attachment to cells of the vector insect for the plant pathogenic Spiroplasma species (Ammar et al., 2004; Duret et al., 2014). Another interesting feature of spiroplasmas is their diameter of about 100–150 nm, much smaller than a typical bacterial cell (Figures 2A,B). The factors determining the diameter of the cell are currently unknown.
Motility
During motility, Spiroplasma cells exhibit twitching, bending and flexing and rotation along the helical axis of the cell (Gilad et al., 2003). Spiroplasmas swim by formation and propagation of kinks (Figure 2C). Cell helicity seems to facilitate processive movement of kinks along the cell body (Shaevitz et al., 2005). Cells having lost helicity upon decrease of the transmembrane electric potential become non-motile (Béven and Wróblewski, 1997). The helical, non-motile mutant of S. citri obtained by Jacob et al. (1997) indicates that in addition to helical shape a functional scm1 gene is required for rotation about the helix axis. However the exact role of Scm1 in motility of spiroplasmas remains to be elucidated.
Fibril filaments are proposed to be responsible for Spiroplasma motility in addition to conferring helical shape (Razin, 1978). The Spiroplasma fibrils are distinct from the flagellar machinery; these filaments are intra-cellular. While fibril filaments are believed to undergo co-ordinated conformational changes to produce kinks in the cell body during motility, the role of MreBs in Spiroplasma motility is unknown. The introduction and propagation of kinks requires a flexible cell body. The absence of cell wall in spiroplasmas probably provides flexibility for enabling “kinking motility.”
High resolution video microscopy and electron microscopy studies suggested that the kinks are always introduced at the tapered end (Shaevitz et al., 2005; Liu et al., 2017). It was later shown that the kinks are preferentially initiated at the tip, but initiation can occasionally occur at the opposite pole and propagate along the whole cell body (Boudet et al., 2018). In S. eriocheiris, chemotactic activity was clearly shown to be linked to change in cell helicity and reversal of motion (Liu et al., 2017). In the absence of homologs of conventional chemotaxis proteins found in other bacteria, it is possible that the tip structure is involved in the change in helicity from front to back (Liu et al., 2017). For some Spiroplasma species, attachment to host cells occurs through the tip (Ammar et al., 2004; Duret et al., 2014) and involves binding to glycoconjugates (Duret et al., 2014). In some Mycoplasma species, the attachment organelle also binds glycosylated compounds (Kasai et al., 2015). It is thus tempting to propose roles for Spiroplasma tip in motility similar to those of AO in Mycoplasma species (Liu et al., 2017). Since different mechanisms exist in Mycoplasma genus, it is quite possible that another independent mechanism evolved in Spiroplasma.
Cell Division
Most of the Mollicutes have only 4 (mraZ, mraW, ftsA, and ftsZ) of over 15 genes coded by the division and cell wall (dcw) cluster in cell-walled bacteria (Zhao et al., 2004). Given the reduced genomes of these bacteria, this suggests that these four proteins constitute the machinery sufficient for cell division in bacteria lacking a cell wall. However, it is unknown if all these proteins are necessary for Spiroplasma cell division.
Two modes of cell division have been proposed for Spiroplasma (Figure 2D). The first mode was based on observation of S. citri growth. It proposes that Spiroplasma cells grow at one of the two poles by insertion of new membrane components and divide by one or multiple constrictions along the short axis of the cell (Garnier et al., 1981, 1984). In contrast, in S. poulsonii, another mechanism involves longitudinal fission (Y-shape) dependent on FtsZ (Ramond et al., 2016). It is unclear if both these mechanisms exist in all Spiroplasma species and if the choice between them depends on specific circumstances or environmental conditions. If Y-shaped division was the predominant mechanism, the Spiroplasma cells should have a fixed length. However, cells of varying lengths are always observed in growing cultures. This suggests that the Y-shaped division may be an alternate mode of cytokinesis occurring more or less often depending on the species. The constituent proteins of the Spiroplasma cell division machinery and mechanism governing their positioning at the appropriate site needs to be investigated.
Figure 2 summarizes the open questions in Spiroplasma biology. How they can be addressed using a combination of approaches is discussed below.
Available Tools and Future Directions
Morphology Variants, Deletion Mutants and Genome Analysis
Some of the attempts to understand gene function in Spiroplasma have been conducted through random mutagenesis using ultraviolet (UV) light (Labarère and Barroso, 1989) or by insertion of transposon (Foissac et al., 1997). These methods require extensive functional or morphological screening to identify mutants of interest. A major hurdle in preparing Spiroplasma mutants is the low recombination rate. Disruption of multiple genes simultaneously is technically very challenging (Duret et al., 2005). The limited number of antibiotics to which Spiroplasma is sensitive also makes the process difficult (Duret et al., 2005). In general, fundamental cellular processes in Spiroplasma remain poorly understood due to the above difficulties associated with genome modifications.
Nonetheless, the toolbox for genetic manipulation is continuously expanding (Renaudin et al., 2014). Moreover, the possibility to insert and modify a Mycoplasma genome in yeast and transplant it back to a recipient cell gives hope that this technology will soon be applicable to spiroplasmas. An alternate approach could be to perform vector-based expression of recombination proteins as carried out in M. hyorhinis (Ishag et al., 2017). One may also explore the possibility of standardizing CRISPR-Cas (Clustered Regularly Interspaced Short Palindromic Repeats and CRISPR associated genes) for specific gene targeting (Ipoutcha et al., 2019) in Spiroplasma. Given the uncertainties and inefficiency of currently available recombination tools in Spiroplasma, use of heterologous potent recombination machinery may facilitate its target gene disruption efficaciously.
Given the challenges in genetic manipulation, one may exploit the Spiroplasma species differing in their helicity (Figure 2B; e.g., cells of S. platyhelix are flattened, helical filaments while S. ixodetis cells are tightly helical) to understand helicity determinants or explore the naturally occurring mutants (e.g., S. citri ASP-I). The molecular basis for helicity can be studied by performing genome analysis and checking expression levels of cytoskeletal proteins. Indeed, natural species differ in their number of MreB paralogs, in cell diameter and helix pitch (Figure 2B; Regassa and Gasparich, 2006). These studies are feasible given the increasing number of Spiroplasma genomes available (NCBI database for genome assemblies contains 31 Spiroplasma genomes out of which 22 were deposited during the five last years). Naturally occurring strains of Spiroplasma with morphology variations have been isolated [e.g., Spiroplasma citri ASP-I (Townsend et al., 1977)], which can be characterized for identifying genetic defects (Harne et al., 2020). For example, strains which exhibit helical morphology (Abalain-Colloc et al., 1987; Whitcomb et al., 1997) despite a probable disruption (a stop codon) in fibril gene such as S. sabaudiense Ar-1343 (Chang T. H. et al., 2014) and S. helicoides TABS-2 (Shen et al., 2016) could provide further insights into the functionality of the fibril gene.
Imaging Technologies
Dark-field light microscopy is commonly used in order to visualize unlabeled, living spiroplasmas in liquid. Motility of single cells and kink propagation can be quantified using this technique (Boudet et al., 2018). Recently, the development of a line-scanning optical trap was applied to record shape deformation of the Spiroplasma body under various stress conditions (Koch and Rohrbach, 2012, 2014). For live cell imaging, it is essential to create gene disruption strains and complementation of the gene with a fluorescently tagged version of the protein encoded by the gene. These can provide clues about cell division process and role of cytoskeletal proteins in motility. Successful visualization of YFP-tagged protein in S. eriocheiris cells in the recent past (Terahara et al., 2017) is a promising step toward identifying intra-cellular localization of proteins. However, considering the diffraction limit of light and the small diameter of the cell (∼0.15 μm), super resolution microscopy techniques are essential for Spiroplasma imaging experiments.
In contrast, the small diameter of the cell (∼100–150 nm) makes Spiroplasma an ideal system to explore using electron cryotomography (ECT). The technique is useful for three-dimensional (3D) visualization of samples within the cell in a near-native state, and is commonly used for visualization of protein filaments, secretion systems, chemoreceptor arrays and localization of proteins as a part of protein assembly (Briegel et al., 2006, 2012; Basler et al., 2012; Chang Y. W. et al., 2014). It has also been applied to Spiroplasma to visualize arrangement of protein filaments in cytoskeleton (Kürner et al., 2005). In this context, conjugating fluorescence and electron microscopy using cryo-correlative light and electron microscopy (CLEM) should prove useful for visualization of intra-cellular localization of protein with high precision in a near-native state. Comparative CLEM studies can be performed on wild type cells, (naturally occurring or artificially generated) mutants and rescued mutants of Spiroplasma to identify localization of cytoskeletal proteins such as fibril, MreB, FtsZ.
Conclusion
Spiroplasma is a unique bacterium without a cell wall, which possesses a definite helical shape and is motile. Despite the absence of a cell wall, it utilizes the cytoskeletal elements such as MreB and FtsZ, like cell-walled bacteria, for physiological processes such as cell elongation, cell division and motility. For understanding the molecular mechanisms of these processes in biology, Spiroplasma thus qualifies to be a promising model organism.
Author Contributions
All authors contributed to the drafting and revising process and approved the final version of this manuscript.
Funding
Work in the lab of LB is supported by grants for Interdisciplinary Programs from the University of Bordeaux, and CASDAR grant 1617. Work in the lab of PG was supported by funds from Department of Science and technology (DST) INSPIRE Faculty Fellowship (IFA12/LSBM-52), Innovative Young Biotechnologist Award (BT/07/IYBA/2013), Department of Biotechnology Membrane Structural Biology Programme Grant (BT/PR28833/BRB/10/1705/2018) and IISER Pune. SH acknowledged IISER, Pune and Raman-Charpak Fellowship from CEFIPRA (IFC//4109/RCF 2017/895).
Conflict of Interest
The authors declare that the research was conducted in the absence of any commercial or financial relationships that could be construed as a potential conflict of interest.
Acknowledgments
We would like to thank the many young scientists, engineers, and technicians who have been working on Spiroplasma at UMR 1332, Biologie du Fruit et Pathologie, Villenave d’Ornon, France and at Biology division, Indian Institute of Science Education and Research, Pune, India.
Footnotes
References
Abalain-Colloc, M. L., Chastel, C., Tully, J. G., Bové, J. M., Whitcomb, R., Gilot, B., et al. (1987). Spiroplasma sabaudiense sp. nov. from mosquitoes collected in France. Int. J. Syst. Bacteriol. 37, 260–265. doi: 10.1099/00207713-37-3-260
Ammar, E., Fulton, D., Bai, X., Meulia, T., and Hogenhout, S. A. (2004). An attachment tip and pili-like structures in insect- and plant-pathogenic spiroplasmas of the class Mollicutes. Arch. Microbiol. 181, 97–105. doi: 10.1007/s00203-003-0630-8
Aquilino, A., Masiá, M., López, P., Galiana, A. J., Tovar, J., Andrés, M., et al. (2015). First human systemic infection caused by Spiroplasma. J. Clin. Microbiol. 53, 719–721. doi: 10.1128/JCM.02841-14
Balish, M. F. (2014). Mycoplasma pneumoniae, an underutilized model for bacterial cell biology. J. Bacteriol. 196, 3675–3682. doi: 10.1128/JB.01865-14
Balish, M. F., Santurri, R. T., Ricci, A. M., Lee, K. K., and Krause, D. C. (2003). Localization of Mycoplasma pneumoniae cytadherence-associated protein HMW2 by fusion with green fluorescent protein: implications for attachment organelle structure. Mol. Microbiol. 47, 49–60. doi: 10.1046/j.1365-2958.2003.03282.x
Basler, M., Pilhofer, M., Henderson, G. P., Jensen, G. J., and Mekalanos, J. J. (2012). Type VI secretion requires a dynamic contractile phage tail-like structure. Nature 483, 182–186. doi: 10.1038/nature10846
Béven, L., and Wróblewski, H. (1997). Effect of natural amphipathic peptides on viability, membrane potential, cell shape and motility of Mollicutes. Res. Microbiol. 148, 163–175. doi: 10.1016/S0923-2508(97)87647-4
Bisson-Filho, A. W., Hsu, Y. P., Squyres, G. R., Kuru, E., Wu, F., Jukes, C., et al. (2017). Treadmilling by FtsZ filaments drives peptidoglycan synthesis and bacterial cell division. Science 355, 739–743. doi: 10.1126/science.aak9973
Boudet, J. F., Mathelié-Guinlet, M., Vilquin, A., Douliez, J. P., Béven, L., and Kellay, H. (2018). Large variability in the motility of spiroplasmas in media of different viscosities. Sci. Rep. 18, 1–14. doi: 10.1038/s41598-018-35326-2
Briegel, A., Dias, D. P., Li, Z., Jensen, R. B., Frangakis, A. S., and Jensen, G. J. (2006). Multiple large filament bundles observed in Caulobacter crescentus by electron cryotomography. Mol. Microbiol. 62, 5–14. doi: 10.1111/j.1365-2958.2006.05355.x
Briegel, A., Li, X., Bilwes, A. M., Hughes, K. T., Jensen, G. J., and Crane, B. R. (2012). Bacterial chemoreceptor arrays are hexagonally packed trimers of receptor dimers networked by rings of kinase and coupling proteins. Proc. Natl. Acad. Sci. U.S.A. 109, 3766–3771. doi: 10.1073/pnas.1115719109
Cabeen, M. T., and Jacobs-wagner, C. (2005). Bacterial cell shape. Nat. Rev. Microbiol. 3, 601–610. doi: 10.1038/nrmicro1205
Carballido-López, R. (2006). Orchestrating bacterial cell morphogenesis. Mol. Microbiol. 60, 815–819. doi: 10.1111/j.1365-2958.2006.05161.x
Chang, T. H., Lo, W. S., Ku, C., Chen, L. L., and Kuo, C. H. (2014). Molecular evolution of the substrate utilization strategies and putative virulence factors in mosquito-associated Spiroplasma species. Genome Biol. Evol. 6, 500–509. doi: 10.1093/gbe/evu033
Chang, Y. W., Chen, S., Tocheva, E. I., Treuner-Lange, A., Löbach, S., Søgaard-Andersen, L., et al. (2014). Correlated cryogenic photoactivated localization microscopy and cryo-electron tomography. Nat. Methods 11, 737–739. doi: 10.1038/nmeth.2961
Charbonneau, D. L., and Ghiorse, W. C. (1984). Ultrastructure and location of cytoplasmic fibrils in Spiroplasma floricola OBMG. Curr. Microbiol. 10, 65–72. doi: 10.1007/BF01575761
Clark, T. B., Whitcomb, R. F., Tully, J. G., Mouches, C., Saillard, C., Bové, J. M., et al. (1985). Spiroplasma melliferum, a new species from the honeybee (Apis mellifera). Int. J. Syst. Bacteriol. 35, 296–308. doi: 10.1099/00207713-35-3-296
Cohen-Krausz, S., Cabahug, P. C., and Trachtenberg, S. (2011). The monomeric, tetrameric, and fibrillar organization of Fib: the dynamic building block of the bacterial linear motor of Spiroplasma melliferum BC3. J. Mol. Biol. 410, 194–213. doi: 10.1016/j.jmb.2011.04.067
Daniels, M. J., Longland, J. M., and Gilbart, J. (1980). Aspects of motility and chemotaxis in spiroplasmas. J. Gen. Microbiol. 118, 429–436. doi: 10.1099/00221287-118-2-429
Davis, R. E., and Worley, J. F. (1973). Spiroplasma: motile, helical microorganism associated with corn stunt disease. Phytopathology 63, 403–408. doi: 10.1094/Phyto-63-403
Duret, S., André, A., and Renaudin, J. (2005). Specific gene targeting in Spiroplasma citri: improved vectors and production of unmarked mutations using site-specific recombination. Microbiology 151, 2793–2803. doi: 10.1099/mic.0.28123-0
Duret, S., Batailler, B., Dubrana, M.-P., Saillard, C., Renaudin, J., Béven, L., et al. (2014). Invasion of insect cells by Spiroplasma citri involves spiralin relocalization and lectin/glycoconjugate-type interactions. Cell. Microbiol. 16, 1119–1132. doi: 10.1111/cmi.12265
Etienne, N., Bret, L., Le Brun, C., Lecuyer, H., Moraly, J., Lanternier, F., et al. (2018). Disseminated Spiroplasma apis infection in patient with agammaglobulinemia. France Emerg. Infect. Dis. 24, 2382–2384. doi: 10.3201/eid2412.180567
Fletcher, J., Wayadande, A., Melcher, U., and Ye, F. (1998). The phytopathogenic mollicute-insect vector interface: a closer look. Phytopathology 88, 1351–1358. doi: 10.1094/PHYTO.1998.88.12.1351
Foissac, X., Saillard, C., and Bové, J. M. (1997). Random insertion of transposon Tn4001 in the genome of Spiroplasma citri strain GII3. Plasmid 37, 80–86. doi: 10.1006/plas.1996.1271
Garcıa-Morales, L., González-González, L., Querol, E., and Piñol, J. (2016). A minimized motile machinery for Mycoplasma genitalium. Mol. Microbiol. 100, 125–138. doi: 10.1111/mmi.13305
Garnier, M., Clerc, M., and Bové, J. M. (1981). Growth and division of spiroplasmas: morphology of Spiroplasma citri during growth in liquid medium. J. Bacteriol. 147, 642–652. doi: 10.1128/jb.147.2.642-652.1981
Garnier, M., Clerc, M., and Bové, J. M. (1984). Growth and division of Spiroplasma citri: elongation of elementary helices. J. Bacteriol. 158, 23–28. doi: 10.1128/jb.158.1.23-28.1984
Gilad, R., Porat, A., and Trachtenberg, S. (2003). Motility modes of Spiroplasma melliferum BC3: a helical, wall-less bacterium driven by a linear motor. Mol. Microbiol. 47, 657–669. doi: 10.1046/j.1365-2958.2003.03200.x
Granados, R. R., Maramorosch, K., and Shikata, E. (1968). Mycoplasma: suspected etiologic agent of corn stunt. Proc. Natl. Acad. Sci. U.S.A. 60, 841–844. doi: 10.1073/pnas.60.3.841
Hale, C. A., and De Boer, P. A. J. (1997). Direct binding of FtsZ to ZipA, an essential component of the septal ring structure that mediates cell division in E. coli. Cell 88, 175–185. doi: 10.1016/S0092-8674(00)81838-3
Harne, S., Duret, S., Pande, V., Bapat, M., Béven, L., and Gayathri, P. (2020). MreB5 is a determinant of rod-to-helical transition in the cell-wall-less bacterium Spiroplasma. Curr. Biol. 20:93. doi: 10.1016/j.cub.2020.08.093
Hasselbring, B. M., and Krause, D. C. (2007). Proteins P24 and P41 function in the regulation of terminal-organelle development and gliding motility in Mycoplasma pneumoniae. J. Bacteriol. 189, 7442–7449. doi: 10.1128/JB.00867-07
Hasselbring, B. M., Page, C. A., Sheppard, E. S., and Krause, D. C. (2006). Transposon mutagenesis identifies genes associated with Mycoplasma pneumoniae gliding motility. J. Bacteriol. 188, 6335–6345. doi: 10.1128/JB.00698-06
Ipoutcha, T., Tsarmpopoulos, I., Talenton, V., Gaspin, C., Moisan, A., Walker, C. A., et al. (2019). Multiple origins and specific evolution of CRISPR/Cas9 systems in minimal bacteria (Mollicutes). Front. Microbiol. 10:2701. doi: 10.3389/fmicb.2019.02701
Ishag, H. Z. A., Xiong, Q., Liu, M., Feng, Z., and Shao, G. (2017). E. coli recA gene improves gene targeted homologous recombination in Mycoplasma hyorhinis. J. Microbiol. Methods 136, 49–56. doi: 10.1016/j.mimet.2017.03.004
Jacob, C., Nouzières, F., Duret, S., Bové, J. M., and Renaudin, J. (1997). Isolation, characterization, and complementation of a motility mutant of Spiroplasma citri. J. Bacteriol. 179, 4802–4810. doi: 10.1128/jb.179.15.4802-4810.1997
Jones, L. J. F., Carballido-López, R., and Errington, J. (2001). Control of cell shape in bacteria: helical, actin-like filaments in Bacillus subtilis. Cell 104, 913–922. doi: 10.1016/S0092-8674(01)00287-2
Kasai, T., Hamaguchi, T., and Miyata, M. (2015). Gliding motility of Mycoplasma mobile on uniform oligosaccharides. J. Bacteriol. 197, 2952–2957. doi: 10.1128/JB.00335-15
Kirchhoff, H., Boldt, U., Rosengarten, R., and Klein-Struckmeier, A. (1987). Chemotactic response of a gliding Mycoplasma. Curr. Microbiol. 15, 57–60. doi: 10.1007/BF01577215
Koch, M., and Rohrbach, A. (2012). Object-adapted optical trapping and shape-tracking of energy-switching helical bacteria. Nat. Photonics 6, 680–686. doi: 10.1038/nphoton.2012.232
Koch, M., and Rohrbach, A. (2014). How to calibrate an object-adapted optical trap for force sensing and interferometric shape tracking of asymmetric structures. Opt. Express 22, 25242–25257. doi: 10.1364/oe.22.025242
Ku, C., Lo, W.-S., and Kuo, C.-H. (2014). Molecular evolution of the actin-like MreB protein gene family in wall-less bacteria. Biochem. Biophys. Res. Commun. 446, 927–932. doi: 10.1016/j.bbrc.2014.03.039
Kürner, J., Frangakis, A. S., and Baumeister, W. (2005). Cryo – electron tomography reveals the cytoskeletal structure of Spiroplasma melliferum. Science 307, 436–439. doi: 10.1126/science.1104031
Labarère, J., and Barroso, G. (1989). Lethal and mutation frequency responses of Spiroplasma citri cells to UV irradiation. Mutat. Res. Fundam. Mol. Mech. Mutagen 210, 135–141. doi: 10.1016/0027-5107(89)90052-3
Laflèche, D., and Bové, J. M. (1970). Mycoplasmes dans les argumes atteints de “Greening”, de “Stubborn” ou de maladies similaires. Fruits 25, 455–465.
Liu, P., Zheng, H., Meng, Q., Terahara, N., Gu, W., Wang, S., et al. (2017). Chemotaxis without conventional two-component system, based on cell polarity and aerobic conditions in helicity-switching swimming of Spiroplasma eriocheiris. Front. Microbiol. 8:58. doi: 10.3389/fmicb.2017.00058
Lluch-Senar, M., Querol, E., and Piñol, J. (2010). Cell division in a minimal bacterium in the absence of ftsZ. Mol. Microbiol. 78, 278–289. doi: 10.1111/j.1365-2958.2010.07306.x
Miyata, M. (2010). Unique centipede mechanism of Mycoplasma gliding. Annu. Rev. Microbiol. 64, 519–537. doi: 10.1146/annurev.micro.112408.134116
Miyata, M., and Hamaguchi, T. (2016). Prospects for the gliding mechanism of Mycoplasma mobile. Curr. Opin. Microbiol. 29, 15–21. doi: 10.1016/j.mib.2015.08.010
Miyata, M., Robinson, R. C., Uyeda, T. Q. P., Fukumori, Y., Fukushima, S.-I., Haruta, S., et al. (2020). Tree of motility – A proposed history of motility systems in the tree of life. Genes Cells 25, 6–21. doi: 10.1111/gtc.12737
Pichoff, S., and Lutkenhaus, J. (2002). Unique and overlapping roles for ZipA and FtsA in septal ring assembly in Escherichia coli. EMBO J. 2, 685–693. doi: 10.1093/emboj/21.4.685
Raina, J.-B., Fernandez, V., Lambert, B., Stocker, R., and Seymour, J. R. (2019). The role of microbial motility and chemotaxis in symbiosis. Nat. Rev. Microbiol. 17, 284–294. doi: 10.1038/s41579-019-0182-9
Ramond, E., Maclachlan, C., Clerc-Rosset, S., Knott, G. W., and Lemaitre, B. (2016). Cell division by longitudinal scission in the insect endosymbiont Spiroplasma poulsonii. MBio 7, 1–5. doi: 10.1128/mBio.00881-16
Razin, S., Hasin, M., Ne’eman, Z., and Rottem, S. (1973). Isolation, chemical composition, and ultrastructural features of the cell membrane of the mycoplasma-like organism Spiroplasma citri. J. Bacteriol. 116, 1421–1435. doi: 10.1128/JB.116.3.1421-1435.1973
Regassa, L. B., and Gasparich, G. E. (2006). Spiroplasmas: evolutionary relationships and biodiversity. Front. Biosci. 11:2983–3002. doi: 10.2741/2027
Renaudin, J., Breton, M., and Citti, C. (2014). “Molecular genetic tools for Mollicutes,” in Mollicutes: Molecular Biology and Pathogenesis, eds G. Browning and C. Citti (Norfolk: Caister Academic Press), 55–76.
Saillard, C., Vignault, J. C., Bové, J. M., Raie, A., Tully, J. G., Williamson, D. L., et al. (1987). Spiroplasma phoeniceum sp. nov., a new plant-pathogenic species from Syria. Int. J. Syst. Bacteriol. 37, 106–115. doi: 10.1099/00207713-37-2-106
Schmitt, U., Petzold, H., and Marwitz, R. (1984). An in situ freeze-fracture study of Spiroplasma citri and the corn stunt Spiroplasma. Phytopathology 111, 297–304. doi: 10.1111/j.1439-0434.1984.tb00773.x
Shaevitz, J. W., Lee, J. Y., and Fletcher, D. A. (2005). Spiroplasma swim by a processive change in body helicity. Cell 122, 941–945. doi: 10.1016/j.cell.2005.07.004
Shen, W. Y., Lo, W. S., Lai, Y. C., and Kuo, C. H. (2016). Complete genome sequence of Spiroplasma helicoides TABS-2T (DSM 22551), a bacterium isolated from a horsefly (Tabanus abactor). Genome Announc. 4, e1201–e1216. doi: 10.1128/genomeA.01201-16
Terahara, N., Tulum, I., and Miyata, M. (2017). Transformation of crustacean pathogenic bacterium Spiroplasma eriocheiris and expression of yellow fluorescent protein. Biochem. Biophys. Res. Commun. 487, 488–493. doi: 10.1016/j.bbrc.2017.03.144
Townsend, R., and Archer, D. B. (1983). A Fibril protein antigen specific to Spiroplasma. J. Gen. Microbiol. 129, 199–206. doi: 10.1099/00221287-129-1-199
Townsend, R., Markham, P. G., Plaskitt, K. A., and Daniels, M. J. (1977). Isolation and characterization of a non-helical strain of Spiroplasma citri. J. Gen. Microbiol. 100, 15–21. doi: 10.1099/00221287-100-1-15
Townsend, R., and Plaskitt, K. A. (1985). Immunogold localization of p55-fibril protein and p25-spiralin in Spiroplasma cells. Microbiology 131, 983–992. doi: 10.1099/00221287-131-5-983
Trachtenberg, S., Dorward, L. M., Speransky, V. V., Jaffe, H., Andrews, S. B., and Leapman, R. D. (2008). Structure of the cytoskeleton of Spiroplasma melliferum BC3 and its interactions with the cell membrane. J. Mol. Biol. 378, 778–789. doi: 10.1016/j.jmb.2008.02.020
Trachtenberg, S., and Gilad, R. (2001). A bacterial linear motor: Cellular and molecular organization of the contractile cytoskeleton of the helical bacterium Spiroplasma melliferum BC3. Mol. Microbiol. 41, 827–848. doi: 10.1046/j.1365-2958.2001.02527.x
Uenoyama, A., and Miyata, M. (2005). Identification of a 123-kilodalton protein (Gli123) involved in machinery for gliding motility of Mycoplasma mobile. J. Bacteriol. 187, 5578–5584. doi: 10.1128/JB.187.16.5578
van den Ent, F., Amos, L. A., and Löwe, J. (2001). Prokaryotic origin of the actin cytoskeleton. Nature 413, 39–44. doi: 10.1038/35092500
Whitcomb, R. F., French, F. E., Tully, J. G., Gasparich, G. E., Rose, D. L., Carle, P., et al. (1997). Spiroplasma chrysopicola sp. nov., Spiroplasma gladiatoris sp. nov., Spiroplasma helicoides sp. nov., and Spiroplasma tabanidicola sp. nov., from Tabanid (Diptera: Tabanidae) flies. Int. J. Syst. Bacteriol. 47, 713–719. doi: 10.1099/00207713-47-3-713
Williamson, D. L. (1974). Unusual fibrils from the spirochete-like sex ratio organism. J. Bacteriol. 117, 904–906. doi: 10.1128/JB.117.2.904-906.1974
Williamson, D. L., Renaudin, J., and Bové, J. M. (1991). Nucleotide sequence of the Spiroplasma citri fibril protein gene. J. Bacteriol. 173, 4353–4362. doi: 10.1128/jb.173.14.4353-4362.1991
Yang, D. C., Blair, K. M., and Salama, N. R. (2016). Staying in shape: the impact of cell shape on bacterial survival in diverse environments. Microbiol. Mol. Biol. Rev. 80, 187–203. doi: 10.1128/MMBR.00031-15
Keywords: Spiroplasma, shape determination, motility, bacterial cytoskeleton, fibril, MreB, Spiroplasma genetics, bacterial division
Citation: Harne S, Gayathri P and Béven L (2020) Exploring Spiroplasma Biology: Opportunities and Challenges. Front. Microbiol. 11:589279. doi: 10.3389/fmicb.2020.589279
Received: 30 July 2020; Accepted: 28 September 2020;
Published: 21 October 2020.
Edited by:
Florence Tardy, Agence Nationale de Sécurité Sanitaire de l’Alimentation, de l’Environnement et du Travail (ANSES), FranceReviewed by:
Makoto Miyata, Osaka City University, JapanAharon Oren, The Hebrew University of Jerusalem, Israel
Copyright © 2020 Harne, Gayathri and Béven. This is an open-access article distributed under the terms of the Creative Commons Attribution License (CC BY). The use, distribution or reproduction in other forums is permitted, provided the original author(s) and the copyright owner(s) are credited and that the original publication in this journal is cited, in accordance with accepted academic practice. No use, distribution or reproduction is permitted which does not comply with these terms.
*Correspondence: Pananghat Gayathri, Z2F5YXRocmlAaWlzZXJwdW5lLmFjLmlu; Laure Béven, bGF1cmUuYmV2ZW5AaW5yYWUuZnI=