- 1Immunoendocrinology, Division of Medical Biology, Department of Pathology and Medical Biology, University Medical Center Groningen, University of Groningen, Groningen, Netherlands
- 2Laboratory of Food Chemistry, Wageningen University & Research, Wageningen, Netherlands
- 3FrieslandCampina, Amersfoort, Netherlands
- 4Stratingh Institute for Chemistry, University of Groningen, Groningen, Netherlands
- 5Department of Medical Microbiology, University Medical Center Groningen, University of Groningen, Groningen, Netherlands
Human milk oligosaccharides (hMOs) are important bioactive components in mother’s milk contributing to infant health by supporting colonization and growth of gut microbes. In particular, Bifidobacterium genus is considered to be supported by hMOs. Approximately 200 different hMOs have been discovered and characterized, but only a few abundant hMOs can be produced in sufficient amounts to be applied in infant formula. These hMOs are usually supplied in infant formula as single molecule, and it is unknown which and how individual hMOs support growth of individual gut bacteria. To investigate how individual hMOs influence growth of several relevant intestinal bacteria species, we studied the effects of three hMOs (2′-fucosyllactose, 3-fucosyllactose, and 6′-sialyllactose) and an hMO acid hydrolysate (lacto-N-triose) on three Bifidobacteria and one Faecalibacterium and introduced a co-culture system of two bacterial strains to study possible cross-feeding in presence and absence of hMOs. We observed that in monoculture, Bifidobacterium longum subsp. infantis could grow well on all hMOs but in a structure-dependent way. Faecalibacterium prausnitzii reached a lower cell density on the hMOs in stationary phase compared to glucose, while B. longum subsp. longum and Bifidobacterium adolescentis were not able to grow on the tested hMOs. In a co-culture of B. longum subsp. infantis with F. prausnitzii, different effects were observed with the different hMOs; 6′-sialyllactose, rather than 2′-fucosyllactose, 3-fucosyllactose, and lacto-N-triose, was able to promote the growth of B. longum subsp. infantis. Our observations demonstrate that effects of hMOs on the tested gut microbiota are hMO-specific and provide new means to support growth of these specific beneficial microorganisms in the intestine.
Introduction
It is widely accepted that breastfeeding is the gold standard for infant nutrition, which offers complete nutrition for the newborn. Mother’s milk contains bioactive components that contribute to the healthy development of the newborn (Le Doare et al., 2018). For these reasons, the World Health Organization (WHO) recommends to feed infants for at least 6 months exclusively with breastfeeding (World Health Organization [WHO], 2002; Walker, 2010). However, for a variety of reasons, there are still over 70% of the infants that cannot be exclusively breastfed (Walker, 2010; Heymann et al., 2013). These non-breastfed infants are most often fed with cow-derived infant formula (Coulet et al., 2014; Aly et al., 2018). Up to now, these cow-milk derived infant formula lack human milk oligosaccharides (hMOs), which are one of the most important bioactive components of mother’s milk. These hMOs are unique to humans and provide numerous health-promoting effects (Bode, 2015; Triantis et al., 2018). Until recently, it was not possible to produce hMOs in large amounts for the application in infant formula, but this lately changed. Major advances have been made in large-scale production of hMOs allowing application of a few abundant hMOs in infant formula (Vandenplas et al., 2018).
The beneficial effects of hMOs in human milk are well-established and numerous (Bode, 2015; Cheng et al., 2019, 2020; Kong et al., 2019). One important effect of hMOs is considered to be the support of growth of beneficial gut bacteria (Thomson et al., 2018). The first year of a baby’s life is critical for the establishment of the intestinal microbiome, and hMOs are an important factor in shaping the gut microbiome in the first year of life (Goldsmith et al., 2015). It is, however, still unclear which and how individual hMOs, which are already applied or considered for infant formula, support growth of individual gut bacteria. Bifidobacterium is one of the dominant species in the intestine of healthy breastfed infants, and can represent up to 90% of the total microbiome (Moore and Townsend, 2019). hMOs are specifically known to support the growth of Bifidobacterium genus (Thomson et al., 2018), e.g., Bifidobacterium longum subsp. infantis, a strong hMO user that grows well when cultured with hMOs isolated from human milk as the sole carbohydrate source (Underwood et al., 2015). However, whether individual hMOs currently developed for infant formula can also influence the growth of B. longum subsp. infantis is unknown.
The infant intestine needs, however, fast colonization not only by Bifidobacterium genus but also by other species that contribute to making fermentation products such as short-chain fatty acids (SCFAs) that support metabolism and immunity (Conlon and Bird, 2015). Some bacteria ferment hMO and other carbohydrates to produce SCFAs such as acetate, propionate, and butyrate, which are an important energy source for intestinal epithelium, and modulate epithelial integrity (McLeod et al., 2019). A potent SCFA producer is, for example, Faecalibacterium prausnitzii, which produces butyrate. This bacterium colonizes the gut during late infancy and is one of the most dominant bacterial species in the large intestine of healthy adults (Laursen et al., 2017). Although its importance for a healthy gut is broadly recognized, it is unknown how F. prausnitzii behaves when exposed to hMOs. It is also not known how F. prausnitzii is influenced by already present Bifidobacterium genus, and whether it benefits from cross-feeding of hMO fermented by Bifidobacterium genus.
To gain more insight into how currently applied or proposed hMOs for infant formula influence growth of several relevant intestinal species, we studied the effects of three hMOs 2′-fucosyllactose (2′-FL), 3-fucosyllactose (3-FL), 6′-sialyllactose (6′-SL) and one hMO’s acid hydrolysate lacto-N-triose (LNT2) on B. longum subsp. infantis, B. longum subsp. longum, Bifidobacterium adolescentis, and F. prausnitzii. We first used individual hMOs as the only carbohydrate source for different bacterial strains in monoculture to investigate whether individual hMOs can modulate single-strain growth. Then, B. longum subsp. infantis and F. prausnitzii were brought into co-culture to study the possible interaction between these two bacteria strains. The fermentation products of B. longum subsp. infantis and F. prausnitzii as well as glycosidic degradation of effective hMOs under mono- and co-culture systems were analyzed.
Results
Effects on Bacterial Growth of 2′-FL, 3-FL, 6′-SL, and LNT2 Were Bacterial Strain Dependent
Figure 1 shows the growth curves of B. longum subsp. infantis, B. longum subsp. longum, B. adolescentis, and F. prausnitzii in YCFA broth with glucose, 2′-FL, 3-FL, 6′-SL, or LNT2 as the single carbon source. We found that all bacterial strains were able to grow on glucose, and the effects of 2′-FL, 3-FL, 6′-SL, and LNT2 were variations with the tested strain. B. longum subsp. infantis could grow on all the substrates we provided, but the effects were hMO structure-dependent (Figure 1A). When grown in the presence of 2′-FL, B. longum subsp. infantis could reach OD600 of 3.6 and reach stationary phase after 56 h of culture. On 3-FL, it grew to an OD600 of 1.7 and reached stationary phase after 32 h of culture, which was 24 h earlier than on 2′-FL. With 6′-SL, the growth of B. longum subsp. infantis started at 48 h of culture, which is much slower than with the other substrates, and reached an OD600 of 1.6 after 64 h of culture. On LNT2, B. longum subsp. infantis grew to an OD600 of 1.2 and reached stationary phase after 32 h of culture. In the presence of 2′-FL, the OD600 of 3.6 of B. longum subsp. infantis in stationary phase was significantly higher than with 3-FL, 6′-SL, and LNT2 as the carbon source (p < 0.0001, Supplementary Figure S1). In contrast, B. longum subsp. longum only reached a high cell density (OD600 > 1) on glucose, where the OD600 was 2.8 after 32 h of culture. None of the tested hMOs was able to support the growth of B. longum subsp. longum (Figure 1B). The growth behavior of B. adolescentis was similar to that observed for B. longum subsp. longum. On glucose, B. adolescentis grew to a high OD600 of 3.9 after 32 h of culture, but was not able to grow on the different hMOs and on the hMOs acid hydrolysis product LNT2 (Figure 1C). F. prausnitzii reached a lower cell density on the hMOs compared to glucose, and the growth pattern was hMO structure-dependent (Figure 1D). F. prausnitzii quickly reached a final cell density at OD600 of 1.8 after 12 h of culture on glucose. On 2′-FL and 3-FL, it reached OD600 0.2 in the stationary phase, while with LNT2, it grew to OD600 0.3 at the endpoint. With 6′-SL, the OD600 reached 0.5 at the endpoint, which was higher than on 2′-FL, 3-FL, and LNT2, but still significantly lower than on glucose (p < 0.0001, Supplementary Figure S1). For the subsequent cross-feeding studies, we selected two bacterial strains to determine whether and which hMOs may impact the growth of the two beneficial bacteria. We chose two essential early life colonizing bacteria; B. longum subsp. infantis, which is a potent hMO user, and F. prausnitzii, which is a major anti-inflammatory commensal bacterium in early life in the gut (Sokol et al., 2008) and a less capable utilizer of hMOs.
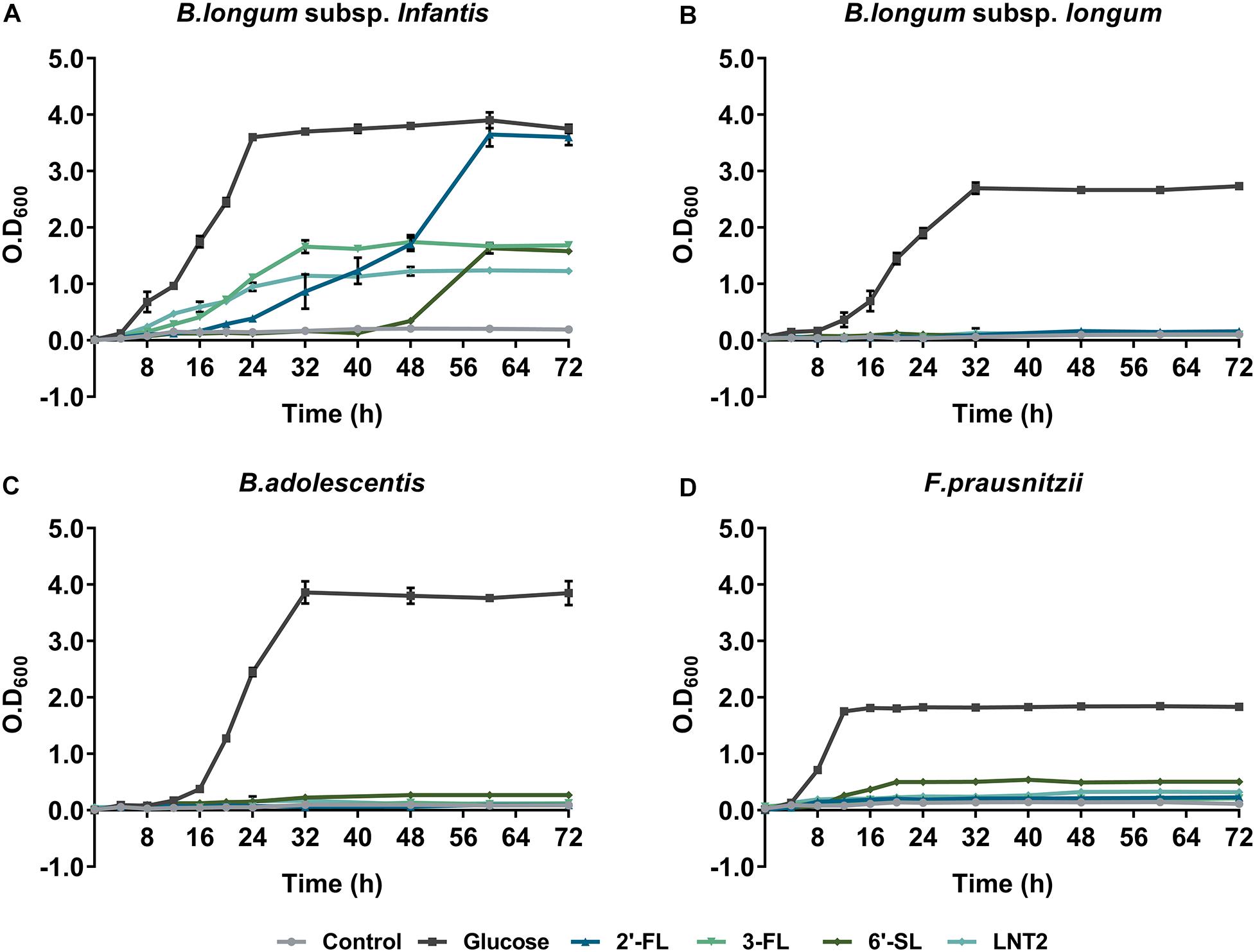
Figure 1. The growth curve of (A) B. longum subsp. infantis, (B) B. longum subsp. longum, (C) B. adolescentis, and (D) F. prausnitzii in monoculture, determined by OD600. Glucose, 2′-FL, 3-FL, 6′-SL, and LNT2 were included as a sole carbon source as indicated. Basal broth with no carbohydrates added was used as negative control. The assays were carried out three times in duplicate. A representative curve for each condition is shown.
The Co-culture System Can Promote the Growth of the Bacteria
We found that co-culture of B. longum subsp. infantis and F. prausnitzii resulted in different growth rates compared to monoculture and that it was influenced by the type of hMO. In the presence of 2′-FL, co-culture and B. longum subsp. infantis monoculture cell densities reached similar values (Figure 2A). However, bacteria grew significantly faster in co-cultures than in B. longum subsp. infantis monocultures, as co-cultures reached stationary phase after 32 h compared to 56 h for the monoculture of B. longum subsp. infantis (Figure 2A). In the presence of 3-FL, co-culture and B. longum subsp. infantis monoculture reached a similar cell density, 1.80 and 1.75 at OD600, respectively, while the F. prausnitzii monoculture reached OD600 0.2 at the endpoint (Figure 2B). Interestingly, in the presence of 6′-SL, the growth curves showed a similar trend as observed with 2′-FL, with the co-culture growing faster than the B. longum subsp. infantis monoculture, albeit at an overall delayed time. The B. longum subsp. infantis and F. prausnitzii co-culture reached stationary phase after 48 h culturing, while the B. longum subsp. infantis monoculture just started to grow at this time point. It took 16 additional hours before the B. longum subsp. infantis monoculture reached the stationary phase. At this faster growth, the OD600 in stationary phase was 1.8 in co-culture, 1.6 in B. longum subsp. infantis monoculture, and 0.5 in F. prausnitzii monoculture (Figure 2C). With LNT2 as carbon source, co-cultures and monocultures of B. longum subsp. infantis reached stationary phases on similar time, but in co-culture, it reached a higher cell density in stationary phase of 1.5 at OD600, while in the monocultures of B. longum subsp. infantis and F. prausnitzii, the cell density was only 1.15 and 0.3 at OD600, respectively, in the stationary phase (Figure 2D). The different growth rates and final cell density differences in co-cultures and monocultures containing 2′-FL, 3-FL, 6′-SL, and LNT2 as carbon source indicate that the bacteria influence each other’s growth pattern and promote the growth of the bacteria in an hMO structure-dependent way.
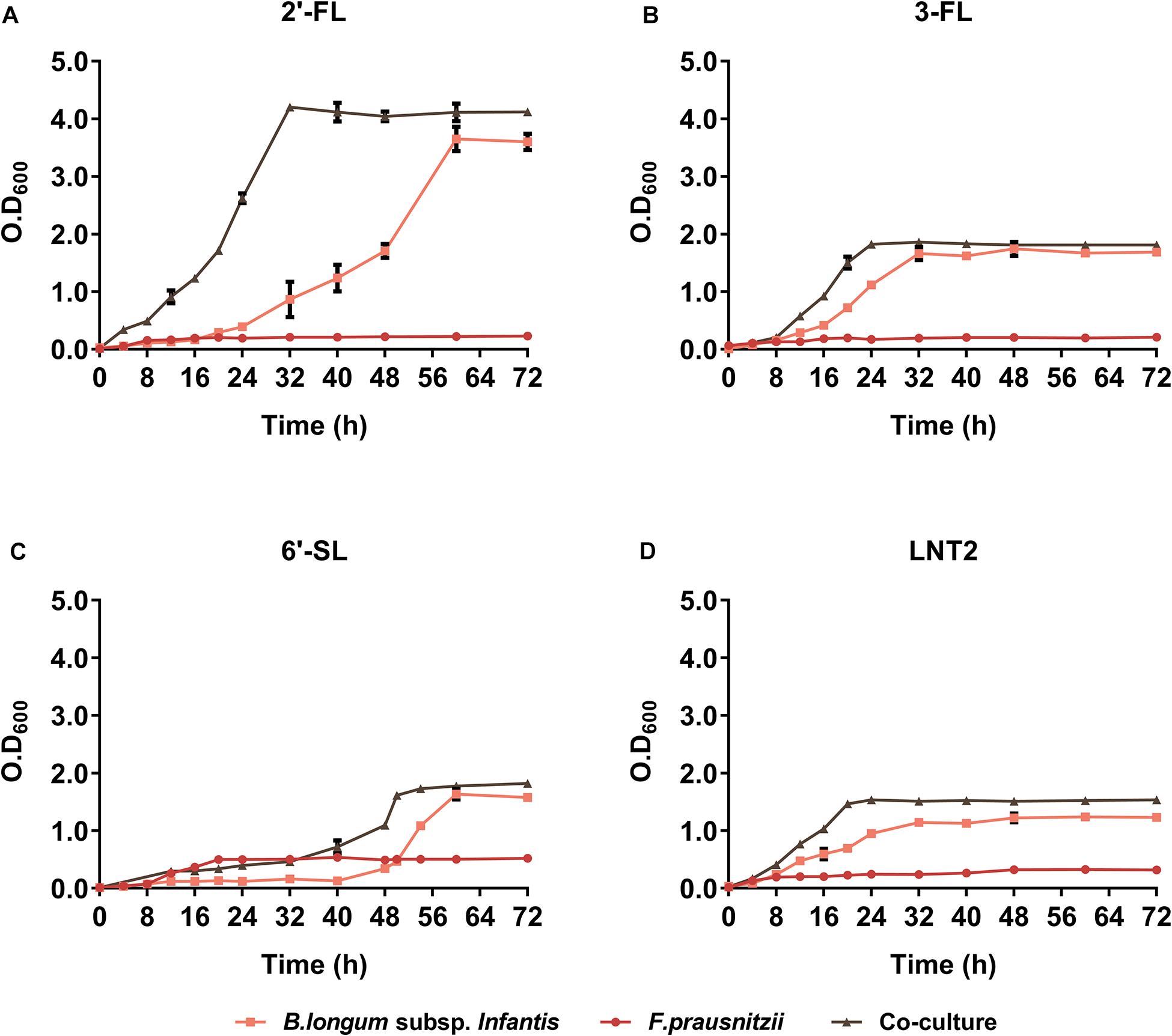
Figure 2. The growth curve of B. longum subsp. infantis and F. prausnitzii in monoculture and co-cultures, determined at OD600. (A) 2′-FL, (B) 3-FL, (C) 6′-SL, and (D) LNT2 were included as carbon source. The fermentations were carried out three times in duplicate. A representative curve for each condition is shown.
2′-FL, 3-FL, and LNT2 Do Not Enhance SCFA Production in Co-cultures While 6′-SL Promotes Acetate Production
As B. longum subsp. infantis ferments the hMOs (Ward et al., 2007), we also investigated the production of SCFAs in the mono- and co-cultures (Figures 3, 4). SCFAs are one of the most important metabolic products of the bacteria and reflects activity of the metabolic processes. To this end, we quantified the SCFAs acetate, propionic acid, and butyrate after 48 h and 72 h of monoculture and co-culture. The concentrations of the produced metabolites were calculated by subtracting the initial values at 0 h.
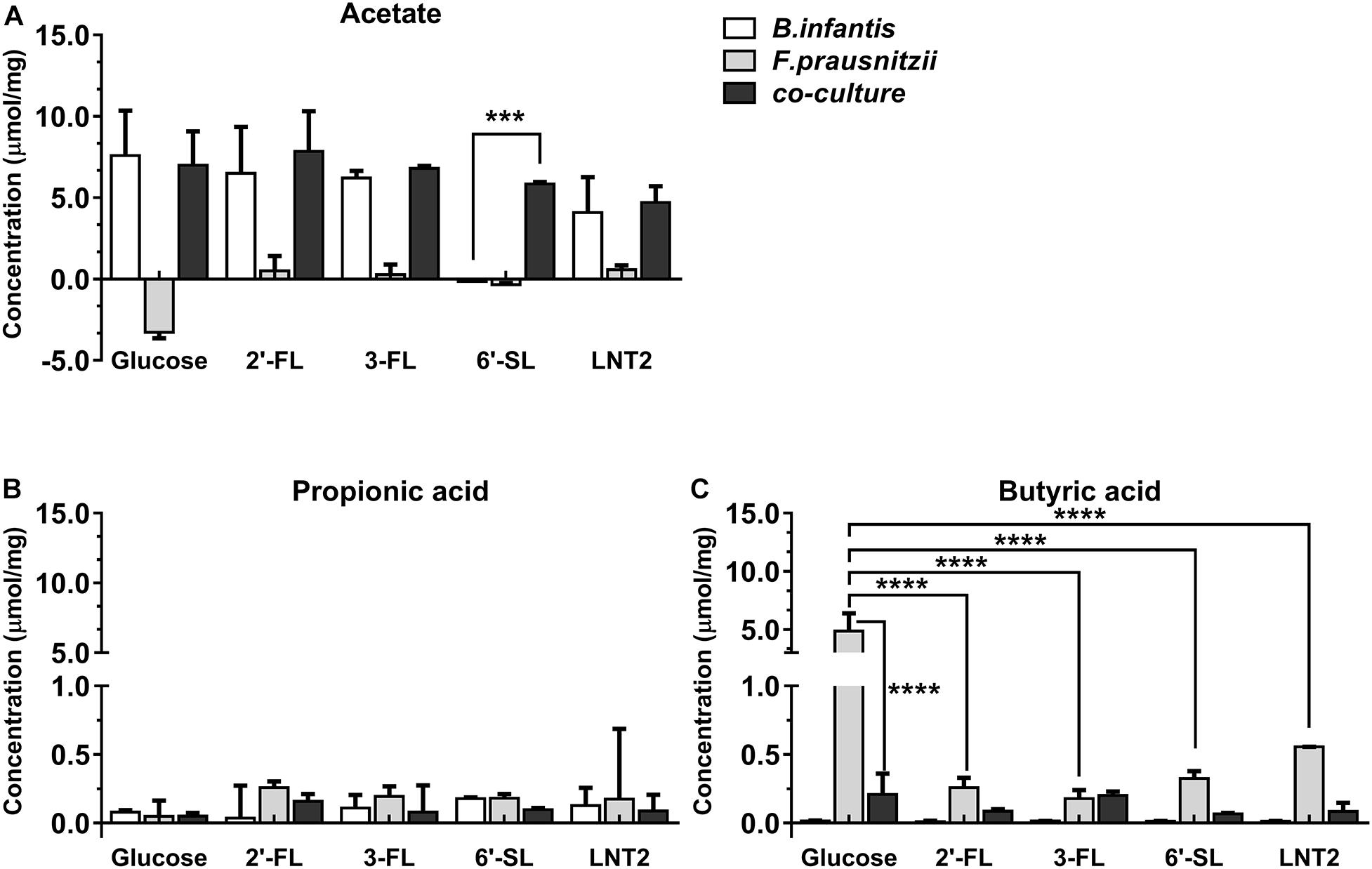
Figure 3. SCFA production of glucose, 2′-FL, 3-FL, 6′-SL, and LNT2 in monoculture and co-cultures of B. longum subsp. infantis and F. prausnitzii after 48-h cultures. The acetate (A), propionic acid (B), and butyrate (C) products of B. longum subsp. infantis and F. prausnitzii were measured after 48 h of monoculture and co-cultures when having either 2′-FL, 3-FL, 6′-SL, and LNT2 as carbon source. Glucose served as positive control. Values are changes in concentrations calculated by subtracting the initial values from 0 h. Data are presented as median ± range (n = 3). Statistical significance was measured using Kruskal–Wallis test followed by the Dunn’s test and indicated by *p < 0.05, **p < 0.01, ***p < 0.001, or by ****p < 0.0001.
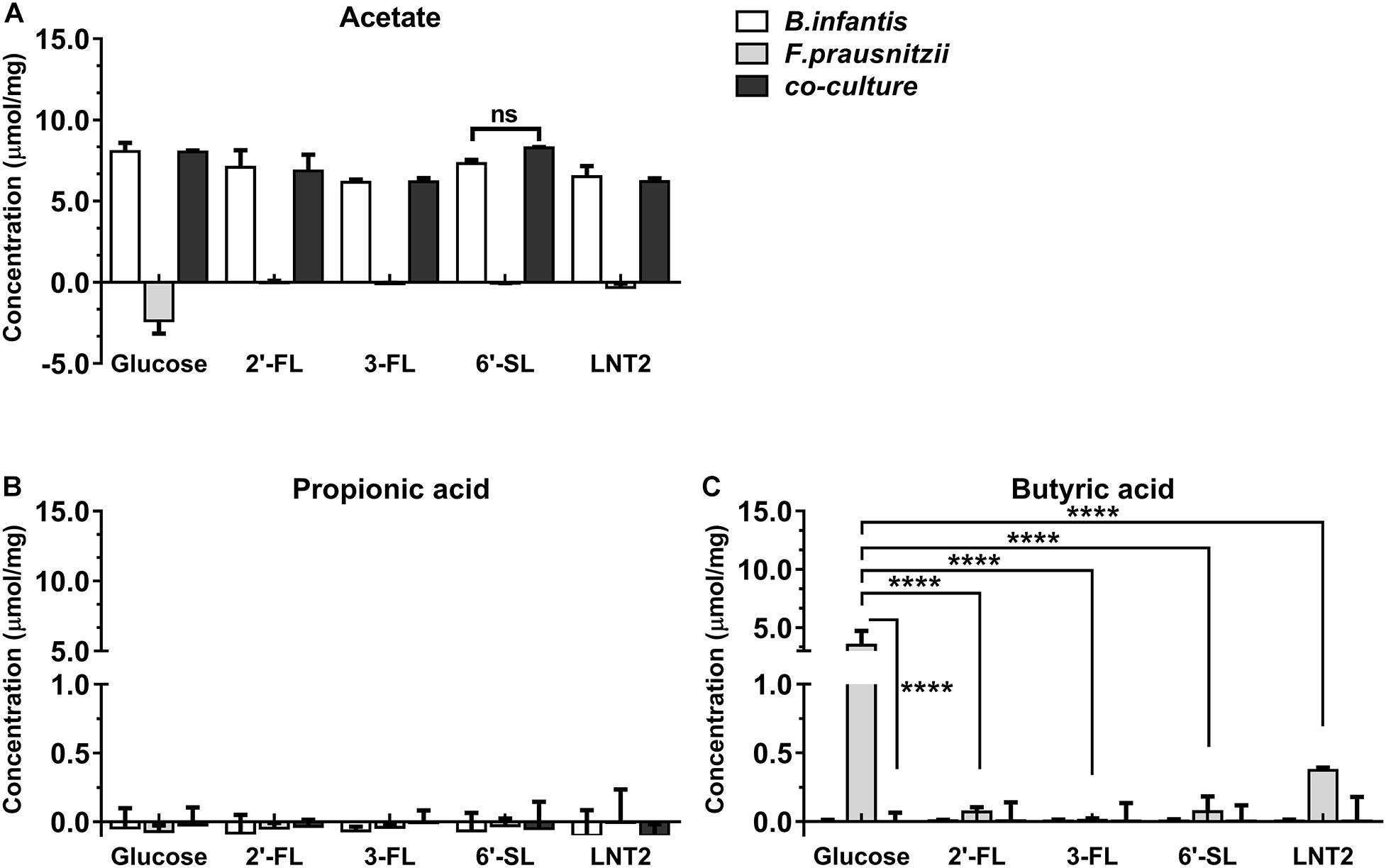
Figure 4. SCFA production of glucose, 2′-FL, 3-FL, 6′-SL, and LNT2 in monoculture and co-cultures of B. longum subsp. infantis and F. prausnitzii after 72-h cultures. The acetate (A), propionic acid (B), and butyrate (C) products of B. longum subsp. infantis and F. prausnitzii were measured after 72 h of monoculture and co-cultures when having either 2′-FL, 3-FL, 6′-SL, and LNT2 as carbon source. Glucose served as positive control. Values are changes in concentrations calculated by subtracting the initial values from 0 h. Data are presented as median ± range (n = 3). Statistical significance was measured using Kruskal–Wallis test followed by the Dunn’s test and indicated by *p < 0.05, **p < 0.01, ***p < 0.001, or by ****p < 0.0001.
Bifidobacterium longum subsp. infantis in monoculture with glucose as carbon source (control) produced a high concentration of acetic acid and minor amounts of propionic acid and butyric acid at both 48 h and 72 h. Results were different when 2′-FL, 3-FL, 6′-SL, and LNT2 were used as carbon sources. With 2′-FL, 3-FL, and LNT2 as carbon source in monoculture with B. longum subsp. infantis, we observed that no significant differences were detected compared to glucose at 48 and 72 h (Figures 3A, 4A). While on 6′-SL, no acetate was detected after 48 h monoculture, and 7.31 μmol/mg acetate was produced after 72 h (Figures 3A, 4A). With F. prausnitzii, grown on glucose as carbon source, F. prausnitzii consumed acetate in the broth and produced butyric acid and propionic acid in monoculture (Figures 3, 4). With 2′-FL, 3-FL, 6′-SL, and LNT2, only a small amount of butyrate was produced in monoculture. This SCFA production was always significantly lower than on glucose (p < 0.0001), but no significant differences between hMOs were observed on both 48- and 72-h cultures (Figures 3C, 4C).
When co-cultures of B. longum subsp. infantis and F. prausnitzii were incubated with the different substrates, high levels of acetate were observed (Figures 3A, 4A). With regard to butyrate production, only a small amount of butyrate was detected with 2′-FL, 3-FL, 6′-SL, or LNT2 after 48 h as well as after 72 h of culture. On glucose, we found similar production rates of acetate when comparing B. longum subsp. infantis monoculture and co-cultures. However, the butyrate production of the co-culture was significantly lower when compared to F. prausnitzii monoculture (p < 0.0001) at 48 and 72 h. For acetate production, in the presence of 2′-FL, 3-FL, or LNT2, no differences were found between B. longum subsp. infantis monocultures and co-cultures. Interestingly, a different result was obtained with 6′-SL. We found that in co-cultures, 5.84 μmol/mg acetate was produced, which was significantly higher than in monoculture of B. longum subsp. infantis (p < 0.0001, Figure 3A), and no acetate was detected in the monoculture of F. prausnitzii after 48-h cultures, and the differences between co-culture and monoculture disappeared after 72-h cultures (Figure 4A). With 2′-FL, 3-FL, 6′-SL, and LNT2, only small amounts of butyrate were detected in co-culture, and no significant differences were detected between the groups at 48- and 72-h cultures.
Co-culture Promotes the Utilization of 6′-SL
As 6′-SL was differently stimulating the production of SCFAs in co-cultures compared to monoculture of B. longum subsp. infantis, we decided to study and compare the degradation profile of 6′-SL in B. longum subsp. infantis monocultures and B. longum subsp. infantis and F. prausnitzii co-cultures. To this end, we took samples at 48 and 72 h from the B. longum subsp. infantis monocultures and B. longum subsp. infantis and F. prausnitzii co-cultures and studied glycosidic degradation in the samples as a measure for carbohydrate utilization.
As shown in Figure 5, we observed that only small amounts of 6′-SL were utilized in the monocultures of B. longum subsp. infantis after 48 h, and the peak of 6′-SL was not decreased in high-performance anion exchange chromatography (HPAEC) chromatogram profiles. In contrast, a clear degradation of 6′-SL in the B. longum subsp. infantis and F. prausnitzii co-culture was observed after 48 h (Figure 5A). The quantification results also showed that only 5.4% of the 6′-SL was utilized in the monocultures of B. longum subsp. infantis after 48 h, while 65.1% of 6′-SL in the B. longum subsp. infantis and F. prausnitzii co-culture was consumed (Figure 5B). After 72 h, 6′-SL 74.0% and 84.7% was used in B. longum subsp. infantis monoculture and in the co-culture system, respectively.
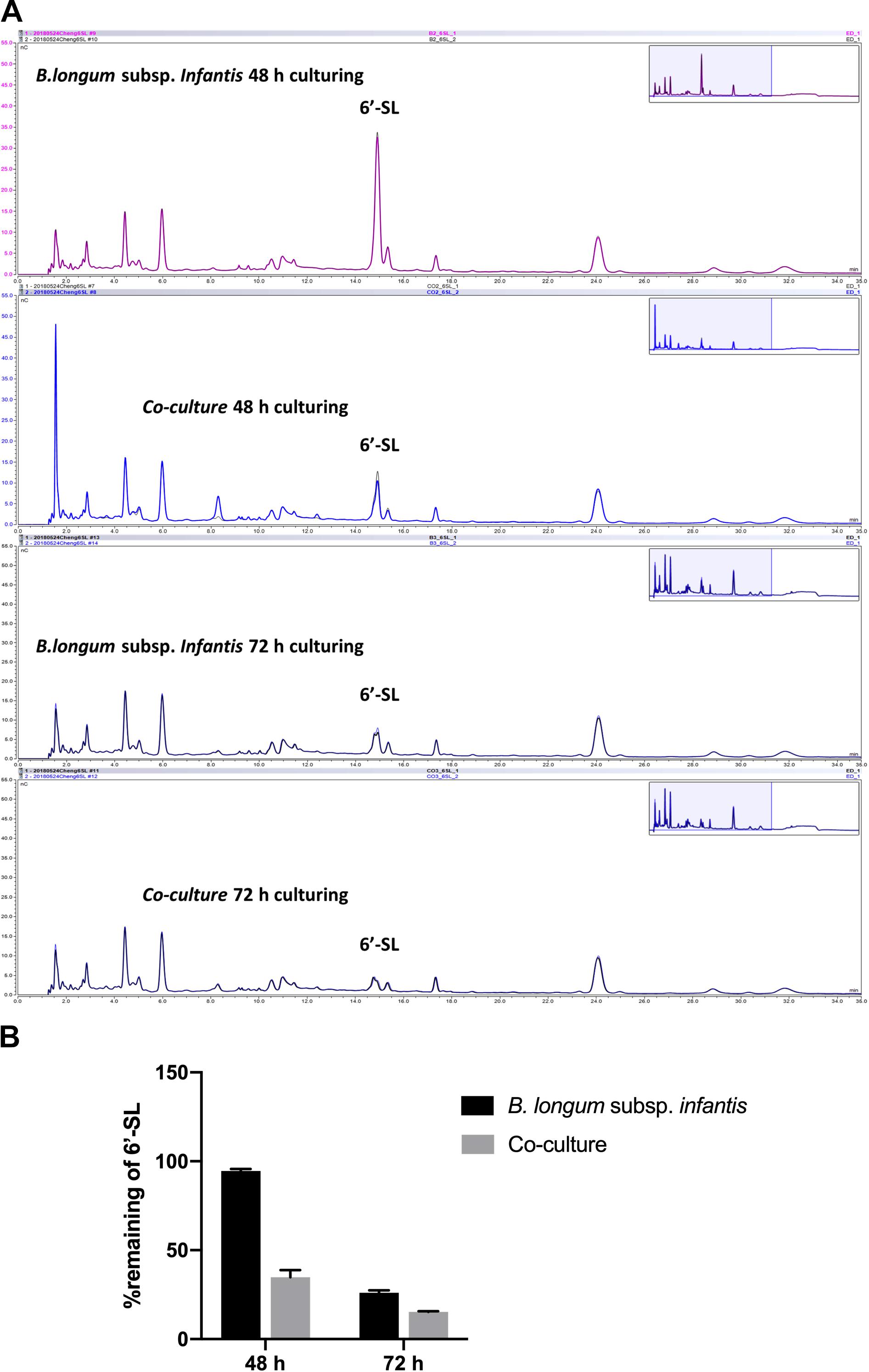
Figure 5. Degradation of 6′-SL after 48-h and 72-h fermentation by B. longum subsp. infantis and F. prausnitzii in mono- and co-cultures. (A) HPAEC chromatogram profiles of 6′-SL when B. longum subsp. infantis in monoculture or co-culture with F. prausnitzii after 48 h and 72 h. (B) The percentage of remaining 6′-SL in the broth when B. longum subsp. infantis was in monoculture or co-culture with F. prausnitzii after 48 h and 72 h.
Discussion
Human milk oligosaccharides are specifically known to support the growth of beneficial microorganisms in the infant gut (Jost et al., 2015). In particular, Bifidobacterium genus (Kirmiz et al., 2018) is acknowledged for that, but which individual hMO and how specific hMOs modulate this process is still unclear. In the present study, our results show that the modulatory effects of individual hMOs on bacterial growth are strongly structure-dependent in both monoculture and co-cultures.
In monoculture, the effects of 2′-FL, 3-FL, 6′-SL, and LNT2 on bacterial growth are bacterial strain- as well as hMO structure-dependent. Different growth patterns were observed for different bacteria strains when exposed to the same hMO. The growth of bacteria on 2′-FL, 3-FL, 6′-SL, and LNT2 was hMO structure-dependent. As shown in Figure 6, hMOs are composed of five monomers: D-glucose (Glc), D-galactose (Gal), N-acetylglucosamine (GlcNAc), L-fucose (Fuc), and sialic acid (Sia) (Figure 6A). All hMOs are synthesized from lactose (Galβ1-4Glc), which can be further extended and form more than 200 different structures of hMOs (Figure 6B; Bode, 2015; Thurl et al., 2017). Several studies have demonstrated that the individual hMOs have different effects and that the final outcome of a specific health benefit depends on the composition of individual hMOs (Cheng et al., 2019, 2020; Kong et al., 2019). In the current study, we found that B. longum subsp. infantis grew faster on 3-FL as carbon source and reached higher cell densities on 2′-FL. 2′-FL and 3-FL are both trisaccharide hMOs that are formed by fucosylation of lactose. The molecules have the same molecular composition and only differ in the attachment position of L-fucose (Fuc) residues on the lactose core region (Figure 6C).
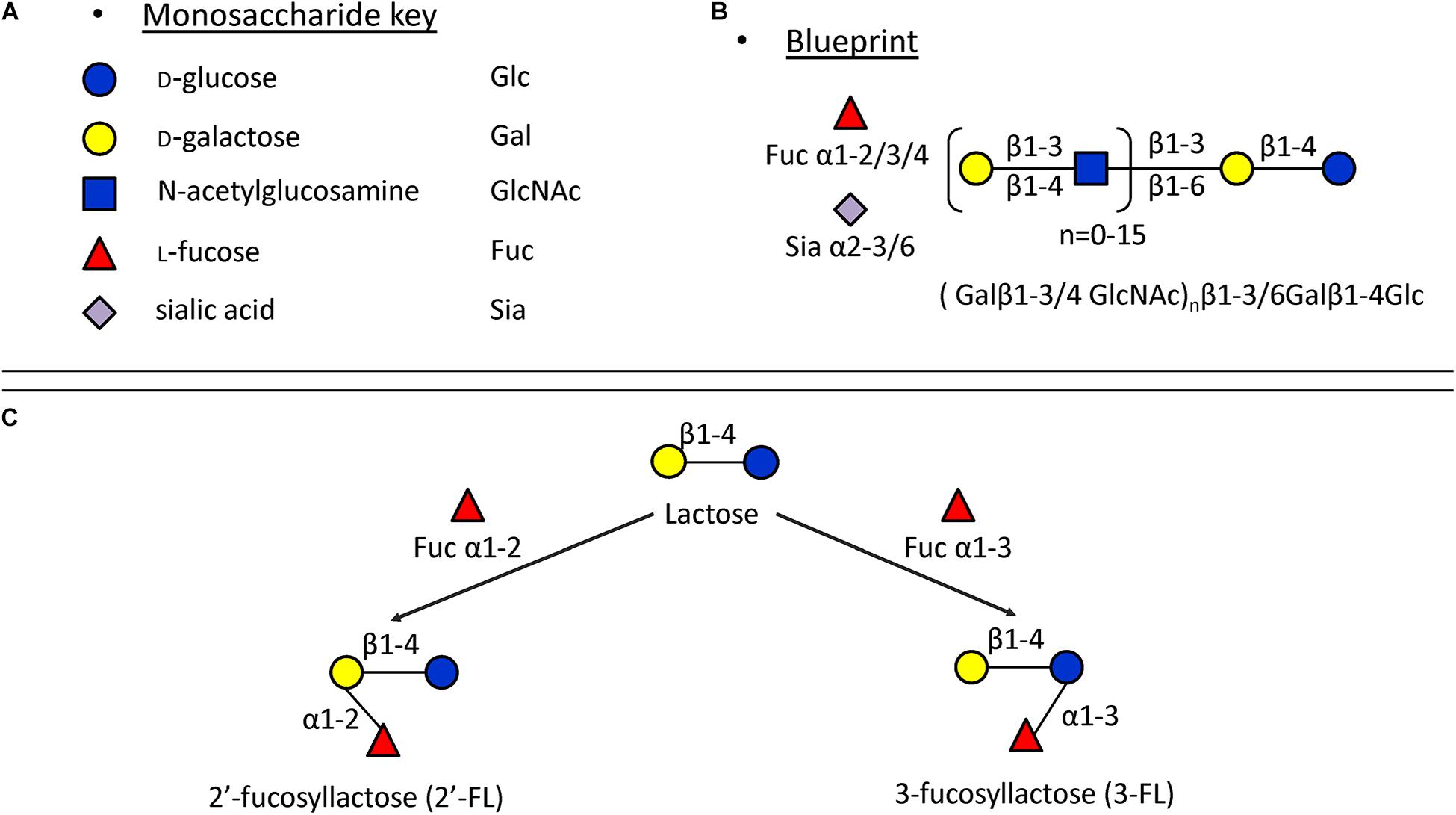
Figure 6. Structures of hMOs. (A) Building blocks of hMOs. (B) Structural blueprint of hMOs. (C) Lactose is fucosylated through different linkages to generate 2′-FL and 3-FL.
The fermentation of both 2′-FL and 3-FL by bacteria occurs via bacterial α-fucosidase and β-galactosidase enzymes (Thomson et al., 2018), and we observed that the growth rate was higher with 3-FL, and the final cell density was higher with 2′-FL. This indicates different kinetics of fermentation of 2′-FL and 3-FL by B. longum subsp. infantis. Different enzymes are needed to ferment 6′-SL and LNT2. For fermenting 6′-SL, the bacteria need β-galactosidase and α-sialidase, and fermentation of LNT2 requires β-galactosidase and β-hexosaminidase (Thomson et al., 2018). The different growth patterns of B. longum subsp. infantis on individual hMOs might be impacted by the catalytic ability of the enzymes. The observation that F. prausnitzii reaches a higher final OD600 on 6′-SL than when grown with 2′-FL, 3-FL, or LNT2 suggests that F. prausnitzii has a higher α-sialidase activity and less α-fucosidase and β-hexosaminidase activity.
Bifidobacterium longum subsp. infantis produce a high concentration of acetic acid, with minor amounts of propionic acid and butyric acid, while F. prausnitzii used acetate in the broth and produced butyric acid (Moens et al., 2016). In the co-culture system, the growth rate was higher than in monoculture of the individual strains. Therefore, we decided to investigate whether the acetate supplied by B. longum subsp. infantis would stimulate the metabolic activity of F. prausnitzii in co-culture. Hence, the SCFA production was quantified and compared between monoculture and co-cultures of B. longum subsp. infantis and F. prausnitzii. Interestingly, under all co-culture conditions tested, no butyrate was detected after 48 h of culture, which suggests that F. prausnitzii, while being a potent butyrate producer, was not able to grow in co-culture. This might be associated with the hMO utilization strategy of B. longum subsp. infantis (Thomson et al., 2018). The utilization of hMOs by B. longum subsp. infantis is based on the uptake of intact hMOs inside the bacteria, where it is intracellularly degraded (Garrido et al., 2011).
Although F. prausnitzii was not able to grow in the co-cultures, we still observed that with 6′-SL as carbon source, B. longum subsp. infantis and F. prausnitzii co-cultures had higher growth rates than in the monocultures (Figure 2C). This indicated that the presence of F. prausnitzii may promote the growth of B. longum subsp. infantis on 6′-SL in co-culture. Since only a small amount of butyrate was produced in F. prausnitzii monoculture as well as co-culture (Figures 3, 4), and since there were no statistically significant difference between F. prausnitzii monoculture and co-culture, we concluded that 6′-SL was not substantially fermented by F. prausnitzii. Therefore the degradation of 6′-SL in F. prausnitzii monoculture was not included. This is in accordance with the SCFA production and carbohydrate degradation results, which showed that B. longum subsp. infantis and F. prausnitzii co-culture promotes acetate production and 6′-SL utilization. However, this promoting effect of F. prausnitzii was only observed on 6′-SL, and not on 2′-FL, 3-FL, and LNT2. As only the utilization of 6′-SL involves a sialidase (Thomson et al., 2018), we hypothesized that the co-culture of B. longum subsp. infantis and F. prausnitzii might enhance sialidase expression, as only 6′-SL promoted the growth and metabolism of B. longum subsp. infantis. However, in the current study, only one sialylated hMO was included. Hence, in order to confirm this hypothesis, more sialylated hMOs, such as 3′-sialyllactose (3′-SL), are needed. Unfortunately, due to the technical limitations, pure 3′-SL is not available yet, but would be of great value to test our hypothesis.
In conclusion, we demonstrate that the utilization of individual hMOs as sole carbohydrate sources is bacterial strain- and hMO structure-dependent. Our results show that hMOs currently applied or developed to be applied in infant formula are able to modulate the growth of B. longum subsp. infantis in a structure-dependent way and stimulate further growth of B. longum subsp. infantis during co-cultures. In particular, 6′-SL, which can promote the growth of B. longum subsp. infantis in B. longum subsp. infantis and F. prausnitzii co-culture, showed promising results. Again, we demonstrate that the effects of individual hMOs are highly structure-dependent (Cheng et al., 2019; Kong et al., 2019). Small differences in the molecular structure of hMOs can have significant impact on their biological efficacy. Follow-up studies are needed to identify the specific structure responsible for modulation of bacterial growth, e.g., impact of other sialylated hMOs, which might provide new effective and targeted ways of supporting the growth of beneficial microorganisms in the infant intestine.
Materials and Methods
Components
In the present study, 2′-FL (provided by FrieslandCampina Domo, Amersfoort, Netherlands), 3-FL, 6′-SL, and LNT2 (provided by Glycosyn LLC, Woburn, MA, United States) were tested. An overview of the structures of these components are shown in Table 1.
Bacterial Strains
Faecalibacterium prausnitzii A2-165 (DSM 17677) was obtained from the Deutsche Sammlung von Mikroorganismen and Zellkulturen (DSMZ, Göttingen, Germany). B. longum subsp. longum, B. longum subsp. infantis ATCC 15697, and B. adolescentis (collection strain, NIZO B659) were kindly provided by HH, Department of Medical Microbiology, University Medical Center Groningen (UMCG, Groningen, Netherlands).
Growth Broth, Monoculture, and Co-culture Conditions
All strains were grown and maintained in yeast extract, casitone, and fatty acid broth (YCFA) (Lopez-Siles et al., 2012). First, the growth and substrate fermentation capacities of the individual strains on different hMO sources were studied. In order to do this, various hMOs were added to the broth at a concentration of 5 mg/ml. YCFA containing 5 mg/ml glucose (Thermo Scientific, Breda, Netherlands) was used as a positive control, while media without carbohydrate served as a negative control. Broth was autoclaved at 121°C for 20 min first, after which the carbohydrate source was added to the cooled broth after sterile filtration. The final pH of the broth was adjusted to 6.5 ± 0.1 by using sterile HCl.
All strains were inoculated in 5 ml of YCFA broth supplemented with glucose as the sole energy source (YCFAG) and incubated anaerobically at 37°C for 24 h. Subsequently, the strains were propagated to 5 ml of YCFAG broth culture overnight as pre-culture; after that, different strains were added to the tubes of YCFA broth supplemented with the different carbon sources at a ratio of 1:100 in duplicate at 37°C. Growth in cultures was monitored spectrophotometrically every 4 h from 0- to 72-h culture by measuring the OD600 by using Ultrospec 10 cell density meter (Amersham Biosciences GmbH, Germany).
To study the interaction between B. longum subsp. infantis and F. prausnitzii, co-culture fermentations were performed in YCFA with 5 mg/ml of the different carbon sources. B. longum subsp. infantis and F. prausnitzii cells were inoculated as described above. Subsequently, the strains were propagated to 5 ml of YCFAG media and were pre-cultured separately, and the cell density was monitored spectrophotometrically by measuring the OD600 using Ultrospec 10 cell density meter. When OD600 reached 1.0 in the pre-culture, B. longum subsp. infantis and F. prausnitzii were added to the same tube of YCFA broth supplemented with the different carbon sources at a ratio of 1:100. Each batch of experiments was made with the same inocula for both B. longum subsp. infantis and F. prausnitzii.
SCFA Production
Samples for the SCFA analyses were taken at 48 h and 72 h of monoculture and co-culture incubation. The fermentation digest (0.5 ml) was heated at 100°C for 5 min and then centrifuged at 13,200 × g for 10 min at room temperature. Analysis of SCFAs (acetate, propionate, and butyrate) by gas chromatography (GC) was done as described previously by Gu et al. (2018). A 250-μl aliquot of the fivefold diluted supernatant of the fermentation product was mixed with 125 μl of a solution containing oxalic acid (0.09 M), HCl (0.3 M), and internal standard 2-ethyl butyric acid (0.45 mg/ml). Afterward, the mixture was allowed to stand at room temperature for 30 min. The temperature profile during GC analysis was as follows: 100°C, maintained for 0.5 min; raised to 180°C at 8°C/min, maintained for 1 min; raised to 200°C at 20°C/min, maintained for 5 min. Xcalibur software (Thermo Scientific, Breda, Netherlands) was used to process data from GC.
Glycosidic Degradation
Samples for the degradation analysis were taken at 48 and 72 h of B. longum subsp. infantis monoculture and co-culture. HPAEC was used to measure the carbohydrate degradation as a measure for utilization of carbohydrates by the microorganisms (Cardarelli et al., 2016). An ISC 3000 (Dionex, Sunnyvale, CA, United States), equipped with a 2 × 250 mm Dionex CarboPac PA-1 column and a 2 × 50 mm CarboPac PA-1 guard column, was used for quantification (Albrecht et al., 2009). Briefly, samples were adjusted to a final concentration of 0.05 mg of the substrate per milliliter, and 10 μl of the samples was injected using a Dionex ISC3000 autosampler. The oligosaccharides were eluted (0.3 ml/min) by a gradient of 0–350 mM sodium acetate in 100 mM NaOH for 35 min. Each elution was followed by a washing step with 1 M NaOAc in 100 mM NaOH for 5 min and an equilibration step with 100 mM NaOH for 15 min. A Dionex ED40 detector in pulsed amperometric detection mode was used for detection. 6′-SL at final concentrations of 0.05 mg/ml were used as standards. Chromeleon software Version 6.70 (Dionex) was used for the integration and evaluation of the chromatograms obtained.
Statistics
The results were analyzed using GraphPad Prism. The normality of distribution of the data was tested by using the Kolmogorov–Smirnov test. Values are expressed as median ± range. Statistical comparisons were performed using the Kruskal–Wallis test followed by the Dunn’s test. p < 0.05 was considered as statistically significant (∗p < 0.05, ∗∗p < 0.01, ∗∗∗p < 0.001, ****p < 0.0001).
Data Availability Statement
The raw data supporting the conclusions of this article will be made available by the authors, without undue reservation.
Author Contributions
LC and PV conceived and designed the experiments. LC and ML performed the experiments and analyzed the data. AG supplied hMOs. LC, MK, ML, AG, AN, and PV participated in the discussion. LC, MK, ML, AG, AN, HS, MW, HH, and PV wrote the manuscript. All authors contributed to the article and approved the submitted version.
Conflict of Interest
AG and AN are employed by the company Friesl and Campina.
The remaining authors declare that the research was conducted in the absence of any commercial or financial relationships that could be construed as a potential conflict of interest.
Funding
This project was financially supported by the China Scholarship Council (CSC). This research was performed within the framework of the Sino-Dutch Doctoral Program on Sustainable Dairy coordinated by the Carbohydrate Competence Center (CCC, www.cccresearch.nl).
Supplementary Material
The Supplementary Material for this article can be found online at: https://www.frontiersin.org/articles/10.3389/fmicb.2020.569700/full#supplementary-material
Supplementary Figure S1 | The endpoint OD600 of B. longum subsp. infantis, B. longum subsp. longum, B. adolescentis and F. prausnitzii in mono-culture. Glucose, 2′-FL, 3-FL, 6′-SL, and LNT2 were included as carbon source. The assays were carried out 3 times in duplicate. Values are expressed as median ± range. Statistical significance was measured using Kruskal-Wallis test followed by the Dunn’s test and indicated by * (p < 0.05), ** (p < 0.01), *** (p < 0.001) or by **** (p < 0.0001).
References
Albrecht, S., van Muiswinkel, G. C. J., Schols, H. A., Voragen, A. G. J., and Gruppen, H. (2009). Introducing Capillary Electrophoresis with Laser-Induced Fluorescence Detection (CE-LIF) for the Characterization of Konjac Glucomannan Oligosaccharides and Their in Vitro Fermentation Behavior. J. Agric. Food Chem. 57, 3867–3876. doi: 10.1021/jf8038956
Aly, E., Ali Darwish, A., Lopez-Nicolas, R., Frontela-Saseta, C., and Ros-Berruezo, G. (2018). “Bioactive components of human milk: similarities and differences between human milk and infant formula,” in Selected Topics in Breastfeeding, ed. R. Mauricio Barría (London: IntechOpen). doi: 10.5772/intechopen.73074
Bode, L. (2015). The functional biology of human milk oligosaccharides. Early Hum. Dev. 91, 619–622. doi: 10.1016/j.earlhumdev.2015.09.001
Cardarelli, H. R., Martinez, R. C. R., Albrecht, S., Schols, H., Franco, B. D. G. M., Saad, S. M. I., et al. (2016). In vitro fermentation of prebiotic carbohydrates by intestinal microbiota in the presence of Lactobacillus amylovorus DSM 16998. Benef. Microbes 7, 119–133. doi: 10.3920/BM2014.0151
Cheng, L., Kiewiet, M. B. G., Groeneveld, A., Nauta, A., and de Vos, P. (2019). Human milk oligosaccharides and its acid hydrolysate LNT2 show immunomodulatory effects via TLRs in a dose and structure-dependent way. J. Funct. Foods 59, 174–184. doi: 10.1016/j.jff.2019.05.023
Cheng, L., Kong, C., Walvoort, M. T. C., Faas, M. M., and de Vos, P. (2020). Human milk oligosaccharides differently modulate goblet cells under homeostatic, proinflammatory conditions and ER stress. Mol. Nutr. Food Res. 64:1900976. doi: 10.1002/mnfr.201900976
Conlon, M. A., and Bird, A. R. (2015). The impact of diet and lifestyle on gut microbiota and human health. Nutrients 7, 17–44. doi: 10.3390/nu7010017
Coulet, M., Phothirath, P., Allais, L., and Schilter, B. (2014). Pre-clinical safety evaluation of the synthetic human milk, nature-identical, oligosaccharide 2’-O-Fucosyllactose (2’FL). Regul. Toxicol. Pharmacol. 68, 59–69. doi: 10.1016/j.yrtph.2013.11.005
Garrido, D., Kim, J. H., German, J. B., Raybould, H. E., and Mills, D. A. (2011). Oligosaccharide binding proteins from Bifidobacterium longum subsp. infantis reveal a preference for host glycans. PLoS One 6:e17315. doi: 10.1371/journal.pone.0017315
Goldsmith, F., O’Sullivan, A., Smilowitz, J. T., and Freeman, S. L. (2015). Lactation and intestinal microbiota: how early diet shapes the infant gut. J. Mammary Gland Biol. Neoplasia 20, 149–158. doi: 10.1007/s10911-015-9335-2
Gu, F., Borewicz, K., Richter, B., van der Zaal, P. H., Smidt, H., Buwalda, P. L., et al. (2018). In vitro fermentation behavior of isomalto/malto-polysaccharides using human fecal inoculum indicates prebiotic potential. Mol. Nutr. Food Res. 62:1800232. doi: 10.1002/mnfr.201800232
Heymann, J., Raub, A., and Earle, A. (2013). Breastfeeding policy: a globally comparative analysis. Bull. World Health Organ. 91, 398–406. doi: 10.2471/BLT.12.109363
Jost, T., Lacroix, C., Braegger, C., and Chassard, C. (2015). Impact of human milk bacteria and oligosaccharides on neonatal gut microbiota establishment and gut health. Nutr. Rev. 73, 426–437. doi: 10.1093/nutrit/nuu016
Kirmiz, N., Robinson, R. C., Shah, I. M., Barile, D., and Mills, D. A. (2018). Milk glycans and their interaction with the infant-gut microbiota. Annu. Rev. Food Sci. Technol. 9, 429–450. doi: 10.1146/annurev-food-030216-030207
Kong, C., Elderman, M., Cheng, L., de Haan, B. J., Nauta, A., and de Vos, P. (2019). Modulation of intestinal epithelial glycocalyx development by human milk oligosaccharides and non-digestible carbohydrates. Mol. Nutr. Food Res. 63:1900303. doi: 10.1002/mnfr.201900303
Laursen, M. F., Laursen, R. P., Larnkjær, A., Mølgaard, C., Michaelsen, K. F., Frøkiær, H., et al. (2017). Faecalibacterium Gut Colonization Is Accelerated by Presence of Older Siblings. mSphere 2:e00448-17. doi: 10.1128/msphere.00448-17
Le Doare, K., Holder, B., Bassett, A., and Pannaraj, P. S. (2018). Mother’s Milk: a purposeful contribution to the development of the infant microbiota and immunity. Front. Immunol. 9:361. doi: 10.3389/fimmu.2018.00361
Lopez-Siles, M., Khan, T. M., Duncan, S. H., Harmsen, H. J. M., Garcia-Gil, L. J., and Flint, H. J. (2012). Cultured representatives of two major phylogroups of human colonic Faecalibacterium prausnitzii can utilize pectin, uronic acids, and host-derived substrates for growth. Appl. Environ. Microbiol. 78, 420–428. doi: 10.1128/AEM.06858-11
McLeod, K. H., Richards, J. L., Yap, Y. A., and Mariño, E. (2019). “Dietary short chain fatty acids: how the gut microbiota fight against autoimmune and inflammatory diseases,” in Bioactive Food as Dietary Interventions for Arthritis and Related Inflammatory Diseases, eds R. Watson and V. Preedy (Cambridge, MA: Academic Press), 139–159. doi: 10.1016/b978-0-12-813820-5.00007-6
Moens, F., Weckx, S., and De Vuyst, L. (2016). Bifidobacterial inulin-type fructan degradation capacity determines cross-feeding interactions between bifidobacteria and Faecalibacterium prausnitzii. Int. J. Food Microbiol. 231, 76–85. doi: 10.1016/j.ijfoodmicro.2016.05.015
Moore, R. E., and Townsend, S. D. (2019). Temporal development of the infant gut microbiome. Open Biol. 9:190128. doi: 10.1098/rsob.190128
Sokol, H., Pigneur, B., Watterlot, L., Lakhdari, O., Bermudez-Humaran, L. G., Gratadoux, J.-J., et al. (2008). Faecalibacterium prausnitzii is an anti-inflammatory commensal bacterium identified by gut microbiota analysis of Crohn disease patients. Proc. Natl. Acad. Sci. U.S.A. 105, 16731–16736. doi: 10.1073/pnas.0804812105
Thomson, P., Medina, D. A., and Garrido, D. (2018). Human milk oligosaccharides and infant gut bifidobacteria: molecular strategies for their utilization. Food Microbiol. 75, 37–46. doi: 10.1016/j.fm.2017.09.001
Thurl, S., Munzert, M., Boehm, G., Matthews, C., and Stahl, B. (2017). Systematic review of the concentrations of oligosaccharides in human milk. Nutr. Rev. 75, 920–933. doi: 10.1093/nutrit/nux044
Triantis, V., Bode, L., and van Neerven, J. R. J. (2018). Immunological effects of human milk oligosaccharides. Front. Pediatr. 6:190. doi: 10.3389/fped.2018.00190
Underwood, M. A., German, J. B., Lebrilla, C. B., and Mills, D. A. (2015). Bifidobacterium longum subspecies infantis: champion colonizer of the infant gut. Pediatr. Res. 77, 229–235. doi: 10.1038/pr.2014.156
Vandenplas, Y., Berger, B., Carnielli, V. P., Ksiazyk, J., Lagström, H., Luna, M. S., et al. (2018). Human milk oligosaccharides: 2’-fucosyllactose (2’-FL) and lacto-n-neotetraose (LNnT) in infant formula. Nutrients 10:1161. doi: 10.3390/nu10091161
Walker, A. (2010). Breast milk as the gold standard for protective nutrients. J. Pediatr. 156, S3–S7. doi: 10.1016/j.jpeds.2009.11.021
Ward, R. E., Niñonuevo, M., Mills, D. A., Lebrilla, C. B., and German, J. B. (2007). In vitro fermentability of human milk oligosaccharides by several strains of bifidobacteria. Mol. Nutr. Food Res. 51, 1398–1405. doi: 10.1002/mnfr.200700150
Keywords: human milk oligosaccharides, co-culture, Bifidobacterium longum subsp. infantis, Faecalibacterium prausnitzii, hMO structure-specific
Citation: Cheng L, Kiewiet MBG, Logtenberg MJ, Groeneveld A, Nauta A, Schols HA, Walvoort MTC, Harmsen HJM and de Vos P (2020) Effects of Different Human Milk Oligosaccharides on Growth of Bifidobacteria in Monoculture and Co-culture With Faecalibacterium prausnitzii. Front. Microbiol. 11:569700. doi: 10.3389/fmicb.2020.569700
Received: 04 June 2020; Accepted: 02 October 2020;
Published: 30 October 2020.
Edited by:
Katia Sivieri, São Paulo State University, BrazilReviewed by:
Catherine Mullié, University of Picardy Jules Verne, FranceMarciane Magnani, Federal University of Paraíba, Brazil
Copyright © 2020 Cheng, Kiewiet, Logtenberg, Groeneveld, Nauta, Schols, Walvoort, Harmsen and de Vos. This is an open-access article distributed under the terms of the Creative Commons Attribution License (CC BY). The use, distribution or reproduction in other forums is permitted, provided the original author(s) and the copyright owner(s) are credited and that the original publication in this journal is cited, in accordance with accepted academic practice. No use, distribution or reproduction is permitted which does not comply with these terms.
*Correspondence: Lianghui Cheng, bGlhbmdodWljaGVuZ0Bob3RtYWlsLmNvbQ==