- Department of Renewable Resources, University of Alberta, Edmonton, AB, Canada
Fungal volatile organic compounds (FVOCs) can act as intra- and inter-kingdom communication signals that influence the growth and behaviors of organisms involved in antagonistic or mutualistic relationships with fungi. There is growing evidence suggesting that FVOCs can mediate interactions between organisms within and across different ecological niches. Bark beetles have established mutualistic relationships with ophiostomatoid fungi which can serve as a food source and condition host plant tissues for developing beetle larvae. While the profiles (both composition and concentrations) of volatile emission from ophiostomatoid fungi can be influenced by abiotic factors, whether emissions from a given fungal species can be influenced by those from another is still unknown. Here, we analyzed FVOCs emitted from the two ophiostomatoid fungi, Grosmannia clavigera and Ophiostoma ips, associated with mountain pine beetle and pine engraver beetle, respectively, when each fungus was growing alone or in a shared headspace. We used two isolates of each fungus species. Overall, we detected a total of eight volatiles in both G. clavigera alone or in combination with O. ips including acetoin, ethyl acetate, cis-grandisol, isoamyl alcohol, isobutanol, 2-methyl-1-butanol, phenethyl acetate, and phenethyl alcohol. The profiles of volatiles emitted differed between the two fungal species but not between the two isolates of the same fungus. Six compounds were common between the species, whereas two compounds were detected only when G. clavigera was present. Moreover, the majority of volatiles were detected less frequently and at lower concentrations when the two fungi were grown together in a shared headspace. These results are likely due to reduced volatile emissions from O. ips in the presence of G. clavigera. However, changes in the profiles of fungal volatiles did not correspond with the observed changes in the growth of either species. Overall, these results suggest that the similarities in fungal volatiles among different species of fungi may reflect a common ecological niche and that the differences may correspond to species-specific adaptation to their respective host beetles or genetic factors.
Introduction
Ecological interactions between fungi and other organisms can be mediated by fungal volatile organic compounds (FVOCs) (Davis et al., 2013; Hung et al., 2015; Kandasamy et al., 2016, 2019; Schulz-Bohm et al., 2017). These compounds can act as intra- and inter-kingdom communication signals to influence the behaviors of animals involved in antagonisms or mutualisms with fungi (Schulz-Bohm et al., 2017; Schenkel et al., 2018; Zhao et al., 2019). Intra- and inter-specific interactions between fungi can also be mediated by FVOCs (Hofstetter et al., 2005; Cale et al., 2016; El Ariebi et al., 2016; Wang et al., 2020). For example, volatiles of Penicillium paneum can inhibit spore germination of conspecifics and other species of fungi, representing a variety of genera (Chitarra et al., 2004). Moreover, VOCs emitted by older cultures of some fungal species can stimulate or inhibit the growth and reproduction of cultures of other species (Cale et al., 2016). How the outcomes of such interactions could be indirectly influenced by factors that affect FVOC emissions is unknown.
Many factors affect the profiles of FVOCs (both composition and concentrations of individual compounds) emitted by a fungus (Hung et al., 2015). Fungal VOCs can be produced directly and indirectly from metabolic processes in fungal cells (Martín et al., 2014); thus, genetic factors likely underlie some of the intra- and inter-specific variation in FVOC emissions (Polizzi et al., 2012; Buśko et al., 2014). Emissions also can be influenced by the environment in which a fungus is growing. For instance, large variations in FVOCs can result from apparent interactions among abiotic factors such as temperature, humidity, and growth substrate (Polizzi et al., 2012). Likewise, VOCs originating from host plants and insects can influence emission of FVOCs (Cale et al., 2019). However, how ecological factors may affect the profiles of FVOC emitted is unknown. Understanding the semiochemical aspects of fungal ecology can help clarify the mechanisms underlying interactions between co-occurring species that occupy similar niches. This is especially important for understanding the ecology of economically important fungi, such as the species of ophiostomatoid fungi (Ophiostomataceae and Ceratocystidaceae) vectored by tree-killing bark beetle species (Coleoptera: Curculionidae, Scolytinae).
Several species of bark beetles have caused extensive forest mortality throughout the world (Seidl et al., 2008; Bentz et al., 2010). This mortality is in part due to infections of tree vascular tissues by mutualistic ophiostomatoid fungi vectored by the beetles (Six, 2013). The fungi can support beetles by serving as a nutritional supplement (i.e., nitrogen) and by conditioning tree tissue for developing larvae (Bleiker and Six, 2007; Six, 2013; Therrien et al., 2015; Ojeda-Alayon et al., 2017). The successful colonization of host trees by beetles can also be supported by the use of complex semiochemical systems derived from host tree, beetle and fungal cues, that help beetles coordinate the activities of conspecifics in overwhelming healthy trees that otherwise resist attack (Erbilgin, 2019). These semiochemical systems could potentially be supported in part by beetle mutualistic fungi, which can emit a wide array of FVOCs including some that influence beetle behavior (Hanssen, 1993; Hofstetter et al., 2005; Cale et al., 2016, 2019; Kandasamy et al., 2016; Zhao et al., 2019). Because multiple ophiostomatoid species often co-occur in a given tree (Roe et al., 2011), FVOC-mediated interactions may potentially occur among co-occurring fungi in nature. While the FVOCs from cultures of some fungi can inhibit the growth and reproduction of other fungal species (Hofstetter et al., 2005; Cale et al., 2016; Kandasamy et al., 2019), whether these effects are associated with changes to the profiles of FVOCs emitted by the recipient fungus is unknown. However, understanding such changes could help elucidate novel aspects of the ecology of bark beetle-ophiostomatoid fungus symbioses.
Large-scale outbreaks of the mountain pine beetle MPB, Dendroctonus ponderosae Hopkins have killed millions of hectares of mostly lodgepole pine (Pinus contorta Douglas var. latifolia Engelmann) forests in western North America (Bentz et al., 2010; Safranyik et al., 2010). Moreover, warmer temperatures have allowed MPB to expand their range eastward from lodgepole pine-dominated forests into jack pine (Pinus banksiana Lambert)-dominated forests (Cullingham et al., 2011; Erbilgin et al., 2014) where it is likely interact with another species of bark beetle, the pine engraver beetle (Ips pini Say) (Kegley et al., 1997). Co-occurrence of these two beetle species in lodgepole pine can negatively affect the number of emerging beetles of either species (Rankin and Borden, 1991), which may in part result from interactions between the ophiostomatoid fungal species commonly vectored by each of these beetle species. How interactions between Grosmannia clavigera (Robinson-Jeffrey and Davidson) Zipfel, de Beer, and Wing, the primary fungus vectored by MPB (Lee et al., 2005, 2006), and Ophiostoma ips (Schenk and Benjamin, 1969; Kopper et al., 2004), the primary fungus vectored by I. pini, influence either fungus is poorly understood. While variation in host plant substrate quality (e.g., amounts of host defense compounds and nutrients) can influence how interactions between G. clavigera and O. ips influence the growth of either fungus (Wang et al., 2020), how such interactions could affect FVOC production or be mediated by FVOCs is unknown. Clarifying such aspects of G. clavigera–O. ips interactions is important to understanding the development of the fungi in the jack pine trees and, thus, understanding the success and persistence of their associated host bark beetles in the conifer forests of the Western North America.
Here, we collected FVOCs from and conducted laboratory bioassays with two fungal species, G. clavigera and O. ips. Specifically, headspace FVOCs above separate cultures of G. clavigera and O. ips were identified, quantified, and compared among treatments where cultures occurred alone or in combination in a shared headspace, and tested for potential effects of FVOCs on fungal growth. This approach was used to address two related research questions: (i) does the quality and/or quantity of FVOCs emitted from an ophiostomatoid fungus change when the fungus is growing in the presence of FVOCs from a different ophiostomatoid species?, and (ii) does the presence of FVOC emissions affect the growth of another ophiostomatoid fungi?
Materials and Methods
Fungal Growth and Volatile Collections
The following experiments used the two isolates each for G. clavigera and O. ips that were reported in Cale et al. (2019) and Wang et al. (2020). For G. clavigera, both isolates (NOF2894 and NOF2896) were provided by the Northern Forestry Centre Culture Collection (Edmonton, Alberta) and were originally cultured from the phloem of MPB-infested lodgepole pine trees near Banff, Alberta (Rice et al., 2007). The O. ips isolates (NOF1205 and NOF1284) were isolated from bark beetle galleries in lodgepole pine (Cale et al., 2019). Master cultures of the four isolates were grown on potato dextrose agar (PDA) at 22°C in total darkness for 10 days. These cultures were subcultured onto PDA media in small Petri plates (60 mm diam.) at 22°C in total darkness for 4 days. These subcultures were used in the experiment described below. Throughout the paper, isolate refers different isolates of the same fungal species while species refer to the two fungal species (G. clavigera and O. ips).
Two-day old cultures of fungi were used in the following three treatment groups for the collection of headscape volatiles by air entrainment: (1) a culture of G. clavigera (Gc Control), (2) a culture of O. ips (Oi Control), and (3) separate cultures of G. clavigera and O. ips together (Combination). For the fungus-control treatments, a single 2-day old culture plate of the respective fungus was closed inside a volatile collection apparatus (see details under Fungal volatile collection apparatus subheading below). For the combination treatment, one culture plate from one species of fungus (either G. clavigera or O. ips) was placed 5–6 cm below a culture of the other fungus in the same chamber. The higher culture plate was held in place by a wire support. We randomly determined the placement of the fungus in the volatile collection apparatus to remove any bias. Since the air inside the apparatus was circulated, both fungi were likely equally affected by one another. Furthermore, Cale et al. (2016) showed that the vertical placement of fungal cultures inside the collection apparatus did not significantly affect fungal growth of volatile emissions. Lids of the culture plates were moved to cover only half of the plate area. Headspace volatiles from cultures in the control and combination treatments were then sampled for 48 h. Cultures of the two control treatments were each replicated ten times (N = 5 for each isolate). The combination treatment was replicated 12 times, representing three replicates from each of four isolate combinations (N = 3 for each isolate). Headspace volatiles were also collected from non-inoculated PDA plates (N = 10; PDA control) in order to help distinguish compounds emitted by fungi from those emitted by the media (data not shown); these compounds were removed from analysis. Total fungal growth (mm2) on each culture plate was measured by image analysis using ImageJ software (Abramoff et al., 2004). We used the same sampling apparatus to sample volatiles from each group of four treatments including the control. The mean (±SE) 4-day growth was calculated for cultures of each isolate (1845.7 ± 142.2 mm2 for NOF2894, 1905.0 ± 73.6 mm2 for NOF2969, 1397.7 ± 49.4 mm2 for NOF1284, and 1095.7 ± 28.3 mm2 for NOF1205).
Fungal Volatile Collection Apparatus
The volatile collection apparatus was similar to one reported in Cale et al. (2016) (Supplementary Figure S1). This was a closed-air system consisting of a 473 mL glass jar (collection chamber) whose threading was wrapped with Teflon tape and fitted with a metal cap fitted with two pairs of brass spigots (each pair fitted together by brass Swagelok) such that each pair consisted of a spigot extending above and below the cap surface. These spigots served as channels to allow constant airflow (475 mL min–1) using a flowmeter into and out of the collection chamber. The inlet channel was attached to 30.5 cm piece of Teflon tubing packed halfway down with activated carbon (800 mg; 6–14 mesh; held in place with glass wool) in order to purify air entering the collection chamber (air scrubber). This tubing was attached to a stainless-steel gang-valve connected to the outlet spigot of a bellows vacuum/pressure pump. A 15 cm section of Teflon tubing was attached to the outlet channel and attached to an adsorbant trap consisting of a 7.5 cm piece of Teflon tubing packed with 150 mg of activated carbon (held in place with glass wool). This trap collected culture headspace volatiles carried in the air stream as it flowed out of the collection chamber. The trap was attached to an eight cm long tube that joined the outlet channel to a gang-valve connected to the inlet spigot of the pump. A tight wrapping of Teflon tape was used at sites of tube and fitting connections in order to prevent outside air from entering and, thus, contaminating the system. The two gang-valves consisted of four spigots each, allowing us to connect to a set of four collection chambers/systems to each pump. Sample sets consisted of one replicate from each treatment plus a PDA control.
The sampling of headspace volatiles occurred over 48 h after culture/control plates were sealed in the collection chambers. The first 24 h represented a “charge period” that allowed headspace volatiles to accumulate in the jars before air started flowing through the system. The charge period was immediately followed by a 24 h “collection period” when the pumps were engaged and volatiles carried in an airstream were collected in the adsorbent traps. Pumps were then disengaged at the end of the collection period, and trap tubes were detached from the system, wrapped in labeled aluminum foil, and stored at −40°C prior to chemical extraction. Collection jars and caps were cleaned with acetone prior to each sampling set.
Chemical Analysis of FVOCs
Fungal VOCs were extracted from collection traps by placing the adsorbant into a 2 mL tube containing 1 mL of dichloromethane containing a tridecane as an internal standard (0.001%). The mixture was vortexed for 30 s, sonicated for 10 min, and centrifuged (18,213 rcf) at 0°C for 30 min. The extract was collected and transferred to a 2 mL gas chromatograph (GC) vial. This procedure was repeated a second time. The extracts were then analyzed using a GC (Agilent Tech., Santa Clara, CA, United States) fitted with a DB-5MS column (30 m length, 0.25 μm film, 0.25 mm I.D.; Agilent Tech., Santa Clara, CA, United States) and coupled to a mass spectrometer (GC-MS; GC: 7890A, MS: 5975C; Agilent Tech.). Helium was used as a carrier gas flowing at 1 mL min–1 with a temperature program beginning 50°C (held for 1 min) then increased by 5°C min–1 to 200°C, followed by an increase of 30°C min–1 to 325°C (held for 2 min). A 1 μL sample injection was used, and samples were run in splitless mode. Peaks present in the PDA controls were ignored when analyzing samples from the treatments. Peak identifications were confirmed using a NIST/EPA/NIH Mass Spectral library version 2.0f and analytical standards. The following standards were used: acetoin (≥96% chemical purity), ethyl acetate (≥99%), cis-grandisol [(1R,2S)-cis-2-isopropenyl-1-methylcyclobutaneethanol; ≥96%], isoamyl alcohol (≥98%), isobutanol (≥99%), 2-methyl-1-butanol (≥99%), phenethyl acetate (≥98%), and phenethyl alcohol (≥99%). All standards were purchased from Sigma-Aldrich (St. Louis, MO, United States) except cis-gransisol, which was purchased from Alpha Scents (West Linn, OR, United States). We quantified each individual compound using standard curves calculated from three serial dilutions of analytical standards. The internal standard (tridecane) was used to improve the precision of quantitative analysis with the calibration curve by plotting the signal from analyte with the signal from the internal standard as a function of the analyte concentration of the standards. The concentrations of the headspace volatiles detected were calculated as the amount of compound per unit culture area (as mean area of 4-day growth for a given isolate) per day (ng/mm2/day).
Fungal Growth Bioassays
The bioassays of fungal growth responses to FVOCs used new subcultures (on 60 mm PDA plates) of the same G. clavigera and O. ips isolates from which FVOCs were collected above. Bioassays were overall designed to expose a newly inoculated subculture (response culture) of a fungus to FVOCs emitted from 2- to 4-day old cultures (source cultures). The three plates were sealed together inside a glass jar (473 mL) such that the source culture plates were adjacent to each other on the bottom of the jar and the response culture was held 5–6 cm above them on a support of coiled wire. This approach was used to test the effects of source treatments on the growth of either G. clavigera or O. ips. Four source treatments were used: two plates of G. clavigera, two plates of O. ips one plate of each species of fungus (combination treatment), or two non-inoculated PDA plates as control. Isolates from each species of the fungus were randomly selected. The eight treatments were replicated ten times, with each isolate of a given fungus representing half of the replicates. Response cultures were grown in this manner for either three (G. clavigera) or four (O. ips) days to account for inherent inter-specific differences in growth rates. The total area (mm2) of response cultures was measured by image analysis using ImageJ software at this time (Abramoff et al., 2004).
Data Analysis
To test difference in the qualitative variation of fungal headspace VOC profiles (both composition and concentration of individual compounds) for statistical significance among collection treatments, matrices of the presence/absence of individual compounds (detection profiles) were constructed for each sample and analyzed using two-way permutational multivariate analysis of variance (PERMANOVA). This PERMANOVA tested the significance of main effects of isolate and treatment along with an effect of isolate-treatment interaction. Significant effects on the quality profiles were visualized using principle coordinate analysis (PCoA). To analyze differences in the quantitative variation of these VOCs, total VOC concentrations were calculated by summing the concentrations of each individual compound detected in a given sample. Total concentration differences among collection treatments were tested for statistical significance using two-way analysis of variance (ANOVA), which tested main effects of isolate and treatment and an isolate-treatment interaction effect. Pairwise Tukey Honest Significant Difference (HSD) tests were conducted following the identification of significant effects. Quantitative differences among collection treatments were also analyzed using profiles of individual compound concentrations. Two-way PERMANOVA was used to test statistical significance of isolate and treatment main effects as well as an isolate-treatment interaction, with significant effects being visualized using PCoA.
Fungal growth responses to VOC source treatments were analyzed separately for G. clavigera and O. ips response cultures. For each species, two-way ANOVA was used to test the statistical significance of source treatment and the isolate main effects of the responding fungus. Treatment-isolate interactions were also tested for significance. Pairwise comparisons were performed using Tukey HSD tests for significant effects.
Data were log-transformed to satisfy assumptions of normality and heteroscedasticity of the ANOVAs, as needed. All data analyses were conducted in the R software environment version 3.6.0 (R Core Team, 2019). All PERMANOVAs and PCoAs were calculated and tested using functions provided by the R package “vegan” version 2.5-5 (Oksanen et al., 2019).
Results
Fungal Volatile Responses
Eight compounds were detected from the headspace of G. clavigera and O. ips isolates growing either alone (fungal controls) or in the presence of the other species (combination): acetoin, ethyl acetate, cis-grandisol, isoamyl alcohol, isobutanol, 2-methyl-1-butanol, phenethyl acetate, and phenethyl alcohol (Table 1). However, the FVOC profiles of compounds varied among treatments, as the profiles were influenced by fungal species and whether the fungus was growing alone or in the presence of FVOCs of the other species. The FVOC profiles differed among the three treatments (PERMANOVA F(2,26) = 3.54, P = 0.003; Figure 1A), with the combination treatment having profiles similar to that of the G. clavigera control but different from the O. ips control. Profiles from these controls differed from each other. However, the fungal isolates (or isolate combinations) used did not influence FVOC emission as the isolate main effect and the isolate-treatment interaction effect were not significant. The inter-treatment patterns in FVOC profiles were likely due to variation in the total number of compounds detected and their detection frequencies (percentage of samples) among the treatments (Table 1). Similarly, all compounds detected from the combination treatment were detected at least once from either of the fungal controls, indicating that for a given fungus, the presence of the other fungus did not elicit emission of any novel compounds (Table 1). However, cis-grandisol and phenethyl acetate were not detected from the O. ips controls. The detection frequencies of all compounds other than isobutanol were lowest for the combination treatment (Table 1).
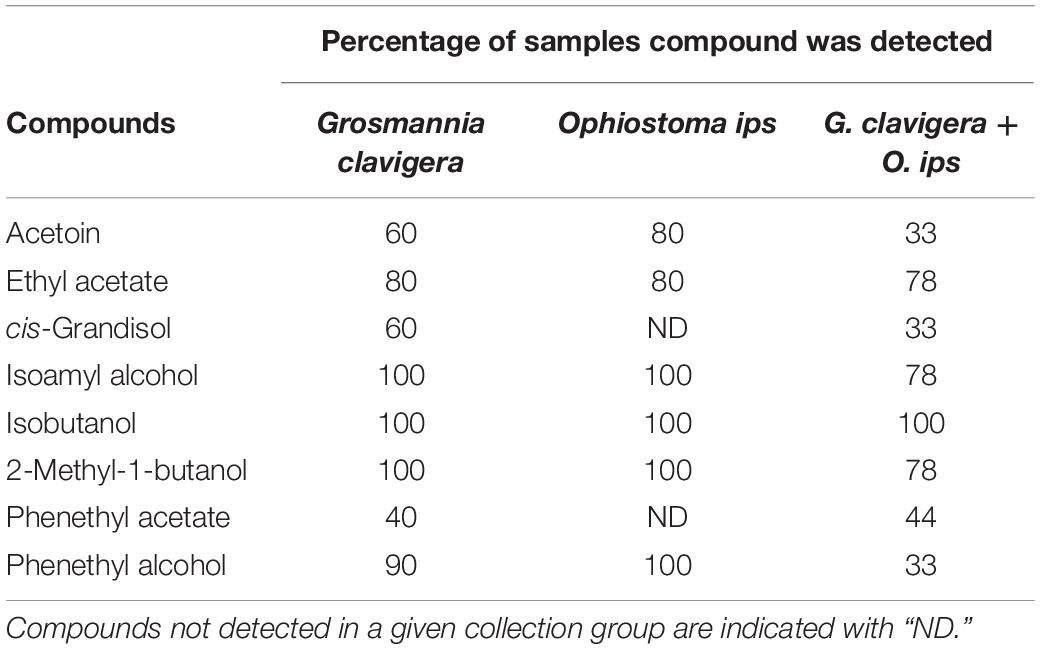
Table 1. Percentage of samples in which individual volatile organic compounds were detected from headspace collections of Grosmannia clavigera (N = 10), Ophiostoma ips (N = 10), and G. clavigera plus O. ips grown on potato dextrose agar (N = 9).
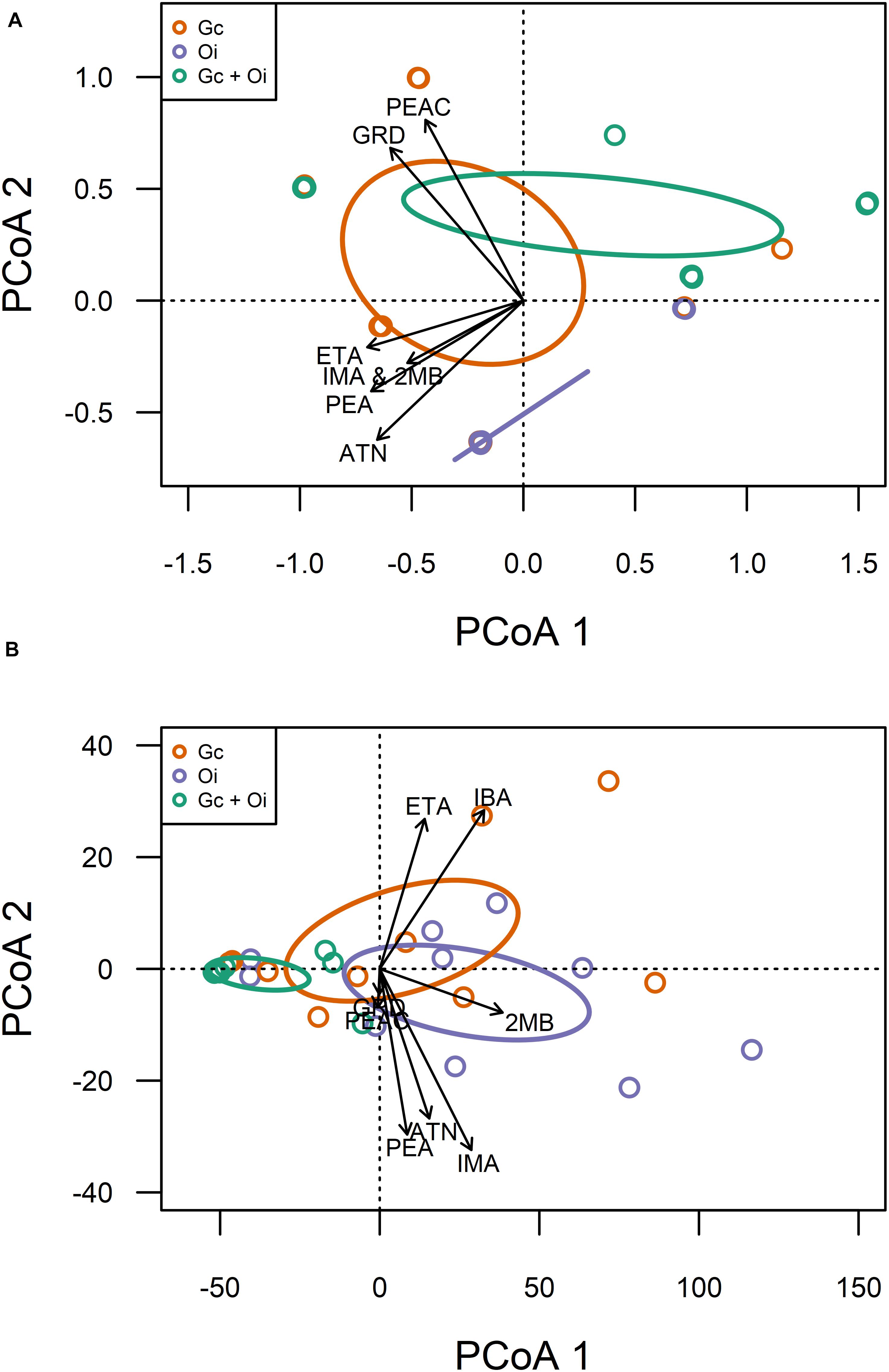
Figure 1. Qualitative (A) and quantitative (B) variation in volatile organic compound profiles detected from the headspace of Grosmannia clavigera (Gc; orange) cultures, Ophiostoma ips (Oi; purple) cultures, and these fungi together (Gc + Oi; green). Circles represent 95% confidence ellipses around cluster centroids. Acronyms for chemicals: ATN, Acetoin; IBA, Isobutanol; 2MB, 2-methyl-1-butanol; IMA, Isoamyl alcohol; PEA, Phenethyl alcohol; GRD, cis-Grandisol; ETA, Ethyl acetate; PEAC, Phenethyl acetate.
The headspace VOCs detected from G. clavigera and O. ips exhibited quantitative variation. The total concentrations of VOCs the fungi emitted responded to the treatments, as a significant main effect of treatment was detected (F(2,22) = 5.58, P = 0.011; Figure 2). Total FVOC concentrations from the combined treatment were 75 and 81% lower than those of the G. clavigera and O. ips controls, respectively. The fungal controls had similar concentrations of total FVOCs. Total FVOC concentrations did not vary by fungal isolate, as isolate main effects of isolate-treatment interactions were non-significant.
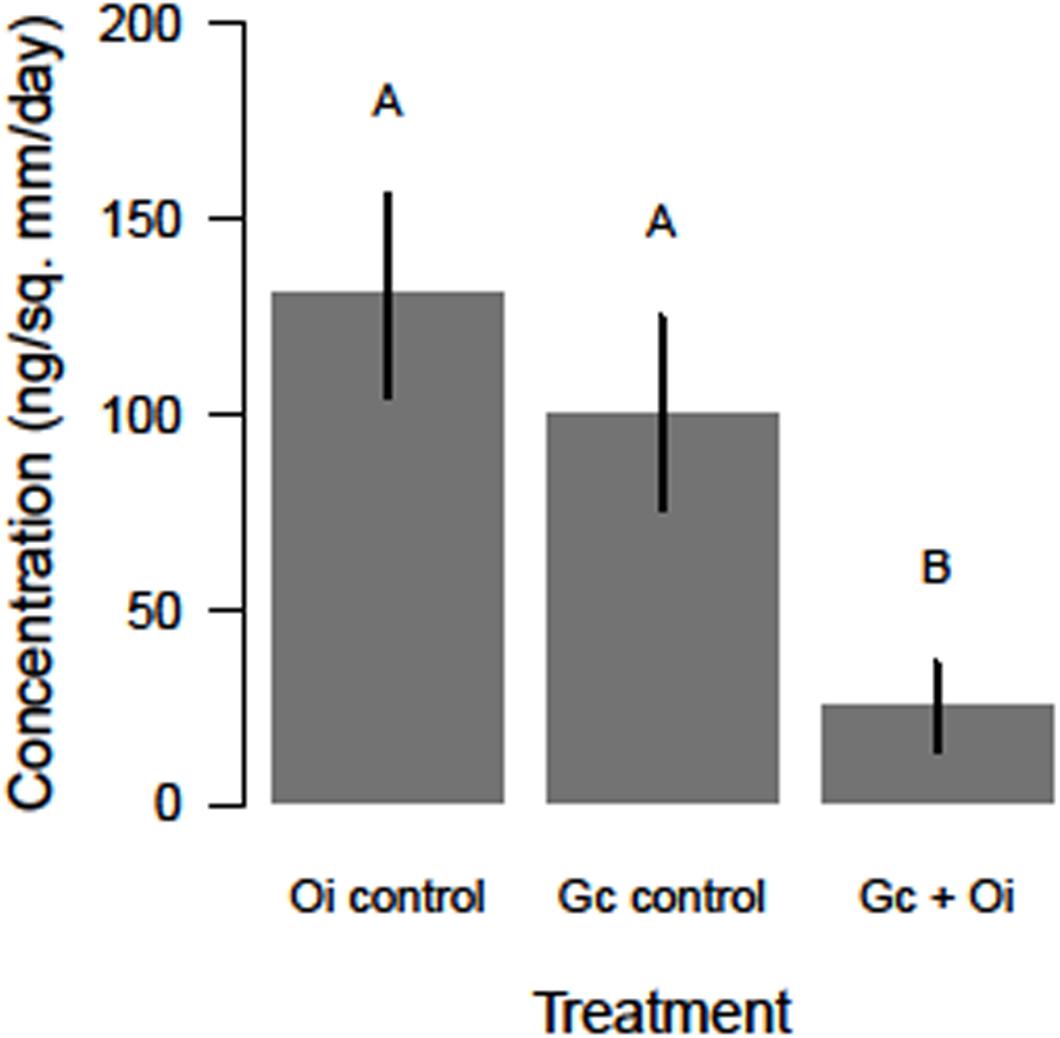
Figure 2. Differences in the total concentration (ng/mm2/day ± standard error) of volatile organic compounds detected in the headspace of Ophiostoma ips (Oi control) cultures, Grosmannia clavigera (Gc control) cultures, and these fungi together (Gc + Oi). Bars with different letter superscripts were different as indicated by Tukey’s Honest Significant Different tests.
Emission of individual FVOCs also significantly varied among treatments (PERMANOVA F(2,22) = 5.22, P = 0.011; Figure 1B), with the profiles of the combination treatment being significantly different from those of the O. ips control but similar to the G. clavigera control. Fungal isolate did not influence these profiles, as significant isolate main effects of isolate-treatment interactions were not detected. The lowest concentrations of individual FVOCs were consistently detected from the combination treatment, which had concentrations ranging from 92 to 34% lower than either of the fungal controls, depending on the compound (Table 2). However, the O. ips control tended to have the highest concentrations for most of the individual VOCs detected in that treatment.
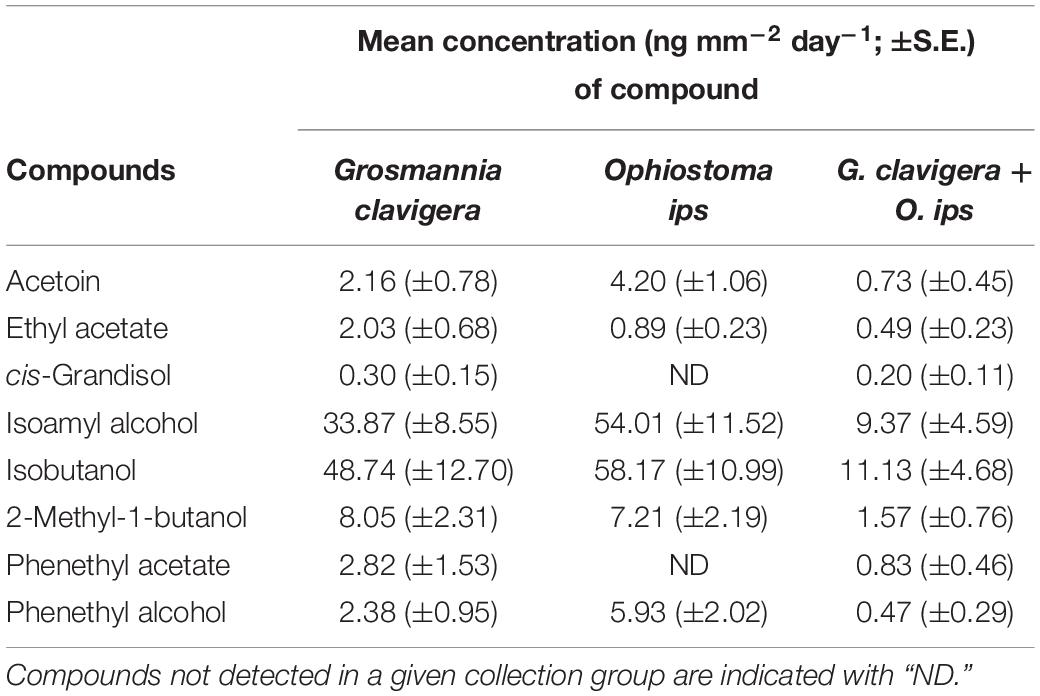
Table 2. Mean concentration of individual and total fungal volatile organic compounds detected from headspace collections of Grosmannia clavigera (N = 10), Ophiostoma ips (N = 10), and G. clavigera plus O. ips grown on potato dextrose agar (N = 9).
Fungal Growth Responses
The total growth (mm2) of G. clavigera and O. ips varied after the cultures were grown in the presence of VOCs from source fungal cultures, occurring either separately or in combination, or two PDA control plates (Figure 3).
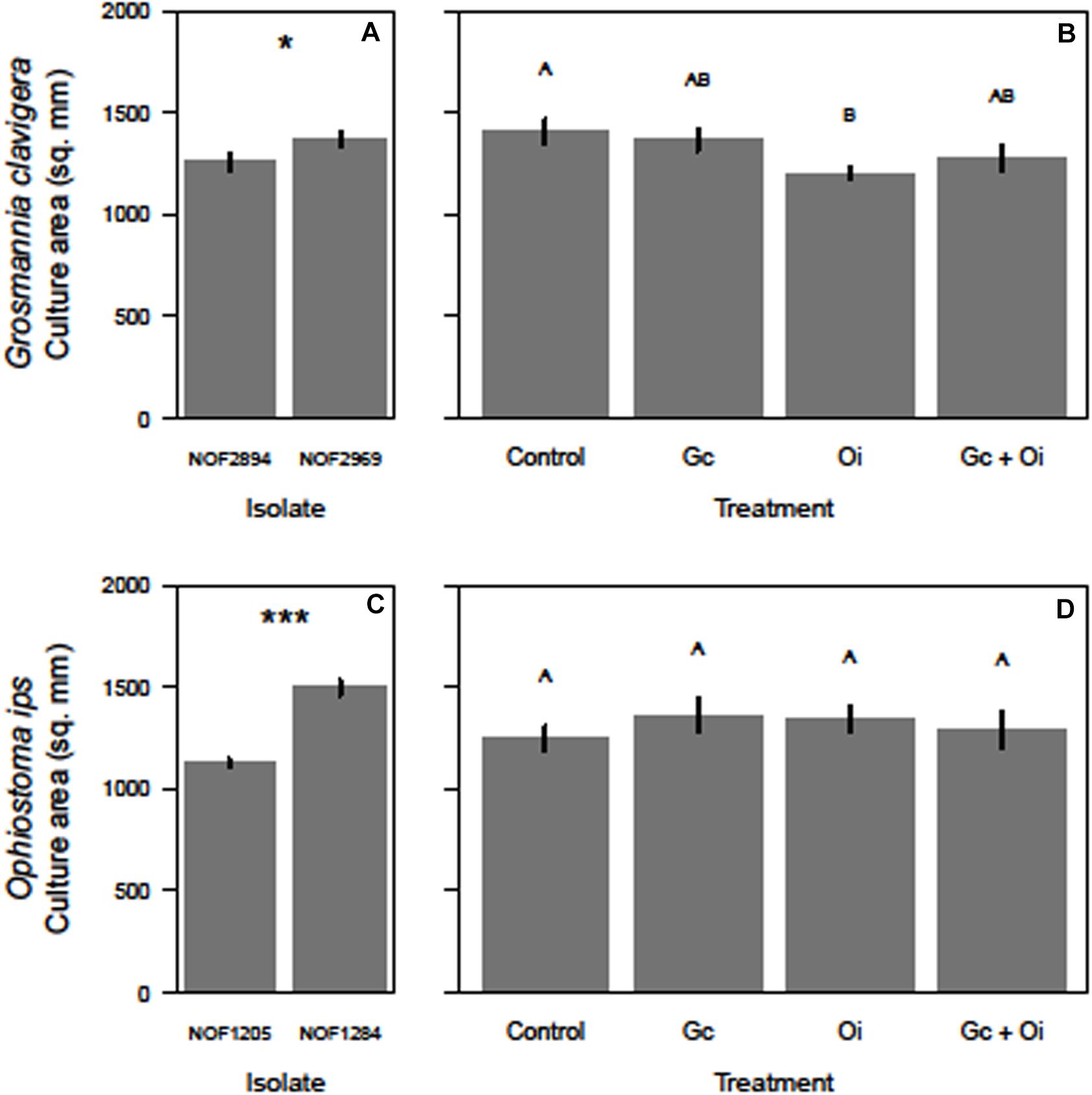
Figure 3. Differences in the growth (mm2) of the two isolates of Grosmannia clavigera (Gc) and Ophiostoma ips (Oi) when they grow alone (A,C, respectively) or in in a shared headspace environment (B,D, respectively). We used 4-day old cultures of each fungal species. Bars with different letter superscripts were different as indicated by Tukey’s Honest Significant Different tests. Statistical significance at P < 0.050 indicated by “*” and at P < 0.001 indicated by “***.”
For G. clavigera, the growth of response cultures significantly differed between the two isolates used (F(1,32) = 4.58, P = 0.040; Figure 3A), with the growth of isolate NOF2969 was slightly (8%) larger than isolate NOF2894 after 3 days, independent of emission treatment (Figure 3A). However, the isolate of G. clavigera grown did not influence their response to the emission treatments, as there was no significant treatment-isolate interaction effect. The growth of response cultures was influenced by the emission treatments as a significant treatment main effect was detected (F(3,32) = 3.43, P = 0.028; Figure 3B). Pairwise comparisons indicated that G. clavigera grew 15% less when exposed to VOCs of O. ips compared to when growing with controls (Figure 3B). However, G. clavigera growth was not influenced by VOCs from the G. clavigera or combination emission treatments (Figure 3B).
For O. ips, the growth of response cultures significantly differed between isolates (F(1,32) = 104.38, P < 0.001), with the growth of isolate NOF1284 being 33% larger than that of NOF1205 (Figure 3C) after 4 days. However, the growth of O. ips was not influenced by the emission treatments (Figure 3D), as the main effect of treatment was not significant. The isolate-treatment interaction for O. ips response cultures was non-significant.
Discussion
Fungal VOCs emitted by one ophiostomatoid fungus can be affected by the headspace of FVOCs from another species. The FVOC profiles from the combination treatment (G. clavigera and O. ips together) differed from those of the O. ips alone treatment but not from those of the G. clavigera alone. The contribution of G. clavigera to the FVOC profiles of the combination treatment does not necessarily explain the difference between profiles of the O. ips control and the combination treatments, as the incidence of detection and the concentrations of several compounds (e.g., acetoin, isoamyl alcohol, and phenethyl alcohol) in the combination treatment were lower than those in the O. ips control. Taken together, these findings suggest that the FVOCs emitted from some ophiostomatoid fungi can alter FVOC profiles of other fungal species. These results complement to those observed in other systems, where changes in FVOC profiles were elicited by physical (mycelial) interactions among multiple species of fungi sharing a niche (Hynes et al., 2007; El Ariebi et al., 2016). However, our findings suggest that such effects could also result from FVOC-mediated interactions between fungal species without physical contact. Currently, the mechanism underlying such inter-fungal interactions compare to those mediated by water soluble chemical signals secreted by fungi growing in close proximity is unknown (Hofstetter et al., 2005; Schmidt et al., 2015).
While FVOC profiles changed as a result of growing both G. clavigera and O. ips in a shared headspace environment, these changes did not influence the growth of interacting fungi. However, the growth of ophiostomatoid species can respond to FVOCs from other ophiostomatoid fungi, with the outcome of such treatments varying with the species of fungus. Hofstetter et al. (2005) showed that the growth rate of Ophiostoma minus was reduced by the volatiles emitted from actively growing Entomocorticium sp. A and Ophiostoma ranaculosum in the southern pine beetle (Dendroctonus frontalis) system. However, in the same study another fungus tested Leptographium terebrantis had no effect on O. minus growth rate. Similarly, Cale et al. (2016) showed that the outcomes of interactions among three ophiostomatoid fungi vectored by MPB can result in either no-effect or inhibition of fungal growth in response to their FVOCs. In particular, G. clavigera cultures growing in the headspace of FVOCs from cultures of Ophiostoma montium were 50% smaller than controls but the fungus Leptographium longiclavatum did not respond to FVOCs from G. clavigera.
The FVOC profiles emitted differed between G. clavigera and O. ips. While six compounds were common between species, two compounds were detected only when G. clavigera grew alone. The six FVOCs (acetoin, ethyl acetate, isoamyl acetate, isobutanol, 2-methyl-1-butanol, and phenethyl alcohol) have also been detected in headspace of several ophiostomatoid fungal species, likely because they are byproducts of primary metabolism during vegetative growth (Kandasamy et al., 2016; Cale et al., 2019). Furthermore, the similarities in FVOCs among different ophiostomatoid fungal species may also reflect a common ecological niche as fungi with a common ecological niche share similar FVOC profiles (Müller et al., 2013). While only detected from the headspace of G. clavigera, here, phenethyl acetate has been detected in FVOC profiles of some other ophiostomatoid fungi (e.g., L. longiclavatum, Endoconidiophora polonica) (Cale et al., 2016, 2019; Kandasamy et al., 2016). However, among ophiostomatoid fungi, cis-grandisol has only been reported from the FVOC profiles of G. clavigera (Cale et al., 2016, 2019), suggesting species-specific production of FVOCs.
There are a number of studies reported the ecological roles of many FVOCs including those ophiostomatoid species identified in this study, in other organisms including fungi, bark beetles, and other insect species (Davis et al., 2013; Kandasamy et al., 2016; Cale et al., 2019). Thus, we here will keep our discussion on the ecological roles of FOVs identified in this study short. Phenethyl alcohol can inhibit attraction of MPB and D. frontalis (Renwick et al., 1976; Pureswaran et al., 2000; Pureswaran and Borden, 2004; Sullivan et al., 2007). Here, O. ips emitted more phenylethyl alcohol than G. clavigera, suggesting that trees attacked by I. pini vectoring O. ips may be less attractive to MPB because of strong phenethyl alcohol emissions from the fungus. Such behavioral effects may reduce competition between MPB and I. pini. Conversely, isoamyl alcohol is emitted by a number of ophiostomatoid fungal species and attractive to D. frontalis (Brand et al., 1977). Although the behavioral responses of MPB and I. pini to isoamyl alcohol are unknown, the emission of this compound by O. ips and G. clavigera may suggest it plays similar roles in the chemical ecology of both beetles. 2-methyl-1-butanol is a common FVOC that attracts a wide diversity of insect species, representing several phyla (Davis et al., 2013; Zhao et al., 2015; Cale et al., 2016). Although only detected from G. clavigera, among reported FVOC surveys of ophiostomatoid fungi, cis-grandisol (grandlure I) is an important aggregation pheromone of several weevil species (Tewari et al., 2014).
Individual FVOCs may be accurately sampled using different methodologies. While, we collected FVOCs using an air entrainment approach, our recent study with the same fungal isolates extracted FVOCs from filtrate from liquid cultures growing in potato dextrose broth (Cale et al., 2019). Each of the eight compounds detected in the current study were also detected in the liquid culture. Furthermore, in both studies, phenethyl alcohol, isoamyl alcohol, 2-methyl-1-butanol, and isobutanol were determined to be dominant components of FVOCs, being detected in at least 90% of samples and were major components of total FVOC concentrations from G. clavigera or O. ips isolates growing alone. Similarly, the same individual FVOCs were determined, here, and by Cale et al. (2019) to be minor components of the FVOCs of the same fungi here. Specifically, acetoin was the compound least frequently detected and represented a small percentage of total FVOC concentrations. Furthermore, cis-grandisol and phenethyl acetate were detected only from cultures of G. clavigera, here and by Cale et al. (2019). However, it should be noted that the chemistry of fungal growth media can influence FVOC profiles, and should be chosen carefully when designing studies of FVOCs (Cale et al., 2019).
Caveats are an inherent part of conducting in vitro bioassays investigating fundamental aspects of ecological interactions. Several such caveats should be considered with the study, here. First, the two isolates of G. clavigera and O. ips used here differed in growth rate, which may not accurately reflect the range of growth rates of either species exhibit in nature or culture. However, this may not influence how representative the FVOCs detected are of the species as profiles of both isolates were qualitatively and quantitatively similar when standardized by culture area. Furthermore, such similarities in FVOC profiles may be further representative of a particular ecological function/niche (Müller et al., 2013). Second, FVOCs were collected from culture growing on artificial growth media. Substrate chemistry can influence FVOCs from at least ophiostomatoid fungi (Cale et al., 2019), and the FVOCs profiles determined here may differ from those emitted by the fungi growing on their natural substrate of pine vascular tissue. However, the use of artificial media is integral in fungal ecology as growing conditions of cryptic species either cannot be replicated under laboratory conditions or cannot be designed using natural substrates in a manner that affords a mechanistic understanding of the interactions under study (Crowther et al., 2018). For studies of FVOCs from fungal cultures on any growth media, sampling FVOCs from a non-inoculated control is critical to distinguishing between fungus- and media-emitted compounds. Lastly, in nature, G. clavigera and O. montium would interact under pine bark, an anaerobic environment when intact that is rich with plant primary and secondary metabolites (Hofstetter et al., 2005). The outcome of inter-fungal interactions in such an environment may differ from those shown here as substrate chemistry can at least affect the FVOC emissions from these fungi (Cale et al., 2019).
Data Availability Statement
The raw data supporting the conclusions of this article will be made available by the authors, without undue reservation.
Author Contributions
FW, JAC, and NE conceived the research project and wrote the manuscript. FW designed and performed the studies. JAC and AH assisted in running chemical analyses. JAC helped FW data analyses. All authors provided editorial advice.
Funding
Funding for this research was provided by the Natural Sciences and Engineering Research Council of Canada-Discovery Award to NE.
Conflict of Interest
The authors declare that the research was conducted in the absence of any commercial or financial relationships that could be construed as a potential conflict of interest.
Acknowledgments
We thank Guncha Ishangulyyeva for laboratory assistance and Rahmatollah Rajabzadeh for assistance with GC-MS analysis.
Supplementary Material
The Supplementary Material for this article can be found online at: https://www.frontiersin.org/articles/10.3389/fmicb.2020.567462/full#supplementary-material
FIGURE S1 | Fungal volatile collection apparatus. This is a closed-air system consisting of a 473 mL glass jar fitted with a metal cap fitted with two pairs of brass spigots. Spigots serve as channels to allow constant airflow (475 mL min–1) into and out of the glass jar. The inlet channel is attached to 30.5 cm piece of Teflon tubing packed halfway down with activated carbon (800 mg; 6–14 mesh; held in place with glass wool) in order to purify air entering the glass jar. This tubing is attached to a stainless-steel gang-valve connected to the outlet spigot of a bellows vacuum/pressure pump. A 15 cm section of Teflon tubing is attached to the outlet channel and attached to an adsorbant trap consisting of a 7.5 cm piece of Teflon tubing packed with 150 mg of activated carbon (held in place with glass wool). This trap collects culture headspace volatiles carried in the air stream as it flows out of the collection chamber. The trap is attached to 8 cm long tube that joins the outlet channel to a gang-valve connected to the inlet spigot of the pump. A tight wrapping of Teflon tape is used at sites of tube and fitting connections in order to prevent outside air from entering and, thus, contaminating the system.
References
Abramoff, M. D., Magalhaes, P. J., and Ram, S. J. (2004). Image processing with ImageJ. Biophotonics Int. 11, 36–42.
Bentz, B. J., Regnière, J., Fettig, C. J., Hansen, E. M., Hayes, J. L., Hicke, J. A., et al. (2010). Climate change and bark beetles of the western United States and Canada: direct and indirect effects. BioScience 60, 602–613. doi: 10.1525/bio.2010.60.8.6
Bleiker, K. P., and Six, D. L. (2007). Dietary benefits of fungal associates to an eruptive herbivore: potential implications of multiple associates on host population dynamics. Environ. Entomol. 36, 1384–1396. doi: 10.1093/ee/36.6.1384
Brand, J. M., Schultz, J., Barras, S. J., Edson, L. J., Payne, T. L., and Hedden, R. L. (1977). Bark-beetle pheromones: enhancement of Dendroctonus frontalis (Coleoptera: Scolytidae) aggregation pheromones by yeast metabolites in laboratory bioassays. J. Chem. Ecol. 3, 657–666. doi: 10.1007/BF00988065
Buśko, M., Kulik, T., Ostrowska, A., Góral, T., and Perkowski, J. (2014). Quantitative volatile compound profiles in fungal cultures of three different Fusarium graminearum chemotypes. FEMS Microbiol. Lett. 359, 85–93. doi: 10.1111/1574-6968.12569
Cale, J. A., Collignon, R. M., Klutsch, J. G., Kanekar, S. S., Hussain, A., and Erbilgin, N. (2016). Fungal volatiles can act as carbon sources and semiochemicals to mediate interspecific interactions among bark beetle-associated fungal symbionts. PLoS One 11:e0162197. doi: 10.1371/journal.pone.0162197
Cale, J. A., Ding, R., Wang, F., Rajabzadeh, R., and Erbilgin, N. (2019). Ophiostomatoid fungi can emit the bark beetle pheromone verbenone and other semiochemicals in media amended with various pine chemicals and beetle-released compounds. Fungal Ecol. 39, 285–295. doi: 10.1016/j.funeco.2019.01.003
Chitarra, G. S., Abee, T., Rombouts, F. M., Posthumus, M. A., and Dijksterhuis, J. (2004). Germination of Penicillium paneum conidia is regulated by 1-octen-3-ol, a volatile self-inhibitor. Appl. Environ. Microbiol. 70, 2823–2829. doi: 10.1128/AEM.70.5.2823-2829.2004
Crowther, T. W., Boddy, L., and Maynard, D. S. (2018). The use of artificial media in fungal ecology. Fun. Ecol. 32, 87–91. doi: 10.1016/j.funeco.2017.10.007
Cullingham, C., Cooke, J., Dang, S., Davis, C., Cooke, B., and Coltman, D. (2011). Mountain pine beetle host-range expansion threatens the boreal forest. Mol. Ecol. 20, 2157–2171. doi: 10.1111/j.1365-294x.2011.05086.x
Davis, T. S., Crippen, T. L., Hofstetter, R. W., and Tomberlin, J. K. (2013). Microbial volatile emissions as insect semiochemicals. J. Chem. Ecol. 39, 840–859. doi: 10.1007/s10886-013-0306-z
El Ariebi, N., Hiscox, J., Scriven, S. A., Müller, C. T., and Boddy, L. (2016). Production and effects of volatile organic compounds during interspecific interactions. Fungal Ecol. 20, 144–154. doi: 10.1016/j.funeco.2015.12.013
Erbilgin, N. (2019). Phytochemicals as mediators for host range expansion of a native invasive forest insect herbivore. New Phytol. 221, 1268–1278. doi: 10.1111/nph.15467
Erbilgin, N., Ma, C., Whitehouse, C., Shan, B., Najar, A., and Evenden, M. (2014). Chemical similarity between historical and novel host plants promotes range and host expansion of the mountain pine beetle in a naïve host ecosystem. New Phytol. 201, 940–950. doi: 10.1111/nph.12573
Hanssen, H. (1993). “Volatile metabolites produced by species of Ophiostoma and Ceratocystis,” in Ceratocystis and Ophiostoma: Taxonomy, Ecology, and Pathology, eds M. Wingfield, K. Seifert, and J. Webber (St. Paul: APS Press), 117–125.
Hofstetter, R. W., Mahfouz, J. B., Klepzig, K. D., and Ayres, M. P. (2005). Effects of tree phytochemistry on the interactions among endophloedic fungi associated with the southern pine eetle. J. Chem. Ecol. 31, 539–560. doi: 10.1007/s10886-005-2035-4
Hung, R., Lee, S., and Bennett, J. W. (2015). Fungal volatile organic compounds and their role in ecosystems. Appl. Microbiol. Biotechnol. 99, 3395–3405. doi: 10.1007/s00253-015-6494-4
Hynes, J., Müller, C. T., Jones, T. H., and Boddy, L. (2007). Changes in volatile production during the course of fungal mycelial interactions between Hypholoma fasciculare and Resinicium bicolor. J. Chem. Ecol. 33, 43–57. doi: 10.1007/s10886-006-9209-6
Kandasamy, D., Gershenzon, J., Andersson, M. N., and Hammerbacher, A. (2019). Volatile organic compounds influence the interaction of the eurasian spruce bark beetle (Ips typographus) with its fungal symbionts. ISME J. 13, 1788–1800. doi: 10.1038/s41396-019-0390-3
Kandasamy, D., Gershenzon, J., and Hammerbacher, A. (2016). Volatile organic compounds emitted by fungal associates of conifer bark beetles and their potential in bark beetle control. J. Chem. Ecol. 42, 952–969. doi: 10.1007/s10886-016-0768-x
Kegley, S., Livingston, R., and Gibson, K. (1997). Pine engraver, Ips pini (Say), in the western United States. USDA For. Serv. For. Insect Dis. Leaflet 122:5.
Kopper, B., Klepzig, K., and Raffa, K. (2004). Components of antagonism and mutualism in Ips pini–fungal interactions: relationship to a life history of colonizing highly stressed and dead trees. Environ. Entomol. 33, 28–34. doi: 10.1603/0046-225x-33.1.28
Lee, S., Kim, J. J., and Breuil, C. (2005). Leptographium longiclavatum sp. nov., a new species associated with the mountain pine beetle, Dendroctonus ponderosae. Mycol. Res. 109, 1162–1170. doi: 10.1017/s0953756205003588
Lee, S., Kim, J. J., and Breuil, C. (2006). Diversity of fungi associated with the mountain pine beetle, Dendroctonus ponderosae and infested lodgepole pines in British Columbia. Fungal Divers. 22, 91–105.
Martín, J.-F., García-Estrada, C., and Zeilinger, S. (eds) (2014). Biosynthesis and Molecular Genetics of Fungal Secondary Metabolites. New York, NY: Springer.
Müller, A., Faubert, P., Hagen, M., zu Castell, W., Polle, A., Schnitzler, J., et al. (2013). Volatile profiles of fungi – chemotyping of species and ecological functions. Fungal Gen. Biol. 54, 25–33. doi: 10.1016/j.fgb.2013.02.005
Ojeda-Alayon, D. I., Tsui, C. K. M., Feau, N., Capron, A., Dhillon, B., Zhang, Y., et al. (2017). Genetic and genomic evidence of niche partitioning and adaptive radiation in mountain pine beetle fungal symbionts. Mol. Ecol. 26, 2077–2091. doi: 10.1111/mec.14074
Oksanen, J., Guillaume Blanchet, F., Friendly, M., Kindt, R., Legendre, P., McGlinn, D., et al. (2019). Vegan: Community Ecology Package. R Package Version 2.5-5.
Polizzi, V., Adams, A., Malysheva, S. V., De Saeger, S., van Peteghem, C., Moretti, A., et al. (2012). Identification of volatile markers for indoor fungal growth and chemotaxonomic classification of Aspergillus species. Fungal Biol. 116Ti, 941–953. doi: 10.1016/j.funbio.2012.06.001
Pureswaran, D., and Borden, J. (2004). New repellent semiochemicals for three species of Dendroctonus (Coleoptera: Scolytidae). Chemoecology 14, 67–75. doi: 10.1007/s00049-003-0260-2
Pureswaran, D. S., Gries, R., Borden, J. H., and Pierce, H. D. Jr. (2000). Dynamics of pheromone production and communication in the mountain pine beetle, Dendroctonus ponderosae Hopkins, and the pine engraver, Ips pini (Say) (Coleoptera: Scolytidae). Chemoecology 10:153e168.
R Core Team (2019). R: A Language and Environment for Statistical Computing. Vienna: R Foundation for Statistical Computing.
Rankin, L. J., and Borden, J. H. (1991). Competitive interactions between the mountain pine beetle and the pine engraver in lodgepole pine. Can. J. For. Res. 21, 1029–1036. doi: 10.1139/x91-141
Renwick, J., Hughes, P., Pitman, G., and Vité, J. (1976). Oxidation products of terpenes identified from Dendroctonus and Ips bark beetles. J. Insect Physiol. 22, 725–727. doi: 10.1016/0022-1910(76)90238-9
Rice, A. V., Thormann, M. N., and Langor, D. W. (2007). Mountain pine beetle associated blue-stain fungi cause lesions on jack pine, lodgepole pine, and lodgepole x jack pine hybrids in Alberta. Can. J. Bot. 85, 307–315. doi: 10.1139/b07-014
Roe, A. D., Rice, A. V., Coltman, D. W., Cooke, J. E. K., and Sperling, F. A. H. (2011). Comparative phylogeography, genetic differentiation and contrasting reproductive modes in three fungal symbionts of a multipartite bark beetle symbiosis. Mol. Ecol. 20, 584–600. doi: 10.1111/j.1365-294X.2010.04953.x
Safranyik, L., Carroll, A. L., Regniere, J., Langor, D. W., Riel, W. G., Shore, T. L., et al. (2010). Potential for range expansion of mountain pine beetle into the boreal forest of North America. Can. Entomol. 142, 415–442.
Schenk, J., and Benjamin, D. (1969). Notes on the biology of Ips pini in central Wisconsin jack pine forests. Ann. Entomol. Soc. Ame. 62, 480–485. doi: 10.1093/aesa/62.3.480
Schenkel, D., Maciá-Vicente, J. G., Bissell, A., and Splivallo, R. (2018). Fungi indirectly affect plant root architecture by modulating soil volatile organic compounds. Front. Microbiol. 9:1847. doi: 10.3389/fmicb.2018.01847
Schmidt, R., Cordovez, V., de Boer, W., Raaijmakers, J., and Garbeva, P. (2015). Volatile affairs in microbial interactions. ISME J. 9, 2329–2335. doi: 10.1038/ismej.2015.42
Schulz-Bohm, K., Martín-Sánchez, L., and Garbeva, P. (2017). Microbial volatiles: small molecules with an important role in intra- and inter-kingdom interactions. Front. Microbiol. 8:2484. doi: 10.3389/fmicb.2017.02484
Seidl, R., Rammer, W., Jager, D., and Lexer, M. J. (2008). Impact of bark beetle (Ips typographus L.) disturbance on timber production and carbon sequestration in different management strategies under climate change. For. Ecol. Manage. 256, 209–220. doi: 10.1016/j.foreco.2008.04.002
Six, D. L. (2013). The bark beetle holobiont: why microbes matter. J. Chem. Ecol. 39, 989–1002. doi: 10.1007/s10886-013-0318-8
Sullivan, B. T., Dalusky, M. J., Wakarchuk, D., and Berisford, C. W. (2007). Field evaluations of potential aggregation inhibitors for the southern pine beetle, Dendroctonus frontalis (Coleoptera: Curculionidae). J. Entomol. Sci. 42:139e149.
Tewari, S., Leskey, T., Nielsen, A., Pinero, J., and Rodriguez-Saona, C. (2014). Integrated pest management. Current concepts and Ecological Perspective. San Diego: Academic Press.
Therrien, J., Mason, C. J., Cale, J. A., Adams, A., Aukema, B. H., Currie, C. R., et al. (2015). Bacteria influence mountain pine beetle brood development through interactions with symbiotic and antagonistic fungi: implications for climate-driven host range expansion. Oecologia 179, 467–485. doi: 10.1007/s00442-015-3356-9
Wang, F., Cale, J. A., and Erbilgin, N. (2020). Induced defenses of a novel host tree affect the growth and interactions of bark beetle-vectored fungi. Microb. Ecol. 80, 181–190. doi: 10.1007/s00248-020-01490-0
Zhao, T., Axelsson, K., Krokene, P., and Borg-Karlson, A.-K. (2015). Fungal symbionts of the spruce bark beetle synthesize the beetle aggregation pheromone 2-methyl-3-buten-2-ol. J. Chem. Ecol. 41, 848–852. doi: 10.1007/s10886-015-0617-3
Keywords: fungal chemical ecology, insect-fungus interactions, bark beetles, mountain pine beetle, pine engraver beetle
Citation: Wang F, Cale JA, Hussain A and Erbilgin N (2020) Exposure to Fungal Volatiles Can Influence Volatile Emissions From Other Ophiostomatoid Fungi. Front. Microbiol. 11:567462. doi: 10.3389/fmicb.2020.567462
Received: 01 June 2020; Accepted: 31 August 2020;
Published: 17 September 2020.
Edited by:
Isabel Diaz, Polytechnic University of Madrid, SpainReviewed by:
Emile Gluck-Thaler, University of Pennsylvania, United StatesManuel Martinez, Polytechnic University of Madrid, Spain
Copyright © 2020 Wang, Cale, Hussain and Erbilgin. This is an open-access article distributed under the terms of the Creative Commons Attribution License (CC BY). The use, distribution or reproduction in other forums is permitted, provided the original author(s) and the copyright owner(s) are credited and that the original publication in this journal is cited, in accordance with accepted academic practice. No use, distribution or reproduction is permitted which does not comply with these terms.
*Correspondence: Nadir Erbilgin, ZXJiaWxnaW5AdWFsYmVydGEuY2E=
†These authors have contributed equally to this work
‡ORCID: Jonathan A. Cale, orcid.org/0000-0002-8902-2032; Altaf Hussain, orcid.org/0000-0003-0076-0483; Nadir Erbilgin, orcid.org/0000-0001-9912-8095