- 1UCIBIO, Departamento de Ciências da Vida, Faculdade de Ciências e Tecnologia, Universidade NOVA de Lisboa, Caparica, Portugal
- 2Biomedical Research Centre, University of Granada, Granada, Spain
The pyogenic streptococci group includes pathogenic species for humans and other animals and has been associated with enduring morbidity and high mortality. The main reason for the treatment failure of streptococcal infections is the increased resistance to antibiotics. In recent years, infectious diseases caused by pyogenic streptococci resistant to multiple antibiotics have been raising with a significant impact to public health and veterinary industry. The rise of antibiotic-resistant streptococci has been associated to diverse mechanisms, such as efflux pumps and modifications of the antimicrobial target. Among streptococci, antibiotic resistance emerges from previously sensitive populations as result of horizontal gene transfer or chromosomal point mutations due to excessive use of antimicrobials. Streptococci strains are also recognized as biofilm producers. The increased resistance of biofilms to antibiotics among streptococci promote persistent infection, which comprise circa 80% of microbial infections in humans. Therefore, to overcome drug resistance, new strategies, including new antibacterial and antibiofilm agents, have been studied. Interestingly, the use of systems based on nanoparticles have been applied to tackle infection and reduce the emergence of drug resistance. Herein, we present a synopsis of mechanisms associated to drug resistance in (pyogenic) streptococci and discuss some innovative strategies as alternative to conventional antibiotics, such as bacteriocins, bacteriophage, and phage lysins, and metal nanoparticles. We shall provide focused discussion on the advantages and limitations of agents considering application, efficacy and safety in the context of impact to the host and evolution of bacterial resistance.
Introduction
The pyogenic group belonging to the genus Streptococcus includes species are habitually part of the flora of animals (including humans) and, as such, most species are regarded as commensal, but under fitting circumstances may cause localized and systemic infections (Nobbs et al., 2009; Peters, 2017). Species of the pyogenic streptococci group include Streptococcus pyogenes, Streptococcus agalactiae, Streptococcus dysgalactiae subsp. dysgalactiae (SDSD), and Streptococcus dysgalactiae subsp. equisimilis (SDSE) which, together with Streptococcus pneumoniae, are the key pathogens belonging to the genus Streptococcus (Parks et al., 2015). For example, S. pyogenes is the cause of numerous severe human diseases, including septicemia and streptococcal “toxic-shock” syndrome (Isaacs and Dobson, 2016). S. agalactiae is the most frequent cause of sepsis and meningitis in neonates and children (Rajagopal, 2009; Melin, 2011). Considering domestic animals, S. agalactiae is one of the main causes of bovine mastitis (Rato et al., 2013). SDSE was primarily considered a human commensal organism but nowadays its relevance as human pathogen is on the raising, causing a similar range of diseases in humans as does S. pyogenes (Brandt and Spellerberg, 2009). SDSD has been considered an animal pathogen and is frequently associated with bovine mastitis (Abdelsalam et al., 2013). Human infections associated with this subspecies have been sporadically reported (Koh et al., 2009; Park et al., 2012; Jordal et al., 2015), and its role in human disease remains unclear.
In recent years, severe outbreaks of infectious diseases caused by organisms resistant to multiple antibiotics have occurred. Drug resistance is mounting globally, threatening our capability to treat common infections, resulting in persistent illness and death. It is estimated that by 2050, around 10 million human deaths per year might be attributable to antimicrobial resistance (Neill, 2014, 2016). The increase in antimicrobial resistance is more frightening derived from the considerable narrow number of new antimicrobial agents currently under development (World Health Organization, 2020). The growing of resistance in bacteria has been associated to increased consumption of antimicrobials, and improper prescribing of antimicrobials, leading to selective pressure that trigger drug resistance in exposed bacteria and, consequently, in the persistence of antibiotic resistance genes in populations of the same ecological niches, mainly as a result of horizontal gene transfer (Fair and Tor, 2014). Indeed, high-throughput sequencing and other molecular genetics tools led to a better understanding of the underlying mechanisms of horizontal gene transfer. For instance, in average, about 20% of the fully sequenced genome of Streptococcus consists of mobile and exogenous DNA, comprising conjugative and composite transposons, phage regions, and plasmid (Lier et al., 2015; Yamada et al., 2019). Thus, horizontal gene transfer constitutes one of the leading modes of originating gene diversity which confers new antibiotic resistance mechanisms in Streptococcus. These gene transfer events frequently strike in the pyogenic group, particularly in S. pyogenes, S. agalactiae, Streptococcus canis, SDSD, SDSE, and Streptococcus uberis (Haenni et al., 2010; Richards et al., 2012; Wong and Yuen, 2012; Rohde and Cleary, 2016). Too, there have been reports of an increasing incidence of multiple drug resistance (MDR) among streptococci strains, which hamper customary empirical antimicrobial therapy for these infections. Still, even though pyogenic streptococci remain susceptible to most prescribed antibiotics, treatment failure due to MDR has also been reported both in human and veterinary patients (Doumith et al., 2017; Lai et al., 2017).
The quest for effective approaches to tackle MDR bacteria has put forward several alternatives, such as competitive exclusion of pathogenic bacteria via bacteriocin, and bacteriophages (Rotello et al., 2016; Furfaro et al., 2018; Lopetuso et al., 2019). The effectiveness of some of these new approaches for therapeutics is highly variable, but positive effects have been reported in some species. Irrespective of the mechanism of action, the ways bacteria seem to be able to develop resistance to these new approaches has not received enough attention, making it more difficult to find long-term solutions. Herein, we present an overview of mechanisms of resistance to antimicrobials in pyogenic streptococci, factors that contribute to antibiotic resistance and news approach to treating infectious diseases as an alternative to antibiotics, such as bacteriocins, bacteriophage and phage lysins, and nanoparticles. We shall provide focused discussion on the advantages and limitations of agents considering application, effectiveness, resistance development, and interactions with the immune system.
Antibiotics and Mechanisms of Resistance
An ideal antimicrobial ought to show high selective toxicity for bacteria with minimal adverse impact to the host (Kohanski et al., 2010). Antibacterial may be organized into four main clusters based on the mechanism of action and target in the bacterial cell – see summary in Table 1. Still, the mechanisms of resistance to antimicrobials are complex, and different mechanisms may be present in the same strain promoting a multidrug resistance phenotype, but whose main genotypic and phenotypic characteristics may be schematically grouped as shown in Figure 1. Some of these fundamental biochemical mechanisms of antimicrobial resistance include: (i) enzymatic inactivation of antibiotics, e.g., β-lactamases (Munita et al., 2016); (ii) modifications of the antimicrobial target preventing efficient binding of the antibiotic, which often results from spontaneous mutations, including genome and RNA variations (e.g., rRNA mutations associated to resistance to several antibiotics) (Malbruny et al., 2002; Gomez et al., 2017); (iii) preventing drug access to targets, for example through the reduced uptake by the cell via a decrease of outer membrane permeability in Gram-negative and/or active efflux pumps that increase clearance from within the cell (Petchiappan and Chatterji, 2017).
To fully realize the propagation of antibiotic resistance, one needs to recognize the molecular mechanisms of resistance to antibiotics and to map the resistome in different ecological niches. Several studies have assessed the resistome in the environment, namely in wastewater, soil, and gut microbiota of animals (humans included) (Pehrsson, 2016; Von Wintersdorff et al., 2016). Metagenomics directly analyze DNA in a biological sample, allowing for analysis of the resistome within distinct microbial ecosystems (Von Wintersdorff et al., 2016). These studies highlight that determinants of antibiotic resistance, including those clinically relevant, are prevalent in these environments (Lehtinen et al., 2019). Sequence-based studies provide large datasets, but one limitation is that they focus on genes already known to be involved in the resistance, or (less frequently) to predict new functions based on the homology to known sequences. These genome annotation schemes will provide more and more information to complement the output of functional metagenomics, which shall result in the identification of new determinants of antibiotic resistance (Von Wintersdorff et al., 2016).
In general, bacterial drug resistance can be divided into intrinsic and acquired resistance (Reygaert, 2018). Intrinsic resistance is a naturally occurring phenomenon, which prevents antimicrobial activity and it is common to the majority of strains of a given species. The intrinsic resistance may be constitutive, i.e., independent of previous antibiotic exposure (e.g., reduced permeability of the outer membrane), or induced via the exposure to antibiotic or environmental stress (e.g., multidrug efflux pumps and biofilm formation) (Baldassarri et al., 2006; Cox and Wright, 2013). Acquired resistance is due to chromosomal point mutations or by acquisition of mobile resistance genes, in which resistant strains emerge from previously sensitive bacterial populations, customarily subsequently to exposure to the antimicrobial (Haenni et al., 2010; Enault et al., 2017).
The acquisition of mobile genetic elements (MGEs), such as bacteriophages, plasmids, integrative and conjugative elements, is recognized as a key point in the emergence of multidrug-resistant (MDR) strains (Lehtinen et al., 2019). The main mechanisms of DNA uptake in bacteria are conjugation, transduction, and transformation (Figure 2), which must be followed by recombination to allow stable insertion into the chromosome. These MGEs are self-transmissible elements common in bacteria. Further to genes involved in mobility, regulation, or maintenance, MGEs convey antibiotic resistance genes and virulence factors, such as exotoxins (Haenni et al., 2010). Horizontal transfer of genes (HGT) can modulate host-pathogen interactions and extending the host range. Indeed, the use of high-throughput sequencing tools allowed for a better understanding of HGT. For example, in S. pyogenes the lateral exchange of virulence genes, mediated by bacteriophage infection, is a very important factor in the diversification of the species. What is more, bacteriophages may convey genes that provide for selective advantage to the host, thus fostering their own dissemination (Colomer-Lluch et al., 2011; Von Wintersdorff et al., 2016).
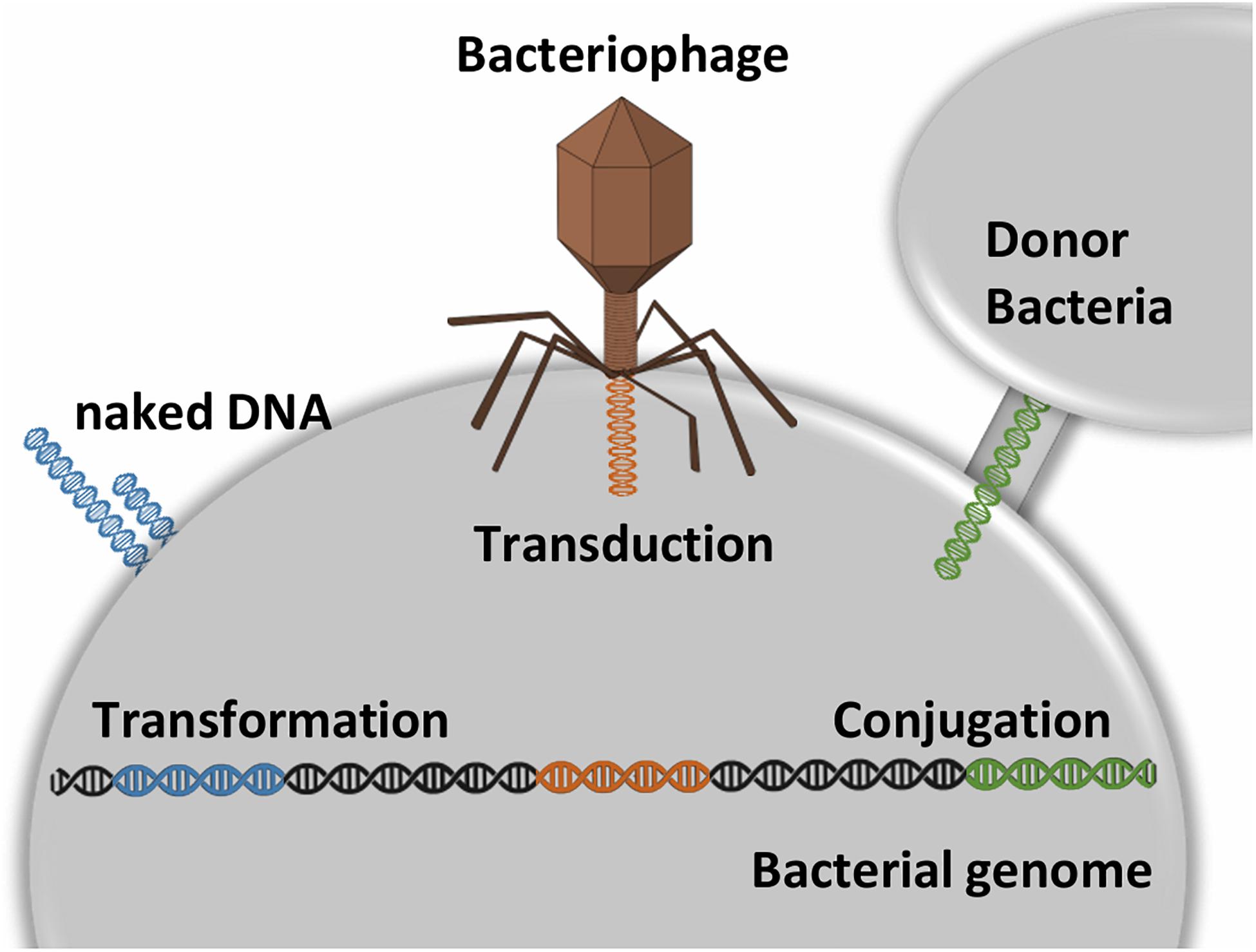
Figure 2. Transformation is the process by which naked DNA from the external environment is incorporated into a bacterial cell. For this process is requires the recipient cell to exhibit on its membrane special DNA binding proteins. Transduction is the process by which a phage transfers DNA from one bacterial strain to another. Conjugation is the process mediated by cell-to-cell contact that provides direct DNA transfer. Conjugative transfer systems associated with plasmids usually code the necessary proteins to DNA exchange. The plasmids are kept as extra-chromosomal genetic material by external selective pressure (e.g., presence of metal or antibiotic). Overall, these mechanisms can be followed by recombination events that allow the genetic determinants to be inserted stably into the chromosome.
Many determinants of resistance are frequently present on a single R plasmid (harboring several antibiotics-resistance genes), thus, multiple resistance can be shared among bacteria in single-event of conjugation (Nikaido, 2009). Many of these R plasmids contain resistance genes against the main classes of antibiotics, such as aminoglycosides, macrolides, phenicol, and tetracycline (Nikaido, 2009).
Streptococcus harbor various plasmids associated with the transfer of antibiotic resistance and virulence (Grohmann et al., 2003; Cook et al., 2013). In addition to plasmids, a wide variety of transposons have been isolated in streptococci (Brenciani et al., 2007; Fléchard and Gilot, 2014), namely Tn3-family transposons, composite and conjugative transposons. For example, Tn916, encoding tetM for the ribosomal protection protein TET(M), associated to independent transfer of resistance between a multitude of strains via a plasmid, including Enterococcus faecalis, Staphylococcus aureus, S. pneumoniae, S. agalactiae, and SDSD (Franke and Clewell, 1981; Haenni et al., 2010; Fléchard and Gilot, 2014; Osei Sekyere and Mensah, 2019), that act as reservoirs of functional antibiotic resistance genes.
Several mechanisms of antibiotic resistance among pyogenic streptococci have been reported, whose main mechanism of action and associated resistances shall be briefly described. Table 2 summarizes the main antibiotics used for the treatment of streptococcal infections and resistance mechanisms.
β-lactams, targeting the bacterial cell wall peptidoglycan, particularly enzymes linked to peptidoglycan synthesis, are one of the most prescribed antibiotics for streptococcal infections due to the broad spectrum of action (Kohanski et al., 2010; Kong et al., 2010). β-lactamases are secreted enzymes capable of destroying these antibiotics and are the most frequent cause of resistance, but not the only (Bush and Jacoby, 2010; Munita et al., 2016). In fact, pyogenic streptococci have been recognized as non-β-lactamase-producing bacteria, where resistance to β-lactams is essentially mediated by alterations to the binding site of penicillin-binding proteins (PBPs) (Vannice et al., 2019). Nevertheless, a recent study based on whole-genome sequencing revealed the presence of β-lactamases determinants of S. uberis and SDSD isolates bovine mastitis (Vélez et al., 2017). Still, there is a need for actional studies to assess the potential of these β-lactamases, and the role of these species as a reservoir of determinants of resistance.
Macrolides are the first choice against streptococcal infections in patients allergic to β-lactam (Kanoh and Rubin, 2010) and, clindamycin (lincosamides) has been used for the treatment of infections associated with anaerobic bacteria as an alternative to penicillin G (Greenwood and Irving, 2012). Three main mechanisms have been associated with resistance to these antibiotics: (i) target modification by methylation of rRNA (erm genes) or target mutations, (ii) active efflux, and (iii) enzymatic inactivation (Matsuzaki et al., 2005; Petinaki and Papagiannitsis, 2018). Although most streptococci strains remain sensitive to macrolides and lincosamides, resistance phenotypes have emerged among pyogenic streptococci (Rato et al., 2013; Cattoir, 2016; De Greef et al., 2019).
Since active electron transport is required for aminoglycoside uptake into bacteria, aminoglycosides have weak activity against anaerobic bacteria (Ramirez and Tolmasky, 2010; Krause et al., 2016). Still, low levels of resistance to aminoglycosides are observed in most Streptococcus spp., and high-level resistance to aminoglycosides appears to be rare. This resistance occurs due to the production of AAC(6′)-APH(2′′), APH(3′)-IIIa, and ANT(6)-Ia enzymes and has been demonstrated to be transferable by conjugation (Cattoir, 2016).
Chloramphenicol-resistant streptococci are not common even though some studies show high levels of resistance among the pyogenic group species, namely, S. pyogenes, S. agalactiae, and SDSE (Trieu-Cuot et al., 1993; Schwarz et al., 2004; Woodford, 2005). Among streptococci and other Gram-positive bacteria, the resistance to chloramphenicol is mainly mediated by Chloramphenicol O-acetyltransferase (CAT) enzymes encoded by plasmids or chromosomally integrated. Several CATs are shared by streptococci, staphylococci, and enterococci strains (Woodford, 2005).
Currently, fluoroquinolones (FQ) have also been put forward as a therapeutic option for the treatment of streptococcal infections (Pinho et al., 2010). However, emergence of resistance among several streptococcal species, including SDSE, S. pyogenes, and S. agalactiae, S. pneumoniae, and viridans group streptococci has been reported (Guerin et al., 2000; Martinez-Garriga et al., 2007; Duesberg et al., 2008; Pinho et al., 2010; Pires et al., 2010; Kimura et al., 2013; Dang et al., 2014; Arias et al., 2019). The most frequent mechanism of high-level FQ resistance is the target modification due to mutations in parC and gyrA genes that occur mainly in quinolone resistance-determining regions (QRDRs) (Hooper and Jacoby, 2016; Pham et al., 2019). Resistance to FQ can also be mediated by modifying enzymes, target-protection proteins (Pham et al., 2019) and by increased production of multidrug-resistance efflux pumps (Hooper, 2002).
Resistance to tetracyclines (TET) among streptococci strains is often found in high rates (Nakamur et al., 2011; Emaneini et al., 2014; Gherardi et al., 2014; Vélez et al., 2017; Gizachew et al., 2019). In streptococci, genes encoding resistance to TET are frequently acquired by MGEs, which also harbor erythromycin resistance determinants (Brenciani et al., 2004, 2007, 2011; Emaneini et al., 2014; Cattoir, 2016). The presence of determinants of tetracycline resistance (tet genes) in conjugative transposons, which can efficiently translocate among related bacteria, may explain the high prevalence of resistance (Santoro et al., 2014). There is a significant association between tetM and ermB (genetic determinant for erythromycin resistance) that has been identified among the strains of pyogenic streptococci, and it can be co-transferred among S. agalactiae and S. pyogenes strains (Brenciani et al., 2007; Emaneini et al., 2014). There is also evidence of the linkage between tetO and ermTR/mefA genes (Giovanetti et al., 2003) and lysogenic transfer of these genes carried by Φ m46.1 among S. pyogenes (Di Luca et al., 2010), that contribute to a multi-resistant phenotype.
Due to the rise of pathogens resistant to multiple antibiotics, new strategies have been proposed as an alternative to conventional antimicrobials. One such example is the use of as phage-derived lysins that degrade peptidoglycan (Maciejewska et al., 2018), which may be considered as an alternative to β-lactams, or of bacteriocins that provide a more targeted approach, i.e., strain- or species-specific (Nigam et al., 2014; Matsumoto-Nakano, 2018; Hols et al., 2019). Another emerging field of research has been the use of nanoparticles, particularly metallic nanoparticles (e.g., gold and silver), as direct antimicrobial agents, as drug delivery systems that improve the pharmacokinetics parameters (Masri et al., 2019a), or taking advantage of these nanostructures’ optical properties, e.g., photothermal ablation of cells. The potential of these new approaches against streptococci shall be further discussed in the following sections.
Biofilms and Antimicrobial Resistance
Generally, bacteria populations may strive as planktonic, i.e., freely existing in solution, and/or sessile forming a biofilm. Biofilms are defined as tri-dimensional agglomerations of cells, attached to biotic or abiotic surfaces, and encased in a self-produced matrix composed by extracellular polymeric substances (Jamal et al., 2018). Their formation might be induced by environmental changes that cause stress cells, such as nutrient limitation and antimicrobial agents (Garrett et al., 2008; Kumar et al., 2017).
In humans, biofilms account for up to 80% of bacterial infections, according to the United States National Institutes of Health (Khatoon et al., 2018). One of the most important characteristics of biofilms is their ability to increase bacterial tolerance to antimicrobial agents. Biofilms protect the microorganism not only from antimicrobial agents but from nutrients scarcity, mechanical forces, and from the host’s immune system. Several in vitro studies demonstrated that bacterial biofilm could become 10 to 1,000 times more resistant to the effects of antimicrobials as their planktonic counterparts (Melchior et al., 2006). Therefore, biofilm formation should be considered as a core mechanism of resistance since it increases treatment failure and promotes persistent infection.
Biofilm growth of streptococci has been extensively investigated, but insights in the genetic origin and mechanisms of biofilm formation in this genus are limited. Although most pyogenic streptococci are able to form biofilms, there is substantial heterogeneity among strains in the strength of adherence to different surfaces. Like most bacterial genera, in streptococci biofilms, a gradient of nutrients, waste, and signaling molecules are formed, thus allowing groups of cells to adapt to different environments within the same biofilm, which may be growing at a different rate. Besides that, studies show that a biofilm-specific phenotype is stimulated in a particular subpopulation, resulting in the differential expression of mechanisms against the antimicrobials (Konto-Ghiorghi et al., 2009; Genteluci et al., 2015). Even though the resistance associated to streptococci biofilms are not entirely understood, several mechanisms have been proposed in support of increased resistance to antimicrobials. These mechanisms result from of the multicellular nature of biofilms, which leads to an additive (or synergistic) effect between the biofilm community’s protection and the conventional mechanisms of resistance referred above (Rosini and Margarit, 2015; Young et al., 2016).
Formation of biofilms also favors horizontal gene transfer between community members, thus provides conditions for the uptake of resistance genes, e.g., high cell density or accumulation of genetic elements. Some studies suggest that conjugation is more efficient in biofilms than in planktonic cells (Van Meervenne et al., 2014; Kragh et al., 2016). Marks et al. (2014) demonstrated that the biofilm microenvironment of S. pyogenes populations results in the induction of competence genes; therefore, it is more conducive to HGT. This study shows for the first time that S. pyogenes can be naturally transformed when grown as biofilms.
Overall, upon biofilm formation, there is a delayed internalization of the antimicrobial through the biofilm matrix, as the primary physical and/or chemical diffusion barrier prevents the entrance of polar and charged antibiotics. Additionally, the heterogeneous growth of the biofilm cells and activation of the stress response genes contribute to the resistance phenotype.
The extracellular polymeric substances (EPS) matrix composition is essential for the properties of the biofilm since it offers cohesion and three-dimensional architecture of biofilms (Flemming and Wingender, 2010). The EPS matrix compose 80% of the biofilm containing alginates, poly-N-acetyl glucosamine, extracellular teichoic acid, proteins, lipids, nucleic acids, phospholipids, polysaccharides, and extracellular DNA. EPS is 97% of water, which is found as a solvent, dictating viscosity, and mobility (Flemming and Wingender, 2010; Kumar et al., 2017; Jamal et al., 2018). For certain compounds, it is known that the EPS matrix represents an initial barrier, but recent studies showed that the biofilm matrix does not form an impermeable barrier to the diffusion of antimicrobial, and other mechanisms can contribute to promoting biofilm cell survival (Trappetti et al., 2011).
Several reports indicate that the extracellular matrix of pyogenic streptococcal biofilms is rich in proteins (Genteluci et al., 2015; Young et al., 2016; Alves-Barroco et al., 2019). In some cases, the biofilm contains a large amount of mucus-like extracellular component, probably formed by DNA released from dead cells (Alves-Barroco et al., 2019). A role for extracellular DNA was also demonstrated by the reduction of biofilms formed by SDSE isolates after treatment with DNase I (Genteluci et al., 2015). The addition of a carbohydrate oxidant, such as sodium metaperiodate, to the biofilm of SDSE indicated the presence of an exopolysaccharide, like for Streptococcus mutans biofilms (Liao et al., 2014) and Streptococcus intermedius (Nur et al., 2013). Doern et al. (2009) examined S. pyogenes strains from different clinical sources and demonstrated the requirement for protein and DNA in the matrix of biofilm, and only passive role for carbohydrates. This is in contrast to SDSE, for which several polysaccharides have been shown to be required (Genteluci et al., 2015).
Overall, the nature of the biofilm matrix depends on the microbial cells, their physiological status, the nutrients available, and the physical conditions. The composition of the EPS matrix likely influences the resistance against different antimicrobial classes. Responses to specific stress sources such as nutrient limitation the bacterial cell slow its growth. During biofilm development, a gradient is established, in which outer layers are metabolically active and aerobic, while and the more inner layers are anaerobic with the reduced growth rate. This slow growth has been observed in streptococci biofilms that are frequently accompanied to a significant increase in antibiotics resistance (Bjarnsholt et al., 2013; Macià et al., 2014). Several antibiotics, such as aminoglycosides, β-lactams, and fluoroquinolones, do not seem to be active in anaerobic conditions, affecting only the outermost layers of the biofilm (Borriello et al., 2004). Cell-wall active antibiotics, namely, β-lactams and glycopeptide, have minimal activity against bacteria that are not replicating and are metabolically inactive (Del Pozo, 2018).
Clinical strains response to most antibiotics is assessed according to standard MIC determination. However, several studies have indicated that, as a biofilm, the same strain/isolate may be resistant, suggesting that most of the antibiotics evaluated would be ineffective in therapy. Still, information regarding the minimum concentration for biofilm eradication of pyogenic streptococcal is scarce (Conley et al., 2003; Baldassarri et al., 2006).
Biofilm formation of S. pyogenes protects against some drug but does not confer complete resistance to some antibiotics, namely, penicillin and fluoroquinolone (Conley et al., 2003; Baldassarri et al., 2006; Young et al., 2016). Therapeutic failures against infections caused by S. pyogenes may be due to the ability to internalize human cell and biofilm formation facilitating the persistence of genetically susceptible organisms, additionally supporting the HGT, and consequently, the emergence of virulent clones (Baldassarri et al., 2006). The increased resistance of biofilms to antibiotics was also observed in SDSD and S. agalactiae (Mah and O’Toole, 2001; Olson et al., 2002).
As explained above, the successful treatment of infections caused by biofilm-forming bacteria is troubled due to the multidrug-resistant phenotype. Conventional antimicrobial therapy is unable to eradicate the biofilm infection. Consequently, to fight the resistance of bacterial biofilm, several different strategies and antibiofilm agents have been proposed. A promising strategy is the application of nanoparticles, which have been considered as an alternative approach to combat and biofilm-based infections (Baptista et al., 2018). Applications of nanomedicine and other alternative therapies will be discussed below.
Alternative Antibacterial Therapies
In order to tackle the growing MDR concerns, a plethora of alternative compounds, strategies and platforms has been proposed as an alternative to conventional antimicrobials. Some of these alternatives are mere concepts whose promising in vitro efficacy has been the focus of attention. Many of these novel solutions have been proposed to be used alone against MDR bacteria, but many other have been proposed to be used in combinatory strategies with traditional antibacterial drugs to enhance efficacy, circumvent the onset of mechanisms of resistance.
Bacteriocins
Bacteriocins are peptides, of prokaryotic origin, with inhibitory activity against diverse groups of microorganisms (Nigam et al., 2014; Hols et al., 2019). Several authors have documented the ability of numerous bacteriocins to inhibit the growth of pathogenic microorganisms. Here we shall refer to a general representation of bacteriocins as an alternative to traditional antibiotics. Overall, bacteriocins interact with the bacterial cell membrane and alter its properties, causing cell death. These molecules normally only target closely related species, and given their bactericidal or bacteriostatic effects, they can offer an advantage relative to conventional antibiotics since treatment could be targeted against specific pathogenic (Lopetuso et al., 2019). These peptides are typically used by commensals microbiota to colonize in the human gastrointestinal tract allowing the survival of specific communities, and thus improving gut barrier function and host immune response (Hols et al., 2019). Four major classes of bacteriocins have been identified: (i) Class I, including small heat-resistant peptides, modified post-translationally, known as “lanthionine-containing bacteriocins” (e.g., lantibiotics, sactipeptides, and glycocins); (ii) Class II, including small heat-resistant peptides (<10 kDa) post-translational modifications. These are “non-lanthionine-containing bacteriocins” which are divided into four subclasses based on their size; (iii) Class III harboring heat-labile and large proteins (>30 kDa); and (iv) Class IV including complex bacteriocins, namely, large proteins with carbohydrate and/or lipid (Pieterse and Todorov, 2010; Hols et al., 2019).
Widespread applications of bacteriocins have been documented with variable efficacy reports. There has been some experimental evidence supporting the antimicrobial properties of bacteriocin nisin (produced by Lactococcus) against relevant oral pathogenic bacteria. It has been shown that nisin A could inhibit the growth of cariogenic streptococci, including Streptococcus gordonii, Streptococcus sanguinis, Streptococcus sobrinus, and S. mutans (Tong et al., 2010). Additionally, it was demonstrated that the nisin associated with poly-lysine and sodium fluoride can inhibit the formation of S. mutans biofilms (Tong et al., 2011).
Among bacteriocins used against bovine mastitis, besides the nisin, the lacticin3147 has largely been researched. This bacteriocins has proved effective against the most mastitis-causing pathogens, namely S. aureus, SDSD, S. agalactiae and S. uberis (Ryan et al., 1996, 1998). Studies have shown that bacteriocins produced by several streptococci to be able to inhibit closely related strains (Nigam et al., 2014; Matsumoto-Nakano, 2018; Hols et al., 2019). Some S. mutans and Streptococcus salivarius strains that are part of the commensal microbiota of the oral cavity are also producers bacteriocin producers (Tagg, 2004; Tagg et al., 2006). Healthy microbiota of the nasopharynx also harbors bacteriocin-producing strains, including S. salivarius strains. The bacteriocins produced by this species have been investigated for the treatment of pharyngitis and otitis (Walls et al., 2003). In order to shield against streptococcal infections, bacteriocin-producing strains are inoculated in the nasopharynx (Walls et al., 2003). The ability of normal microbiota strains to inhibit the growth of other bacteria has a critical role in its colonization of the host and suggest that these bacteriocins provide protection against S. pyogenes infection (Wescombe et al., 2012).
To date, few streptococci bacteriocins against mastitis-causing pathogens have been identified. However, the natural environment of bacteriocin-producing bacteria consists of a particular field for application. S. uberis strains isolated from bovine mastitis bacteriocin-producing has been described, the most studied is the nisin U. This bacteriocin showed activity against important mastitis-causing pathogens, specifically E. faecalis, SDSD and S. agalactiae (Wirawan et al., 2006).
Larger bacteriocins (above 10 kDa) also produced by some streptococci strains and are identified as bacteriolytic enzymes or non-lytic inhibitory. Examples comprise streptococcin A-M57 produced by S. pyogenes and dysgalacticin provided by SDSE. The genes that encode for SA-M57 (scnM57) and dysgalacticin (dysA) have been found on plasmids pDN571 and pW2580, respectively (Heng et al., 2006). The DysA and ScnM57 are polypeptides with 220 and 179 amino acids, respectively, both are exported via the Sec-dependent transport pathways. Interestingly, a pW2580-like plasmid is also harbored by some S. pyogenes strains, emphasizing the HGT between SDSE and S. pyogenes (Heng et al., 2006). Overall, lateral transfer of bacteriocin production underscores the contribution of the microbial ecology within the specific niche.
Nonetheless, the broad use of bacteriocin can also confer threatening for its usage on a large scale. Usually, bacteriocin resistance is acquired by lateral transfer of the immunity gene harbored in bacteriocin-producing strain. Resistance genes located on MGE can facilitate the transfer to closely related or even different species providing the means to resist specific bacteriocins (Dicks et al., 2018).
Multidrug efflux pumps also provide resistance to bacteriocins of several bacterial species (Van Hoang et al., 2011). Furthermore, bacteriocins may be degraded by proteolytic enzymes; consequently, they may not be as stable as conventional antibiotics (Tolinački et al., 2010).
Bacteriophage and Phage Lysins
Bacteriophages (or only phages) are viruses that specifically infect bacteria. The interaction between phage and bacteria usually involves particular receptors located in the cell membranes. Therefore, the phage is a natural killer of bacteria (Ghosh et al., 2019; Lopetuso et al., 2019). For this reason, the bacteriophages and phage proteins, namely enzymes, are extensively studied as a future alternative against bacterial infections.
There are many types of phage viruses, but the vast majority of phages can be distinguished into lytic and temperate. The most common approach for therapy involves lytic phages, which are phages that induce cell lysis, and therefore cause bacterial death (Ghosh et al., 2019), whereas the temperate phages integrate within the host genome (lysogenic conversion) (Di Luca et al., 2010). Typically, in the lytic phage life cycle, after the interaction between tail fibers and the host cell surface receptors, the phage secretes specific enzymes that degrade lipopolysaccharide, peptidoglycan and outer membrane (in Gram-negative) to inject the phage DNA. Subsequently, late genes are expressed and take control of the host cell’s to then initiate phage DNA replication. The phage DNA replicated expresses genes that encode proteins necessary for new phage particle assembly, endolysins, and holins for host cell lysis. Finally, the new phage particles are released into the environment.
The most significant factor ensuring the efficacy of phage therapy is its self-replicating nature, which distinguishes them from conventional antibiotics. Therefore, the main advantage of using phages for antibacterial treatment is that it can be administered in a low dose, that is, a small number of phages allows producing more of the particles at the infection site (Maciejewska et al., 2018).
Since their discovery in 1915 by Frederick William Twort, the phages were recognized as potential antibacterial, and due to the facility of administration and absence of side effects, phages were used immediately for antibacterial therapy (oral and topical preparations) (Maciejewska et al., 2018). The discovery and introduction of penicillin in the 1940s led to the practically total abandonment of antibacterial therapy with phage in the western countries (de Almeida and Sundberg, 2020). However, the benefits of antibiotics were lost considerably with the emergence and dissemination of bacterial resistance. The emergence of infectious diseases caused by multidrug-resistant bacterial generated an essential need for alternatives approaches to traditional antibiotics (Chang et al., 2015; Lehtinen et al., 2019). Along these lines, bacteriophages and phage-derived protein therapy get revitalized.
Since the 1980s, the phage therapy revival in western countries has been considered a possible option for combat antimicrobial resistance (de Almeida and Sundberg, 2020). Many research groups have concentrated on this theme of increasing importance, with Belgium pioneering in studies for the clinical use of phages (Pirnay et al., 2018; Jault et al., 2019). Despite the large potential of phages for antibacterial therapy, a small number of clinical trials have been performed in human patients. Besides that, few clinical trials are accepted by public health authorities, for example, the European Medicines Agency (EMA) and the Food and Drug Administration (FDA) (Rios et al., 2016). In the United States and European Union, the phages and phage-encoded enzymes classified as human therapeutic material are subjected to the same implementation regulations as traditional antibiotics (Maciejewska et al., 2018).
There has been a growing interest in phage-derived enzymes with antibacterial activity, including lysins (degrade peptidoglycan), and depolymerases (that degrade polysaccharide, e.g., capsule, biofilm matrix, and lipopolysaccharide) (Maciejewska et al., 2018). Regarding the application of these enzymes, previous studies, including animal models and clinical trials, showed antibacterial activity and reaffirmed the safety of its use (Lopetuso et al., 2019). Nonetheless, the current legislation limits the use of recombinant enzymes in human therapy, mainly for systemic therapy (Schmelcher et al., 2012).
The potential of phage against biofilm-forming bacteria has been demonstrated. The ability of the bacteria to produce biofilms has been considered the most common reason for failure therapeutic of antibiotics due to the impermeability of the biofilm matrix and the diversity of bacterial cells at different metabolic stages. Studies show that some phages have naturally depolymerases able to degrade the biofilm matrix (Abedon, 2015b). Probably, the depolymerases have evolved in response to polysaccharide of the biofilm matrix that covers the membrane receptor required for the interaction between the phage particle and the host cell and the subsequent attachment. The phages can also infect metabolically inactive bacteria of the biofilm since the receptor is present, but the lytic cycle stays pendent until bacterial metabolism to be active (Pearl et al., 2008). The complete eradication by one phage is rather difficult due to a complex structure of the mature biofilm. A combined action (combined therapy) has been suggested as a valid approach, in which depolymerase that degrades polysaccharides of the matrix allowing the phage or antibiotics to achieve the bacteria (Abedon, 2015a). Phage lysins have been also effectively used to remove bacterial biofilms (Meng et al., 2011; Shen et al., 2013; Rico-Lastres et al., 2015).
Bacteriophages infection occurs through specific protein receptors on the bacterial surface, which is the cause of extreme selectivity of these agents but also their main limitation. Due to this high specificity, phage therapy is of narrow-spectrum compared to traditional antibiotics whose targets are general pathways and processes common to most bacteria (e.g., protein synthesis). Unlike traditional antibiotics, one particular phage has a restricted number of strains as target, in other words, several and different phages are required to combat one only bacterial species. Moreover, phage-based therapy requires the previous identification of bacteria, causing infection to the isolation of specific lytic phage. Methods to isolate bacteriophages with broad-host-range and modifications to expand the specificity have been the target of several approaches, which could reduce the number of phages needed per species (Fairhead et al., 2017; Hyman, 2019). Moreover, the use of a “phage-cocktail” (composed of strictly lytic phages) can expand the spectrum of action and be administered in combination with other antibacterial agents, thus, increasing the potential of phage therapy.
The success of antibacterial phage-based therapy broadly depends on the patient’s immune system (PIS) that may recognize and inactivate viral particles. A low-level of antibodies specific to several viral proteins can naturally exist. Moreover, during phage therapy, the activation mechanisms of the immune response can be triggered; thus, phage may be recognized by the PIS, severely compromising the therapeutic effectiveness (Borysowski et al., 2012).
The activity of antibodies in phage lysines inactivation has also been investigated. Studies have shown that antibodies were effective in reducing the half-life of these enzymes (Rashel et al., 2007; Jun et al., 2014). Nonetheless, modification of lysins by dimerization to broaden their half-life has been investigated (Resch et al., 2011). For example, the ClyS (chimeric endolysin) that showed insensitive to antibodies (Pastagia et al., 2011).
Furthermore, phage particles may undergo denaturation by conformational changes irreversible or reversible. A proposed solution is encapsulation within nanocarriers to become the particles insoluble and protecting them from the digestive and immune system (Balcão et al., 2014; Rios et al., 2018). Another critical question is to get the phage particles to the infection site, given that phages do not have pharmacokinetic properties (Ghosh et al., 2019). One of the biggest concerns of phage-based therapy is the gap in understanding of phage-bacteria-human interaction, namely their safety. Concerning phage therapy in immunocompromised patients, although considered safe, its use may be less effective with more associated risks (Roach et al., 2017).
Another aspect associated with security difficulties is horizontal gene transfer. Although low, there is the possibility of HGT affect the pathogenic potential of co-existing bacterial strains by sharing of antibiotic resistance and virulence genes into the population (Lim et al., 2014). The proper phage selection against a given infection still is a challenging question. Moreover, clinical phage resistance in vivo also is a complicated issue.
Recent studies in animal models suggest that bacterial mutations resulting in phage-resistance may enhance the pathogen’s fitness in its regular niche within the host (Oechslin, 2018). Experimental data showed that phage-resistance occurs in 80% of researches targeting the intestinal environment and 50% of investigations with a model of sepsis. In human studies, phage-resistance has also been observed.
In the pyogenic streptococcus group, strains can escape to phage attack through several mechanisms, comprising spontaneous mutations of the genes encoding receptor, restriction-modification systems, abortive infection mechanisms, and adaptive immunity mediated by CRISPR-Cas systems (Almeida et al., 2016; Euler et al., 2016). The spontaneous mutation is the principal mechanism emergence of resistance and phage–bacterial coevolution. The mutations may provide resistance by changing the bacterial surface molecules in particular phage receptors, and that also determine phage specificity.
Concerning modification-restriction mechanisms, it is based on its abilities to restrict incoming foreign genetic material and to protect host DNA from restriction through modification (methylation, for example) of specific bases in the DNA sequence. Due to host DNA modification, unmodified sequences are then assumed to be foreign and thus cleaved by restriction endonuclease (Stern and Sorek, 2011). Usually, this mechanism causes the death of phage particles but preserves the host. If the system fails, intruding phages will be replicated and modified by the cell, becoming resistant to restriction. In abortive infection (Abi), the host mechanisms arrest phage development at its different steps, e.g., phage transcription, genome replication, or phage genome assembly. Abi mediated resistance ultimately results in the death of both the bacteriophage and the host. It is a selfless defense mechanism since the host dies, but the surrounding population is benefitted (Stern and Sorek, 2011). Although some of these systems work similarly to toxin-antitoxin systems, Abi systems are vastly diverse, and their modes of action are still not completely understood.
The CRISPR-Cas system consists of a multistep process by which small fragments of foreign nucleic acids (or protospacers) are first recognized and included in the host genome. Afterward, these fragments (or spacers), along with Cas proteins, are used as an adaptive immune system that recognizes, degrades or silences foreign nucleic acids (Bondy-Denomy et al., 2013; Rath et al., 2015). However, phages have acquired mutation-based strategies to evade CRISPR/Cas systems, e.g., losing their spacer sequences or encoding products that target Cas proteins (Stern and Sorek, 2011).
Nanoparticles
Nanomaterials have recently gained great interest due to the variety of applications in biomedicine (McNamara and Tofail, 2017). Nanomaterials comprise a range of constructs, materials and functional systems of particles whose size is between 1 and 100 nm. Particularly, these nanotechnology-based materials have found plenty of applications as alternative tools to traditional antibiotics and, more interestingly, as means to prevent the surge in antibiotic resistance (Baptista et al., 2018). The use of nanoparticles as antibiotic therapy has relied on these nanostructures acting as carrier of drugs, either via integration or incorporation into the nanoformulation, or adsorbed to the surface so as to improve biodistribution and pharmacokinetics, e.g., solubility, controlled drug liberation and therapeutic effectivity (Gao et al., 2018), the main mechanism of action of metallic nanoparticles in bacteria are described in Figure 3. However, several nanomaterials have been proposed as antimicrobials, with particular emphasis metallic nanoparticles (the most recent application of these metallic nanoparticles against Streptococcus spp. are shown in Table 3).
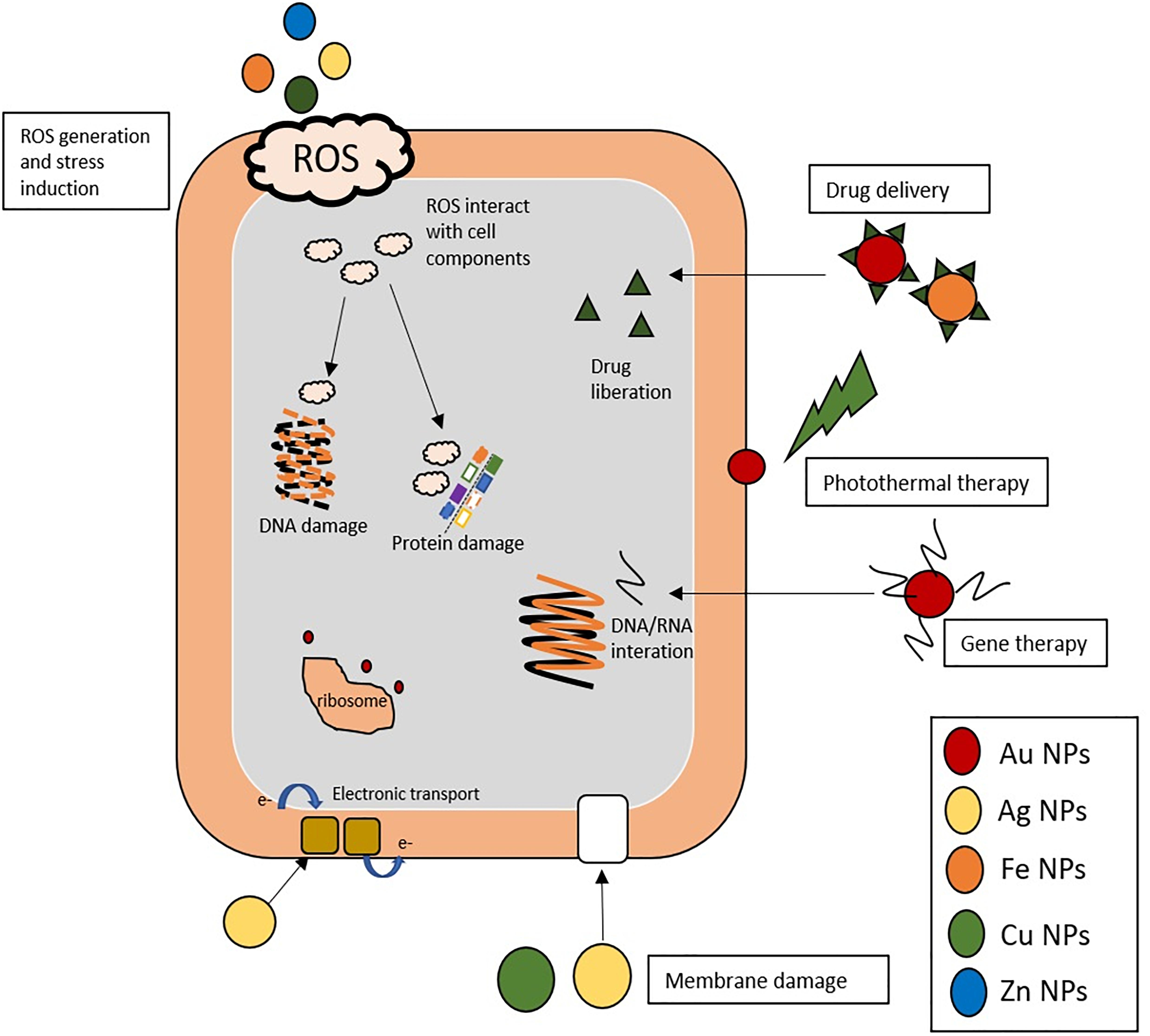
Figure 3. Different mechanism of action of metallic NPs in bacteria. Nanoparticles induce wide effects in bacterial metabolism by different approaches: (i) ROS generation: Ag, Fe, Cu, and Zn NPs induce ROS (reactive oxygen species), the ROS generated are highly reactive toward biological molecules such as proteins and DNA and interact and damage them. (ii) Damage membrane: Ag and Cu NPs interact with chemical groups of bacterial membrane (sulfate or phosphate) and disturb the normal functions. (iii) Drug and gene delivery systems: Au and Fe NPs could be carrier of gene moieties (DNA/RNA) that interact with bacterial gene, or deliver drug improving some pharmacodynamic parameters. (iv) Ribosome: Au NPs inhibit the union of transfer RNA (tRNA) to ribosome. (v) Photothermal therapy of Au NPs mediated by laser irradiation that disturb the membrane structure. (vi) Bacterial respiration: Ag NPs alter the electronic transport and inhibit the respiratory chain.
Some of the value of nanotechnology for antimicrobial therapy relate to modulation of the pharmacokinetic profile, where nanoparticle mediated drug delivery might improve conveyance of the drug to the desired tissue. Furthermore, nanoparticles could be designed to enhance solubility, control the release of drug and increase clearance from the organism, thus improving the therapeutic window of the cargo drug. Also, some synergistic strategies may be used, such as photothermal ablation of cells, where combination to the “traditional” chemotherapeutic may lead to an increase of efficacy. Nevertheless, there are some limitations to the use of these nanomaterials before their successful translation to clinics. One is the limited amount of data on the use of such systems to tackle infection in in vivo models, thus preventing adequate assessment of optimal dose, appropriate administration routes and possible interaction of nanoparticles with cells and tissues, whose toxicological profile is not completely understood (Baptista et al., 2018; Lee et al., 2019).
Silver Nanoparticles
Traditionally, Silver (Ag) has been employed as antimicrobial, namely silver sulfadiazine and silver nitrate (Lansdown, 2006), which relied on the release of Ag+ that triggers a range of processes resulting in hampered bacterial growth. Silver nanoparticles has allowed improved release of silver ions and, thus, enhance the bactericide action (Dakal et al., 2016). Silver nanoparticles (AgNPs) may be synthesized by different protocols relying on thermic vaporization, chemical or photochemical reduction of silver ions to form nanoparticles that are then capped either by the same reagent or by additional compounds, which promote solubility and stability (Lee and Jun, 2019). The most used method is based on using citrate as reducing and capping agent of silver salts. Nowadays, other methodologies using biological extracts (e.g., plant extracts) has been employed for the “green synthesis” of this nanomaterial (Masooleh et al., 2019). Regardless of the synthesis method, the antibacterial activity of AgNPs depends on the size and shape of the particles; for example, the antibiotic effect increases with smaller sizes due to the dramatic increase in surface area available for ion release and/or to interact with the bacteria. In fact, some authors propose that AgNPs between 1 and 10 nm could interact more efficiently with the bacteria cell membrane (Morones et al., 2005), and spherical nanoparticles seem to be more effective in bacterial eradication than triangles or cylinders (Raza et al., 2016). Still, for AgNPs, size is the stronger determinant associated with antibiotic activity, which is clear in conceptual studies of efficiency for Pseudomonas aeruginosa and Escherichia coli eradication (Raza et al., 2016). However, AgNPs have shown biocidal effects against a range of bacterial species with clinical interest, such as Staphylococcus epidermidis, Enterococcus faecalis, Vibrio cholerae, and Salmonella spp. (Morones et al., 2005; Sánchez-López et al., 2020).
Although the biocidal action of AgNPs has been attributed mainly to the Ag+ release, the actual mechanism is not yet completely elucidated. Crucial to their effect is the fact that AgNPs tend to accumulate at the membrane where they progressively aggregate, allowing silver ions to interact with different functional groups, such as sulfate or phosphate, and disturb the function of the bacterial membrane, promoting its rupture and liberate the cytoplasmatic content (Le Ouay and Stellacci, 2015). Other studies have suggested that Ag+ is able to interact and inactivate some biological structures and affect the bacteria’ respiratory process, namely inhibiting the respiratory chain (Costa et al., 2010). However, perhaps the most widely accepted hypothesis is the production of reactive oxygen species (ROS), like superoxide or hydrogen peroxide, which interact with the lipids, proteins or DNA, promoting alteration in the normal functions, triggering lysis and cell death (Quinteros et al., 2016).
AgNPs have also found a range of industrial applications that require some level of inhibition of bacterial growth. One such examples is the development of new tools for odontology, where AgNPs have been added to customary compounds for dental implants (e.g., polydopamine and titanium) to improve biocompatibility and provide for added antibacterial efficacy against S. mutans, commonly implicated in the caries disease (Choi et al., 2019). Green synthesized AgNPs by Epigallocatechin gallate (green tea extract) as reducing and chitosan as capping agent decreased the MIC and MBC against S. mutans (Yin et al., 2019). What is more, these AgNPs induced lower amounts of lactic acid and polysaccharides in the biofilm, thus enhancing the protective action of the nanoparticle extracts. The synergistic effect of the bio-extracts and AgNPs may be associated to the bactericide activity of the green tea polyphenols and the large surface area of AgNPs which increase the contact with bacteria and facilitate disruption of cell metabolism. In addition, E. gallate is able to inhibit the S. mutans glucosyltransferase reducing bacterial adherence and biofilm formation. Another interesting application for AgNPs has been its inclusion in toothpaste formulations with promising antibacterial efficacy against S. mutans (Ahmed et al., 2019).
Furthermore, the synergistic effect with other conventional antibiotics makes possible the application of AgNPs as an alternative tool to tackle MDR strains. In fact, AgNPs and conventional antibiotics exert their biocidal action via different mechanisms and, therefore, their combination would prevent the development of added resistance. For example, clindamycin has already been combined with AgNPs resulting in lower MICs in a synergistic effect and rifampicin coupled to AgNP increased the antibiotic effect against methicillin resistant bacteria (Khan et al., 2019a).
Gold Nanoparticles
Gold nanoparticles (AuNPs) have also been employed in different fields of biomedical research due to their ease of synthesis, biocompatibility and low toxicity to higher eukaryotes. They are easier to functionalize with different biological moieties like DNA, mRNA, peptides, etc. than their silver counterparts. Moreover, AuNPs present remarkable optical and photoelectric properties that have demonstrated high potential toward the development of new therapy tools (Amendoeira et al., 2020). The chemical processes for the synthesis of AuNPs are similar to those of AgNPs, where the citrate reduction method is clearly the most used method. Still, in the last years, as for AgNPs, several green synthesis methods with plant or other extract have been proposed (Khan et al., 2019b).
AuNPs have been reported to exhibit antimicrobial activity against a wide range of bacteria and fungus (Tao, 2018). Several mechanisms of action have been highlighted as the basis of their antimicrobial properties, namely: AuNPs may bind to the membrane of bacteria, modify the membrane’s potential, decrease intracellular ATP levels, disturb intracellular trafficking, aggregate together with proteins and disturb the assembly of tRNA to the ribosome (Cui et al., 2012). Perhaps, the main aspect related to antimicrobial efficacy relates to nanoparticle dispersion and the AuNPs’ surface roughness that could interact with the bacteria membrane (Lima et al., 2013). AuNPs have also been proposed as drug carriers, conveyors of gene therapy and photothermal therapy (Li et al., 2019; Masri et al., 2019b).
The use of AuNPs as drug delivery systems for traditional antibiotics has made possible to administrate drugs more effectively and uniformly distributed toward the target tissue, improving the efficacy and biocompatibility of antibiotic-conjugated AuNPs. The surface of AuNPs may be easily functionalized with small ligands harboring carboxylic acid, hydroxyl, or amine functional groups that can then be used to conjugate antimicrobials (Lee et al., 2017). The so assemble nanoformulation improves solubility of non-water-soluble drugs and allows for controlled and localized release of the antibiotic, for example with an external stimulus (Canaparo et al., 2019). For example, a formulation of imipenem and meropenem on AuNPs increased the antibacterial effect against carbapenems resistant Gram-negative bacteria, like Klebsiella pneumoniae, Proteus mirabilis and Acinetobacter baumannii isolated from human patients, and decreased the MIC while potentiating the effect in antibiotic kill test (Shaker and Shaaban, 2017). Moreover, these studies showed a size-dependent efficacy of the drug system, with optimal efficacy for 35 nm nanoparticles. Another advantage antibiotic delivery system mediated by AuNPs is the drug-controlled release, where AuNPs loaded with Amphotericin B showed an increase of biocidal efficiency of 78%, with less cytotoxicity and hemolytic toxicity to the host when compared to the antibiotic alone (Kumar et al., 2019).
When AuNPs are irradiated by light with an appropriate wavelength, they tend to convert the received energy into heat, thus resulting in a hyperthermal effect capable to induce damage to the membrane structure (Kirui et al., 2019; Amendoeira et al., 2020). The antimicrobial effect through the use of photothermally active nanomaterials may become an interesting tool against antibiotic resistance. For example, near-infrared (NIR) radiation is useful to promote hyperthermy based on AuNPs, effective against S. aureus and E. coli after 10 min of irradiation at 808 nm (Alhmoud et al., 2017). NIR photothermy using AuNPs has been also used as an efficient technique to eliminate a broader range of microorganism with improved antibiofilm activity. In fact this approach was demonstrated effective at damaging the cell wall of streptococci, such as S. mutans, S. sobrinus, Streptococcus oralis and S. salivarius (Castillo-Martínez et al., 2015).
The combination of these two metals, Silver and Gold, in alloy nanoparticles has also been proposed as suitable nanoagent against microbes. In fact, such approach combines the improved stability and ease of functionalization provided by gold, with the higher antimicrobial activity of silver, while avoiding some problems associated with the aggregation and toxicity to the host (Dos Santos et al., 2012). The positive results of this association have been proposed in a system where gold-silver alloy “nanoflowers” decreased the MIC against E. coli three-fold when compared to AgNP alone (Yan et al., 2018). Gold-silver alloy nanoparticles have shown their potential to eradicate biofilm and reduce the MICs against Gram-positive and Gram-negative bacteria, which could then be used to circumvent drug resistance (Ramasamy et al., 2016). Kyaw et al. (2017) showed that submitting triangular AuNPs coated by silver to laser irradiation, induced a change of shape to spherical and increased the antibacterial activity.
Other Metallic Nanoparticles
Nanoparticles employing iron (Fe) have been applied due to their antimicrobial properties, which has been associated with the generation of ROS (Arakha et al., 2015). The most used is iron oxide nanoparticles which provide good efficiency in a wide range spectrum mediated by the damage in different structures like proteins or DNA (Saqib et al., 2019). Most of these iron nanoparticles present some magnetic properties that may be used for a range of applications, from diagnostics to therapeutics (Rodrigues et al., 2019). Magnetic nanoparticles have been shown to interfere with the thiol groups at the respiratory base of bacteria and, thus, assisting in disrupting effective metabolism, resulting in biocidal activity against some drug resistant bacteria (Madubuonu et al., 2019). Ion NPs have also been used as delivery vehicles for antibiotics, such as chlorhexidine and erythromycin against S. mutans (Vieira et al., 2019).
Copper nanoparticles have also been used as antimicrobial against a wide range of microorganisms including bacteria, fungi, and even algae (Hou et al., 2018; Sardella et al., 2018). As for AgNPs, the size is related to CuNP activity due to the dramatic increase of the surface/volume ratio, which promote the generation of ROS that trigger cell damage according, such as oxidation of proteins, cleavage of DNA/RNA molecules or lipid peroxidation in membranes (Shaikh et al., 2019). Usually, CuNPs are combined within polymers or functionalized in core-shell structures to provide stability and control possible ion leakage (Anyaogu et al., 2008). For example, CuNPs coated with chitosan showed an antibacterial effect comparable to that of traditional oral antimicrobial agents (chlorhexidine and cetylpyridinium chloride) against S. mutans (Covarrubias et al., 2018). In another example, the combination of AgNPs and CuNPs was shown to have a preventive and therapeutic effect in mastitis caused by S. agalactiae (Kalińska et al., 2019).
Some authors have studied the antibacterial activity of zinc NPs (ZnNPs) against Streptococcus mitis, where the biocidal action was associated to ROS induction identified via the increase of superoxide dismutase activity (SOD) (Khan et al., 2016). Moreover, ZnNPs showed the capability to inhibit the formation of biofilm by S. mitis in a dose dependent manner, corroborated the evaluation of bapA1 gene expression, which is associated to generation of the biofilm. In another study, ZnNPs showed the capability to inhibit S. sobrinus biofilm formation (Aydin Sevinç and Hanley, 2010).
Concluding Remarks
The progressive emergence of resistance to conventional antibiotics is reducing the ability to control infectious diseases and, particularly, those caused by pyogenic streptococci. To combat this public health threat, several alternative strategies have been proposed, and the promising efficacy in vitro of some of these antibacterial approaches has been the focus of attention.
Despite the progress achieved to date, most alternative approaches are of narrow spectrum unlike the broad-spectrum of conventional antibiotics. However, the combined action of one of these alternative approaches with traditional antibiotics may increase the success rate of therapeutics once that most new strategies attenuate bacterial pathogenesis allowing bacteria to be eliminated by antibiotics and action of the immune system. Moreover, combination therapies may decrease the selective pressure for resistance to antibiotics, and consequently to reduce the rate of emergence of resistance.
Bacteriocins are considered as a hopeful therapeutic alternative caused by its proven efficacy and chemical structural and functional diversity. Nonetheless, the broad use of bacteriocin can also confer threatening for its usage on a large scale, namely, the possible resistance to bacteriocins could limit their future way. Bacteriophages allow the development of specific therapies, phage-derived enzymes can be used as a substitute for conventional antibiotics, for example, phage-derived lysins that degrade peptidoglycan can be considered as an alternative to β-lactam antibiotics. However, the advancement of new laws that regulate the use of Bacteriophages and phage-derived enzymes is necessary. The application of nanomaterials may provide for new therapy tools to assist in tackling the traditional mechanisms of resistance. Still, there is plenty of work ahead to facilitate the translation to the clinics, namely toward better characterization of these materials, the capability to effectively scale-up for widespread use, and clarification of toxicity aspect, which altogether pave the way for robust assessment in clinical trials. Nowadays, the cost associated with the development of nanotechnology platforms is high and, consequently, the use of more conventional therapies are still preferred.
The most significant concern safety of alternative therapies is the gap of understanding of interaction with the human host. Thus, evaluate the impacts of alternative therapies on the host is essential for future widespread application. Future studies must investigate in vivo efficacy of combination therapy to assess their potential, impact of the host, and evolution of bacterial resistance.
Author Contributions
CA-B and LR-G: revision of literature and drafting the manuscript. AF and PB: revision of literature and drafting and editing the final manuscript. All authors contributed to the article and approved the submitted version.
Funding
This work was supported by the Unidade de Ciências Biomoleculares Aplicadas-UCIBIO which is financed by national funds from FCT/MEC (UID/Multi/04378/2020). FCT-MEC has also acknowledged the grant SFRH/BD/118350/2016 to CA-B and LR-G (Inn-Indigo 0002/2015 RA Detect).
Conflict of Interest
The authors declare that the research was conducted in the absence of any commercial or financial relationships that could be construed as a potential conflict of interest.
References
Abdelsalam, M., Asheg, A., and Eissa, A. E. (2013). Streptococcus dysgalactiae: an emerging pathogen of fishes and mammals. Int. J. Vet. Sci. Med. 1, 1–6. doi: 10.1016/j.ijvsm.2013.04.002
Abedon, S. T. (2015a). Bacteriophage exploitation of bacterial biofilms: phage preference for less mature targets? FEMS Microbiol. Lett. 363:fnv246. doi: 10.1093/femsle/fnv246
Abedon, S. T. (2015b). Ecology of anti-biofilm agents II: bacteriophage exploitation and biocontrol of biofilm bacteria. Pharmaceuticals 8, 559–589. doi: 10.3390/ph8030559
Ahmed, F., Prashanth, S., Sindhu, K., Nayak, A., and Chaturvedi, S. (2019). Antimicrobial efficacy of nanosilver and chitosan against Streptococcus mutans, as an ingredient of toothpaste formulation: an in vitro study. J. Indian Soc. Pedod. Prev. Dent. 37, 46–54. doi: 10.4103/JISPPD.JISPPD_239_18
Alhmoud, H., Cifuentes-Rius, A., Delalat, B., Lancaster, D. G., and Voelcker, N. H. (2017). Gold-decorated porous silicon nanopillars for targeted hyperthermal treatment of bacterial infections. ACS Appl. Mater. Interfaces 9, 33707–33716. doi: 10.1021/acsami.7b13278
Almeida, A., Alves-Barroco, C., Sauvage, E., Bexiga, R., Albuquerque, P., Tavares, F., et al. (2016). Persistence of a dominant bovine lineage of group B Streptococcus reveals genomic signatures of host adaptation. Environ. Microbiol. 18, 4216–4229. doi: 10.1111/1462-2920.13550
Alves-Barroco, C., Roma-Rodrigues, C., Balasubramanian, N., Guimarães, M. A., Ferreira-Carvalho, B. T., Muthukumaran, J., et al. (2019). Biofilm development and computational screening for new putative inhibitors of a homolog of the regulatory protein BrpA in Streptococcus dysgalactiae subsp. dysgalactiae. Int. J. Med. Microbiol. 309, 169–181. doi: 10.1016/j.ijmm.2019.02.001
Amendoeira, A., Rivas-García, L., Fernandes, A. R., and Baptista, P. V. (2020). Light irradiation of gold nanoparticles toward advanced cancer therapeutics. Adv. Ther. 3:1900153. doi: 10.1002/adtp.201900153
Anyaogu, K. C., Fedorov, A. V., and Neckers, D. C. (2008). Synthesis, characterization, and antifouling potential of functionalized copper nanoparticles. Langmuir 24, 4340–4346. doi: 10.1021/la800102f
Arakha, M., Pal, S., Samantarrai, D., Panigrahi, T. K., Mallick, B. C., Pramanik, K., et al. (2015). Antimicrobial activity of iron oxide nanoparticle upon modulation of nanoparticle-bacteria interface. Sci. Rep. 5:14813. doi: 10.1038/srep14813
Arias, B., Kovacec, V., Vigliarolo, L., Suárez, M., Tersigni, C., Müller, L., et al. (2019). Fluoroquinolone-resistant Streptococcus agalactiae invasive isolates recovered in Argentina. Microb. Drug Resist. 25, 739–743. doi: 10.1089/mdr.2018.0246
Aydin Sevinç, B., and Hanley, L. (2010). Antibacterial activity of dental composites containing zinc oxide nanoparticles. J. Biomed. Mater. Res. Part B Appl. Biomater. 94, 22–31. doi: 10.1002/jbm.b.31620
Balcão, V. M., Barreira, S. V. P., Nunes, T. M., Chaud, M. V., Tubino, M., and Vila, M. M. D. C. (2014). Carbohydrate hydrogels with stabilized phage particles for bacterial biosensing: bacterium diffusion studies. Appl. Biochem. Biotechnol. 172, 1194–1214. doi: 10.1007/s12010-013-0579-2
Baldassarri, L., Creti, R., Recchia, S., Imperi, M., Facinelli, B., Giovanetti, E., et al. (2006). Therapeutic failures of antibiotics used to treat macrolide-susceptible Streptococcus pyogenes infections may be due to biofilm formation. J. Clin. Microbiol. 44, 2721–2727. doi: 10.1128/JCM.00512-06
Balsalobre, L., Ferrándiz, M. J., Liñares, J., Tubau, F., and De la Campa, A. G. (2003). Viridans group streptococci are donors in horizontal transfer of topoisomerase IV genes to Streptococcus pneumoniae. Antimicrob. Agents Chemother. 47, 2072–2081. doi: 10.1128/AAC.47.7.2072-2081.2003
Baptista, P. V., McCusker, M. P., Carvalho, A., Ferrreira, D. A., Mohan, N., Martins, M., et al. (2018). Nano-Strategies to fight Multidrug Resistant Bacteria – “A battle of the titans”. Front. Microbiol. 9:1441. doi: 10.3389/fmicb.2018.01441
Bhattacharyya, P., Agarwal, B., Goswami, M., Maiti, D., Baruah, S., and Tribedi, P. (2018). Zinc oxide nanoparticle inhibits the biofilm formation of Streptococcus pneumoniae. Antonie Van Leeuwenhoek 111, 89–99. doi: 10.1007/s10482-017-0930-7
Bjarnsholt, T., Ciofu, O., Molin, S., Givskov, M., and Høiby, N. (2013). Applying insights from biofilm biology to drug development-can a new approach be developed? Nat. Rev. Drug Discov. 12, 791–808. doi: 10.1038/nrd4000
Bondy-Denomy, J., Pawluk, A., Maxwell, K. L., and Davidson, A. R. (2013). Bacteriophage genes that inactivate the CRISPR/Cas bacterial immune system. Nature 493, 429–432. doi: 10.1038/nature11723
Borriello, G., Werner, E., Roe, F., Kim, A. M., Ehrlich, G. D., and Stewart, P. S. (2004). Oxygen limitation contributes to antibiotic tolerance of Pseudomonas aeruginosa in Biofilms. Antimicrob. Agents Chemother. 48, 2659–2664. doi: 10.1128/AAC.48.7.2659
Borysowski, J., Da, K., Wierzbicki, P., Ohams, M., Kaniuga, E., Kłak, M., et al. (2012). Phage as a modulator of immune responses: practical implications for phage therapy. Adv. Virus Res. 83, 41–71. doi: 10.1016/B978-0-12-394438-2.00002-5
Bouqellah, N. A., Mohamed, M. M., and Ibrahim, Y. (2019). Synthesis of eco-friendly silver nanoparticles using Allium sp. and their antimicrobial potential on selected vaginal bacteria. Saudi J. Biol. Sci. 26, 1789–1794. doi: 10.1016/j.sjbs.2018.04.001
Brandt, C. M., and Spellerberg, B. (2009). Human infections due to Streptococcus dysgalactiae subspecies equisimilis. Clin. Infect. Dis. 49, 766–772. doi: 10.1086/605085
Brenciani, A., Bacciaglia, A., Vecchi, M., Vitali, L. A., Varaldo, P. E., and Giovanetti, E. (2007). Genetic elements carrying erm(B) in Streptococcus pyogenes and association with tet(M) tetracycline resistance gene. Antimicrob. Agents Chemother. 51, 1209–1216. doi: 10.1128/AAC.01484-06
Brenciani, A., Ojo, K. K., Monachetti, A., Menzo, S., Roberts, M. C., Varaldo, P. E., et al. (2004). Distribution and molecular analysis of mef(A)-containing elements in tetracycline-susceptible and -resistant Streptococcus pyogenes clinical isolates with efflux-mediated erythromycin resistance. J. Antimicrob. Chemother. 54, 991–998. doi: 10.1093/jac/dkh481
Brenciani, A., Tiberi, E., Bacciaglia, A., Petrelli, D., Varaldo, P. E., and Giovanetti, E. (2011). Two distinct genetic elements are responsible for erm(TR)-mediated erythromycin resistance in tetracycline-susceptible and tetracycline-resistant strains of Streptococcus pyogenes. Antimicrob. Agents Chemother. 55, 2106–2112. doi: 10.1128/AAC.01378-10
Bush, K., and Jacoby, G. A. (2010). Updated functional classification of beta-lactamases. Antimicrob. Agents Chemother. 54, 969–976. doi: 10.1128/AAC.01009-09
Canaparo, R., Foglietta, F., Giuntini, F., Della Pepa, C., Dosio, F., and Serpe, L. (2019). Recent developments in antibacterial therapy: focus on stimuli-responsive drug-delivery systems and therapeutic nanoparticles. Molecules 24:1991. doi: 10.3390/molecules24101991
Castillo-Martínez, J. C., Martínez-Castañón, G. A., Martínez-Gutierrez, F., Zavala-Alonso, N. V., Patiño-Marín, N., Niño-Martinez, N., et al. (2015). Antibacterial and antibiofilm activities of the photothermal therapy using gold nanorods against seven different bacterial strains. J. Nanomater. 2015:783671. doi: 10.1155/2015/783671
Cattoir, V. (2016). Mechanisms of antibiotic resistance. Front. Microbiol. 6:34. doi: 10.3389/fmicb.2015.00034
Chang, H. H., Cohen, T., Grad, Y. H., Hanage, W. P., O’Brien, T. F., and Lipsitch, M. (2015). Origin and proliferation of multiple-drug resistance in bacterial pathogens. Microbiol. Mol. Biol. Rev. 79, 101–116. doi: 10.1128/mmbr.00039-14
Choi, S.-H., Jang, Y.-S., Jang, J.-H., Bae, T.-S., Lee, S.-J., and Lee, M.-H. (2019). Enhanced antibacterial activity of titanium by surface modification with polydopamine and silver for dental implant application. J. Appl. Biomater. Funct. Mater. 17:2280800019847067. doi: 10.1177/2280800019847067
Colomer-Lluch, M., Jofre, J., and Muniesa, M. (2011). Antibiotic resistance genes in the bacteriophage DNA fraction of environmental samples. PLoS One 6:e17549. doi: 10.1371/journal.pone.0017549
Conley, J., Cook, L. S., Davies, H. D., Olson, M. E., Ceri, H., and Phan, V. (2003). Biofilm formation by group A streptococci: Is there a relationship with treatment failure? J. Clin. Microbiol. 41, 4043–4048. doi: 10.1128/JCM.41.9.4043-4048.2003
Cook, L. C., LaSarre, B., and Federle, M. J. (2013). Interspecies communication among commensal and pathogenic streptococci. mBio 4:e00382-13. doi: 10.1128/mBio.00382-13
Costa, C. S., Ronconi, J. V. V., Daufenbach, J. F., Gonçalves, C. L., Rezin, G. T., Streck, E. L., et al. (2010). In vitro effects of silver nanoparticles on the mitochondrial respiratory chain. Mol. Cell. Biochem. 342, 51–56. doi: 10.1007/s11010-010-0467-9
Covarrubias, C., Trepiana, D., and Corral, C. (2018). Synthesis of hybrid copper-chitosan nanoparticles with antibacterial activity against cariogenic Streptococcus mutans. Dent. Mater. J. 37, 379–384. doi: 10.4012/dmj.2017-195
Cox, G., and Wright, G. D. (2013). Intrinsic antibiotic resistance: mechanisms, origins, challenges and solutions. Int. J. Med. Microbiol. 303, 287–292. doi: 10.1016/j.ijmm.2013.02.009
Cui, Y., Zhao, Y., Tian, Y., Zhang, W., Lü, X., and Jiang, X. (2012). The molecular mechanism of action of bactericidal gold nanoparticles on Escherichia coli. Biomaterials 33, 2327–2333. doi: 10.1016/j.biomaterials.2011.11.057
Da Cunha, V., Davies, M. R., Douarre, P., Rosinski-Chupin, I., Margarit, I., Spinali, S., et al. (2014). Streptococcus agalactiae clones infecting humans were selected and fixed through the extensive use of tetracycline. Nat. Commun. 5:4544. doi: 10.1038/ncomms5544
Dakal, T. C., Kumar, A., Majumdar, R. S., and Yadav, V. (2016). Mechanistic basis of antimicrobial actions of silver nanoparticles. Front. Microbiol. 7:1831. doi: 10.3389/fmicb.2016.01831
Dang, T. N. D., Srinivasan, U., Britt, Z., Marrs, C. F., Zhang, L., Ki, M., et al. (2014). Efflux-mediated resistance identified among norfloxacin resistant clinical strains of Group B Streptococcus from South Korea. Epidemiol. Health 36:e2014022. doi: 10.4178/epih/e2014022
de Almeida, G. M. F., and Sundberg, L.-R. (2020). The forgotten tale of Brazilian phage therapy. Lancet Infect. Dis. 20, e90–e101. doi: 10.1016/S1473-3099(20)30060-8
De Greef, S. C., Mouton, J. W., Schoffelen, A. F., and Verduin, C. M. (2019). Data from: NethMap: CONSUMPTION of Antimicrobial agents and Antimicrobial Resistance among Medically Important Bacteria in the Netherlands. Rijksinstituut voor Volksgezondheid en Milieu RIVM. Available online at: https://rivm.openrepository.com/handle/10029/623134 (accessed August 4, 2020).
Del Grosso, M., Camilli, R., Barbabella, G., Northwood, J. B., Farrell, D. J., and Pantosti, A. (2011). Genetic resistance elements carrying mef subclasses other than mef(A) in Streptococcus pyogenes. Antimicrob. Agents Chemother. 55, 3226–3230. doi: 10.1128/AAC.01713-10
Del Pozo, J. L. (2018). Biofilm-related disease. Expert Rev. Anti Infect. Ther. 16, 51–65. doi: 10.1080/14787210.2018.1417036
Di Luca, M. C., D’Ercole, S., Petrelli, D., Prenna, M., Ripa, S., and Vitali, L. A. (2010). Lysogenic transfer of mef(A) and tet(O) genes carried by Φm46.1 among group A streptococci. Antimicrob. Agents Chemother. 54, 4464–4466. doi: 10.1128/AAC.01318-09
Dias, H. B., Bernardi, M. I. B., Marangoni, V. S., de Abreu Bernardi, A. C., de Souza Rastelli, A. N., and Hernandes, A. C. (2019). Synthesis, characterization and application of Ag doped ZnO nanoparticles in a composite resin. Mater. Sci. Eng. C 96, 391–401. doi: 10.1016/j.msec.2018.10.063
Dicks, L. M. T., Dreyer, L., Smith, C., and van Staden, A. D. (2018). A review: the fate of bacteriocins in the human gastro-intestinal tract: Do they cross the gut–blood barrier? Front. Microbiol. 9:2297. doi: 10.3389/fmicb.2018.02297
Doern, C. D., Roberts, A. L., Hong, W., Nelson, J., Lukomski, S., Swords, W. E., et al. (2009). Biofilm formation by group A Streptococcus: a role for the streptococcal regulator of virulence (Srv) and streptococcal cysteine protease (SpeB). Microbiology 155, 46–52. doi: 10.1099/mic.0.021048-0
Dos Santos, M. M., Queiroz, M. J., and Baptista, P. V. (2012). Enhancement of antibiotic effect via gold:silver-alloy nanoparticles. J. Nanoparticle Res. 14, 859–867. doi: 10.1007/s11051-012-0859-8
Doumith, M., Mushtaq, S., Martin, V., Chaudhry, A., Adkin, R., Coelho, J., et al. (2017). Genomic sequences of Streptococcus agalactiae with high-level gentamicin resistance, collected in the BSAC bacteraemia surveillance. J. Antimicrob. Chemother. 72, 2704–2707. doi: 10.1093/jac/dkx207
Duesberg, C. B., Malhotra-Kumar, S., Goossens, H., McGee, L., Klugman, K. P., Welte, T., et al. (2008). Interspecies recombination occurs frequently in quinolone resistance-determining regions of clinical isolates of Streptococcus pyogenes. Antimicrob. Agents Chemother. 52, 4191–4193. doi: 10.1128/AAC.00518-08
Emaneini, M., Mirsalehian, A., Beigvierdi, R., Fooladi, A. A. I., Asadi, F., Jabalameli, F., et al. (2014). High incidence of macrolide and tetracycline resistance among Streptococcus agalactiae strains isolated from clinical samples in Tehran. Iran. Maedica 9, 157–161.
Emmanuel, R., Saravanan, M., Ovais, M., Padmavathy, S., Shinwari, Z. K., and Prakash, P. (2017). Antimicrobial efficacy of drug blended biosynthesized colloidal gold nanoparticles from Justicia glauca against oral pathogens: a nanoantibiotic approach. Microb. Pathog. 113, 295–302. doi: 10.1016/j.micpath.2017.10.055
Enault, F., Briet, A., Bouteille, L., Roux, S., Sullivan, M. B., and Petit, M. A. (2017). Phages rarely encode antibiotic resistance genes: A cautionary tale for virome analyses. ISME J. 11, 237–247. doi: 10.1038/ismej.2016.90
Euler, C. W., Juncosa, B., Ryan, P. A., Deutsch, D. R., McShan, W. M., and Fischetti, V. A. (2016). Targeted curing of all lysogenic bacteriophage from Streptococcus pyogenes using a novel counter-selection technique. PLoS One 11:e0146408. doi: 10.1371/journal.pone.0146408
Fair, R. J., and Tor, Y. (2014). Perspectives in medicinal chemistry antibiotics and bacterial resistance in the 21st Century. Perspect. Medicin. Chem. 6, 25–64. doi: 10.4137/PMC.S14459
Fairhead, H., Wilkinson, A., and Severi, E. (2017). US Patent No. WO 2017/174809, A1. Washington, DC: U.S. Patent and Trademark Office.
Ferrándiz, M. J., Fenoll, A., Liñares, J., and De La Campa, A. G. (2000). Horizontal transfer of parC and gyrA in fluoroquinolone-resistant clinical isolates of Streptococcus pneumoniae. Antimicrob. Agents Chemother. 44, 840–847. doi: 10.1128/AAC.44.4.840-847.2000
Fléchard, M., and Gilot, P. (2014). Physiological impact of transposable elements encoding DDE transposases in the environmental adaptation of Streptococcus agalactiae. Microbiology 160, 1298–1315. doi: 10.1099/mic.0.077628-0
Flemming, H. C., and Wingender, J. (2010). The biofilm matrix. Nat. Rev. Microbiol. 8, 623–633. doi: 10.1038/nrmicro2415
Franke, A. E., and Clewell, D. B. (1981). Evidence for a chromosome-borne resistance transposon (Tn916) in Streptococcus faecalis that is capable of “conjugal” transfer in the absence of a conjugative plasmid. J. Bacteriol. 145, 494–502. doi: 10.1128/jb.145.1.494-502.1981
Furfaro, L. L., Chang, B. J., and Payne, M. S. (2018). Applications for bacteriophage therapy during pregnancy and the perinatal period. Front. Microbiol. 8:2660. doi: 10.3389/fmicb.2017.02660
Fuursted, K., Stegger, M., Hoffmann, S., Lambertsen, L., Andersen, P. S., Deleuran, M., et al. (2016). Description and characterization of a penicillin-resistant Streptococcus dysgalactiae subsp. equisimilis clone isolated from blood in three epidemiologically linked patients. J. Antimicrob. Chemother. 71, 3376–3380. doi: 10.1093/jac/dkw320
Galimand, M., Lambert, T., Gerbaud, G., and Courvalin, P. (1999). High-level aminoglycoside resistance in the beta-hemolytic group G Streptococcus isolate BM2721. Antimicrob. Agents Chemother. 43, 3008–3010. doi: 10.1128/aac.43.12.3008
Gao, W., Chen, Y., Zhang, Y., Zhang, Q., and Zhang, L. (2018). Nanoparticle-based local antimicrobial drug delivery. Adv. Drug Deliv. Rev. 127, 46–57. doi: 10.1016/j.addr.2017.09.015
Garrett, T. R., Bhakoo, M., and Zhang, Z. (2008). Bacterial adhesion and biofilms on surfaces. Prog. Nat. Sci. 18, 1049–1056. doi: 10.1016/j.pnsc.2008.04.001
Genteluci, G. L., Silva, L. G., Souza, M. C., Glatthardt, T., de Mattos, M. C., Ejzemberg, R., et al. (2015). Assessment and characterization of biofilm formation among human isolates of Streptococcus dysgalactiae subsp. equisimilis. Int. J. Med. Microbiol. 305, 937–947. doi: 10.1016/j.ijmm.2015.10.004
Gherardi, G., Imperi, M., Palmieri, C., Magi, G., Facinelli, B., Baldassarri, L., et al. (2014). Genetic diversity and virulence properties of Streptococcus dysgalactiae subsp. equisimilis from different sources. J. Med. Microbiol. 63, 90–98. doi: 10.1099/jmm.0.062109-0
Ghosh, C., Sarkar, P., Issa, R., and Haldar, J. (2019). Alternatives to conventional antibiotics in the era of antimicrobial resistance. Trends Microbiol. 27, 323–338. doi: 10.1016/j.tim.2018.12.010
Giovanetti, E., Brenciani, A., Lupidi, R., Roberts, M. C., and Varaldo, P. E. (2003). Presence of the tet(O) gene in erythromycin- and tetracycline-resistant strains of Streptococcus pyogenes and linkage with either the mef(A) or the erm(A) gene. Antimicrob. Agents Chemother. 47, 2844–2849. doi: 10.1128/AAC.47.9.2844-2849.2003
Gizachew, M., Tiruneh, M., Moges, F., and Tessema, B. (2019). Streptococcus agalactiae maternal colonization, antibiotic resistance and serotype profiles in Africa: a meta-analysis. Ann. Clin. Microbiol. Antimicrob. 18:14. doi: 10.1186/s12941-019-0313-1
Gomez, J. E., Kaufmann-Malaga, B. B., Wivagg, C. N., Kim, P. B., Silvis, M. R., Renedo, N., et al. (2017). Ribosomal mutations promote the evolution of antibiotic resistance in a multidrug environment. eLife 6:e20420. doi: 10.7554/eLife.20420
Grohmann, E., Muth, G., and Espinosa, M. (2003). Conjugative Plasmid Transfer in Gram-Positive Bacteria. Microbiol. Mol. Biol. Rev. 67, 277–301. doi: 10.1128/mmbr.67.2.277-301.2003
Gu, Y., Huang, Y., Qiu, Z., Xu, Z., Li, D., Chen, L., et al. (2020). Vitamin B2 functionalized iron oxide nanozymes for mouth ulcer healing. Sci. China Life Sci. 63, 68–79. doi: 10.1007/s11427-019-9590-6
Guerin, F., Varon, E., Hoï, A. B., Gutmann, L., and Podglajen, I. (2000). Fluoroquinolone resistance associated with target mutations and active efflux in oropharyngeal colonizing isolates of viridans group streptococci. Antimicrob. Agents Chemother. 44, 2197–2200. doi: 10.1128/AAC.44.8.2197-2200.2000
Hadjirin, N. F., Harrison, E. M., Holmes, M. A., and Paterson, G. K. (2014). Conjugative transfer frequencies of mef(A)-containing Tn1207.3 to macrolide-susceptible Streptococcus pyogenes belonging to different emm types. Lett. Appl. Microbiol. 58, 299–302. doi: 10.1111/lam.12213
Haenni, M., Saras, E., Bertin, S., Leblond, P., Madec, J.-Y., and Payot, S. (2010). Diversity and mobility of integrative and conjugative elements in bovine isolates of Streptococcus agalactiae, S. dysgalactiae subsp. dysgalactiae, and S. uberis. Appl. Environ. Microbiol. 76, 7957–7965. doi: 10.1128/AEM.00805-10
Heng, N. C. K., Swe, P. M., Ting, Y. T., Dufour, M., Baird, H. J., Ragland, N. L., et al. (2006). The large antimicrobial proteins (bacteriocins) of streptococci. Int. Congr. Ser. 1289, 351–354. doi: 10.1016/j.ics.2005.11.020
Hols, P., Ledesma-García, L., Gabant, P., and Mignolet, J. (2019). Mobilization of microbiota commensals and their bacteriocins for therapeutics. Trends Microbiol. 27, 690–702. doi: 10.1016/j.tim.2019.03.007
Hooper, D. C. (2002). Fluoroquinolone resistance among Gram-positive cocci. Lancet Infect. Dis. 2, 530–538. doi: 10.1016/S1473-3099(02)00369-9
Hooper, D. C., and Jacoby, G. A. (2016). Topoisomerase inhibitors: fluoroquinolone mechanisms of action and resistance. Cold Spring Harb. Perspect. Med. 6:a025320. doi: 10.1101/cshperspect.a025320
Hou, J., Wu, Y., Li, X., Wei, B., Li, S., and Wang, X. (2018). Toxic effects of different types of zinc oxide nanoparticles on algae, plants, invertebrates, vertebrates and microorganisms. Chemosphere 193, 852–860. doi: 10.1016/j.chemosphere.2017.11.077
Hyman, P. (2019). Phages for phage therapy: isolation, characterization, and host range breadth. Pharmaceuticals 12:35. doi: 10.3390/ph12010035
Isaacs, D., and Dobson, S. R. M. (2016). Severe Group A Streptococcal Infections. Curr. Opin. Infect. Dis. 2, 453–456. doi: 10.1097/00001432-198906000-00022
Jalava, J., Vaara, M., and Huovinen, P. (2004). Mutation at the position 2058 of the 23S rRNA as a cause of macrolide resistance in Streptococcus pyogenes. Ann. Clin. Microbiol. Antimicrob. 3:5. doi: 10.1186/1476-0711-3-5
Jamal, M., Ahmad, W., Andleeb, S., Jalil, F., Imran, M., Nawaz, M. A., et al. (2018). Bacterial biofilm and associated infections. J. Chinese Med. Assoc. 81, 7–11. doi: 10.1016/j.jcma.2017.07.012
Jault, P., Leclerc, T., Jennes, S., Pirnay, J. P., Que, Y. A., Resch, G., et al. (2019). Efficacy and tolerability of a cocktail of bacteriophages to treat burn wounds infected by Pseudomonas aeruginosa (PhagoBurn): a randomised, controlled, double-blind phase 1/2 trial. Lancet Infect. Dis. 19, 35–45. doi: 10.1016/S1473-3099(18)30482-1
Javanbakht, T., Laurent, S., Stanicki, D., and Wilkinson, K. J. (2016). Relating the surface properties of superparamagnetic iron oxide nanoparticles (SPIONs) to their bactericidal effect towards a biofilm of Streptococcus mutans. PLoS One 11:e0154445. doi: 10.1371/journal.pone.0154445
Jordal, S., Glambek, M., Oppegaard, O., and Kittang, B. R. (2015). New tricks from an old cow: infective endocarditis caused by Streptococcus dysgalactiae subsp. dysgalactiae. J. Clin. Microbiol. 53, 731–734. doi: 10.1128/JCM.02437-14
Jun, S. Y., Jung, G. M., Yoon, S. J., Choi, Y. J., Koh, W. S., Moon, K. S., et al. (2014). Preclinical safety evaluation of intravenously administered SAL200 containing the recombinant phage endolysin SAL-1 as a pharmaceutical ingredient. Antimicrob. Agents Chemother. 58, 2084–2088. doi: 10.1128/AAC.02232-13
Kalińska, A., Jaworski, S., Wierzbicki, M., and Gołêbiewski, M. (2019). Silver and copper nanoparticles—an alternative in future mastitis treatment and prevention? Int. J. Mol. Sci. 20:1672. doi: 10.3390/ijms20071672
Kanoh, S., and Rubin, B. K. (2010). Mechanisms of action and clinical application of macrolides as immunomodulatory medications. Clin. Microbiol. Rev. 23, 590–615. doi: 10.1128/CMR.00078-09
Karthika, V., and Arumugam, A. (2017). Synthesis and characterization of MWCNT/TiO2/Au nanocomposite for photocatalytic and antimicrobial activity. IET Nanobiotechnol. 11, 113–118. doi: 10.1049/iet-nbt.2016.0072
Khan, A., Farooq, U., Ahmad, T., Sarwar, R., Shafiq, J., Raza, Y., et al. (2019a). Rifampicin conjugated silver nanoparticles: a new arena for development of antibiofilm potential against methicillin resistant staphylococcus aureus and klebsiella pneumonia. Int. J. Nanomed. 14, 3983–3993. doi: 10.2147/IJN.S198194
Khan, S. T., Ahmad, J., Ahamed, M., Musarrat, J., and Al-Khedhairy, A. A. (2016). Zinc oxide and titanium dioxide nanoparticles induce oxidative stress, inhibit growth, and attenuate biofilm formation activity of Streptococcus mitis. J. Biol. Inorg. Chem. 21, 295–303. doi: 10.1007/s00775-016-1339-x
Khan, T., Ullah, N., Khan, M. A., Mashwani, Z. R., and Nadhman, A. (2019b). Plant-based gold nanoparticles; a comprehensive review of the decade-long research on synthesis, mechanistic aspects and diverse applications. Adv. Colloid Interface Sci. 272:102017. doi: 10.1016/j.cis.2019.102017
Khatoon, Z., Mctiernan, C. D., Suuronen, E. J., and Mah, T. (2018). Bacterial biofilm formation on implantable devices and approaches to its treatment and prevention. Heliyon 4:e01067. doi: 10.1016/j.heliyon.2018.e01067
Kim, S., Byun, J. H., Park, H., Lee, J., Lee, H. S., Yoshida, H., et al. (2018). Molecular epidemiological features and antibiotic susceptibility patterns of Streptococcus dysgalactiae subsp. equisimilis isolates from Korea and Japan. Ann. Lab. Med. 38, 212–219. doi: 10.3343/alm.2018.38.3.212
Kimura, K., Nagano, N., Nagano, Y., Suzuki, S., Wachino, J. I., Shibayama, K., et al. (2013). High frequency of fluoroquinolone- and macrolide-resistant streptococci among clinically isolated group b streptococci with reduced penicillin susceptibility. J. Antimicrob. Chemother. 68, 539–542. doi: 10.1093/jac/dks423
Kimura, K., Suzuki, S., Wachino, J. I., Kurokawa, H., Yamane, K., Shibata, N., et al. (2008). First molecular characterization of group B streptococci with reduced penicillin susceptibility. Antimicrob. Agents Chemother. 52, 2890–2897. doi: 10.1128/AAC.00185-08
Kirui, D. K., Weber, G., Talackine, J., and Millenbaugh, N. J. (2019). Targeted laser therapy synergistically enhances efficacy of antibiotics against multi-drug resistant Staphylococcus aureus and Pseudomonas aeruginosa biofilms. Nanomed. Nanotechnol. Biol. Med. 20:102018. doi: 10.1016/j.nano.2019.102018
Koh, T. H., Sng, L. H., Yuen, S. M., Thomas, C. K., Tan, P. L., Tan, S. H., et al. (2009). Streptococcal cellulitis following preparation of fresh raw seafood. Zoonoses Public Health 56, 206–208. doi: 10.1111/j.1863-2378.2008.01213.x
Kohanski, M. A., Dwyer, D. J., and Collins, J. J. (2010). How antibiotics kill bacteria: from targets to networks. Nat. Rev. Microbiol. 8, 423–435. doi: 10.1038/nrmicro2333
Kong, K.-F., Schneper, L., and Mathee, K. (2010). Beta-lactam antibiotics: from antibiosis to resistance and bacteriology. APMIS 118, 1–36. doi: 10.1111/j.1600-0463.2009.02563.x
Konto-Ghiorghi, Y., Mairey, E., Mallet, A., Duménil, G., Caliot, E., Trieu-Cuot, P., et al. (2009). Dual role for pilus in adherence to epithelial cells and biofilm formation in Streptococcus agalactiae. PLoS Pathog. 5:e1000422. doi: 10.1371/journal.ppat.1000422
Kragh, K. N., Hutchison, J. B., Melaugh, G., Rodesney, C., Roberts, A. E. L., Irie, Y., et al. (2016). Role of multicellular aggregates in biofilm formation. mBio 7:e0023716. doi: 10.1128/mBio.00237-16
Krause, K. M., Serio, A. W., Kane, T. R., and Connolly, L. E. (2016). Aminoglycosides: an overview. Cold Spring Harb. Perspect. Med. 6:a027029. doi: 10.1101/cshperspect.a027029
Kristich, C. J., Rice, L. B., and Arias, C. A. (2014). “Enterococcal infection- treatment and antibiotic resistance,” in Enterococci: From Commensals to Leading Causes of Drug Resistant Infection, eds M. S. Gilmore, D. B. Clewell, Y. Ike, and N. Shankar (Boston, MA: Massachusetts Eye and Ear Infirmary), 123–185. Available online at: https://www.ncbi.nlm.nih.gov/books/NBK190420/ (accessed August 4, 2020).
Kumar, A., Alam, A., Rani, M., Ehtesham, N. Z., and Hasnain, S. E. (2017). Biofilms: survival and defense strategy for pathogens. Int. J. Med. Microbiol. 307, 481–489. doi: 10.1016/j.ijmm.2017.09.016
Kumar, P., Shivam, P., Mandal, S., Prasanna, P., Kumar, S., Prasad, S. R., et al. (2019). Synthesis, characterization, and mechanistic studies of a gold nanoparticle-amphotericin B covalent conjugate with enhanced antileishmanial efficacy and reduced cytotoxicity. Int. J. Nanomed. 14, 6073–6101. doi: 10.2147/IJN.S196421
Kyaw, K., Ichimaru, H., Kawagoe, T., Terakawa, M., Miyazawa, Y., Mizoguchi, D., et al. (2017). Effects of pulsed laser irradiation on gold-coated silver nanoplates and their antibacterial activity. Nanoscale 9, 16101–16105. doi: 10.1039/c7nr06513b
Lai, L., Dai, J., Tang, H., Zhang, S., Wu, C., Qiu, W., et al. (2017). Streptococcus suis serotype 9 strain GZ0565 contains a type VII secretion system putative substrate EsxA that contributes to bacterial virulence and a vanZ-like gene that confers resistance to teicoplanin and dalbavancin in Streptococcus agalactiae. Vet. Microbiol. 205, 26–33. doi: 10.1016/j.vetmic.2017.04.030
Lansdown, A. B. G. (2006). Silver in health care: antimicrobial effects and safety in use. Curr. Probl. Dermatol. 33, 17–34. doi: 10.1159/000093928
Le Ouay, B., and Stellacci, F. (2015). Antibacterial activity of silver nanoparticles: a surface science insight. Nano Today 10, 339–354. doi: 10.1016/j.nantod.2015.04.002
Lee, B., Park, J., Ryu, M., Kim, S., Joo, M., Yeom, J. H., et al. (2017). Antimicrobial peptide-loaded gold nanoparticle-DNA aptamer conjugates as highly effective antibacterial therapeutics against Vibrio vulnificus. Sci. Rep. 7:13572. doi: 10.1038/s41598-017-14127-z
Lee, N.-Y., Ko, W.-C., and Hsueh, P.-R. (2019). Nanoparticles in the treatment of infections caused by multidrug-resistant organisms. Front. Pharmacol. 10:1153. doi: 10.3389/fphar.2019.01153
Lee, S. H., and Jun, B. H. (2019). Silver nanoparticles: synthesis and application for nanomedicine. Int. J. Mol. Sci. 20:865. doi: 10.3390/ijms20040865
Lehtinen, S., Blanquart, F., Lipsitch, M., and Fraser, C. (2019). On the evolutionary ecology of multidrug resistance in bacteria. PLoS Pathog. 15:e1007763. doi: 10.1371/journal.ppat.1007763
Li, W., Geng, X., Liu, D., and Li, Z. (2019). Near-infrared light-enhanced protease-conjugated gold nanorods as a photothermal antimicrobial agent for elimination of exotoxin and biofilms. Int. J. Nanomed. 14, 8047–8058. doi: 10.2147/IJN.S212750
Liao, S., Klein, M. I., Heim, K. P., Fan, Y., Bitoun, J. P., Ahn, S. J., et al. (2014). Streptococcus mutans extracellular DNA is upregulated during growth in biofilms, actively released via membrane vesicles, and influenced by components of the protein secretion machinery. J. Bacteriol. 196, 2355–2366. doi: 10.1128/JB.01493-14
Lier, C., Baticle, E., Horvath, P., Haguenoer, E., Valentin, A. S., Glaser, P., et al. (2015). Analysis of the type II-A CRISPR-Cas system of Streptococcus agalactiae reveals distinctive features according to genetic lineages. Front. Genet. 6:214. doi: 10.3389/fgene.2015.00214
Lim, J. A., Shin, H., Heu, S., and Ryu, S. (2014). Exogenous lytic activity of SPN9CC endolysin against gram-negative Bacteria. J. Microbiol. Biotechnol. 24, 803–811. doi: 10.4014/jmb.1403.03035
Lima, E., Guerra, R., Lara, V., and Guzmán, A. (2013). Gold nanoparticles as efficient antimicrobial agents for Escherichia coli and Salmonella typhi. Chem. Cent. J. 7:111. doi: 10.1186/1752-153X-7-11
Liu, L. C., Tsai, J. C., Hsueh, P. R., Tseng, S. P., Hung, W., Chen, H. J., et al. (2008). Identification of tet (S) gene area in tetracycline-resistant Streptococcus dysgalactiae subsp. equisimilis clinical isolates [2]. J. Antimicrob. Chemother. 61, 453–455. doi: 10.1093/jac/dkm500
Lopetuso, L. R., Giorgio, M. E., Saviano, A., Scaldaferri, F., Gasbarrini, A., and Cammarota, G. (2019). Bacteriocins and bacteriophages: therapeutic weapons for gastrointestinal diseases? Int. J. Mol. Sci. 20:183. doi: 10.3390/ijms20010183
Lupien, A., Gingras, H., Leprohon, P., and Ouellette, M. (2015). Induced tigecycline resistance in Streptococcus pneumoniae mutants reveals mutations in ribosomal proteins and rRNA. J. Antimicrob. Chemother. 70, 2973–2980. doi: 10.1093/jac/dkv211
Macià, M. D., Rojo-Molinero, E., and Oliver, A. (2014). Antimicrobial susceptibility testing in biofilm-growing bacteria. Clin. Microbiol. Infect. 20, 981–990. doi: 10.1111/1469-0691.12651
Maciejewska, B., Olszak, T., and Drulis-Kawa, Z. (2018). Applications of bacteriophages versus phage enzymes to combat and cure bacterial infections: an ambitious and also a realistic application? Appl. Microbiol. Biotechnol. 102, 2563–2581. doi: 10.1007/s00253-018-8811-1
Madubuonu, N., Aisida, S. O., Ali, A., Ahmad, I., Zhao, T.-K., Botha, S., et al. (2019). Biosynthesis of iron oxide nanoparticles via a composite of Psidium guavaja-Moringa oleifera and their antibacterial and photocatalytic study. J. Photochem. Photobiol. B Biol. 199:111601. doi: 10.1016/j.jphotobiol.2019.111601
Mah, T. F., and O’Toole, G. A. (2001). Mechanisms of biofilm resistance to antimicrobial agents. Trends Microbiol. 9, 34–39. doi: 10.1016/S0966-842X(00)01913-2
Majoumouo, M. S., Sibuyi, N. R. S., Tincho, M. B., Mbekou, M., Boyom, F. F., and Meyer, M. (2019). Enhanced anti-bacterial activity of biogenic silver nanoparticles synthesized from terminalia mantaly extracts. Int. J. Nanomed. 14, 9031–9046. doi: 10.2147/IJN.S223447
Malbruny, B., Nagai, K., Coquemont, M., Bozdogan, B., Andrasevic, A. T., Hupkova, H., et al. (2002). Resistance to macrolides in clinical isolates of Streptococcus pyogenes due to ribosomal mutations. J. Antimicrob. Chemother. 49, 935–939. doi: 10.1093/jac/dkf038
Marks, L. R., Mashburn-Warren, L., Federle, M. J., and Hakansson, A. P. (2014). Streptococcus pyogenes biofilm growth in vitro and in vivo and its role in colonization, virulence, and genetic exchange. J. Infect. Dis. 210, 25–34. doi: 10.1093/infdis/jiu058
Martinez-Garriga, B., Vinuesa, T., Hernandez-Borrell, J., and Viñas, M. (2007). The contribution of efflux pumps to quinolone resistance in Streptococcus pneumoniae clinical isolates. Int. J. Med. Microbiol. 297, 187–195. doi: 10.1016/j.ijmm.2007.01.004
Masooleh, A. K., Ahmadikhah, A., and Saidi, A. (2019). Green synthesis of stable silver nanoparticles by the main reduction component of green tea (Camellia sinensis L.). IET Nanobiotechnol. 13, 183–188. doi: 10.1049/iet-nbt.2018.5141
Masri, A., Anwar, A., Khan, N. A., Shahbaz, M. S., Khan, K. M., Shahabuddin, S., et al. (2019a). Antibacterial effects of quinazolin-4(3H)-one functionalized-conjugated silver nanoparticles. Antibiotics 8:179. doi: 10.3390/antibiotics8040179
Masri, A., Anwar, A., Khan, N. A., and Siddiqui, R. (2019b). The use of nanomedicine for targeted therapy against bacterial infections. Antibiotics 8:260. doi: 10.3390/antibiotics8040260
Matsumoto-Nakano, M. (2018). Role of Streptococcus mutans surface proteins for biofilm formation. Jpn. Dent. Sci. Rev. 54, 22–29. doi: 10.1016/j.jdsr.2017.08.002
Matsuzaki, S., Rashel, M., Uchiyama, J., Sakurai, S., Ujihara, T., Kuroda, M., et al. (2005). Bacteriophage therapy: a revitalized therapy against bacterial infectious diseases. J. Infect. Chemother. 11, 211–219. doi: 10.1007/s10156-005-0408-9
McNamara, K., and Tofail, S. A. M. (2017). Nanoparticles in biomedical applications. Adv. Phys. X 2, 54–88. doi: 10.1080/23746149.2016.1254570
Melchior, M. B., Vaarkamp, H., and Fink-Gremmels, J. (2006). Biofilms: a role in recurrent mastitis infections? Vet. J. 171, 398–407. doi: 10.1016/j.tvjl.2005.01.006
Melin, P. (2011). Neonatal group B streptococcal disease: from pathogenesis to preventive strategies. Clin. Microbiol. Infect. 17, 1294–1303. doi: 10.1111/j.1469-0691.2011.03576.x
Meng, X., Shi, Y., Ji, W., Meng, X., Zhang, J., Wang, H., et al. (2011). Application of a bacteriophage lysin to disrupt biofilms formed by the animal pathogen Streptococcus suis. Appl. Environ. Microbiol. 77, 8272–8279. doi: 10.1128/AEM.05151-11
Mingoia, M., Morici, E., Brenciani, A., Giovanetti, E., and Varaldo, P. E. (2015). Genetic basis of the association of resistance genes mef(I) (macrolides) and catQ (chloramphenicol) in streptococci. Front. Microbiol. 6:747. doi: 10.3389/fmicb.2014.00747
Mirza, A. U., Kareem, A., Nami, S. A. A., Khan, M. S., Rehman, S., Bhat, S. A., et al. (2018). Biogenic synthesis of iron oxide nanoparticles using Agrewia optiva and Prunus persica phyto species: characterization, antibacterial and antioxidant activity. J. Photochem. Photobiol. B Biol. 185, 262–274. doi: 10.1016/j.jphotobiol.2018.06.009
Moon, K. S., Park, Y. B., Bae, J. M., and Oh, S. (2018). Near-infrared laser-mediated drug release and antibacterial activity of gold nanorod–sputtered titania nanotubes. J. Tissue Eng. 9:2041731418790315. doi: 10.1177/2041731418790315
Moroi, H., Kimura, K., Kotani, T., Tsuda, H., Banno, H., Jin, W., et al. (2019). Isolation of group B Streptococcus with reduced β-lactam susceptibility from pregnant women. Emerg. Microbes Infect. 8, 2–7. doi: 10.1080/22221751.2018.1557987
Morones, J. R., Elechiguerra, J. L., Camacho, A., Holt, K., Kouri, J. B., Ramírez, J. T., et al. (2005). The bactericidal effect of silver nanoparticles. Nanotechnology 16, 2346–2353. doi: 10.1088/0957-4484/16/10/059
Munita, J. M., Arias, C. A., Unit, A. R., and De Santiago, A. (2016). HHS public access mechanisms of antibiotic resistance. HHS Public Access 4, 1–37. doi: 10.1128/microbiolspec.VMBF-0016-2015
Musser, J. M., Beres, S. B., Zhu, L., Olsen, R. J., Vuopio, J., Hyyryläinen, H.-L., et al. (2020). Reduced in vitro susceptibility of Streptococcus pyogenes to beta-lactam antibiotics associated with mutations in the pbp2x gene is geographically widespread. J. Clin. Microbiol. 58:e0199319. doi: 10.1128/jcm.01993-19
Nakamur, P. A. M., Schuab, R. B. B., Neves, F. P. G., Pereira, C. F. A., de Paula, G. R., and Barros, R. R. (2011). Antimicrobial resistance profiles and genetic characterisation of macrolide resistant isolates of Streptococcus agalactiae. Mem. Inst. Oswaldo Cruz 106, 119–122. doi: 10.1590/S0074-02762011000200001
Neill, J. O. (2014). Antimicrobial Resistance: Tackling a Crisis for the Health and Wealth of Nations. London: The review on antimicrobial resistance.
Neill, J. O. (2016). Tackling drug-Resistant Infections Globally: Final Report and Recommendations. London: The review on antimicrobial resistance.
Nguyen, F., Starosta, A. L., Arenz, S., Sohmen, D., Dönhöfer, A., and Wilson, D. N. (2014). Tetracycline antibiotics and resistance mechanisms. Biol. Chem. 395, 559–575. doi: 10.1515/hsz-2013-0292
Nigam, A., Gupta, D., and Sharma, A. (2014). Treatment of infectious disease: beyond antibiotics. Microbiol. Res. 169, 643–651. doi: 10.1016/j.micres.2014.02.009
Nikaido, H. (2009). Multidrug resistance in bacteria. Annu. Rev. Biochem. 119–146. doi: 10.1146/annurev.biochem.78.082907.145923.Multidrug
Nobbs, A. H., Lamont, R. J., and Jenkinson, H. F. (2009). Streptococcus adherence and colonization. Microbiol. Mol. Biol. Rev. 73, 407–450. doi: 10.1128/mmbr.00014-09
Nur, A., Hirota, K., Yumoto, H., Hirao, K., Liu, D., Takahashi, K., et al. (2013). Effects of extracellular DNA and DNA-binding protein on the development of a Streptococcus intermedius biofilm. J. Appl. Microbiol. 115, 260–270. doi: 10.1111/jam.12202
Oechslin, F. (2018). Resistance development to bacteriophages occurring during bacteriophage therapy. Viruses 10:351. doi: 10.3390/v10070351
Olson, M. E., Ceri, H., Morck, D. W., Buret, A. G., and Read, R. R. (2002). Biofilm bacteria: formation and comparative susceptibility to antibiotics. Can. J. Vet. Res. 66, 86–92.
Orscheln, R. C., Johnson, D. R., Olson, S. M., Presti, R. M., Martin, J. M., Kaplan, E. L., et al. (2005). Intrinsic reduced susceptibility of Serotype 6 Streptococcus pyogenes to fluoroquinolone antibiotics. J. Infect. Dis. 191, 1272–1279. doi: 10.1086/428856
Ortiz-Benítez, E. A., Velázquez-Guadarrama, N., Durán Figueroa, N. V., Quezada, H., and De Jesús Olivares-Trejo, J. (2019). Antibacterial mechanism of gold nanoparticles on: Streptococcus pneumoniae. Metallomics 11, 1265–1276. doi: 10.1039/c9mt00084d
Osei Sekyere, J., and Mensah, E. (2019). Molecular epidemiology and mechanisms of antibiotic resistance in Enterococcus spp., Staphylococcus spp., and Streptococcus spp. in Africa: a systematic review from a One Health perspective. Ann. N. Y. Acad. Sci. 1465, 29–58. doi: 10.1111/nyas.14254
Park, M. J., Eun, I.-S., Jung, C.-Y., Ko, Y.-C., Kim, Y.-J., Kim, C.-K., et al. (2012). Streptococcus dysgalactiae subspecies dysgalactiae infection after total knee arthroplasty: a case report. Knee Surg. Relat. Res. 24, 120–123. doi: 10.5792/ksrr.2012.24.2.120
Park, S., Cha, S. H., Cho, I., Park, S., Park, Y., Cho, S., et al. (2016). Antibacterial nanocarriers of resveratrol with gold and silver nanoparticles. Mater. Sci. Eng. C 58, 1160–1169. doi: 10.1016/j.msec.2015.09.068
Parks, T., Barrett, L., and Jones, N. (2015). Invasive streptococcal disease: a review for clinicians. Br. Med. Bull. 115, 77–89. doi: 10.1093/bmb/ldv027
Pastagia, M., Euler, C., Chahales, P., Fuentes-Duculan, J., Krueger, J. G., and Fischetti, V. A. (2011). A novel chimeric lysin shows superiority to mupirocin for skin decolonization of methicillin-resistant and -sensitive staphylococcus aureus strains. Antimicrob. Agents Chemother. 55, 738–744. doi: 10.1128/AAC.00890-10
Pearl, S., Gabay, C., Kishony, R., Oppenheim, A., and Balaban, N. Q. (2008). Nongenetic individuality in the host-phage interaction. PLoS Biol. 6:e120. doi: 10.1371/journal.pbio.0060120
Pehrsson. (2016). Interconnected microbiomes and resistomes in low-income human habitats. Am. J. Med. Genet. Part A 533, 212–216. doi: 10.1002/ajmg.a.38191
Petchiappan, A., and Chatterji, D. (2017). Antibiotic resistance: current perspectives. ACS Omega 2, 7400–7409. doi: 10.1021/acsomega.7b01368
Peters, J. (2017). Staphylococcal and streptococcal infections key points. Medicine 45, 727–734. doi: 10.1016/j.mpmed.2017.09.010
Petinaki, E., and Papagiannitsis, C. (2018). “Resistance of Staphylococci to Macrolides-Lincosamides-Streptogramins B (MLSB): epidemiology and mechanisms of resistance,” in Staphylococcus aureus eds H. Hemeg, H. Ozbak, and F. Afrin (London: IntechOpen). doi: 10.5772/intechopen.75192
Pham, T. D. M., Ziora, Z. M., and Blaskovich, M. A. T. (2019). Quinolone antibiotics. Medchemcomm 10, 1719–1739. doi: 10.1039/c9md00120d
Pieterse, R., and Todorov, S. D. (2010). Bacteriocins: exploring alternatives to antibiotics in mastitis treatment. Brazilian J. Microbiol. 41, 542–562. doi: 10.1590/S1517-83822010000300003
Pillai, D. R., Shahinas, D., Buzina, A., Pollock, R. A., Lau, R., Khairnar, K., et al. (2009). Genome-wide dissection of globally emergent multi-drug resistant serotype 19A Streptococcus pneumoniae. BMC Genomics 10:642. doi: 10.1186/1471-2164-10-642
Pinho, M. D., Melo-Cristino, J., and Ramirez, M. (2010). Fluoroquinolone resistance in Streptococcus dysgalactiae subsp. equisimilis and evidence for a shared global gene pool with Streptococcus pyogenes. Antimicrob. Agents Chemother. 54, 1769–1777. doi: 10.1128/AAC.01377-09
Pires, R., Ardanuy, C., Rolo, D., Morais, A., Brito-Avô, A., Gonçalo-Marques, J., et al. (2010). Emergence of ciprofloxacin-nonsusceptible Streptococcus pyogenes isolates from healthy children and pediatric patients in Portugal. Antimicrob. Agents Chemother. 54, 2677–2680. doi: 10.1128/AAC.01536-09
Pirnay, J. P., Verbeken, G., Ceyssens, P. J., Huys, I., de Vos, D., Ameloot, C., et al. (2018). The magistral phage. Viruses 10:64. doi: 10.3390/v10020064
Pletz, M. W. R., Mcgee, L., Van Beneden, C. A., Petit, S., Bardsley, M., Barlow, M., et al. (2006). Fluoroquinolone resistance in invasive Streptococcus pyogenes isolates due to spontaneous mutation and horizontal gene transfer. Antimicrob. Agents Chemother. 50, 943–948. doi: 10.1128/AAC.50.3.943-948.2006
Prudhomme, M., Turlan, C., Claverys, J. P., and Chandler, M. (2002). Diversity of Tn4001 transposition products: the flanking IS256 elements can form tandem dimers and IS circles. J. Bacteriol. 184, 433–443. doi: 10.1128/JB.184.2.433-443.2002
Quinteros, M. A., Cano Aristizábal, V., Dalmasso, P. R., Paraje, M. G., and Páez, P. L. (2016). Oxidative stress generation of silver nanoparticles in three bacterial genera and its relationship with the antimicrobial activity. Toxicol. In Vitro 36, 216–223. doi: 10.1016/j.tiv.2016.08.007
Rajagopal, L. (2009). Understanding the regulation of Group B Streptococcal virulence factors. Future Microbiol. 4, 201–221. doi: 10.2217/17460913.4.2.201
Ramasamy, M., Lee, J. H., and Lee, J. (2016). Potent antimicrobial and antibiofilm activities of bacteriogenically synthesized gold-silver nanoparticles against pathogenic bacteria and their physiochemical characterizations. J. Biomater. Appl. 31, 366–378. doi: 10.1177/0885328216646910
Ramirez, M., and Tolmasky, M. E. (2010). Aminoglycoside Modifying Enzymes. Drug Resist Updat. 13, 151–171. doi: 10.1016/j.drup.2010.08.003.Aminoglycoside
Rashel, M., Uchiyama, J., Ujihara, T., Uehara, Y., Kuramoto, S., Sugihara, S., et al. (2007). Efficient Elimination of multidrug-resistant Staphylococcus aureus by cloned lysin derived from Bacteriophage φMR11. J. Infect. Dis. 196, 1237–1247. doi: 10.1086/521305
Rath, D., Amlinger, L., Rath, A., and Lundgren, M. (2015). The CRISPR-Cas immune system: biology, mechanisms and applications. Biochimie 17, 119–28. doi: 10.1016/j.biochi.2015.03.025
Rato, M. G., Bexiga, R., Florindo, C., Cavaco, L. M., Vilela, C. L., and Santos-Sanches, I. (2013). Antimicrobial resistance and molecular epidemiology of streptococci from bovine mastitis. Vet. Microbiol. 161, 286–294. doi: 10.1016/j.vetmic.2012.07.043
Raza, M., Kanwal, Z., Rauf, A., Sabri, A., Riaz, S., and Naseem, S. (2016). Size- and shape-dependent antibacterial studies of silver nanoparticles synthesized by wet chemical routes. Nanomaterials 6:74. doi: 10.3390/nano6040074
Resch, G., Moreillon, P., and Fischetti, V. A. (2011). A stable phage lysin (Cpl-1) dimer with increased antipneumococcal activity and decreased plasma clearance. Int. J. Antimicrob. Agents 38, 516–521. doi: 10.1016/j.ijantimicag.2011.08.009
Reygaert, W. (2018). An overview of the antimicrobial resistance mechanisms of bacteria. AIMS Microbiol. 4, 482–501. doi: 10.3934/microbiol.2018.3.482
Richards, V. P., Zadoks, R. N., Pavinski Bitar, P. D., Lefébure, T., Lang, P., Werner, B., et al. (2012). Genome characterization and population genetic structure of the zoonotic pathogen, Streptococcus canis. BMC Microbiol. 12:293. doi: 10.1186/1471-2180-12-293
Rico-Lastres, P., Díez-Martínez, R., Iglesias-Bexiga, M., Bustamante, N., Aldridge, C., Hesek, D., et al. (2015). Substrate recognition and catalysis by LytB, a pneumococcal peptidoglycan hydrolase involved in virulence. Sci. Rep. 5:16198. doi: 10.1038/srep16198
Rios, A. C., Moutinho, C. G., Pinto, F. C., Del Fiol, F. S., Jozala, A., Chaud, M. V., et al. (2016). Alternatives to overcoming bacterial resistances: state-of-the-art. Microbiol. Res. 191, 51–80. doi: 10.1016/j.micres.2016.04.008
Rios, A. C., Vila, M. M. D. C., Lima, R., Del Fiol, F. S., Tubino, M., Teixeira, J. A., et al. (2018). Structural and functional stabilization of bacteriophage particles within the aqueous core of a W/O/W multiple emulsion: a potential biotherapeutic system for the inhalational treatment of bacterial pneumonia. Process Biochem. 64, 177–192. doi: 10.1016/j.procbio.2017.09.022
Roach, D. R., Leung, C. Y., Henry, M., Morello, E., Singh, D., Di Santo, J. P., et al. (2017). Synergy between the host immune system and bacteriophage is essential for successful phage therapy against an acute respiratory pathogen. Cell Host Microbe 22, 38–47. doi: 10.1016/j.chom.2017.06.018
Rodrigues, G. R., López-Abarrategui, C., de la Serna Gómez, I., Dias, S. C., Otero-González, A. J., and Franco, O. L. (2019). Antimicrobial magnetic nanoparticles based-therapies for controlling infectious diseases. Int. J. Pharm 555, 356–367. doi: 10.1016/j.ijpharm.2018.11.043
Rohde, M., and Cleary, P. P. (2016). “Adhesion and invasion of Streptococcus pyogenes into host cells and clinical relevance of intracellular streptococci,” in Streptococcus pyogenes: Basic Biology to Clinical Manifestations eds J. J. Ferretti, D. L. Stevens, and V. A. Fischetti (Oklahoma City, OK: University of Oklahoma Health Sciences Center). Available online at: https://www.ncbi.nlm.nih.gov/books/NBK333420/ (accessed August 4, 2020).
Rosini, R., and Margarit, I. (2015). Biofilm formation by Streptococcus agalactiae: influence of environmental conditions and implicated virulence factors. Front. Cell. Infect. Microbiol. 5:6. doi: 10.3389/fcimb.2015.00006
Rotello, V. M., Gupta, A., and Landis, R. F. (2016). Nanoparticle-based antimicrobials: surface functionality is critical. F1000Research 5:F1000 Faculty Rev-364. doi: 10.12688/f1000research.7595.1
Ryan, M. P., Meaney, W. J., Ross, R. P., and Hill, C. (1998). Evaluation of lacticin 3147 and a teat seal containing this bacteriocin for inhibition of mastitis pathogens. Appl. Environ. Microbiol. 64, 2287–2290. doi: 10.1128/aem.64.6.2287-2290.1998
Ryan, M. P., Rea, M. C., Hill, C., and Ross, R. P. (1996). An application in cheddar cheese manufacture for a strain of Lactococcus lactis producing a novel broad-spectrum bacteriocin, lacticin 3147. Appl. Environ. Microbiol. 62, 612–619. doi: 10.1128/aem.62.2.612-619.1996
Sadony, D. M., and Abozaid, H. E. (2020). Antibacterial effect of metallic nanoparticles on Streptococcus mutans bacterial strain with or without diode laser (970 nm). Bull. Natl. Res. Cent. 44, 2–7. doi: 10.1186/s42269-019-0262-z
Salari, S., Bahabadi, S. E., Samzadeh-Kermani, A., and Yosefzaei, F. (2019). In-vitro evaluation of antioxidant and antibacterial potential of green synthesized silver nanoparticles using prosopis farcta fruit extract. Iran. J. Pharm. Res. 18, 430–445. doi: 10.22037/ijpr.2019.2330
Sánchez-López, E., Gomes, D., Esteruelas, G., Bonilla, L., Lopez-Machado, A. L., Galindo, R., et al. (2020). Metal-based nanoparticles as antimicrobial agents: an overview. Nanomaterials 10:292. doi: 10.3390/nano10020292
Santoro, F., Vianna, M. E., and Roberts, A. P. (2014). Variation on a theme; an overview of the Tn916/Tn1545 family of mobile genetic elements in the oral and nasopharyngeal streptococci. Front. Microbiol. 5:535. doi: 10.3389/fmicb.2014.00535
Saqib, S., Munis, M. F. H., Zaman, W., Ullah, F., Shah, S. N., Ayaz, A., et al. (2019). Synthesis, characterization and use of iron oxide nano particles for antibacterial activity. Microsc. Res. Tech. 82, 415–420. doi: 10.1002/jemt.23182
Sardella, D., Gatt, R., and Valdramidis, V. P. (2018). Assessing the efficacy of zinc oxide nanoparticles against Penicillium expansum by automated turbidimetric analysis. Mycology 9, 43–48. doi: 10.1080/21501203.2017.1369187
Schmelcher, M., Donovan, D. M., and Loessner, M. J. (2012). Bacteriophage endolysins as novel antimicrobials. Future Microbiol. 7, 1147–1171. doi: 10.2217/fmb.12.97
Schwarz, S., Kehrenberg, C., Doublet, B., and Cloeckaert, A. (2004). Molecular basis of bacterial resistance to chloramphenicol and florfenicol. FEMS Microbiol. Rev. 28, 519–542. doi: 10.1016/j.femsre.2004.04.001
Shaikh, S., Nazam, N., Rizvi, S. M. D., Ahmad, K., Baig, M. H., Lee, E. J., et al. (2019). Mechanistic insights into the antimicrobial actions of metallic nanoparticles and their implications for multidrug resistance. Int. J. Mol. Sci. 20:2468. doi: 10.3390/ijms20102468
Shaker, M. A., and Shaaban, M. I. (2017). Formulation of carbapenems loaded gold nanoparticles to combat multi-antibiotic bacterial resistance: in vitro antibacterial study. Int. J. Pharm. 525, 71–84. doi: 10.1016/j.ijpharm.2017.04.019
Shen, Y., Köller, T., Kreikemeyer, B., and Nelson, D. C. (2013). Rapid degradation of Streptococcus pyogenes biofilms by PlyC, a bacteriophage-encoded endolysin. J. Antimicrob. Chemother. 68, 1818–1824. doi: 10.1093/jac/dkt104
Sholkamy, E. N., Ahamd, M. S., Yasser, M. M., and Eslam, N. (2019). Anti-microbiological activities of bio-synthesized silver Nano-stars by Saccharopolyspora hirsuta. Saudi J. Biol. Sci. 26, 195–200. doi: 10.1016/j.sjbs.2018.02.020
Silva, L. G., Genteluci, G. L., de Mattos, M. C., Glatthardt, T., Sà Figueiredo, A. M., and Ferreira-Carvalho, B. T. (2015). Group C Streptococcus dysgalactiae subsp. equisimilis in south-east Brazil: genetic diversity, resistance profile and the first report of human and equine isolates belonging to the same multilocus sequence typing lineage. J. Med. Microbiol. 64, 551–558. doi: 10.1099/jmm.0.000052
Stern, A., and Sorek, R. (2011). The phage-host arms-race: shaping the evolution of microbes. Bioessays 33, 43–51. doi: 10.1002/bies.201000071
Tagg, J., Wescombe, P., and Burton, J. (2006). Oral streptococcal BLIS: heterogeneity of the effector molecules and potential role in the prevention of streptococcal infections. Int. Congr. Ser. 1289, 347–350. doi: 10.1016/j.ics.2005.11.016
Tagg, J. R. (2004). Prevention of streptococcal pharyngitis by anti-Streptococcus pyogenes bacteriocin-like inhibitory substances (BLIS) produced by Streptococcus salivarius. Indian J. Med. Res. Suppl. 119, 13–16.
Tao, C. (2018). Antimicrobial activity and toxicity of gold nanoparticles: research progress, challenges and prospects. Lett. Appl. Microbiol. 67, 537–543. doi: 10.1111/lam.13082
Tolinački, M., Kojić, M., Lozo, J., Terzić-Vidojević, A., Topisirović, L., and Fira, D. (2010). Characterization of the bacteriocin-producing strain Lactobacillus paracasei subsp. Paracasei BGUB9. Arch. Biol. Sci. 62, 889–899. doi: 10.2298/ABS1004889T
Tong, Z., Dong, L., Zhou, L., Tao, R., and Ni, L. (2010). Nisin inhibits dental caries-associated microorganism in vitro. Peptides 31, 2003–2008. doi: 10.1016/j.peptides.2010.07.016
Tong, Z., Zhou, L., Li, J., Jiang, W., Ma, L., and Ni, L. (2011). In vitro evaluation of the antibacterial activities of MTAD in combination with nisin against Enterococcus faecalis. J. Endod. 37, 1116–1120. doi: 10.1016/j.joen.2011.03.020
Toodehzaeim, M. H., Zandi, H., Meshkani, H., and Firouzabadi, A. H. (2018). The effect of CuO nanoparticles on antimicrobial Effects and shear bond strength of orthodontic adhesives. J. Dentristy 19, 1–5.
Trappetti, C., Ogunniyi, A. D., Oggioni, M. R., and Paton, J. C. (2011). Extracellular matrix formation enhances the ability of Streptococcus pneumoniae to cause invasive disease. PLoS One 6:e19844. doi: 10.1371/journal.pone.0019844
Trieu-Cuot, P., De Cespedes, G., Bentorcha, F., Delbos, F., Gaspar, E., and Horaud, T. (1993). Study of heterogeneity of chloramphenicol acetyltransferase (CAT) genes in streptococci and enterococci by polymerase chain reaction: Characterization of a new CAT determinant. Antimicrob. Agents Chemother. 37, 2593–2598. doi: 10.1128/AAC.37.12.2593
Van Hoang, K., Stern, N. J., Saxton, A. M., Xu, F., Zeng, X., and Lin, J. (2011). Prevalence, development, and molecular mechanisms of bacteriocin resistance in Campylobacter. Appl. Environ. Microbiol. 77, 2309–2316. doi: 10.1128/AEM.02094-10
Van Meervenne, E., De Weirdt, R., Van Coillie, E., Devlieghere, F., Herman, L., and Boon, N. (2014). Biofilm models for the food industry: Hot spots for plasmid transfer? Pathog. Dis. 70, 332–338. doi: 10.1111/2049-632X.12134
Vannice, K. S., Ricaldi, J., Nanduri, S., Fang, F. C., Lynch, J. B., Bryson-Cahn, C., et al. (2019). Streptococcus pyogenes pbp2x mutation confers reduced susceptibility to β-Lactam antibiotics. Clin. Infect. Dis. 71, 201–204. doi: 10.1093/cid/ciz1000
Vélez, J. R., Cameron, M., Rodríguez-Lecompte, J. C., Xia, F., Heider, L. C., Saab, M., et al. (2017). Whole-genome sequence analysis of antimicrobial resistance genes in Streptococcus uberis and Streptococcus dysgalactiae isolates from Canadian dairy herds. Front. Vet. Sci. 4:63. doi: 10.3389/fvets.2017.00063
Vieira, A. P. M., Arias, L. S., de Souza Neto, F. N., Kubo, A. M., Lima, B. H. R., de Camargo, E. R., et al. (2019). Antibiofilm effect of chlorhexidine-carrier nanosystem based on iron oxide magnetic nanoparticles and chitosan. Colloids Surfaces B Biointerfaces 174, 224–231. doi: 10.1016/j.colsurfb.2018.11.023
Vinotha, V., Iswarya, A., Thaya, R., Govindarajan, M., Alharbi, N. S., Kadaikunnan, S., et al. (2019). Synthesis of ZnO nanoparticles using insulin-rich leaf extract: anti-diabetic, antibiofilm and anti-oxidant properties. J. Photochem. Photobiol. B Biol. 197, 111541. doi: 10.1016/j.jphotobiol.2019.111541
Von Wintersdorff, C. J. H., Penders, J., Van Niekerk, J. M., Mills, N. D., Majumder, S., Van Alphen, L. B., et al. (2016). Dissemination of antimicrobial resistance in microbial ecosystems through horizontal gene transfer. Front. Microbiol. 7:173. doi: 10.3389/fmicb.2016.00173
Walls, T., Power, D., and Tagg, J. (2003). Bacteriocin-like inhibitory substance (BLIS) production by the normal flora of the nasopharynx: Potential to protect against otitis media? J. Med. Microbiol. 52, 829–833. doi: 10.1099/jmm.0.05259-0
Wang, Y., Wan, J., Miron, R. J., Zhao, Y., and Zhang, Y. (2016). Antibacterial properties and mechanisms of gold-silver nanocages. Nanoscale 8, 11143–11152. doi: 10.1039/c6nr01114d
Wescombe, P. A., Dyet, K. H., Dierksen, K. P., Power, D. A., Jack, R. W., Burton, J. P., et al. (2012). Salivaricin G32, a homolog of the prototype streptococcus pyogenes nisin-like lantibiotic SA-FF22, produced by the commensal species streptococcus salivarius. Int. J. Microbiol. 2012:738503. doi: 10.1155/2012/738503
Wirawan, R. E., Klesse, N. A., Jack, R. W., and Tagg, J. R. (2006). Molecular and genetic characterization of a novel nisin variant produced by Streptococcus uberis. Appl. Environ. Microbiol. 72, 1148–1156. doi: 10.1128/AEM.72.2.1148-1156.2006
Wong, S. S. Y., and Yuen, K. Y. (2012). Streptococcus pyogenes and re-emergence of scarlet fever as a public health problem. Emerg. Microbes Infect. 1:e2. doi: 10.1038/emi.2012.9
Woodford, N. (2005). Biological counterstrike: antibiotic resistance mechanisms of Gram-positive cocci. Clin. Microbiol. Infect. Suppl. 11, 2–21. doi: 10.1111/j.1469-0691.2005.01140.x
World Health Organization (2020). Available online at: https://www.who.int/news-room/detail/17-01-2020-lack-of-new-antibiotics-threatens-global-efforts-to-contain-drug-resistant-infections (accessed August 4, 2020).
Yamada, S., Shibasaki, M., Murase, K., Watanabe, T., Aikawa, C., Nozawa, T., et al. (2019). Phylogenetic relationship of prophages is affected by CRISPR selection in Group A Streptococcus. BMC Microbiol. 19:24. doi: 10.1186/s12866-019-1393-y
Yan, W., Yang, L., Wang, H., Zhang, J., and Shen, W. (2018). Atomic-engineered gold@silvergold alloy nanoflowers for in vivo inhibition of bacteria. Nanoscale 10, 15661–15668. doi: 10.1039/C8NR04196B
Yin, I. X., Yu, O. Y., Zhao, I. S., Mei, M. L., Li, Q. L., Tang, J., et al. (2019). Developing biocompatible silver nanoparticles using epigallocatechin gallate for dental use. Arch. Oral Biol. 102, 106–112. doi: 10.1016/j.archoralbio.2019.03.022
Young, C., Holder, R. C., and Reid, S. D. (2016). “Streptococcus pyogenes Biofilm”, in Streptococcus pyogenes: Basic Biology to Clinical Manifestations eds J. J. Ferretti, D. L. Stevens, and V. A. Fischetti (Oklahoma City, OK: University of Oklahoma Health Sciences Center). Available online at: https://www.ncbi.nlm.nih.gov/books/NBK333419/ (accessed August 4, 2020).
Keywords: antimicrobial resistance, biofilms, pyogenic streptococci, bacteriocins, bacteriophage, nanoparticles, nanomedicine
Citation: Alves-Barroco C, Rivas-García L, Fernandes AR and Baptista PV (2020) Tackling Multidrug Resistance in Streptococci – From Novel Biotherapeutic Strategies to Nanomedicines. Front. Microbiol. 11:579916. doi: 10.3389/fmicb.2020.579916
Received: 03 July 2020; Accepted: 16 September 2020;
Published: 06 October 2020.
Edited by:
Rafael Peña-Miller, National Autonomous University of Mexico, MexicoReviewed by:
Maria Blanca Sanchez, Instituto IMDEA Agua, SpainChang-Ro Lee, Myongji University, South Korea
Copyright © 2020 Alves-Barroco, Rivas-García, Fernandes and Baptista. This is an open-access article distributed under the terms of the Creative Commons Attribution License (CC BY). The use, distribution or reproduction in other forums is permitted, provided the original author(s) and the copyright owner(s) are credited and that the original publication in this journal is cited, in accordance with accepted academic practice. No use, distribution or reproduction is permitted which does not comply with these terms.
*Correspondence: Alexandra R. Fernandes, bWEuZmVybmFuZGVzQGZjdC51bmwucHQ=; Pedro Viana Baptista, cG12YkBmY3QudW5sLnB0