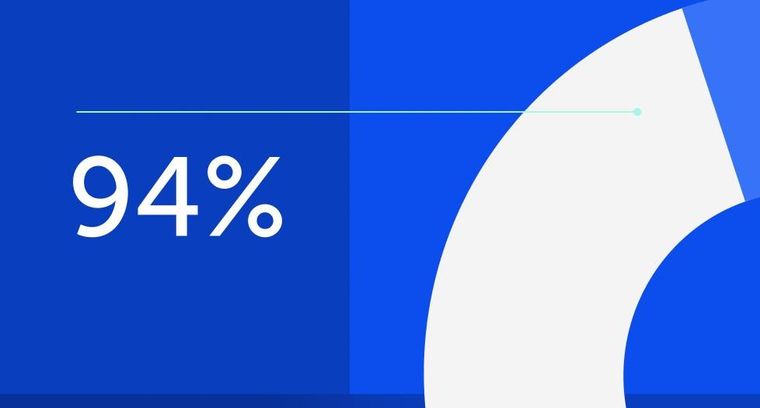
94% of researchers rate our articles as excellent or good
Learn more about the work of our research integrity team to safeguard the quality of each article we publish.
Find out more
ORIGINAL RESEARCH article
Front. Microbiol., 30 November 2020
Sec. Antimicrobials, Resistance and Chemotherapy
Volume 11 - 2020 | https://doi.org/10.3389/fmicb.2020.584603
This article is part of the Research TopicGenomic Basis of Antibiotic Resistance and Virulence in AcinetobacterView all 17 articles
Carbapenem-resistant Acinetobacter baumannii (CRAB) are emerging worldwide. In South America, clinical isolates presenting such a phenotype usually do not belong to the globally distributed international clone 2 (IC2). The majority of these isolates are also resistant to multiple other antimicrobials and are often designated extremely drug-resistant (XDR). The aim of this study was to characterize the resistance mechanisms presented by 18 carbapenem-resistant A. baumannii isolates from five different Brazilian hospitals. Species identification was determined by rpoB sequencing, and antimicrobial susceptibility was determined by broth microdilution. Isolates were submitted to whole genome sequencing using Illumina platform and genetic similarity was determined by PFGE, MLST, and cgMLST. Genome analysis was used to identify intrinsic and acquired resistance determinants, including mutations in the AdeRSABC efflux system and in outer membrane proteins (OMPs). All isolates were identified as A. baumannii and grouped into 4 pulsotypes by PFGE, which belonged to clonal complexes (CC) 15Pas/103Ox (n = 4) and 79Pas/113Ox (n = 14), corresponding to IC4 and IC5, respectively. High MIC values to carbapenems, broad-spectrum cephalosporins, amikacin, and ciprofloxacin were observed in all isolates, while MICs of ampicillin/sulbactam, gentamicin, and tigecycline varied among the isolates. Minocycline was the most active antimicrobial agent tested. Moreover, 12 isolates (66.7%) were considered resistant to polymyxins. Besides intrinsic OXA-51 and ADC variants, all isolates harbored an acquired carbapenem-hydrolyzing class D β-lactamase (CHDL) encoding gene, either blaOXA–23 or blaOXA–72. A diversity of aminoglycoside modifying enzymes and resistance determinants to other antimicrobial classes were found, as well as mutations in gyrA and parC. Non-synonymous mutations have also been identified in the AdeRSABC efflux system and in most OMPs, but they were considered natural polymorphisms. Moreover, resistance to polymyxins among isolates belonging to IC5 were associated to non-synonymous mutations in pmrB, but no known polymyxin resistance mechanism was identified in isolates belonging to IC4. In conclusion, A. baumannii clinical isolates belonging to South America’s major clones present a myriad of antimicrobial resistance determinants. Special attention should be paid to natural polymorphisms observed in each clonal lineage, especially regarding non-synonymous mutations in constitutive genes associated with distinct resistance phenotypes.
Acinetobacter baumannii is a major nosocomial pathogen causing serious infections (Antunes et al., 2014). In Brazil, it is the fourth most frequent pathogen recovered from central catheter-associated bloodstream infections (BSI) in adult intensive care units (ICU), with carbapenem resistance rates as high as 79% (Agência Nacional de Vigilância Sanitária [ANVISA], 2020). Carbapenem resistance in A. baumannii is mainly caused by horizontal transfer of carbapenem-hydrolyzing class D β-lactamases (CHDL) encoding genes, particularly in worldwide epidemic clones (Higgins et al., 2010).
Most carbapenem-resistant isolates also harbor resistance determinants to other antimicrobial classes, such as aminoglycosides and fluoroquinolones. Additionally, resistance to polymyxins has been sporadically reported among some carbapenem-resistant endemic clones (Qureshi et al., 2015), and these isolates are often classified as XDR or pan-drug resistant (PDR) (Nowak et al., 2017). Carbapenem-resistant A. baumannii (CRAB) isolates usually belong to the worldwide disseminated international clone 2 (IC2) (Hamidian and Nigro, 2019) and carbapenem-resistance rates vary between 40 and 80% (Kuo et al., 2012; Chmielarczyk et al., 2016). In Latin America, the frequency of XDR A. baumannii has increased from 17 to 86.6% between 1997 and 2016 (Gales et al., 2019).
Interestingly, CRAB isolates in South America are not associated with IC2. In Brazil, as well as Argentina, Chile, and Paraguay, the major carbapenemase-producing clones belong to clonal complexes (CCs) 15 and 79, which correspond to IC4 and IC5, respectively (Higgins et al., 2010; Chagas et al., 2014; Cardoso et al., 2016; Rodríguez et al., 2016; Opazo-Capurro et al., 2019). Despite the variety of studies focusing on the genetic context of carbapenemase encoding genes (Chagas et al., 2017; Romanin et al., 2019), comprehensive studies on the genetic basis of XDR phenotype among isolates representing these important ICs are still missing (Graña-Miraglia et al., 2020). Herein, we explored the molecular determinants associated with resistance toward multiple drugs among A. baumannii clinical isolates belonging to the major South American clonal lineages recovered in distinct Brazilian hospitals.
A total of 18 Acinetobacter spp. clinical isolates were included in study. They were recovered in five tertiary hospitals located in two Brazilian states between April 2012 and October 2017. Isolates were identified to the species level by rpoB sequencing as previously described (La Scola et al., 2006). Minimal Inhibitory Concentrations (MICs) for amikacin, ceftazidime, ciprofloxacin, colistin, cefepime, gentamicin, imipenem, meropenem, minocycline, polymyxin B, and tigecycline (Sigma-Aldrich, St. Louis, United States) were determined by cation-adjusted broth microdilution and interpreted according to Brazilian Committee on Antimicrobial Susceptibility Testing (BrCAST/EUCAST) guidelines1, when clinical breakpoints for Acinetobacter spp. were available. Ampicillin/sulbactam MICs were determined following the guidelines established by BrCAST/EUCAST for Enterobacteriales.
All the isolates were subjected to WGS using an Illumina MiSeq sequencer (Illumina Inc., CA, United States) (Higgins et al., 2017), and genomes were assembled with the program Velvet as part of the SeqSphere v.7.0.4 software (Ridom GmbH, Münster, Germany) as described previously (Higgins et al., 2017). To determine differences in specific ORFs in each draft genome sequence, the genome assemblies were aligned to the A. baumannii reference strain ACICU and compared to nucleotide and protein sequences of A. baumannii ATCC 19606 (accession number NZ_KL810966.1) and ATCC 17978 (accession number NZ_CP018664.1).
Clonal relatedness of A. baumannii isolates was determined by pulsed-field gel electrophoresis (PFGE) using ApaI restriction enzyme (New England BioLabs, Ipswich, MA, United States) and fingerprints were analyzed with previously described criteria (Seifert et al., 2005). WGS data were also used to characterize the isolates by core genome multilocus sequence typing (cgMLST), as previously published (Higgins et al., 2017) and to determine the sequence types (STs) using PubMLST2.
Intrinsic and acquired resistance determinants were screened using ResFinder, version 3.23 and manually curated using Artemis. The presence of insertion sequences upstream of β-lactamase encoding genes was also screened using ISfinder4. Non-synonymous mutations in gyrA and parC were detected comparing the genome sequences with reference A. baumannii strain ATCC 19606. Moreover, amino acid substitutions in polymyxin resistance associated systems LpxACD and PmrAB were detected as described by Gerson et al. (2020). This approach was also used to identify potential amino acid substitutions associated with antimicrobial resistance in the efflux pumps system AdeABC, its regulator AdeRS, and in outer membrane proteins (OMPs) CarO, OmpA, OmpW, Omp33-36, and OccAB1. Additionally, the relative expression of pmrAB was determined by qRT-PCR using specific primers previously described (Girardello et al., 2017). Assays were performed in triplicate and expression was compared to that of A. baumannii ATCC 19606, using rpoB as a normalizing gene (Hornsey et al., 2010).
All isolates were identified as A. baumannii and were mainly recovered from blood cultures (n = 9) followed by lower respiratory tract cultures (n = 6) (Table 1). According to PFGE, they were grouped into four distinct pulsotypes, which were included in different STs belonging to Institute Pasteur scheme (Pas) CC79 and CC15, and corresponded to the Oxford scheme (Ox) CC113 and CC103, respectively (Table 1 and Supplementary Figure 1). Interestingly, the ST distribution following the Oxford scheme presented a better correlation to the results observed with PFGE, since ST227, ST233, ST236, and the novel ST2141 were strongly associated with PFGE clusters 1, 2, 4, and 3, respectively (Supplementary Figure 1). Moreover, cgMLST delineated the isolates into four transmission clusters and seven singletons (Figure 1 and Supplementary Figure 1). According to MLST analysis, all CRAB clinical isolates were grouped into two major South American clones, namely IC4 (CC15Pas/CC103Ox; n = 4) and IC5 (CC79Pas/CC113Ox; n = 14). It should also be noted that every CC was found over time and in distinct hospitals, confirming the wide spread of those STs in Brazil.
Table 1. Clinical and epidemiological data and antimicrobial susceptibility profile of A. baumannii clinical isolates included in the study.
Figure 1. Minimum spanning tree of A. baumannii clinical isolates, according to cgMLST. Numbers between isolates indicate the allele differences identified among the 2,390 alleles that belong to A. baumannii core genome, ignoring missing values. Colors indicate isolates belonging to the same international clones. Gray zones indicate isolates belonging to the same cluster, and isolate numbers in the same circle were considered identical. Cluster distance threshold: 9. Minimum spanning tree built using Ridom SeqSphere+.
All isolates were resistant to a broad range of antimicrobial agents (Table 1). High MICs were observed in most isolates for ampicillin/sulbactam (MIC range, ≤0.5–>256/4 μg/mL), ceftazidime (MIC range, 8–>128 μg/mL), cefepime (32–>256 μg/mL), imipenem (64–>256 μg/mL), meropenem (32–256 μg/mL), amikacin (16–512 μg/mL), and ciprofloxacin (32–>64 μg/mL). Additionally, 66 and 72% of the isolates were resistant to gentamicin (≤0.5–>128 μg/mL) and both polymyxins (polymyxin B and colistin; ≤0.25–>128 μg/mL), respectively. On the other hand, MICs values for tigecycline ranged from 0.5 to 16 μg/mL. Only minocycline showed consistent activity against all A. baumannii isolates (MICs, ≤0.25–2 μg/mL; Table 1). Interestingly, polymyxin B presented a higher in vitro activity compared to colistin against polymyxin-resistant isolates, since colistin MICs were at least two-fold dilutions higher than polymyxin B. In contrast, polymyxin B and colistin MICs did not differ among polymyxin-susceptible isolates.
As expected, all A. baumannii isolates harbored intrinsic chromosome encoded blaOXA–51–like genes. Moreover, each variant was correlated to an IC, being blaOXA–65 (n = 14; IC5, CC79Pas) the most frequently identified (Figure 2 and Supplementary Table 1). The insertion sequence (IS) ISAba1 was found upstream of those genes in one and five isolates belonging to IC4 and IC5, respectively. All isolates harbored novel variants of blaADC flanked upstream by ISAba1, namely blaADC–181 (n = 4), blaADC–182 (n = 13), and blaADC–183 (n = 1), and were associated with ST15Pas, ST79Pas, and ST730Pas, respectively. The acquired CHDL encoding genes blaOXA–23 and blaOXA–72 were found in 13 (72.2%) and five (27.8%) A. baumannii isolates, respectively. The blaOXA–23 gene was also flanked upstream by ISAba1 in all isolates included in the study. Interestingly, blaOXA–72 was only detected in isolates belonging to IC5 (Figure 2 and Supplementary Table 1). Furthermore, the narrow-spectrum β-lactamase encoding gene blaTEM–1 was also found in 83.3% of isolates (n = 15). No other β-lactamase encoding gene was detected among these isolates.
Figure 2. Overview of the acquired antimicrobial resistance determinants and intrinsic β-lactamases encoding genes observed in A. baumannii clinical isolates. Isolates belonging to distinct CCs are indicated by different colors. Dendrogram was generated using UPGMA method with BioNumerics, version 7.5, based on ApaI-PFGE results.
The reduced susceptibility to amikacin observed in all isolates was associated with the presence of the aminoglycoside modifying enzyme (AME) aph(3′)-VIa (Figure 2 and Supplementary Table 1). Additionally, the isolates belonging to IC5 also harbored the phosphotransferase encoding genes strA and strB, as well as the nucleotidyltransferase encoding gene aadA1. Moreover, one IC5 genetic cluster produced an acetyltransferase belonging to the AAC(6′)-Ib family, which explains the high gentamicin resistance levels observed in such isolates (>128 μg/mL). On the other hand, only one isolate belonging to IC4 harbored an acetyltransferase encoding gene, aac(3)-IIa, and no additional AME was observed in IC4 isolates (Figure 2 and Supplementary Table 1). The arsenal of acquired resistance determinants observed in IC5 also included sul2, dfrA1, and floR-like genes, which promote reduced susceptibility to sulfamethoxazole, trimethoprim, and chloramphenicol, respectively (Figure 2 and Supplementary Table 1). Additionally, the ST79Pas isolates 67098, 67745, and 20216722 harbored the macrolide resistance determinants mph(E) and msr(E), which were also found in the ST15Pas isolates 71813 and 71838. Finally, the isolate 206182 harbored both sul1 and sul2.
The high ciprofloxacin MICs observed in all the isolates can be explained by the simultaneous presence of Ser83Leu and Ser80Leu amino acid substitutions in the quinolone resistance determinant region (QRDR) of gyrA and parC, respectively (Supplementary Table 1). Additionally, point mutations were observed in pmrAB in most polymyxin-resistant isolates belonging to IC5 (Table 2). However, the presence of such mutations was not always associated with overexpression of that two-component system (TCS) (Supplementary Figure 2). Interestingly, ISAba125 was observed within pmrA in two polymyxin-susceptible A. baumannii isolates belonging to ST79Pas, which might have disrupted the function of this transcriptional regulator (Supplementary Table 1). In fact, these isolates presented a reduced expression of pmrA, as assessed by qRT-PCR (Supplementary Figure 2). It was also worth noting that some mutations observed in IC5 were present in both polymyxin-resistant and -susceptible isolates and were considered natural polymorphisms associated with this IC, including a duplication of ten amino acids in PmrB observed in some isolates (Supplementary Table 1). A high number of polymorphisms was also observed in isolates belonging to IC4 (Figure 3). However, none of them was exclusively found in polymyxin-resistant isolates, suggesting that such substitutions were not associated with polymyxin resistance in IC4, and might explain the absence of pmrAB overexpression among those isolates (Supplementary Table 1 and Supplementary Figure 2).
Table 2. Amino acid substitutions and relative expression of TCS PmrAB in polymyxin-resistant A. baumannii clinical isolates belonging to IC5.
Figure 3. Alignment of PmrA (A,B) and PmrB (C,D) protein sequences observed in polymyxin-resistant A. baumannii clinical isolates 182122 and 10042 belonging to IC4, compared to A. baumannii reference strain ATCC 19606 (A,C) or to the polymyxin-susceptible IC4 A. baumannii isolate 71813 (B,D).
The substitutions observed in LpxD in all isolates were also considered to be natural polymorphisms, as they were strictly related to the genetic background of the isolates rather than their resistance phenotype (Supplementary Table 1). While only isolates belonging to IC5 harbored the pmrC homolog eptA, no mcr genes were detected among the 18 A. baumannii isolates evaluated. In fact, no known polymyxin resistance mechanism was identified among those isolates belonging to IC4, suggesting that novel mechanisms might be responsible for the high polymyxins MICs (ranging from 32 to >128 μg/mL) observed in isolates 182122 and 10042 (Table 1).
Genes encoding OMPs also presented clone-associated alleles. As shown in Table 3, the allelic variation of ompA included non-synonymous mutations specific for each CC. This was also observed in CarO, where isolates belonging to IC5 presented protein sequences identical to the one observed in the A. baumannii reference strain ATCC 19606, while those belonging to IC4 presented more than 60 amino acid substitutions (73.1% identity; Figure 4 and Supplementary Table 1). In contrast, the OprD-like protein OccAb1 was identical in both ICs, even though three amino acid substitutions were observed compared to the reference strain (Table 3). No amino acid substitutions were observed in OmpW and Omp33-36.
Figure 4. Alignment of CarO protein sequences observed in A. baumannii clinical isolates belonging to IC4, compared to A. baumannii reference strain ATCC 19606.
Even though the protein sequences of AdeB were conserved in A. baumannii clinical isolates belonging to both IC4 and IC5 and identical to the A. baumannii reference strain ATCC 19606, adeA presented distinct alleles in each IC. While this gene was identified as wild type in isolates belonging to IC5, two amino acid substitutions were identified in all IC4 isolates, namely Ala368Leu and Thr386Asn (Supplementary Table 1). Moreover, proteins belonging to the TCS AdeRS also presented distinct sequences in each CC, which differ from ATCC 19606 (Figure 5). It should be noted that some amino acid substitutions were observed in both IC4 and IC5 isolates, such as Val136Ala and Leu142Ile in AdeR, and Ala153Thr, Leu214Phe, Ser263Ala, Ala280Ser, and Asp281Gln in AdeS.
Figure 5. Alignment of (A) AdeR and (B) AdeS protein sequences observed in A. baumannii clinical isolates belonging to IC4 and IC5, compared to A. baumannii reference strain ATCC 19606.
The worldwide spread of multidrug-resistant (MDR) A. baumannii clones is considered a public health concern. The World Health Organization considers research and surveillance of emerging resistance phenotypes as one of the strategic goals of their Global Action Plan on Antimicrobial Resistance (WHO, 2015). Over the years, the evolution of successful A. baumannii clones allowed them to acquire an increasing number of resistance determinants, not only via mobile genetic elements, but also through mutations in constitutive genes (Antunes et al., 2014). In the present study we demonstrated that this genetic plasticity is also responsible for resistance to several antimicrobials among South American A. baumannii major clones.
The importance of A. baumannii clinical isolates belonging to IC4 and IC5 in South America has been previously demonstrated (Cieslinski et al., 2013; Stietz et al., 2013; Rodríguez et al., 2016; Opazo-Capurro et al., 2019; Müller et al., 2019). The higher frequency of those clones was also observed in different regions in Brazil and has been associated with an XDR phenotype (Royer et al., 2018; Pagano et al., 2019; Tavares et al., 2019). Interestingly, despite their wide distribution through time and space, the IC4 and IC5 clinical isolates included in this study remained highly genetically related, as determined using both PFGE and cgMLST. Vasconcelos et al. (2015) have also identified highly similar A. baumannii clinical isolates causing BSI in distinct Brazilian medical centers by REP-PCR, and the most frequent clones described also belonged to IC4 and IC5. Even though most isolates belonging to the same pulsotype were not grouped in the same cluster according to cgMLST, the number of allele differences between those isolates were usually lower than the ones observed between isolates that were not considered genetically related according to PFGE. It should be noted, however, that the cgMLST cluster distance threshold was established based on the allele differences and designed for the investigation of A. baumannii transmission clusters or outbreaks (Higgins et al., 2017). These values should be interpreted with caution when evaluating isolates broadly distributed over time and space. In the current study, some isolates were separated in time by several years, and by a distance of over 1000 km. Moreover, it should be noted that isolates belonging to the same ST not always form monophyletic groups based on cgMLST, as previously reported (Castillo-Ramírez and Graña-Miraglia, 2019; Gaiarsa et al., 2019). Additionally, the differences observed in isolates belonging to ST227Ox and ST233Ox (both part of ST79Pas) might be associated with recombination events in the vicinities of the gpi allele, as described by Hamouda et al. (2010).
The variety of intrinsic β-lactamase encoding genes was also related to the clonal groups each isolate was assigned to. As expected, all isolates belonging to IC5 harbored blaOXA–65, while blaOXA–51 was present in all isolates belonging to IC4, as previously described (Zander et al., 2012). However, the presence of the ISAba1 upstream the blaOXA–51-like genes was associated to specific pulsotypes within each IC, namely 1B and 1C from IC5, and 3 from IC4. It should be noted that the low frequency of ISAba1-blaOXA–51-like structure among Brazilian isolates, especially in blaOXA–23-producing strains, was previously reported (Pagano et al., 2013). On the other hand, this IS was observed upstream of blaADC-like genes in all isolates and could have been responsible for the high third-generation cephalosporin MIC values observed (Cardoso et al., 2016). It is worth noting that each novel ADC variant was identified in a different ST, even though it is not yet clear if the presence of specific ADCs in A. baumannii isolates can also be linked to an IC, as demonstrated for blaOXA–51 (Rodríguez-Martínez et al., 2010; Karah et al., 2017).
Although the intrinsic OXA-51 and ADC variants were closely related to each IC included in the study, the acquired CHDL encoding gene blaOXA–23 was observed in both ICs, as expected. OXA-23 remains the most frequent carbapenem resistance determinant among A. baumannii worldwide (Hamidian and Nigro, 2019), and is highly frequent in IC4 and IC5, as previously described (Rodríguez et al., 2016). On the other hand, the production of OXA-72 was restricted to A. baumannii clinical isolates belonging CC79Pas/CC113Ox. Previous studies have demonstrated the emergence of this CHDL not only within ST79Pas, but also within its single locus variant (SLV) ST730, in different Brazilian states (Vasconcelos et al., 2015; de Azevedo et al., 2019), as well as among carbapenem-resistant A. baumannii belonging to ST15Pas (Pagano et al., 2017), but such wide distribution of blaOXA–72 was not observed among our isolates.
Overall, isolates belonging to IC5 presented a broader spectrum of acquired antimicrobial resistance determinants compared to IC4. The presence of the AME encoding genes aadA1, strA and strB, as well as dfrA1, has been previously associated to class 2 integrons in IC5 in different Latin American countries (Martins et al., 2015; Ramírez et al., 2015; Pagano et al., 2019; Romanin et al., 2019). Moreover, blaTEM–1 was more frequent in this IC and might have contributed to the high ampicillin-sulbactam MICs observed, especially among OXA-23-producing isolates, as previously described (Krizova et al., 2013; Cardoso et al., 2016). Interestingly, all IC4 and IC5 blaTEM–1-producing isolates also carried sul2, suggesting that both antimicrobial resistance determinants could be located in the same mobile genetic element, as previously reported (Hammad et al., 2019).
Despite the differences observed between the major South American A. baumannii clones, the high amikacin resistance levels observed in all isolates was associated with the presence of aph(3′)-VIa. The Aph(3′)-VIa AME was first described in A. baumannii and its activity spectrum includes amikacin (Ramírez and Tolmasky, 2010). Previous studies have also described the high frequency of this AME among A. baumannii clinical isolates worldwide, including in Brazil (Aghazadeh et al., 2013; Polotto et al., 2019). Other studies have also highlighted that gentamicin resistance was associated with distinct acetyltransferases, as observed among our isolates, and have suggested that a combination of AMEs could be responsible for that phenotype, as observed in our isolate 206182 (Ballaben et al., 2018). Additionally, Matos et al. (2019) described the presence of aac(3)-IIa and blaTEM–1 in a plasmid of an A. baumannii ST15Pas clinical isolate, as observed in our 10042 isolate, which also belonged to the same ST. Interestingly, that plasmid also harbored the CHDL encoding gene blaOXA–58 (Matos et al., 2019), which was not observed in our isolate.
Despite the critical role that acquired resistance determinants play in the acquisition and maintenance of MDR among A. baumannii clones, mutations in constitutive genes can also cause high resistance levels to important antimicrobial agents. Holt et al. (2016) have previously suggested that the presence of mutations in gyrA and parC was one of the features leading to the epidemiological success of IC1, however, this lineage is now rarely found and yet fluoroquinolone resistance remains high. In fact, the combination of Ser83Leu and Ser80Leu in GyrA and ParC, respectively, was additive (Vila et al., 1997), leading to high ciprofloxacin MICs, as observed among our isolates.
Mutations in the constitutive pmrAB genes seemed to be responsible for reduced susceptibility to polymyxins among IC5 isolates. The majority of amino acid substitutions were observed in PmrB and, to the best of our knowledge, most of them have not been described elsewhere. In fact, amino acid substitutions in the histidine kinase of this TCS seem to play an important role in reduced susceptibility to polymyxin in A. baumannii, since a high number of non-synonymous mutations in pmrB among colistin-resistant isolates was previously highlighted (Olaitan et al., 2014; Poirel et al., 2017; Gerson et al., 2020). The Ser119Thr substitution in PmrA, observed in all isolates belonging to IC5, has been previously associated with polymyxin resistance (Arroyo et al., 2011), including in Brazilian isolates (Leite et al., 2019). However, this substitution was also observed among polymyxin-susceptible isolates, suggesting that it might have just a limited role in this phenotype. Moreover, it should be noted that most substitutions described in our study were considered natural polymorphisms associated with distinct clonal linages. These substitutions were also not associated with the higher in vitro activity of polymyxin B, compared to colistin, observed among polymyxin-resistant isolates. Such variation might be related to recombination events, as described for other Acinetobacter species (Kim and Ko, 2015). It has been previously suggested that polymyxin B and colistin MIC values may be distinct among A. baumannii clinical isolates, but the MIC variations were always ± one-fold dilution, and colistin MICs were not consistently higher than polymyxin B (Gales et al., 2001). To test this hypothesis, a larger number of isolates should be evaluated to confirm the distinct activities of both polymyxins among A. baumannii isolates presenting reduced susceptibility to those antimicrobial agents.
Gerson et al. (2020) have recently demonstrated that the identification of PmrCAB amino acid substitutions with potential roles in polymyxin resistance should be carried out in isogenic isolates to eliminate natural polymorphisms between lineages. However, the authors have mainly explored that feature among IC2 isolates. We have demonstrated that this is also true for South American major clones, especially among IC4 isolates, where a high number of pmrAB mutations was observed but none of them was unique to polymyxin-resistant isolates. This is also valid for the lpxACD operon, which has previously been associated with polymyxin resistance in A. baumannii (Moffatt et al., 2010). Some of the amino acid substitutions observed in LpxD have been previously described in polymyxin-susceptible and -resistant isolates (Oikonomou et al., 2015), corroborating our findings and confirming they were natural polymorphisms. Additionally, the absence of eptA among IC4 belonging isolates observed in our study has been previously described (Gerson et al., 2019) and highlights the presence of unknown polymyxin resistance mechanisms among CC15Pas/CC103Oxf A. baumannii clinical isolates.
The reduced susceptibility to tigecycline observed among some A. baumannii isolates suggested that the AdeABC efflux system might have been overexpressed, considering that this glycylcycline is a substrate for this system (Lashinsky et al., 2017). The TCS AdeRS regulates its expression and amino acid substitutions in those proteins have been associated with higher tigecycline MICs (Ruzin et al., 2007; Gerson et al., 2018). Although a high number of amino acid substitutions was observed in AdeRS in the isolates included in this study, they did not seem to have influenced tigecycline MICs, since isolates with the same pattern of mutations presented MICs ranging from 0.5 to 16 μg/mL. In fact, the substitution patterns were identical within each IC, suggesting that they were only natural polymorphisms. Therefore, the activity of other efflux systems, such as AdeIJK, might have been responsible for tigecycline MIC variations observed in our study, as previously reported (Damier-Piolle et al., 2008).
The great variety of resistance determinants observed among the isolates in this study might have masked the role of OMPs in our isolates. The high number of amino substitutions observed, especially in CarO, complicates the identification of mutations with a potential role in antimicrobial resistance. Additionally, the fact that the observed mutation patterns were identical in all isolates belonging to the same lineage suggests that they were polymorphisms associated with the evolution of different clonal lineages. Interestingly, Zhu et al. (2019) have previously highlighted the high variability of CarO protein sequences among A. baumannii isolates, including the presence of small insertions and deletions, as observed among ST15Pas isolates. Moreover, Mussi et al. (2011) have demonstrated that such diversity could be associated with horizontal gene transfer and assertive recombination. Further studies are required to determine the contribution of this variability to antimicrobial resistance.
In summary, we demonstrated that a diversity of antimicrobial resistance determinants was present in the major South American A. baumannii clones. We have also provided evidence that attention should be payed to natural polymorphisms when comparing isolates with distinct phenotypes and genetic background, since most constitutive genes associated with antimicrobial resistance presented amino acid substitutions that did not play a role in reduced antimicrobial susceptibility. In addition, the contribution of high frequency of polymorphisms in hot spot genes among endemic clones must be evaluated with caution. Additionally, we suggested that unknown polymyxin resistance mechanisms might be present in A. baumannii isolates belonging to IC4. The resistance phenotype exhibited by endemic A. baumannii clones was very worrisome and should be carefully studied, considering that the molecular mechanisms involved seem to be, to some extent, lineage-specific.
Carbapenem-resistant Acinetobacter baumannii (CRAB) have emerged worldwide due to the dissemination of successful clones. Even though international clone 2 (IC2) is the most prevalent in many countries, in Latin America CRAB isolates often belong to IC4 and IC5. The majority of CRAB isolates are also resistant to multiple other antimicrobials and have been classified as extremely drug-resistant (XDR). However, data exploring the molecular mechanisms involved in this phenotype among IC4 and IC5 are still scarce. In this study we presented a comprehensive analysis of genome sequencing data from Brazilian A. baumannii belonging to these important clones. We demonstrated that a combination of intrinsic and acquired resistance determinants was responsible for the resistance to several antimicrobial agents among Brazilian endemic clones. We also suggested that an unknown mechanism was responsible for the emergence of polymyxin resistance among IC4 clinical isolates. Finally, we highlighted the importance of comparing isolates with similar genetic background when evaluating mutations in constitutive genes, including efflux systems and outer membrane protein encoding genes.
All raw reads generated were submitted to the Sequencing Read Archive (https://www.ncbi.nlm.nih.gov/sra/) of the National Center for Biotechnology Information (NCBI) under the BioProject number PRJNA632943. The sequences of the novel blaADC–181, blaADC–182, and blaADC–183 genes were deposited in NCBI β-lactamases database under the accession numbers MK248721, MK248722, and MK248723, respectively.
The datasets presented in this study can be found in online repositories. The names of the repository/repositories and accession number(s) can be found below: https://www.ncbi.nlm.nih.gov/, PRJNA632943; https://www.ncbi.nlm.nih.gov/, MK248721; https://www.ncbi.nlm.nih.gov/, MK248722; https://www.ncbi.nlm.nih.gov/, MK248723.
Ethical approval for this study was obtained from Research Ethics Committee from Federal University of São Paulo – UNIFESP/São Paulo Hospital (Process number: CEP N 4665141216).
CN, RC, PH, HS, and AG contributed to the study conception and design. RC and PH supervised the assays. MA, AIdP, and ACCP provided the strains and clinical data. CN, AS, FL, and JW performed the data collection and analysis. CN wrote the first draft of the manuscript and it was edited by RC. PH, HS, and AG reviewed the final draft of manuscript. All authors read and approved the final version of manuscript.
We are grateful to Coordenação de Aperfeiçoamento de Pessoal de Nível Superior (CAPES) for providing PDSE grants to CN (Process number: 88881.187573/2018-01) and AS and to the National Council for Science and Technological Development (CNPq) for providing grants to FL and AG (Process number: 312066/2019).
AG has recently received research funding and/or consultation fees from Bayer, Cristália, InfectoPharm, Eurofarma, MSD, Pfizer and Zambon.
The remaining authors declare that the research was conducted in the absence of any commercial or financial relationships that could be construed as a potential conflict of interest.
We would like to thank Cecília G. Carvalhaes and Thaís Guimarães for providing some of clinical isolates evaluated in this study, and to Larissa Akie Natsumeda and Karen de Castro Bauab for their excellent technical support. This work was presented in part as a Poster (P1109) at the 28th European Congress of Clinical Microbiology and Infectious Diseases (ECCMID) in Madrid, Spain, 2018.
The Supplementary Material for this article can be found online at: https://www.frontiersin.org/articles/10.3389/fmicb.2020.584603/full#supplementary-material
Agência Nacional de Vigilância Sanitária [ANVISA] (2020). Boletim Segurança do Paciente e Qualidade em Serviços de Saúde n° 18: Avaliação dos indicadores nacionais das Infecções Relacionadas à Assistência à Saúde (IRAS) e Resistência microbiana do ano de 2018. Brasília: ANVISA.
Aghazadeh, M., Rezaee, M. A., Nahaei, M. R., Mahdian, R., Pajand, O., Saffari, F., et al. (2013). Dissemination of aminoglycoside-modifying enzymes and 16S rRNA methylases among Acinetobacter baumannii and Pseudomonas aeruginosa isolates. Microb. Drug Resist. 9, 282–288. doi: 10.1089/mdr.2012.0223
Antunes, L. C., Visca, P., and Towner, K. J. (2014). Acinetobacter baumannii: evolution of a global pathogen. Pathog. Dis. 71, 292–301. doi: 10.1111/2049-632X.12125
Arroyo, L. A., Herrera, C. M., Fernandez, L., Hankins, J. V., Trent, M. S., and Hancock, R. E. (2011). The pmrCAB operon mediates polymyxin resistance in Acinetobacter baumannii ATCC 17978 and clinical isolates through phosphoethanolamine modification of lipid A. Antimicrob. Agents Chemother. 55, 3743–3751. doi: 10.1128/AAC.00256-11
Ballaben, A. S., Andrade, L. N., Galetti, R., Ferreira, J. C., McElheny, C. L., Mettus, R. T., et al. (2018). Diversity of high-level aminoglycoside resistance mechanisms among Gram-negative nosocomial pathogens in Brazil. Antimicrob. Agents Chemother. 62:e01550-18. doi: 10.1128/AAC.01550-18
Cardoso, J. P., Cayô, R., Girardello, R., and Gales, A. C. (2016). Diversity of mechanisms conferring resistance to β-lactams among OXA-23-producing Acinetobacter baumannii clones. Diagn. Microbiol. Infect. Dis. 85, 90–97. doi: 10.1016/j.diagmicrobio.2016.01.018
Castillo-Ramírez, S., and Graña-Miraglia, L. (2019). Inaccurate multilocus sequence typing of Acinetobacter baumannii. Emerg. Infect. Dis. 25, 186–187. doi: 10.3201/eid2501.180374
Chagas, T. P., Carvalho, K. R., de Oliveira Santos, I. C., Carvalho-Assef, A. P., and Asensi, M. D. (2014). Characterization of carbapenem-resistant Acinetobacter baumannii in Brazil (2008-2011): countrywide spread of OXA-23-producing clones (CC15 and CC79). Diagn. Microbiol. Infect. Dis. 79, 468–472. doi: 10.1016/j.diagmicrobio.2014.03.006
Chagas, T. P. G., Tavares, E., Oliveira, T. R., D’Alincourt Carvalho-Assef, A. P., Albano, R. M., and Asensi, M. D. (2017). Carbapenem-resistant Acinetobacter pittii strain harboring bla(OXA-72) from Brazil. Diagn. Microbiol. Infect. Dis. 88, 93–94. doi: 10.1016/j.diagmicrobio.2017.01.022
Chmielarczyk, A., Pilarczyk-Żurek, M., Kamiñska, W., Pobiega, M., Romaniszyn, D., Ziółkowski, G., et al. (2016). Molecular Epidemiology and drug resistance of Acinetobacter baumannii isolated from hospitals in Southern Poland: ICU as a risk factor for XDR strains. Microb. Drug Resist. 22, 328–335. doi: 10.1089/mdr.2015.0224
Cieslinski, J. M., Arend, L., Tuon, F. F., Silva, E. P., Ekermann, R. G., Dalla-Costa, L. M., et al. (2013). Molecular epidemiology characterization of OXA-23 carbapenemase-producing Acinetobacter baumannii isolated from 8 Brazilian hospitals using repetitive sequence-based PCR. Diagn. Microbiol. Infect. Dis. 77, 337–340. doi: 10.1016/j.diagmicrobio.2013.07.018
Damier-Piolle, L., Magnet, S., Brémont, S., Lambert, T., and Courvalin, P. (2008). AdeIJK, a resistance-nodulation-cell division pump effluxing multiple antibiotics in Acinetobacter baumannii. Antimicrob. Agents Chemother. 52, 557–562. doi: 10.1128/AAC.00732-07
de Azevedo, F. K. S. F., Dutra, V., Nakazato, L., Mello, C. M., Pepato, M. A., de Sousa, A. T. H. I., et al. (2019). Molecular epidemiology of multidrug-resistant Acinetobacter baumannii infection in two hospitals in Central Brazil: the role of ST730 and ST162 in clinical outcomes. J. Med. Microbiol. 68, 31–40. doi: 10.1099/jmm.0.000853
Gaiarsa, S., Batisti Biffignandi, G., Esposito, E. P., Castelli, M., Jolley, K. A., Brisse, S., et al. (2019). Comparative analysis of the two Acinetobacter baumannii multilocus sequence typing (MLST) schemes. Front. Microbiol. 10:930. doi: 10.3389/fmicb.2019.00930
Gales, A. C., Reis, A. O., and Jones, R. N. (2001). Contemporary assessment of antimicrobial susceptibility testing methods for polymyxin B and colistin: review of available interpretative criteria and quality control guidelines. J. Clin. Microbiol. 39, 183–190. doi: 10.1128/JCM.39.1.183-190.2001
Gales, A. C., Seifert, H., Gur, D., Castanheira, M., Jones, R. N., and Sader, H. S. (2019). Antimicrobial susceptibility of Acinetobacter calcoaceticus-Acinetobacter baumannii complex and Stenotrophomonas maltophilia clinical isolates: results from the SENTRY antimicrobial surveillance program (1997-2016). Open Forum Infect. Dis. 6(Suppl. 1), S34–S46. doi: 10.1093/ofid/ofy293
Gerson, S., Betts, J. W., Lucaßen, K., Nodari, C. S., Wille, J., Josten, M., et al. (2019). Investigation of novel PmrB and EptA mutations in isogenic Acinetobacter baumannii isolates associated with colistin resistance and increased virulence in vivo. Antimicrob. Agents Chemother. 63:e01586-18. doi: 10.1128/AAC.01586-18
Gerson, S., Lucaßen, K., Wille, J., Nodari, C. S., Stefanik, D., Nowak, J., et al. (2020). Diversity of amino acid substitutions in PmrCAB associated with colistin resistance in clinical Acinetobacter baumannii isolates. Int. J. Antimicrob. Agents 55:105862. doi: 10.1016/j.ijantimicag.2019.105862
Gerson, S., Nowak, J., Zander, E., Ertel, J., Wen, Y., Krut, O., et al. (2018). Diversity of mutations in regulatory genes of resistance-nodulation-cell division efflux pumps in association with tigecycline resistance in Acinetobacter baumannii. J. Antimicrob. Chemother. 73, 1501–1508. doi: 10.1093/jac/dky083
Girardello, R., Visconde, M., Cayô, R., Figueiredo, R. C., Mori, M. A., Lincopan, N., et al. (2017). Diversity of polymyxin resistance mechanisms among Acinetobacter baumannii clinical isolates. Diagn. Microbiol. Infect. Dis. 87, 37–44. doi: 10.1016/j.diagmicrobio.2016.10.011
Graña-Miraglia, L., Evans, B. A., López-Jácome, L. E., Hernández-Durán, M., Colín-Castro, C. A., Volkow-Fernández, P., et al. (2020). Origin of OXA-23 Variant OXA-239 from a recently emerged lineage of Acinetobacter baumannii international clone V. mSphere 5, e801–e819. doi: 10.1128/mSphere.00801-19
Hamidian, M., and Nigro, S. J. (2019). Emergence, molecular mechanisms and global spread of carbapenem-resistant Acinetobacter baumannii. Microb. Genom. 5:e000306. doi: 10.1099/mgen.0.000306
Hammad, A. M., Hoffmann, M., Gonzalez-Escalona, N., Abbas, N. H., Yao, K., Koenig, S., et al. (2019). Genomic features of colistin resistant Escherichia coli ST69 strain harboring mcr-1 on IncHI2 plasmid from raw milk cheese in Egypt. Infect. Genet. Evol. 73, 126–131. doi: 10.1016/j.meegid.2019.04.021
Hamouda, A., Evans, B. A., Towner, K. J., and Amyes, S. G. (2010). Characterization of epidemiologically unrelated Acinetobacter baumannii isolates from four continents by use of multilocus sequence typing, pulsed-field gel electrophoresis, and sequence-based typing of bla(OXA-51-like) genes. J. Clin. Microbiol. 48, 2476–2483. doi: 10.1128/JCM.02431-09
Higgins, P. G., Dammhayn, C., Hackel, M., and Seifert, H. (2010). Global spread of carbapenem-resistant Acinetobacter baumannii. J. Antimicrob. Chemother. 65, 233–238. doi: 10.1093/jac/dkp428
Higgins, P. G., Prior, K., Harmsen, D., and Seifert, H. (2017). Development and evaluation of a core genome multilocus typing scheme for whole-genome sequence-based typing of Acinetobacter baumannii. PLoS One 12:e0179228. doi: 10.1371/journal.pone.0179228
Holt, K., Kenyon, J. J., Hamidian, M., Schultz, M. B., Pickard, D. J., Dougan, G., et al. (2016). Five decades of genome evolution in the globally distributed, extensively antibiotic-resistant Acinetobacter baumannii global clone 1. Microb. Genom. 2:e000052. doi: 10.1099/mgen.0.000052
Hornsey, M., Ellington, M. J., Doumith, M., Thomas, C. P., Gordon, N. C., Wareham, D. W., et al. (2010). AdeABC-mediated efflux and tigecycline MICs for epidemic clones of Acinetobacter baumannii. J. Antimicrob. Chemother. 65, 1589–1593. doi: 10.1093/jac/dkq218
Karah, N., Jolley, K. A., Hall, R. M., and Uhlin, B. E. (2017). Database for the ampC alleles in Acinetobacter baumannii. PLoS One 12:e0176695. doi: 10.1371/journal.pone.0176695
Kim, D. H., and Ko, K. S. (2015). A distinct alleles and genetic recombination of pmrCAB operon in species of Acinetobacter baumannii complex isolates. Diagn. Microbiol. Infect. Dis. 82, 183–188. doi: 10.1016/j.diagmicrobio.2015.03.021
Krizova, L., Poirel, L., Nordmann, P., and Nemec, A. (2013). TEM-1 β-lactamase as a source of resistance to sulbactam in clinical strains of Acinetobacter baumannii. J. Antimicrob. Chemother. 68, 2786–2791. doi: 10.1093/jac/dkt275
Kuo, S. C., Chang, S. C., Wang, H. Y., Lai, J. F., Chen, P. C., Shiau, Y. R., et al. (2012). Emergence of extensively drug-resistant Acinetobacter baumannii complex over 10 years: nationwide data from the Taiwan Surveillance of Antimicrobial Resistance (TSAR) program. BMC Infect. Dis. 12:200. doi: 10.1186/1471-2334-12-200
La Scola, B., Gundi, V. A., Khamis, A., and Raoult, D. (2006). Sequencing of the rpoB gene and flanking spacers for molecular identification of Acinetobacter species. J. Clin. Microbiol. 44, 827–832. doi: 10.1128/JCM.44.3.827-832.2006
Lashinsky, J. N., Henig, O., Pogue, J. M., and Kaye, K. S. (2017). Minocycline for the treatment of multidrug and extensively drug-resistant A. baumannii: a review. Infect. Dis. Ther. 6, 199–211. doi: 10.1007/s40121-017-0153-2
Leite, G. C., Stabler, R. A., Neves, P., Perdigão Neto, L. V., Ruedas Martins, R. C., Rizek, C., et al. (2019). Genetic and virulence characterization of colistin-resistant and colistin-sensitive A. baumannii clinical isolates. Diagn. Microbiol. Infect. Dis. 95, 99–101. doi: 10.1016/j.diagmicrobio.2019.04.013
Martins, N., Picão, R. C., Adams-Sapper, S., Riley, L. W., and Moreira, B. M. (2015). Association of class 1 and 2 integrons with multidrug-resistant Acinetobacter baumannii international clones and Acinetobacter nosocomialis isolates. Antimicrob. Agents Chemother. 59, 698–701. doi: 10.1128/AAC.02415-14
Matos, A. P., Cayô, R., Almeida, L. G. P., Streling, A. P., Nodari, C. S., Martins, W. M. B. S., et al. (2019). Genetic characterization of plasmid-borne blaOXA-58 in distinct Acinetobacter species. mSphere 4:e000376-19. doi: 10.1128/mSphere.00376-19
Moffatt, J. H., Harper, M., Harrison, P., Hale, J. D., Vinogradov, E., Seemann, T., et al. (2010). Colistin resistance in Acinetobacter baumannii is mediated by complete loss of lipopolysaccharide production. Antimicrob. Agents Chemother. 54, 4971–4977. doi: 10.1128/AAC.00834-10
Müller, C., Stefanik, D., Wille, J., Hackel, M., Higgins, P. G., and Seifert, H. (2019). “Molecular epidemiology of carbapenem-resistant Acinetobacter baumannii clinical isolates and identification of the novel international clone IC9: results from a worldwide surveillance study (2012 - 2016),” in Proceedings of the Abstract Book of the 29th European Congress of Clinical Microbiology and Infectious Diseases, P0947, Amsterdam.
Mussi, M. A., Limansky, A. S., Relling, V., Ravasi, P., Arakaki, A., Actis, L. A., et al. (2011). Horizontal gene transfer and assortative recombination within the Acinetobacter baumannii clinical population provide genetic diversity at the single carO gene, encoding a major outer membrane protein channel. J. Bacteriol. 193, 4736–4748. doi: 10.1128/JB.01533-10
Nowak, J., Zander, E., Stefanik, D., Higgins, P. G., Roca, I., Vila, J., et al. (2017). High incidence of pandrug-resistant Acinetobacter baumannii isolates collected from patients with ventilator-associated pneumonia in Greece, Italy and Spain as part of the MagicBullet clinical trial. J. Antimicrob. Chemother. 72, 3277–3282. doi: 10.1093/jac/dkx322
Oikonomou, O., Sarrou, S., Papagiannitsis, C. C., Georgiadou, S., Mantzarlis, K., Zakynthinos, E., et al. (2015). Rapid dissemination of colistin and carbapenem resistant Acinetobacter baumannii in Central Greece: mechanisms of resistance, molecular identification and epidemiological data. BMC Infect. Dis. 15:559. doi: 10.1186/s12879-015-1297-x
Olaitan, A. O., Morand, S., and Rolain, J. M. (2014). Mechanisms of polymyxin resistance: acquired and intrinsic resistance in bacteria. Front. Microbiol. 5:643. doi: 10.3389/fmicb.2014.00643
Opazo-Capurro, A., San Martín, I., Quezada-Aguiluz, M., Morales-León, F., Domínguez-Yévenes, M., Lima, C. A., et al. (2019). Evolutionary dynamics of carbapenem-resistant Acinetobacter baumannii circulating in Chilean hospitals. Infect. Genet. Evol. 73, 93–97. doi: 10.1016/j.meegid.2019.04.022
Pagano, M., Martins, A. F., Machado, A. B., Barin, J., and Barth, A. L. (2013). Carbapenem-susceptible Acinetobacter baumannii carrying the ISAba1 upstream blaOXA-51-like gene in Porto Alegre, southern Brazil. Epidemiol. Infect. 141, 330–333. doi: 10.1017/S095026881200074X
Pagano, M., Nunes, L. S., Niada, M., Barth, A. L., and Martins, A. F. (2019). Comparative analysis of carbapenem-resistant Acinetobacter baumannii sequence types in Southern Brazil: from the first outbreak (2007-2008) to the endemic period (2013-2014). Microb. Drug Resist. 25, 538–542. doi: 10.1089/mdr.2018.0262
Pagano, M., Rocha, L., Sampaio, J. L., Martins, A. F., and Barth, A. L. (2017). Emergence of OXA-72-producing Acinetobacter baumannii belonging to high-risk clones (CC15 and CC79) in different Brazilian States. Infect. Control. Hosp. Epidemiol. 38, 252–254. doi: 10.1017/ice.2016.287
Poirel, L., Jayol, A., and Nordmann, P. (2017). Polymyxins: antibacterial activity, susceptibility testing, and resistance mechanisms encoded by plasmids or chromosomes. Clin. Microbiol. Rev. 30, 557–596. doi: 10.1128/CMR.00064-16
Polotto, M., Casella, T., Tolentino, F. M., Mataruco, M. M., Porto, N. K. M., Binhardi, M. F. B., et al. (2019). Investigation of carbapenemases and aminoglycoside modifying enzymes of Acinetobacter baumannii isolates recovered from patients admitted to intensive care units in a tertiary-care hospital in Brazil. Rev. Soc. Bras. Med. Trop. 53:e20190044. doi: 10.1590/0037-8682-0094-2019
Qureshi, Z. A., Hittle, L. E., O’Hara, J. A., Rivera, J. I., Syed, A., Shields, R. K., et al. (2015). Colistin-resistant Acinetobacter baumannii: beyond carbapenem resistance. Clin. Infect. Dis. 60, 1295–1303. doi: 10.1093/cid/civ048
Ramírez, M. S., Montaña, S., Cassini, M., and Centrón, D. (2015). Preferential carriage of class 2 integrons in Acinetobacter baumannii CC113 and novel singletons. Epidemiol. Infect. 143, 3118–3121. doi: 10.1017/S0950268815000060
Ramírez, M. S., and Tolmasky, M. E. (2010). Aminoglycoside modifying enzymes. Drug Resist. Updat. 13, 151–171. doi: 10.1016/j.drup.2010.08.003
Rodríguez, C. H., Balderrama Yarhui, N., Nastro, M., Nuñez Quezada, T., Castro Cañarte, G., Magne Ventura, R., et al. (2016). Molecular epidemiology of carbapenem-resistant Acinetobacter baumannii in South America. J. Med. Microbiol. 65, 1088–1091. doi: 10.1099/jmm.0.000328
Rodríguez-Martínez, J. M., Poirel, L., and Nordmann, P. (2010). Genetic and functional variability of AmpC-type β-lactamases from Acinetobacter baumannii. Antimicrob. Agents Chemother. 54, 4930–4933. doi: 10.1128/AAC.00427-10
Romanin, P., Palermo, R. L., Cavalini, J. F., Fávaro, L. D. S., De Paula-Petroli, S. B., Fernandes, E. V., et al. (2019). Multidrug- and extensively drug-resistant Acinetobacter baumannii in a tertiary hospital from Brazil: the importance of carbapenemase encoding genes and epidemic clonal complexes in a 10-year study. Microb. Drug Resist. 25, 1365–1373. doi: 10.1089/mdr.2019.0002
Royer, S., de Campos, P. A., Araújo, B. F., Ferreira, M. L., Gonçalves, I. R., Batistão, D. W. D. F., et al. (2018). Molecular characterization and clonal dynamics of nosocomial blaOXA-23 producing XDR Acinetobacter baumannii. PLoS One 13:e0198643. doi: 10.1371/journal.pone.0198643
Ruzin, A., Keeney, D., and Bradford, P. A. (2007). AdeABC multidrug efflux pump is associated with decreased susceptibility to tigecycline in Acinetobacter calcoaceticus-Acinetobacter baumannii complex. J. Antimicrob. Chemother. 59, 1001–1004. doi: 10.1093/jac/dkm058
Seifert, H., Dolzani, L., Bressan, R., van der Reijden, T., van Strijen, B., Stefanik, D., et al. (2005). Standardization and interlaboratory reproducibility assessment of pulsed-field gel electrophoresis-generated fingerprints of Acinetobacter baumannii. J. Clin. Microbiol. 43, 4328–4335. doi: 10.1128/JCM.43.9.4328-4335.2005
Stietz, M. S., Ramírez, M. S., Vilacoba, E., Merkier, A. K., Limansky, A. S., Centrón, D., et al. (2013). Acinetobacter baumannii extensively drug resistant lineages in Buenos Aires hospitals differ from the international clones I-III. Infect. Genet. Evol. 14, 294–301. doi: 10.1016/j.meegid.2012.12.020
Tavares, L. C. B., de Vasconcellos, F. M., de Sousa, W. V., Rocchetti, T. T., Mondelli, A. L., Ferreira, A. M., et al. (2019). Emergence and persistence of high-risk clones among MDR and XDR A. baumannii at a Brazilian teaching hospital. Front. Microbiol. 9:2898. doi: 10.3389/fmicb.2018.02898
Vasconcelos, A. T., Barth, A. L., Zavascki, A. P., Gales, A. C., Levin, A. S., Lucarevschi, B. R., et al. (2015). The changing epidemiology of Acinetobacter spp. producing OXA carbapenemases causing bloodstream infections in Brazil: a BrasNet report. Diagn. Microbiol. Infect. Dis. 83, 382–385. doi: 10.1016/j.diagmicrobio.2015.08.006
Vila, J., Ruiz, J., Goñi, P., and Jimenez de Anta, T. (1997). Quinolone-resistance mutations in the topoisomerase IV parC gene of Acinetobacter baumannii. J. Antimicrob. Chemother. 39, 757–762. doi: 10.1093/jac/39.6.757
Zander, E., Nemec, A., Seifert, H., and Higgins, P. G. (2012). Association between β-lactamase-encoding bla(OXA-51) variants and DiversiLab rep-PCR-based typing of Acinetobacter baumannii isolates. J. Clin. Microbiol. 50, 1900–1904. doi: 10.1128/JCM.06462-11
Keywords: Brazil, IC4, IC5, CHDL, outer membrane proteins, polymyxin resistance, nosocomial infection, two component systems
Citation: Nodari CS, Cayô R, Streling AP, Lei F, Wille J, Almeida MS, de Paula AI, Pignatari ACC, Seifert H, Higgins PG and Gales AC (2020) Genomic Analysis of Carbapenem-Resistant Acinetobacter baumannii Isolates Belonging to Major Endemic Clones in South America. Front. Microbiol. 11:584603. doi: 10.3389/fmicb.2020.584603
Received: 17 July 2020; Accepted: 04 November 2020;
Published: 30 November 2020.
Edited by:
Ayush Kumar, University of Manitoba, CanadaReviewed by:
Andres Felipe Opazo-Capurro, University of Concepcion, ChileCopyright © 2020 Nodari, Cayô, Streling, Lei, Wille, Almeida, de Paula, Pignatari, Seifert, Higgins and Gales. This is an open-access article distributed under the terms of the Creative Commons Attribution License (CC BY). The use, distribution or reproduction in other forums is permitted, provided the original author(s) and the copyright owner(s) are credited and that the original publication in this journal is cited, in accordance with accepted academic practice. No use, distribution or reproduction is permitted which does not comply with these terms.
*Correspondence: Carolina S. Nodari, Y2Fyb2wubm9kYXJpQGdtYWlsLmNvbQ==
Disclaimer: All claims expressed in this article are solely those of the authors and do not necessarily represent those of their affiliated organizations, or those of the publisher, the editors and the reviewers. Any product that may be evaluated in this article or claim that may be made by its manufacturer is not guaranteed or endorsed by the publisher.
Research integrity at Frontiers
Learn more about the work of our research integrity team to safeguard the quality of each article we publish.