- 1Department of Botany, Deen Dayal Upadhyay Gorakhpur University, Gorakhpur, India
- 2Department of Microbiology, School for Environmental Sciences, Babasaheb Bhimrao Ambedkar University, Lucknow, India
- 3DST-Center for Policy Research, Babasaheb Bhimrao Ambedkar University, Lucknow, India
- 4Department of Environmental Science, School for Environmental Sciences, Babasaheb Bhimrao Ambedkar University, Lucknow, India
Soil salinization has emerged as one of the prime environmental constraints endangering soil quality and agricultural productivity. Anthropogenic activities coupled with rapid pace of climate change are the key drivers of soil salinity resulting in degradation of agricultural lands. Increasing levels of salt not only impair structure of soil and its microbial activity but also restrict plant growth by causing harmful imbalance and metabolic disorders. Potential of secondary metabolites synthesized by halotolerant plant growth promoting rhizobacteria (HT-PGPR) in the management of salinity stress in crops is gaining importance. A wide array of secondary metabolites such as osmoprotectants/compatible solutes, exopolysaccharides (EPS) and volatile organic compounds (VOCs) from HT-PGPR have been reported to play crucial roles in ameliorating salinity stress in plants and their symbiotic partners. In addition, HT-PGPR and their metabolites also help in prompt buffering of the salt stress and act as biological engineers enhancing the quality and productivity of saline soils. The review documents prominent secondary metabolites from HT-PGPR and their role in modulating responses of plants to salinity stress. The review also highlights the mechanisms involved in the production of secondary metabolites by HT-PGPR in saline conditions. Utilizing the HT-PGPR and their secondary metabolites for the development of novel bioinoculants for the management of saline agro-ecosystems can be an important strategy in the future.
Introduction
The anthropocene era is facing rigorous environmental stress due to aggravated human activities combined with rapid pace of climate change that is negatively affecting agricultural production and its sustainability. Soil salinity is one of the major global issues which is undermining crop yield and jeopardizing productive capacity of soils (Arora et al., 2018). The declining soil fertility and inadequate crop productivity has raised serious concerns for food security of ever rising human population. Reports claim that soil salinity has affected several countries of the world up till now and is growing at a rate of 1–2% each year (Kasim et al., 2016; Shahid et al., 2018). It has been estimated that over one-third of irrigated land could become barren due to increasing levels of soil salinization (Dodd and Perez-Alfocea, 2012). According to the data released by Food and Agriculture Organization (FAO) salinity together with sodicity has affected around 831 million hectares (397 million-hectare saline soils and 434 million hectare sodic soils) of global lands1. Soil salinity is mainly prevalent in arid and semi-arid areas of the world where precipitation is insufficient and evapotranspiration rate is higher, leading to water stress conditions and mineral leaching from the plant root zone (Zörb et al., 2019). Excessive use of chemicals, such as fertilizers and pesticides, and climate change are also responsible for increasing soil salinity around the globe. Salt affected soils are characterized by the high electrical conductivity of soil saturation extract (ECe) i.e., more than 4 dS/m and contain excess amounts of salts of bicarbonates, chlorides, and sulfates of Na+ (sodium ion), Ca2+ (calcium ion), and Mg2+ (magnesium ion) which impairs plant health (Rengasamy, 2006; Numan et al., 2018). Such soils are poor in nutrient content, microbial activity, biomass, and organic matter and thus are rendered as infertile (Diacono and Montemurro, 2015). Salinization also interferes with nutrient assimilation, creates ionic disequilibrium due to accumulation of Na+ and Cl– in their cells, generates reactive oxygen species (ROS), perturbs carbon and nitrogen metabolism, and reduces rate of photosynthesis in stressed plants (Kang et al., 2019). Soil salinization significantly impacts the economy and as per a study by Qadir et al. (2014), salt-induced land degradation of irrigated areas may cost up to US$ 27.3 billion in a year just because of the loss in crop production. Reclamation of such salt degraded lands is of utmost importance in order to meet the future food demands of predicted human population of 10 billion in 2050 (Egamberdieva et al., 2019).
Physical and chemical remediation of saline soils i.e., soil leaching, flushing, gypsum, and lime treatment are time-consuming and cause loss of biodiversity of indigenous species of plants and microbes (Egamberdieva et al., 2019). To circumvent these constraints, we need to find sustainable methods which are cheaper and eco-friendly in origin, to restore saline degraded lands. Recent studies have shown the potential of halotolerant plant growth promoting rhizobacteria (HT-PGPR) and their secondary metabolites in amelioration of salinity stress in affected plants/crops. HT-PGPR are capable of producing a diverse array of secondary metabolites for protection (of both the host bacterial cells and symbiotic plant) and plant growth promotion even under salinity stress (Abbas et al., 2019). Apart from producing phytohormones, siderophores, solubilization of nutrients such as phosphorous (P), zinc (Zn), potassium (K), and 1-aminocyclopropane-1-carboxylic acid (ACC) deaminase to lower stress ethylene level, HT-PGPR have been reported to produce certain metabolites playing a direct role in salt tolerance and these include osmoprotectants (Upadhyay and Singh, 2015), exopolysaccharides (EPS) (Ashraf et al., 2004) and volatile organic compounds (VOCs) (Vaishnav et al., 2015). Many of these metabolites are exclusively produced during abiotic stress conditions and help in the plant’s survival under adverse environmental conditions (Figure 1). These compounds also assure the ecological fitness of plants by maintaining ionic equilibrium through Na+/K+ transporter, improving water potential, and expressing salt overly sensitive (SOS) genes involved in stress tolerance under saline conditions (Bharti et al., 2016; Liu et al., 2017a). The review highlights the potent roles of HT-PGPR and their secondary metabolites which hold a promising future for use as next-generation bioinoculants for salt-affected agro-ecosystems.
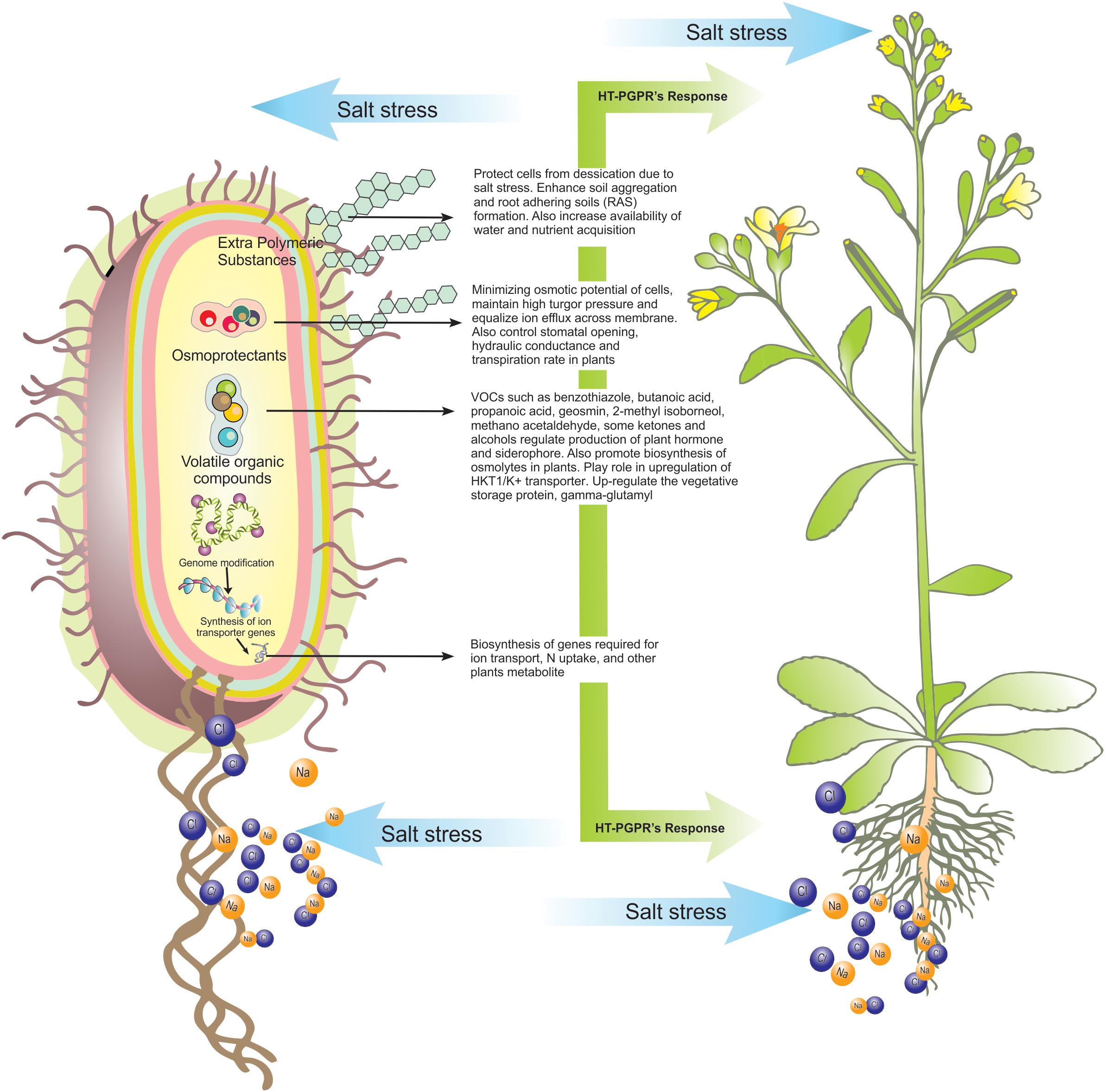
Figure 1. An overview of possible roles of secondary metabolites produced by HT-PGPR in plant’s health improvement under salt-stress.
HT-PGPR: Diversity and Their Impact on Crop Improvement
Halotolerant plant growth promoting rhizobacteria are now well known for their capability to tolerate and mitigate salinity stress in plants. Several species of HT-PGPR such as Arhrobacter, Azospirillum, Alcaligenes, Bacillus, Burkholderia, Enterobacter, Microbacterium, Klebsiella, Pseudomonas, Streptomyces, Rhizobium and Pantoea have been reported to alleviate salt stress in crops (Abbas et al., 2019; Arora et al., 2020). In a study by Nadeem et al. (2013), inoculation of wheat by HT-PGPR Pseudomonas putida, Enterobacter cloacae, Serratia ficaria, and Pseudomonas fluorescens sown in naturally saline fields (ECe = 15 dS m–1) significantly enhanced germination percentage, germination rate and index of wheat seeds by 43, 51, and 123%, respectively, as compared to untreated control. These strains also significantly enhanced yield of wheat in saline field. In another study, inoculation of salt tolerant Pseudomonas argentinensis HMM57 and Pseudomonas azotoformans JMM15 in Brassica juncea grown under salinity stress (ECe = 12 dS m–1) resulted in marked increase in root, shoot and plant dry weight (Phour and Sindhu, 2020). Aslam and Ali (2018) showed that a halotolerant Staphylococcus jettensis F-11 showed threefold increase in dry weight mass of Zea mays under 200 mM salinity stress. Ramadoss et al. (2013) proved that wheat inoculated with Hallobacillus sp. SL3 and Bacillus halodenitrificans PU62 resulted in increased root length by 90% and dry weight by 17.4% under 320 mM NaCl stress. Several examples of HT-PGPR are now known and they are a very diverse group with some important examples quoted in Table 1. Although it is understood that HT-PGPR can help in alleviating salinity stress and enhance crop productivity (in salt affected soil), there still is a lot to explore regarding the interactions and mechanisms occurring between these microbes and plants during a multi-dimensional stress such as salinity. Roles of secondary metabolites produced by HT-PGPR are crucial and have been explained in the subsequent sections.
Major Secondary Metabolites of HT-PGPR and Their Role in Salt-Stress Alleviation in Plants
There are several mechanisms by which halotolerant rhizobacteria provide resilience to plants under saline conditions. However, production of secondary metabolites such as EPS, VOCs and compatible solutes (proline, trehalose, and glycine betaines, etc.) by HT-PGPR have been found to directly modulate plant’s cellular responses through regulation of SOS1 gene (Bharti et al., 2016); expression of stress regulating genes (Morris and González, 2009); expression of high-affinity K+-transporter (HKT1) genes (Kasotia et al., 2016); genes for antioxidant protein and ethylene biosynthesis (Kwon et al., 2010) involved in the alleviation of salt-stress. Major secondary metabolites produced by HT-PGPR and their role in mitigation of salt stress in plants are mentioned in Table 1. In the following section, role of key metabolites, produced or supported by HT-PGPR, in salt-tolerance and its mitigation have been discussed.
Osmoprotectants/Compatible Solutes
Water homeostasis is vital for the proper functioning of physiological and metabolic processes in plants. Accumulation of excess salt in soil perturbs water uptake by plant cells and creates osmotic stress and ionic toxicity (accumulation of Na+ and Cl–) that in turn inhibits growth and developmental process (Carmen and Roberto, 2011). The hypertonic conditions and abundance of Na+ and Cl– ions disturb essential physiological activities that include root and stem growth, maturation of cell structure, transpiration and photosynthesis, enzyme activities, nutrient uptake, hormonal status and many more (Munns, 2002; Pérez-López et al., 2012). To acclimatize under saline conditions, HT-PGPR produce low molecular weight metabolites, known as osmoprotectant compounds or compatible solutes that help in minimizing osmotic stress, maintain high turgor pressure, and equalize ion efflux across the plasma membrane in their mutualistic partner plants (Dodd and Perez-Alfocea, 2012). These compounds also control stomatal opening, hydraulic conductance, and transpiration rate to alleviate the water deficiency in plants (Paul and Lade, 2014; Saghafi et al., 2019). Amino acids and their derivatives (e.g., proline, glutamate, glycine betaine and ectoine), polyols (e.g., glycerol, inositol, sorbitol and mannitol), and non-reducing sugars (e.g., trehaloses) are some of the major compatible solutes produced by HT-PGPR under saline conditions (Sleator and Hill, 2002). Bacteria acquire these compounds either from the surrounding environment or synthesize them de novo in response to salt stress and help in amelioration of this abiotic stress in plants through mechanisms as shown in Figure 2. It is a well-known fact that under osmotic stress synthesis of proline gets accelerated in plant cells as compared to normal conditions (Kaur and Asthir, 2015). It is also well documented that HT-PGPR can enhance proline production in plants under salinity stress (Abbas et al., 2019). In response to different environmental stresses such as drought, high salinity, and heavy metals, proline can act as reactive oxygen species (ROS) scavenger, regulate cytosolic acidity, or stabilize the structure of proteins (Krasensky and Jonak, 2012). Biosynthesis of proline involves combined action of enzymes γ-glutamyl kinase, γ-glutamyl phosphate reductase, and δ1-pyrroline-5-carboxylate reductase for the majority of bacteria. This catalytic reaction is stimulated by genes proB, proA, and proC (Kunst et al., 1997; Roy and Hill, 2002). Nitrogen-fixing bacteria have been reported to show elevated proline metabolism due to increased activity of proline dehydrogenase (PDH) enzyme during salinity stress (Kohl et al., 1994; Meena et al., 2019). A HT-PGPR, Bacillus fortis SSB21, was reported to increase the levels of proline along with a reduction in lipid peroxidation and ROS, alongwith upregulation of stress regulating genes CAPIP2, CaKR1, CaOSM1, and CAChi2 in capsicum under saline conditions (1 and 2 g NaCl kg–1 soil) (Yasin et al., 2018). Bharti et al. (2014) reported that Mentha arvensis inoculated with HT-PGPR belonging to genus Bacillus, Halomonas, and Exiguobacterium showed a higher foliar proline content as compared to control plants. Pan et al. (2019) illustrated that inoculation of HT-PGPR plays an important role in maintaining ion homeostasis (K+/Na+) in salt-sensitive plants and helps in accumulation of osmolytes including soluble sugars and proteins as well as increased bioavailability of nutrients like N, P, K, Ca, and Mg, imparting tolerance against adverse effects of salinity. Inoculation of salt tolerant Paenibacillus yonginensis DCY84T– on ginseng seeds resulted in the accumulation of total soluble sugars, proline, and polyamine content under 300 mM salt stress. The treatment also enhanced nutrient availability, chlorophyll content, abscisic acid (ABA) biosynthesis, and induction of stress-responsive genes in plants under salt stress (Sukweenadhi et al., 2018). Glycine betaine (GB) is another class of compatible solute known to reciprocate salinity induced stresses in plants by accumulating in the cytosol, which in turn reduces the osmotic stress thereby maintaining the overall integrity of the plant cell (Hoque et al., 2007; Wutipraditkul et al., 2015). These are nitrogenous compounds that exist in the zwitterionic state and are known to naturally accumulate during environmental stress conditions (Giri, 2011). Malekzadeh (2015) showed that GB when applied exogenously improved salt-tolerance in soybean which was evident by decreased Na+ concentration and increased superoxide dismutase (SOD) and catalase (CAT) activity in treated plants. HT-PGPR are capable of inducing the synthesis and accumulation of GB in salt stressed plants and hence it is better to utilize them for this purpose. Studies reveal that GB synthesis in Bacillus subtilis is triggered by the action of two enzymes namely (i) type III alcohol dehydrogenase which oxidizes choline, a precursor molecule to the intermediate compound glycine betaine aldehyde and (ii) a glycine betaine aldehyde dehydrogenase which forms the final product (GB) (Kappes et al., 1999; Roy and Hill, 2002). A GB biosynthetic gene, codA (for enzyme choline oxidase) from Arthrobacter globiformis, which converts choline into GB, has been widely used for GB production in transgenic plants (Giri, 2011). Inoculation of HT-PGPR Bacillus HL3RS14 increased levels of GB in maize and also promoted growth of plants under salinity stress (Mukhtar et al., 2019). In the same way, B. subtilis BERA71 inoculated plants showed higher content of GB and other osmolytes in Acacia gerrardii under saline conditions (Hashem et al., 2016). Ectoine (1,4,5,6-tetrahydro-2-methyl-4-pyrimidinecarboxylic acid) is another osmolyte that gets accumulated in plant cytoplasm on the exposure of salinity stress (Masouleh, 2019). Synthesis of ectoine in bacteria involves role of three enzymes i.e., L-2,4-diaminobutyric acid aminotransferase, L-2,4-diaminobutyric acid acetyl transferase and L-ectoine synthase (triggered by genes ectB, ectA, and ectC respectively) to compensate nitrate impairment in plant roots (Moghaieb et al., 2006, 2011). In HT-PGPR, the amount of ectoine formation is directly proportional to the increase in osmotic pressure on the cell due to different reasons including salinity stress (Grammann et al., 2002). In a study, ectoine extracted from a halophilic Chromohalobacter salexigens KT989776 was reported to enhance seed germination of flax as well as reduced sodium accumulation, peroxidase and phenoloxidase activity in plants (Elsakhawy et al., 2019). Another osmoprotectant utilized by HT-PGPR are trehaloses, which are non-reducing disaccharides in which two glucose moieties are linked by α,α-(1,1)-glycosidic bonds (Chandra et al., 2011). Plants lack the machinery to produce trehalose, but HT-PGPR play important role by producing this osmoprotectant and helping plants under salinity stress. There are enzymes such as trehalose synthase, alpha-trehalose-phosphate synthase, and trehalose-6-phosphate phosphatase (coded by otsAB genes) which are involved in the formation of trehaloses in HT-PGPR (Vílchez et al., 2016). Research has revealed that most of the bacteria follow two routes i.e., treY/treZ and treS, for trehalose synthesis and to withstand the harsh effects of salinity stress. Like many other osmoprotectants, it has been observed that the levels of trehaloses spike during drought and salinity stress (Duan et al., 2009). Rodríguez-Salazar et al. (2009) showed that inoculation of Azospirillum brasilence overexpressing trehalose biosynthesis gene improved leaf and root biomass of maize plants and also imparted osmotic stress tolerance to them. Phaseolus vulagris when inoculated with Rhizobium etli having trehalose-6-phosphate synthase overexpressing gene, showed adaptation to osmotic stress, enhanced number of nodules as well as resulted in higher plant biomass (Suárez et al., 2008).
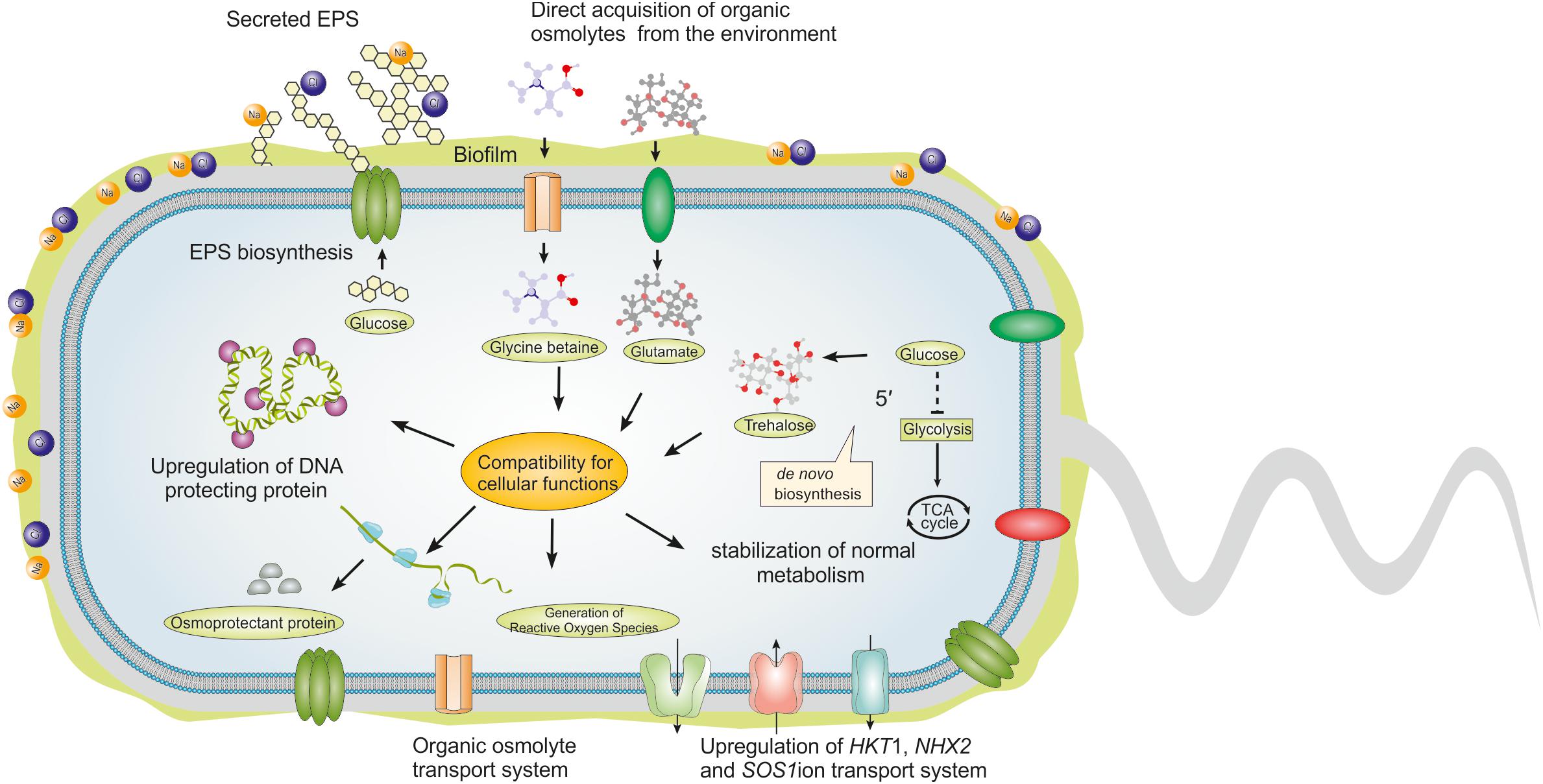
Figure 2. Role of osmoprotective compounds and exopolysaccharides (EPS) produced by HT-PGPR in salt-tolerance. Under salt stress, HT-PGPR produce osmoprotective compounds and EPS. Osmoprotective compounds or compatible solutes can be accumulated either through direct acquisition from the surrounding environment (if available) or through de novo biosynthesis. In general, osmoprotective compounds are highly soluble and carry no net charge at physiological pH. Accumulation of osmoprotective compounds at high intracellular concentrations don’t interrupt vital cellular processes. The utmost importance of osmoprotective compounds is to ensure cellular compatibility for the normal metabolic process. Apart from these, they also take part in the upregulation of ion transporters essential for restoring the osmotic equilibrium. Production of EPS by HT-PGPR is strongly correlated with the plant’s survival in high salt concentrations. HT-PGPR can secrete EPS or form biofilm under salt stress. Physiological and chemical properties of the biofilm are also linked with enhancing water retention and neutralizing the harmful effect of salts. The anionic EPS can hold several times its weight of water and at the same time binds with positive salt ions.
Osmolyte production and accumulation by HT-PGPR is thus one of the key mechanisms which helps in minimizing various stresses caused by salinity in the plants. Genetic manipulation of secondary metabolite producing genes from HT-PGPR can also be utilized for designing salt-resilient transgenic crops in order to reduce agricultural losses (Rodríguez-Salazar et al., 2009). However, ample research regarding salt stress responses in HT-PGPR is required for a better understanding of the metabolic pathways involved in their production. This can give new insights for the development of innovative and novel biotechnological products for enhancing crop productivity of saline agro-ecosystems.
Exopolysaccharides (EPS)
Exopolysaccharides are commonly produced metabolites of HT-PGPR. Under extreme saline conditions about 40–90% of the extracellular matrix of bacterial weight is due to EPS formation only (Flemming and Wingender, 2001; Fatima and Arora, 2019). EPS help in microbial attachment to plant roots, in biofilm formation to protect cells from desiccation due to salt stress and enhance mobility of root associated bacteria (Ruppel et al., 2013; Qin et al., 2016; Liu et al., 2017b). Production of EPS may vary with bacterial growth phase, medium composition, and exposure of environmental stressors such as salinity and drought (De Vuyst and Degeest, 1999; Kaushal and Wani, 2016). EPS have also been reported to exhibit high antioxidant property and confer tolerance to bacteria against ROS- dependent cell death. In this context, Meneses et al. (2017) demonstrated EPS-mediated protection of endophytic bacterium Gluconacetobacter diazotrophicus against oxidative damage in vitro and during colonization of rice plants. Similarly, EPS produced by a halotolerant endophyte Glutamicibacter halophytocola KLBMP 5180 was evaluated for its multilayer antioxidant activity that can be exploited to alleviate salt stress damage in crops (Xiong et al., 2020). EPS are also supposed to be involved in soil aggregation and increasing root adhering of soil (RAS) by the formation of a sheath around plant’s roots thereby increasing availability of water and acquisition of nutrients such as N, P, K and Fe from the soil (Gupta et al., 2015; Etesami and Beattie, 2018). Mukherjee et al. (2019) reported that under salt stress conditions EPS produced by Halomonas sp. EX01 helped in osmotic stress tolerance and promotion of rice growth. EPS can reduce ionic toxicity in plants by minimizing Na+ influx through an expression of HKT1/K+ transporter (Zhang et al., 2008). By influencing key metabolic processes in plants and retaining physicochemical properties of soil, EPS producing bacteria can also contribute to the improvement of crop productivity under saline conditions (Qurashi and Sabri, 2012; Xiong et al., 2019). Apart from these defined roles, bacterial EPS are also linked with cellular sensing and recognition process in the rhizosphere, protection of plant from phytopathogens and to serve as a carbon source during nutrient deficient conditions (Tewari and Arora, 2016; Mishra and Arora, 2018). Tewari and Arora (2018) reported that inoculation of EPS producing Pseudomonas sp. enhanced the yield of sunflower crop in a highly saline field (EC > 10 dS/m). The study also reported that the inoculation of HT-PGPR also reduced incidence of charcoal rot disease in Macrophomina phaseolina infested saline soil. Earlier, Ashraf et al. (2004) demonstrated the role of EPS producing salt-tolerant Bacillus amyloliquefaciens, Bacillus insolitus, Microbacterium spp., and Pseudomonas syringae in improving the growth of wheat by the inhibition of Na+ influx into stele of plant growing under salinity stress. Similarly, Mahmood et al. (2016) concluded that under salinity stress, treatment of mung bean with EPS producing Enterobacter cloacae and Bacillus drentensis enhanced nutrient availability and water uptake in plants by the formation of biofilm in the root zone. In another study, inoculation of EPS producing endophyte, Pantoea alhagi NX-11 improved salt tolerance of rice seedlings by improving the antioxidant activity resulting in better growth in comparison to the plants treated with EPS mutant NX-11eps– (Sun et al., 2020). Likewise, treatment of maize with EPS producing Azotobacter chrococcum strains C5 and C9 under saline conditions alleviated the saline stress by several means i.e., increasing K+/Na+ ratio and ions uptake (Na+, K+, Ca2+, Mg2+), chlorophyll content, and accumulation of proline and polyphenols (Rojas-Tapias et al., 2012). EPS based bioformulation of Alcaligenes sp. was found to be very effective in enhnacing growth and reducing osmotic stress in rice when grown in saline conditions (Fatima et al., 2020). Overall, research suggests that EPS produced by PGPR play an important role to overcome salinity stress in plants and can be used in bioinoculants to improve the rhizosphere colonization, soil quality, and nutrient acquisition in saline conditions. EPS can also be used as amendments in bioinoculants for protecting the PGPR microbe from initial stress faced in saline soils at the time of inoculation.
Volatile Organic Compounds (VOCs)
Halotolerant plant growth promoting rhizobacteria when exposed to stress conditions like salinity, are known to produce VOCs which are low molecular weight compounds (below 300 Da), and lipophilic in nature, with low boiling point (Vespermann et al., 2007; Kanchiswamy et al., 2015). A vast array of microbial volatiles have been reported and among them, many are recognized for their potential in improving overall plant health (Kanchiswamy et al., 2015). These compounds are often used as markers for specific detection of microbial species in the environment and in accessing the nature of interaction among microbial communities (Fiedler et al., 2001; Tirranen and Gitelson, 2006). Their roles in regulating bacterial motility in plant-microbial interactions, modulating virulence factors, biosynthesis of osmolytes like GB, phytohormones (auxins, cytokinins, and gibberellins) and siderophores are also studied and reported (Ryu et al., 2004; Liu and Zhang, 2015; Sharifi and Ryu, 2018). In a study, Tahir et al. (2017) showed that VOCs albuterol and 1,3-propanediol produced by B. subtilis SYST2 modulated levels of plant hormones expansin, auxin, gibberellin, cytokinin, and ethylene. Geosmin, dimethyl disulfide, 2,3-butanediol, and acetoin are the most studied bacterial VOCs that help in soil formation processes, composting, sulfur nutrition, auxin homeostasis and cell expansion, induction of systemic and drought tolerance in plants (Stahl and Parkin, 1996; Li et al., 2004; Zhang et al., 2007; Cho et al., 2008; Meldau et al., 2013). In a study, Gutierrez-Luna et al. (2010) reported emission of 22 types of VOCs (aldehydes, ketones, and alcohols were the most abundant) by six Bacillus strains and further revealed that most of VOCs can stimulate primary root growth and lateral root development in Arabidopsis thaliana. Similarly, volatile compounds produced by root associated Microbacterium spp. showed increment in root and shoot biomass of A. thaliana. The study revealed that even a brief exposure of bacterial VOCs also stimulated plant growth suggesting that these can be used to prime crops without direct and prolonged exposure of plants to bacteria. Moreover, it was also demonstrated that the VOC mediated plant growth promotion was tissue specific and showed biomass increment only in plants exposed to volatiles via roots (Cordovez et al., 2018). Two bacterial volatiles, 4-nitroguaiacol, and quinoline produced by salt-tolerant Pseudomonas simiae were reported to induce soybean growth under 150 mM salt stress (Vaishnav et al., 2016). A VOC producing HT-PGPR Paraburkholderia phytofirmans PsJN, was not only reported to help in salt-stress amelioration but also promoted A. thaliana growth in very saline conditions (Ledger et al., 2016). VOCs production is also linked to the regulation of HKT1/K+ transporter which is responsible for inhibition of Na+ influx during salt stress. For example, Zhang et al. (2008), found that a VOC producing B. subtilis improved salt-tolerance in A. thaliana by downregulating HKT1 gene expression. Bhattacharyya and Lee (2017) investigated the role of mixtures of three bacterial volatiles produced by Alcaligenes faecalis JBCS1294. The study demonstrated that blends of VOCs (butyric acid, propionic acid, and benzoic acid) increased plant growth and induced salt tolerance in A. thaliana by modulating its hormonal pathways and ionic transporters. Systematic exploration of microbial VOCs indicates that these compounds could also be involved in novel biological functions and ecological roles that are presently not known (Kanchiswamy et al., 2015). Although it is certain that VOCs play important roles, such as in regulating growth hormones and ion acquisition, there still is a lot more to be explored regarding the direct role of these metabolites and their potential to ameliorate salt stress in plants. A better understanding of microbial VOCs capable of eliminating the negative effects of salt stress in the plant might be employed in the future for the development of a range of novel bioinoculants with great agronomic importance.
Future
Halotolerant plant growth promoting rhizobacteria are one of the pre-eminent group of microbes that can be utilized in the engineering of the rhizosphere in saline soils to replenish their fertility and increasing crop yield (Stringlis et al., 2018; Arora et al., 2020). It has been established that secondary metabolites produced by HT-PGPR can be very useful to develop novel bioinoculants for the cultivation of crops in saline soil (Arora, 2019). Secondary metabolites, such as EPS from HT-PGPR not only can overcome issues related to shorter shelf-life but also increase the survival of bacterial/fungal strains in the bioinoculants (Arora and Mishra, 2016). Techniques such as metagenomics can be an important tool for identification, designing and reassembling of novel genetic pathways and for the construction of new biosensors in order to produce secondary metabolites from diverse HT-PGPR (de Frias et al., 2018). Based on the results, metagenomic libraries can be constructed related to structural and functional genes for novel and known metabolites. Similarly, Artificial Gene Operon Assembly System (AGOS) has also come up in which a particular metabolite pathway can be optimized and controlled with chemical modifications. This will help in introducing the assembly of artificial gene clusters in native or heterologous hosts and can increase the overexpression of metabolites of interest (Basitta et al., 2017). Bioinformatic tools can be helpful in deciphering distribution and functional traits within bacterial genomes that are involved in rhizosphere colonization under saline conditions (Barea, 2015). Dragon Explorer of Osmoprotection associated Pathways’ (DEOP), a database on osmolytes is already available providing curated information and facilitating deeper insights on osmolytes and their associated pathways in the microbial genome (Bougouffa et al., 2014). The study of plant microbiome and its intricate mechanisms is a crucial strategy to develop designer salt-tolerant crops (Macdonald and Singh, 2014; Rodriguez et al., 2019). This will unravel the hidden untapped arsenal of endophytes associated with halophytic plants and other extreme habitats. Due to the ability to form symbiotic relationship with hosts, salt-tolerant endophytes should be explored in the future (Qin et al., 2018). Stress tolerant genes can also be incorporated in plants through transposon-mediated genetic modification (Arora et al., 2018). Transcriptome analysis has revealed that genes functioning at high salt concentrations from halotolerant bacteria can be used for the development of stress-tolerant plant varieties to expand agricultural productivity (Das et al., 2020). In addition, nanoencapsulation technology has been recently introduced and can be applied for field trials (De Gregorio et al., 2017). The technique can be used to protect the PGPR from sudden environmental stresses, improve their dispersal and will serve in the controlled release of microbes in the field/rhizosphere (Vejan et al., 2016). Further research on the identification and utilization of more such nanoparticles should be made so that these growth enhancers can be used in sustainable agricultural practices. These high throughput protocols will open new gateways for enhanced production of bioactive products from HT-PGPR, reducing the use of agrichemicals (Backer et al., 2018). Research should also be focused on co-cultivation of potent salt-tolerant strains possessing positive synergy for the production of novel metabolites which can work in tandem to tackle diverse problems (Singh et al., 2019). Interlinking these biotechnological processes with agronomy and investigating the diversity and composition of halotolerant microbes will provide improvement in cultivating crops under stressed conditions. The market size of plant growth regulators is currently increasing at the rate of 3.6%2 and is projected to enhance even further in coming future. This presents a scenario of global demand for growth enhancers for plants and hence provides a roadmap for the commercialization of microbial products. However, biostimulants involving HT-PGPR and their metabolites are yet to take off and enter the market. Hence development of tailor made bioformulations from these unique PGPR and their metabolites can be crucial to combat salinity and improve the yield of salt affected agro-ecosystems leading to better productivity and sustainability of agro-ecosystems.
Conclusion
Halotolerant plant growth promoting rhizobacteria act as a promising probiotic for salt-affected plants and in restoring the natural equilibrium of saline soils. The ability of these bacteria to survive in saline conditions makes them an excellent tool in realizing the targets of sustainable agriculture. Information about halotolerant microbiota, their structure and composition are still in its nascent stage. Hence, extensive research is needed to understand the saline mitigation mechanisms which will provide new insights in designing future bioformulations to help remediate salt degraded lands and to achieve targets of food security.
Author Contributions
NKA conceptualized the idea and approved the final manuscript to be published. All the authors read, discussed as well as equally contributed to the writing of the manuscript.
Conflict of Interest
The authors declare that the research was conducted in the absence of any commercial or financial relationships that could be construed as a potential conflict of interest.
Footnotes
- ^ http://www.fao.org/soils-portal/soil-management/management-of-some-problem-soils/salt-affected-soils/more-information-on-salt-affected-soils/en/
- ^ https://www.marketresearch.com/MarketsandMarkets-v3719/Plant-Growth-Regulators-Type-Auxins-9511806/
References
Abbas, R., Rasul, S., Aslam, K., Baber, M., Shahid, M., Mubeen, F., et al. (2019). Halotolerant PGPR: a hope for cultivation of saline soils. J. King. Saud. Univ. Sci. 31, 1195–1201. doi: 10.1016/j.jksus.2019.02.019
Arora, N. K. (2019). Impact of climate change on agriculture production and its sustainable solutions. Environ. Sustain. 2, 95–96. doi: 10.1007/s42398-019-00078-w
Arora, N. K., Fatima, T., Mishra, I., Verma, M., Mishra, J., and Mishra, V. (2018). Environmental sustainability: challenges and viable solutions. Environ. Sustain. 1, 309–340. doi: 10.1007/s42398-018-00038-w
Arora, N. K., Fatima, T., Mishra, J., Mishra, I., Verma, S., Verma, R., et al. (2020). Halotolerant plant growth promoting rhizobacteria for improving productivity and remediation of saline soils. J. Adv. Res. doi: 10.1016/j.jare.2020.07.003
Arora, N. K., and Mishra, J. (2016). Prospecting the roles of metabolites and additives in future bioformulations for sustainable agriculture. Appl. Soil. Ecol. 107, 405–407. doi: 10.1016/j.apsoil.2016.05.020
Ashraf, M., Hasnain, S., Berge, O., and Mahmood, T. (2004). Inoculating wheat seedlings with exopolysaccharide-producing bacteria restricts sodium uptake and stimulates plant growth under salt stress. Biol. Fertil. Soils 40, 157–162. doi: 10.1007/s00374-004-0766-y
Aslam, F., and Ali, B. (2018). Halotolerant bacterial diversity associated with Suaeda fruticosa (L.) forssk. improved growth of maize under salinity stress. Agronomy 8:131. doi: 10.3390/agronomy8080131
Backer, R., Rokem, J. S., Ilangumaran, G., Lamont, J., Praslickova, D., Ricci, E., et al. (2018). Plant growth-promoting rhizobacteria: context, mechanisms of action, and roadmap to commercialization of biostimulants for sustainable agriculture. Front. Plant. Sci. 9:1473. doi: 10.3389/fpls.2018.01473
Barea, J. M. (2015). Future challenges and perspectives for applying microbial biotechnology in sustainable agriculture based on a better understanding of plant-microbiome interactions. J. Soil Sci. Plant Nutr. 15, 261–282. doi: 10.4067/S0718-95162015005000021
Basitta, P., Westrich, L., Rösch, M., Kulik, A., Gust, B., and Apel, A. K. (2017). AGOS: a plug-and-play method for the assembly of artificial gene operons into functional biosynthetic gene clusters. ACS. Synth. Biol. 6, 817–825. doi: 10.1021/acssynbio.6b00319
Bharti, N., Barnawal, D., Awasthi, A., Yadav, A., and Kalra, A. (2014). Plant growth promoting rhizobacteria alleviate salinity induced negative effects on growth, oil content and physiological status in Mentha arvensis. Acta Physiol. Plant. 36, 45–60. doi: 10.1007/s11738-013-1385-8
Bharti, N., Barnawal, D., Maji, D., and Kalra, A. (2015). Halotolerant PGPRs prevent major shifts in indigenous microbial community structure under salinity stress. Microb. Ecol. 70, 196–208. doi: 10.1007/s00248-014-0557-4
Bharti, N., Pandey, S. S., Barnawal, D., Patel, V. K., and Kalra, A. (2016). Plant growth promoting rhizobacteria Dietzia natronolimnaea modulates the expression of stress responsive genes providing protection of wheat from salinity stress. Sci. Rep. 6:34768. doi: 10.1038/srep34768
Bhattacharyya, D., and Lee, Y. H. (2017). A cocktail of volatile compounds emitted from Alcaligenes faecalis JBCS1294 induces salt tolerance in Arabidopsis thaliana by modulating hormonal pathways and ion transporters. J. Plant. Physiol. 214, 64–73. doi: 10.1016/j.jplph.2017.04.002
Bougouffa, S., Radovanovic, A., Essack, M., and Bajic, V. B. (2014). DEOP: a database on osmoprotectants and associated pathways. Database (Oxford). 2014:bau100. doi: 10.1093/database/bau100
Carmen, B., and Roberto, D. (2011). “Soil bacteria support and protect plants against abiotic stresses,” in Abiotic Stress in Plants Mechanisms and Adaptations, ed. A. Shaner, (London: InTech), 143–170. doi: 10.5772/23310
Chandra, G., Chater, K. F., and Bornemann, S. (2011). Unexpected and widespread connections between bacterial glycogen and trehalose metabolism. Microbiology 157, 1565–1572. doi: 10.1099/mic.0.044263-0
Chen, L., Liu, Y., Wu, G., Njeri, V. K., Shen, Q., et al. (2016). Induced maize salt tolerance by rhizosphere inoculation of Bacillus amyloliquefaciens SQR9. Physiol. Plant 158, 34–44. doi: 10.1111/ppl.12441
Cho, S. M., Kang, B. R., Han, S. H., Anderson, A. J., Park, J.-Y., Lee, Y.-H., et al. (2008). 2R,3R-butanediol, a bacterial volatile produced by Pseudomonas chlororaphisO6, is involved in to drought in Arabidopsis thaliana. Mol. Plant-Microbe. Interact. 21, 1067–1075. doi: 10.1094/mpmi-21-8-1067
Cordovez, V., Schop, S., Hordijk, K., de Boulois, H. D., Coppens, F., Hanssen, I., et al. (2018). Priming of plant growth promotion by volatiles of root-associated Microbacterium spp. Appl. Environ. Microbiol. 84:e01865-18. doi: 10.1128/AEM.01865-18
Das, P., Behera, B. K., Chatterjee, S., Das, B. K., and Mohapatra, T. (2020). De novo transcriptome analysis of halotolerant bacterium Staphylococcus sp. strain P-TSB-70 isolated from East coast of India: in search of salt stress tolerant genes. PLoS One. 15:e0228199. doi: 10.1371/journal.pone.0228199
de Frias, U. A., Pereira, G. K. B., Guazzaroni, M. E., and Silva-Rocha, R. (2018). Boosting secondary metabolite production and discovery through the engineering of novel microbial biosensors. Biomed. Res. Int. 2018:7021826. doi: 10.1155/2018/7021826
De Gregorio, P. R., Michavila, G., Muller, L. R., de Souza, B. C., Pomares, M. F., de Sa, E. L. S., et al. (2017). Beneficial rhizobacteria immobilized in nanofibers for potential application as soybean seed bioinoculants. PLoS One 12:e0176930. doi: 10.1371/journal.pone.0176930
De Vuyst, L., and Degeest, B. (1999). Heteropolysaccharides from lactic acid bacteria. FEMS. Microbiol. Rev. 23, 153–177. doi: 10.1111/j.1574-6976.1999.tb00395.x
Diacono, M., and Montemurro, F. (2015). Effectiveness of organic wastes as fertilizers and amendments in salt-affected soils. Agriculture 5, 221–230. doi: 10.3390/agriculture5020221
Dodd, I. C., and Perez-Alfocea, F. (2012). Microbial amelioration of crop salinity stress. J. Exp. Bot. 63, 3415–3428. doi: 10.1093/jxb/ers033
Duan, J., Müller, K. M., Charles, T. C., Vesely, S., and Glick, B. R. (2009). 1-aminocyclopropane-1-carboxylate (ACC) deaminase genes in rhizobia from southern Saskatchewan. Microb. Ecol. 57, 423–436. doi: 10.1007/s00248-008-9407-6
Egamberdieva, D., Wirth, S., Bellingrath-Kimura, S. D., Mishra, J., and Arora, N. K. (2019). Salt-tolerant plant growth promoting rhizobacteria for enhancing crop productivity of saline soils. Front. Microbiol. 10:2791. doi: 10.3389/fmicb.2019.02791
Elsakhawy, T. A., Fetyan, N. A. H., and Ghazi, A. A. (2019). The potential use of ectoine produced by a moderately halophilic bacteria Chromohalobacter salexigens KT989776 for enhancing germination and primary seedling of flax “Linum usitatissimum L.” under salinity conditions. Biotechnol. J. Int. 23, 1–12. doi: 10.9734/bji/2019/v23i330078
Etesami, H., and Beattie, G. A. (2018). Mining halophytes for plant growth-promoting halotolerant bacteria to enhance the salinity tolerance of non-halophytic crops. Front. Microbiol. 9:148. doi: 10.3389/fmicb.2018.00148
Fatima, T., and Arora, N. K. (2019). “Plant growth-gromoting rhizospheric microbes for remediation of saline soils,” in Phyto and Rhizo Remediation, eds N. K. Arora, and N. Kumar, (Singapore: Springer), 121–146. doi: 10.1007/978-981-32-9664-0_5
Fatima, T., Mishra, I., Verma, R., and Arora, N. K. (2020). Mechanisms of halotolerant plant growth promoting Alacaligenes sp. involved in salt tolerance and enhancement of the growth of rice under salinity stress. 3 Biotech 10:361. doi: 10.1007/s13205-020-02348-5
Fiedler, K., Schutz, E., and Geh, S. (2001). Detection of microbial volatile organic compounds (MVOCs) produced by moulds on various materials. Int. J. Hygiene Environ. Health 204, 111–121.
Flemming, H. C., and Wingender, J. (2001). Relevance of microbial extracellular polymeric substances (EPSs)–Part I: structural and ecological aspects. Water Sci. Technol. 43, 1–8. doi: 10.2166/wst.2001.0326
Giri, J. (2011). Glycinebetaine and abiotic stress tolerance in plants. Plant. Signal. Behav. 6, 1746–1751. doi: 10.4161/psb.6.11.17801
Grammann, K., Volke, A., and Kunte, H. J. (2002). New type of osmoregulated solute transporter identified in halophilic members of the bacteria domain: TRAP transporter TeaABC mediates uptake of ectoine and hydroxyectoine in Halomonas elongata DSM2581T. Bacteriology 184, 3078–3085. doi: 10.1128/jb.184.11.3078-3085.2002
Gupta, G., Parihar, S. S., Ahirwar, N. K., Snehi, S. K., and Singh, V. (2015). Plant growth promoting rhizobacteria (PGPR): current and future prospects for development of sustainable agriculture. J. Microb. Biochem. Technol. 7:2.
Gutierrez-Luna, F. M., Lopez-Bucio, J., Altamirano-Hernandez, J., Valencia-Cantero, E., de la Cruz, H. R., and Macias-Rodriguez, L. (2010). Plant growth-promoting rhizobacteria modulate root-system architecture in Arabidopsis thaliana through volatile organic compound emission. Symbiosis 51, 75–83. doi: 10.1007/s13199-010-0066-2
Han, Q. Q., Lü, X. P., Bai, J. P., Qiao, Y., Paré, P. W., Wang, S. M., et al. (2014). Beneficial soil bacterium Bacillus subtilis (GB03) augments salt tolerance of white clover. Front. Plant Sci. 5:525. doi: 10.3389/fpls.2014.00525
Hashem, A., Abd_Allah, E. F., Alqarawi, A. A., Al-Huqail, A. A., and Shah, M. A. (2016). Induction of osmoregulation and modulation of salt stress in acacia gerrardii benth. by arbuscular mycorrhizal fungi and bacillus subtilis (bera 71). BioMed. Res. Int. 2016:6294098. doi: 10.1155/2016/6294098
Hong, B. H., Joe, M. M., Selvakumar, G., Kim, K. Y., Choi, J. H., and Sa, T. M. (2017). Influence of salinity variations on exocellular polysaccharide production, biofilm formation and flocculation in halotolerant bacteria. J. Environ. Biolog. 38, 657–664. doi: 10.22438/jeb/38/4/mrn-284
Hoque, M. A., Banu, M. N., Okuma, E., Amako, K., Nakamura, Y., Shimoishi, Y., et al. (2007). Exogenous proline and glycinebetaine increase NaCl-induced ascorbate-glutathione cycle enzyme activities, and proline improves salt tolerance more than glycinebetaine in tobacco Bright Yellow-2 suspension-cultured cells. J. Plant. Physiol. 164, 1457–1468. doi: 10.1016/j.jplph.2006.10.004
Kanchiswamy, C. N., Malnoy, M., and Maffei, M. E. (2015). Chemical diversity of microbial volatiles and their potential for plant growth and productivity. Front. Plant. Sci. 6:151. doi: 10.3389/fpls.2015.00151
Kang, S. M., Shahzad, R., Bilal, S., Khan, A. L., Park, Y. G., Lee, K. E., et al. (2019). Indole-3-acetic-acid and ACC deaminase producing Leclercia adecarboxylata MO1 improves Solanum lycopersicum L. growth and salinity stress tolerance by endogenous secondary metabolites regulation. BMC. Microbiol. 19:80. doi: 10.1186/s12866-019-1450-6
Kappes, R. M., Kempf, B., Kneip, S., Boch, J., Gade, J., Meier-Wagner, J., et al. (1999). Two evolutionary closely related ABC transporters mediate the uptake of choline for synthesis of the osmoprotectant glycine betaine in Bacillus subtilis. Mol. Microbiol. 32, 203–216. doi: 10.1046/j.1365-2958.1999.01354.x
Kasim, W. A., Gaafar, R. M., Abou-Ali, R., Omar, M. N., and Hewait, H. M. (2016). Effect of biofilm forming plant growth promoting rhizobacteria on salinity tolerance in barley. Ann. Agric. Sci. 61, 217–227. doi: 10.1016/j.aoas.2016.07.003
Kasotia, A., Varma, A., Tuteja, N., and Choudhary, D. (2016). Amelioration of soybean plant from saline-induced condition by exopolysaccharide producing Pseudomonas-mediated expression of high affinity K -transporter (HKT1) gene. Curr. Sci. 111, 1961–1967. doi: 10.18520/cs/v111/i12/1961-1967
Kaur, G., and Asthir, B. (2015). Proline: a key player in plant abiotic stress tolerance. Biol. Plant 59, 609–619. doi: 10.1007/s10535-015-0549-3
Kaushal, M., and Wani, S. P. (2016). Plant-growth-promoting rhizobacteria: drought stress alleviators to ameliorate crop production in drylands. Ann. Microbiol. 66, 35–42. doi: 10.1016/j.agee.2016.06.031
Khan, M. A., Asaf, S., Khan, A. L., Adhikari, A., Jan, R., Ali, S., et al. (2019). Halotolerant rhizobacterial strains mitigate the adverse effects of NaCl stress in soybean seedlings. BioMed Res. Inter. 2019:9530963. doi: 10.1155/2019/9530963
Kim, K., Jang, Y. J., Lee, S. M., Oh, B. T., Chae, J. C., and Lee, K. J. (2014). Alleviation of salt stress by Enterobacter sp. EJ01 in tomato and Arabidopsis is accompanied by up-regulation of conserved salinity responsive factors in plants. Mol. Cells 37, 109–117. doi: 10.14348/molcells.2014.223
Kohl, D. H., Straub, P. F., and Shearer, G. (1994). Does proline play a special role in bacteroid metabolism? Plant. Cell. Environ. 17, 1257–1262. doi: 10.1111/j.1365-3040.1994.tb00527.x
Krasensky, J., and Jonak, C. (2012). Drought, salt, and temperature stress-induced metabolic rearrangements and regulatory networks. J. Exp. Bot. 63, 1593–1608. doi: 10.1093/jxb/err460
Kunst, F., Ogasawara, N., Moszer, I., Albertini, A. M., Alloni, G., Azevedo, V., et al. (1997). The complete genome sequence of the Gram-positive bacterium Bacillus subtilis. Nature 390, 249–256. doi: 10.1038/36786
Kwon, Y. S., Ryu, C.-M., Lee, S., Park, H. B., Han, K. S., Lee, J. H., et al. (2010). Proteome analysis of Arabidopsis seedlings exposed to bacterial volatiles. Planta 232, 1355–1370. doi: 10.1007/s00425-010-1259-x
Ledger, T., Rojas, S., Timmermann, T., Pinedo, I., Poupin, M. J., Garrido, T., et al. (2016). Volatile-Mediated effects predominate in Paraburkholderia phytofirmans growth promotion and salt stress tolerance of Arabidopsis thaliana. Front. Microbiol. 7:1838. doi: 10.3389/fmicb.2016.01838
Li, H. F., Imai, T., Ukita, M., Sekine, M., and Higuchi, T. (2004). Compost stability assessment using a secondary metabolite: geosmin. Environ. Technol. 25, 1305–1312. doi: 10.1080/09593332508618374
Liu, S., Hao, H., Lu, X., Zhao, X., Wang, Y., Zhang, Y., et al. (2017a). Transcriptome profiling of genes involved in induced systemic salt tolerance conferred by Bacillus amyloliquefaciens FZB42 in Arabidopsis thaliana. Sci. Rep. 7:10795. doi: 10.1038/s41598-017-11308-8
Liu, X., Luo, Y., Li, Z., Wang, J., and Wei, G. (2017b). Role of exopolysaccharide in salt stress resistance and cell motility of Mesorhizobium alhagi CCNWXJ12–2T. Appl. Microbiol. Biotechnol. 101, 2967–2978. doi: 10.1007/s00253-017-8114-y
Liu, X. M., and Zhang, H. (2015). The effects of bacterial volatile emissions on plant abiotic stress tolerance. Front. Plant. Sci. 6:774. doi: 10.3389/fpls.2015.00774
Macdonald, C., and Singh, B. (2014). Harnessing plant-microbe interactions for enhancing farm productivity. Bioengineered 5, 5–9. doi: 10.4161/bioe.25320
Mahmood, S., Daur, I., Al-Solaimani, S. G., Ahmad, S., Madkour, M. H., Yasir, M., et al. (2016). Plant growth promoting rhizobacteria and silicon synergistically enhance salinity tolerance of mung bean. Front. Plant. Sci. 7:876. doi: 10.3389/fpls.2016.00876
Mahmoud, O. M. B., Hidri, R., Talbi-Zribi, O., Taamalli, W., Abdelly, C., and Djébalic, N. (2020). Auxin and proline producing rhizobacteria mitigate salt-induced growth inhibition of barley plants by enhancing water and nutrient status. S. Afr. J. 128, 209–217. doi: 10.1016/j.sajb.2019.10.023
Malekzadeh, P. (2015). Influence of exogenous application of glycinebetaine on antioxidative system and growth of salt-stressed soybean seedlings (Glycine max L.). Physiol. Mol. Biol. Plants 21, 225–232. doi: 10.1007/s12298-015-0292-4
Masouleh, S. S. S. (2019). The role of organic solutes in the osmotic adjustment of chilling-stressed plants (vegetable, ornamental and crop plants). Ornam. Hortic 24, 434–442. doi: 10.1590/2447-536X.v25i4.2073
Meena, M., Divyanshu, K., Kumar, S., Swapnil, P., Zehra, A., Shukla, V., et al. (2019). Regulation of L-proline biosynthesis, signal transduction, transport, accumulation and its vital role in plants during variable environmental conditions. Heliyon 12:e02952. doi: 10.1016/j.heliyon.2019.e02952
Meldau, D. G., Meldau, S., Hoang, L. H., Underberg, S., Wünsche, H., and Baldwin, I. T. (2013). Dimethyl disulfide produced by the naturally associated bacterium Bacillus sp. B55 promotes Nicotiana attenuate growth by enhancing sulfur nutrition. Plant. Cell 25, 2731–2747. doi: 10.1105/tpc.113.114744
Meneses, C., Gonçalves, T., Alquéres, S., Rouws, L., Serrato, R., Vidal, M., et al. (2017). Gluconacetobacter diazotrophicus exopolysaccharide protects bacterial cells against oxidative stress in vitro and during rice plant colonization. Plant. Soil 416, 133–147. doi: 10.1007/s11104-017-3201-5
Mishra, J., and Arora, N. K. (2018). Secondary metabolites of fluorescent pseudomonads in biocontrol of phytopathogens for sustainable agriculture. Appl. Soil Ecol. 125, 35–45. doi: 10.1016/j.apsoil.2017.12.004
Moghaieb, R. E. A., Nakamura, H., Saneoka, and Fujita, K. (2011). Evaluation of salt tolerance in ectoine-transgenic tomato plants (Lycopersicon esculentum) in terms of photosynthesis, osmotic adjustment, and carbon partitioning. GM Crops. 2, 58–65. doi: 10.4161/gmcr.2.1.15831
Moghaieb, R. E. A., Tanaka, N., Saneoka, H., Murooka, Y., Ono, H., and Morikawa, H. (2006). Characterization of salt tolerance in ectoine-transformed tobacco plants (Nicotiana tabaccum): photosynthesis, osmotic adjustment, and nitrogen partitioning. Plant. Cell. Environ. 29, 173–182. doi: 10.1111/j.1365-3040.2005.01410.x
Morris, J., and González, J. E. (2009). The Novel Genes emmABC, are associated with exopolysaccharide production, motility, stress adaptation, and symbiosis in Sinorhizobium meliloti. J. Bacteriol. 191:5890. doi: 10.1128/JB.00760-09
Mukherjee, P., Mitra, A., and Roy, M. (2019). Halomonas rhizobacteria of avicennia marina of indian sundarbans promote rice growth under saline and heavy metal stresses through exopolysaccharide production. Front. Microbiol. 10:1207. doi: 10.3389/fmicb.2019.01207
Mukhtar, S., Zareen, M., Khaliq, Z., Mehnaz, S., and Malik, K. A. (2019). Phylogenetic analysis of halophyte-associated rhizobacteria and effect of halotolerant and halophilic phosphate-solubilizing biofertilizers on maize growth under salinity stress conditions. J. Appl. Microbiol. 128, 556–573. doi: 10.1111/jam.14497
Munns, R. (2002). Comparative physiology of salt and water stress. Plant. Cell. Environ. 25, 239–250. doi: 10.1046/j.0016-8025.2001.00808.x
Nadeem, S. M., Zahir, Z. A., Naveed, M., and Nawaz, S. (2013). Mitigation of salinity-induced negative impact on the growth and yield of wheat by plant growth-promoting rhizobacteria in naturally saline conditions. Ann. Microbiol. 63, 225–232. doi: 10.1007/s13213-012-0465-0
Numan, M., Bashir, S., Khan, Y., Mumtaz, R., Shinwari, Z. K., et al. (2018). Plant growth promoting bacteria as an alternative strategy for salt tolerance in plants: a review. Microbiol. Res. 209, 21–32. doi: 10.1016/j.micres.2018.02.003
Orozco-Mosqueda, M. D. C., Duan, J., DiBernardo, M., Zetter, E., Campos-García, J., Glick, B. R., et al. (2019). The production of ACC deaminase and trehalose by the plant growth promoting bacterium Pseudomonas sp. UW4 synergistically protect tomato plants against salt stress. Front. Microbiol. 10:1392. doi: 10.3389/fmicb.2019.01392
Pan, J., Peng, F., Xue, X., You, Q., Zhang, W., Wang, T., et al. (2019). The growth promotion of two salt-tolerant plant groups with PGPR inoculation: a meta-analysis. Sustainability 11:378. doi: 10.3390/su11020378
Paul, D., and Lade, H. (2014). Plant-growth-promoting rhizobacteria to improve crop growth in saline soils: a review. Agron. Sustain. Dev. 34, 737–752. doi: 10.1007/s13593-014-0233-6
Pérez-López, U., Robredo, A., Lacuesta, M., Mena-Petite, A., and Muñoz-Rueda. (2012). Elevated CO2 reduces stomatal and metabolic limitations on photosynthesis caused by salinity in Hordeum vulgare. Photosynth. Res. 111, 269–228. doi: 10.1007/s11120-012-9721-1
Phour, M., and Sindhu, S. S. (2020). Amelioration of salinity stress and growth stimulation of mustard (Brassica juncea L.) by salt-tolerant Pseudomonas species. Appl. Soil. Ecol. 149:103518. doi: 10.1016/j.apsoil.2020.103518
Qadir, M., Quillerou, E., Nangia, V., Murtaza, G., Singh, M., Thomas, R. J., et al. (2014). Economics of salt-induced land degradation and restoration. Nat. Res. Forum 38, 282–295. doi: 10.1111/1477-8947.12054
Qin, S., Feng, W.-W., Zhang, Y.-J., Wang, T.-T., Xiong, Y.-W., and Xing, K. (2018). Diversity of bacterial microbiota of coastal halophyte Limonium sinense and amelioration of salinity stress damage by symbiotic plant growth-promoting actinobacterium Glutamicibacter halophytocola KLBMP 5180. Appl. Environ. Microbiol. 84:e01533-18. doi: 10.1128/AEM.01533-1518
Qin, Y., Druzhinina, I. S., Pan, X., and Yuan, Z. (2016). Microbially mediated plant salt tolerance and microbiome-based solutions for saline agriculture. Biotechnol. Adv. 34, 1245–1259. doi: 10.1016/j.biotechadv.2016.08.005
Qurashi, A. W., and Sabri, A. N. (2012). Bacterial exopolysaccharide and biofilm formation stimulate chickpea growth and soil aggregation under salt stress. Braz. J. Microbiol. 43, 1183–1191. doi: 10.1590/S1517-838220120003000046
Ramadoss, D., Lakkineni, V. K., Bose, P., Ali, S., and Annapurna, K. (2013). Mitigation of salt stress in wheat seedlings by halotolerant bacteria isolated from saline habitats. SpringerPlus 2:6. doi: 10.1186/2193-1801-2-6
Rengasamy, P. (2006). World salinization with emphasis on Australia. J. Exp. Bot. 57, 1017–1023. doi: 10.1093/jxb/erj108
Rodriguez, P. A., Rothballer, M., Chowdhury, S. P., Nussbaumer, T., Gutjahr, C., and Falter-Braun, P. (2019). Systems biology of plant-microbiome interactions. Mol. Plant 12, 804–821. doi: 10.1016/j.molp.2019.05.006
Rodríguez-Salazar, J., Suárez, R., Caballero-Mellado, J., and Iturriaga, G. (2009). Trehalose accumulation in Azospirillum brasilense improves drought tolerance and biomass in maize plants. FEMS. Microbiol. Lett. 296, 52–59. doi: 10.1111/j.1574-6968.2009.01614.x
Rojas-Tapias, D., Moreno-Galván, A., Pardo-Díaz, S., Obando, M., Rivera, D., and Bonilla, R. (2012). Effect of inoculation with plant growth-promoting bacteria (PGPB) on amelioration of saline stress in maize (Zea mays). Appl. Soil Ecol. 61, 264–272. doi: 10.1016/j.apsoil.2012.01.006
Roy, D., and Hill, S. C. (2002). Bacterial osmoadaptation: the role of osmolytes in bacterial stress and virulence. FEMS. Microbiol. Rev. 26, 49–71. doi: 10.1111/j.1574-6976.2002.tb00598.x
Ruppel, S., Franken, P., and Witzel, K. (2013). Properties of the halophyte microbiome and their implications for plant salt tolerance. Funct. Plant Biol. 40, 940–951. doi: 10.1071/FP12355
Ryu, C. M., Farag, M. A., Hu, C. H., Reddy, M. S., Kloepper, J. W., and Pare, P. W. (2004). Bacterial volatiles induce systemic resistance in Arabidopsis. Plant. Physiol. 134, 1017–1026. doi: 10.1104/pp.103.026583
Saghafi, D., Delangiz, N., Lajayer, B. A., and Ghorbanpour, M. (2019). An overview on improvement of crop productivity in saline soils by halotolerant and halophilic PGPRs. 3 Biotech 9:261. doi: 10.1007/s13205-019-1799-0
Shahid, S. A., Zaman, M., and Heng, L. (2018). “Soil salinity: historical perspectives and a world overview of the problem,” in Guideline for Salinity Assessment, Mitigation and Adaptation Using Nuclear and Related Techniques, eds M. Zaman, S. A. Shahid, and L. Heng (Cham: Springer), 43–53. doi: 10.1007/978-3-319-96190-3_2
Sharifi, R., and Ryu, C. (2018). Revisiting bacterial volatile-mediated plant growth promotion: lessons from the past and objectives for the future. Ann. Bot. 122, 349–358. doi: 10.1093/aob/mcy108
Sharma, S., Kulkarni, J., and Jha, B. (2016). Halotolerant rhizobacteria promote growth and enhance salinity tolerance in peanut. Front. Microbiol. 7:1600. doi: 10.3389/fmicb.2016.01600
Singh, B. P., Rateb, M. E., Rodriguez-Couto, S., Polizeli, M. D. L. T. D. M., and Li, W. (2019). Editorial: microbial secondary metabolites: recent developments and technological challenges. Front. Microbiol. 10:914. doi: 10.3389/fmicb.2019.00914
Sleator, R. D., and Hill, C. (2002). Bacterial osmoadaptation: the role of osmolytes in bacterial stress and virulence. FEMS. Microbiol. Rev. 26, 49–71.
Stahl, P. D., and Parkin, T. B. (1996). Microbial production of volatile organic compounds in soil microcosms. Soil. Sci. Soc. Am. J. 60, 821–828. doi: 10.2136/sssaj1996.03615995006000030020x
Stringlis, I. A., Proietti, S., Hickman, R., Van, Verk, M. C., Zamioudis, C., et al. (2018). Root transcriptional dynamics induced by beneficial rhizobacteria and microbial immune elicitors reveal signatures of adaptation to mutualists. Plant J. 293, 166–180. doi: 10.1111/tpj.13741
Suárez, R., Wong, A., Ramírez, M., Barraza, A., Orozco Mdel, C., Cevallos, M. A., et al. (2008). Improvement of drought tolerance and grain yield in common bean by overexpressing trehalose-6-phosphate synthase in rhizobia. Mol. Plant. Microbe. Interact. 21, 958–966. doi: 10.1094/MPMI-21-7-0958
Sukweenadhi, J., Balusamy, S. R., Kim, Y. J., Lee, C. H., Kim, Y. J., Koh, S. C., et al. (2018). A growth-promoting bacteria, Paenibacillus yonginensis DCY84T enhanced salt stress tolerance by activating defense-related systems in Panax ginseng. Front. Plant. Sci. 9:813. doi: 10.3389/fpls.2018.00813
Sun, L., Lei, P., Wang, Q., Ma, J., Zhan, Y., Jiang, K., et al. (2020). The endophyte Pantoea alhagi NX-11 alleviates salt stress damage to rice seedlings by secreting exopolysaccharides. Front. Microbiol. 10:3112. doi: 10.3389/fmicb.2019.03112
Tahir, H. A. S., Gu, Q., Wu, H., Raza, W., Hanif, A., Wu, L., et al. (2017). Plant growth promotion by volatile organic compounds produced by Bacillus subtilis SYST2. Front. Microbiol. 8:171. doi: 10.3389/fmicb.2017.00171
Tewari, S., and Arora, N. K. (2016). Fluorescent Pseudomonas sp. PF17 as an efficient plant growth regulator and biocontrol agent for sunflower crop under saline conditions. Symbiosis 68, 99–108. doi: 10.1007/s13199-016-0389-8
Tewari, S., and Arora, N. K. (2018). Role of salicylic acid from Pseudomonas aeruginosa PF23 EPS+ in growth promotion of sunflower in saline soils infested with phytopathogen Macrophomina phaseolina. Environ. Sustain. 1, 49–59. doi: 10.1007/s42398-018-0002-6
Tewari, S., and Sharma, S. (2020). Rhizobial exopolysaccharides as supplement for enhancing nodulation and growth attributes of Cajanus cajan under multi-stress conditions: a study from lab to field. Soil Till. Res. 198:104545. doi: 10.1016/j.still.2019.104545
Tirranen, L. S., and Gitelson, I. I. (2006). The role of volatile metabolites in microbial communities of the LSS higher plant link. Adv. Space Res. 38, 1227–1232. doi: 10.1016/j.asr.2006.02.038
Upadhyay, S. K., and Singh, D. P. (2015). Effect of salt-tolerant plant growth-promoting rhizobacteria on wheat plants and soil health in a saline environment. Plant Biol. 17, 288–293. doi: 10.1111/plb.12173
Vaishnav, A., Kumari, S., Jain, S., Varma, A., and Choudhary, D. K. (2015). Putative bacterial volatile-mediated growth in soybean (Glycine max L. Merrill) and expression of induced proteins under salt stress. J. Appl. Microbiol. 119, 539–551. doi: 10.1111/jam.12866
Vaishnav, A., Kumari, S., Jain, S., Varma, A., Tuteja, N., and Choudhary, D. K. (2016). PGPR-mediated expression of salt tolerance gene in soybean through volatiles under sodium nitroprusside. J. Basic Microbiol. 56, 1274–1288. doi: 10.1002/jobm.201600188
Vejan, P., Abdullah, R., Khadiran, T., Ismail, S., and Nasrulhaq, B. A. (2016). Role of plant growth promoting rhizobacteria in agricultural sustainability - a review. Molecules 21:573. doi: 10.3390/molecules21050573
Vespermann, A., Kai, M., and Piechulla, B. (2007). Rhizobacterial volatiles affect the growth of fungi and Arabidopsis thaliana. Appl. Environ. Microbiol. 73, 5639–5641. doi: 10.1128/AEM.01078-07
Vílchez, J. I., García-Fontana, C., Román-Naranjo, D., González-López, J., and Manzanera, M. (2016). Plant drought tolerance enhancement by trehalose production of desiccation-tolerant microorganisms. Front. Microbiol. 7:1577. doi: 10.3389/fmicb.2016.01577
Wutipraditkul, N., Wongwean, P., and Buaboocha, T. (2015). Alleviation of salt-induced oxidative stress in rice seedlings by proline and/or glycine betaine. Biol. Plant. 59, 547–555. doi: 10.1007/s10535-015-0523-0
Xiong, Y., Gong, Y., Li, X., Chen, P., Ju, X., Zhang, C., et al. (2019). Enhancement of growth and salt tolerance of tomato seedlings by a natural halotolerant actinobacterium Glutamicibacter halophytocola KLBMP 5180 isolated from a coastal halophyte. Plant. Soil. 445, 307–322. doi: 10.1007/s11104-019-04310-8
Xiong, Y., Ju, X., Li, X., Gong, Y., Xu, M., Zhang, C., et al. (2020). Fermentation conditions optimization, purification, and antioxidant activity of exopolysaccharides obtained from the plant growth-promoting endophytic actinobacterium Glutamicibacter halophytocola KLBMP 5180. Int. J. Biol. Macromol. 153, 1176–1185. doi: 10.1016/j.ijbiomac.2019.10.247
Yang, A. Z., Akhtar, S. S., Iqbal, S., Amjad, M., Naveed, M., Zahir, Z. A., et al. (2016). Enhancing salt tolerance in quinoa by halotolerant bacterial inoculation. Funct. Plant Biol. 43, 632–642. doi: 10.1071/fp15265
Yasin, N. A., Akram, W., Khan, W. U., Ahmad, S. R., Ahmad, A., and Ali, A. (2018). Halotolerant plant-growth promoting rhizobacteria modulate gene expression and osmolyte production to improve salinity tolerance and growth in Capsicum annum L. Environ. Sci. Pollut. Res. 25, 23236–23250. doi: 10.1007/s11356-018-2381-8
Zerrouk, I. Z., Benchabane, M., Khelifi, L., Yokawa, K., Ludwig-Müller, J., and Baluska, F. (2016). A Pseudomonas strain isolated from date-palm rhizospheres improves root growth and promotes root formation in maize exposed to salt and aluminium stress. J. Plant Physiol. 191, 111–119. doi: 10.1016/j.jplph.2015.12.009
Zhang, H., Kim, M. S., Krishnamachari, V., Payton, P., Sun, Y., Grimson, M., et al. (2007). Rhizobacterial volatile emissions regulate auxin homeostasis and cell expansion in Arabidopsis. Planta 226, 839–851. doi: 10.1007/s00425-007-0530-2
Zhang, H., Kim, M.-S., Sun, Y., Dowd, S. E., Shi, H. Z., and Pare, P. W. (2008). Soil bacteria confer plant salt tolerance by tissue-specific regulation of the sodium transporter HKT1. Mol. Plant-Microbe Interact. 21, 737–744. doi: 10.1094/mpmi-21-6-0737
Keywords: salinity, sustainable agriculture, secondary metabolites, halotolerant PGPR, exopolysaccharides, bioinoculants
Citation: Sunita K, Mishra I, Mishra J, Prakash J and Arora NK (2020) Secondary Metabolites From Halotolerant Plant Growth Promoting Rhizobacteria for Ameliorating Salinity Stress in Plants. Front. Microbiol. 11:567768. doi: 10.3389/fmicb.2020.567768
Received: 02 June 2020; Accepted: 29 September 2020;
Published: 22 October 2020.
Edited by:
Dilantha Fernando, University of Manitoba, CanadaCopyright © 2020 Sunita, Mishra, Mishra, Prakash and Arora. This is an open-access article distributed under the terms of the Creative Commons Attribution License (CC BY). The use, distribution or reproduction in other forums is permitted, provided the original author(s) and the copyright owner(s) are credited and that the original publication in this journal is cited, in accordance with accepted academic practice. No use, distribution or reproduction is permitted which does not comply with these terms.
*Correspondence: Naveen Kumar Arora, bmthcm9yYS5iYmF1QGdtYWlsLmNvbQ==