- 1Master of Science Program in Applied Microbiology, Faculty of Science, Chiang Mai University, Chiang Mai, Thailand
- 2Department of Biology, Faculty of Science, Chiang Mai University, Chiang Mai, Thailand
- 3Department of Biochemistry and Microbiology, Faculty of Pharmaceutical Sciences, Chulalongkorn University, Bangkok, Thailand
- 4Department of Biological Sciences, Faculty of Science, Thompson Rivers University, Kamloops, BC, Canada
- 5Research Center of Microbial Diversity and Sustainable Utilization, Faculty of Science, Chiang Mai University, Chiang Mai, Thailand
This study was designed to investigate the cultivable actinobacteria associated with bryophytes and their plant growth promoting ability. Thirteen actinobacteria were isolated and tested for their ability to promote growth of plant in vitro and in planta. All isolates were able to produce IAA and siderophores. Six isolates were identified as members of the genus Micromonospora. Five isolates belonged to the genus Streptomyces and one each of Microbispora and Mycobacterium. Micromonospora sp. CMU55-4 was inoculated to rare moss [Physcomitrium sphaericum (C. Ludw.) Fürnr.] and could increase the amount of carotenoid, fresh weight, and dry weight of this moss. In addition, this strain promoted capsule production, and rescued P. sphaericum’s gametophytes during acclimatization to land. Strain CMU55-4 was identified as Micromonospora chalcea based on whole genome sequence analysis. Its plant growth promoting potential was further characterized through genome mining. The draft genome size was 6.6 Mb (73% GC). The genome contained 5,933 coding sequences. Functional annotation predicted encoded genes essential for siderophore production, phosphate solubilization that enable bacteria to survive under nutrient limited environment. Glycine-betaine accumulation and trehalose biosynthesis also aid plants under drought stress. M. chalcea CMU55-4 also exhibited genes for various carbohydrate metabolic pathways indicating those for efficient utilization of carbohydrates inside plant cells. Additionally, predictive genes for heat shock proteins, cold shock proteins, and oxidative stress such as glutathione biosynthesis were identified. In conclusion, our results demonstrate that bryophytes harbor plant growth promoting actinobacteria. A representative isolate, M. chalcea CMU55-4 promotes the growth of P. sphaericum moss and contains protein coding sequences related to plant growth promoting activities in its genome.
Introduction
Plant microbiomes are involved in the well-being of their host health by inhibiting the growth of plant pathogens and help plant to tolerate stress conditions (Gabriele, 2016). Plant growth-promoting rhizobacteria (PGPR), which live around root area of plants, can be beneficial to plants by mediating plant growth through diverse biochemical mechanisms such as biosynthesis of indole-3-acetic acid (IAA), 1-aminocyclopropane-1-carboxylic acid (ACC) deaminase, siderophore production, and phosphate solubilization. PGPR which are abundant in soil and rhizosphere can penetrate into root endosphere through cellular disjunction during lateral root emergence (Bulgarelli et al., 2013) to colonize inside plants. Actinobacteria, a phylum of Gram-positive bacteria that contained high amount of G+C in their DNA (>55 mol% of genomic DNA) are interesting due to their prolific metabolic capabilities (Yadav et al., 2018). Plant growth promoting actinobacteria (PGPA) are abundant in soil and can enhance nutrients availability, administer plant metabolism and reduce environmental stress in plants (Hamedi and Mohammadipanah, 2014). Bryophytes are group of plants that grow under specific climate conditions and their microbiomes are still not well studied. They are the oldest known land plants that lack true leaves, stem or true vascular system and considered as a remarkable reservoir of novel active compounds, natural products and antibiotics (Chandra, 2014). Actinobacteria associated with bryophytes could have some specific evolutionary adaptations to their host plants and may have potential of bioactive compounds production that need to be investigated. One of the easiest ways for bryophytes propagation is using tissue culture technique. However, the obtained in vitro plantlets are usually unable to compete with other native soil microbes when transfer to soil. In addition, micropropagated plants are struggled to cope with environmental conditions in the field which results in the morphological and anatomical changes of plantlets (Chandra and Bandopadhyay, 2010). The objectives of this study are
1. To investigate the cultivable actinobacteria associated with some bryophytes;
2. To study the plant growth promoting ability of actinobacteria from bryophytes.
One strain, Micromonospora chalcea CMU55-4 shows promising potential to promote the growth of tested bryophyte during acclimatization period in laboratory before transfer to the forest. Its taxonomic characterization and whole genome analysis are also reported.
Materials and Methods
Sample Collection
Bryophyte samples were collected from Doi Inthanon National Park, Chiang Mai, Thailand. Various keys and checklists were used for identification such as Hattori et al. (1977), Eddy (1988, 1996), Zander (1993), and Li et al. (2007). Actinobacteria were isolated from five species of bryophytes, including mosses and liverwort. Four high altitude moss species were Bryum apiculatum Schwägr. (CMU15), Syntrichia gemmascens (P.C. Chen) R.H. Zander (CMU51), Campylopus involutus (Müll. Hal.) A. Jaeger (CMU55), and Plagiomnium maximoviczii (Lindb.) T.J. Kop (CMU13). Liverwort was Frullania nepalensis (Spreng.) Lehm. and Lindenb. (CMU12). The remaining bryophyte samples from isolation were kept at Chiang Mai University Herbarium (Herbarium code: CMUB).
Selective Isolation
Each bryophyte sample (1 g fresh weight) was surface sterilized (70% ethanol 1 min, 3% sodium hypochlorite 10 min, 3 times sterile distilled water). Sterile samples were crushed in sterile mortars, diluted with sterile distilled water up to 10–3 and plated on starch casein agar (Küster and Williams, 1964), humic acid vitamin B agar (Hayakawa and Nonomura, 1987), R2A agar and water proline agar (1% proline). All media were supplemented with 25 μg/ml nystatin and 10 μg/ml cycloheximide. Plates were incubated for 30 days at 25°C. All colonies appeared on agar were picked and purified on ISP2 agar (Shirling and Gottlieb, 1966). The effectiveness of the surface sterilization method was evaluated by spread the final rinse water of each bryophyte sample on ISP2 agar.
Plant Growth Promoting Activity
IAA production was determined following the methods of Glickman and Dessaux (1995). In brief, actinobacteria were grown in ISP2 broth with and without 2 mg/ml L-tryptophan for 7 days in the dark. After that, the supernatant was mixed with Salkowski’s reagent (1 ml of 0.5 M FeCl3 in 50 ml 35% HClO4), stored at room temperature for 30 min and measured the optical density at 530 nm. IAA production is also confirmed by HPLC (Fluorescent detector) with a Restek Ultra C18, 5 μm (150 × 4.6 mm) using 0.1 M acetic acid as mobile phase A and 0.1 M acetic acid in methanol as mobile phase B (1 ml/min flow rate). Standard methods were used to determine siderophore production qualitatively and quantitatively. Siderophore production was first screened on CAS agar (Schwyn and Neilands, 1987) using King’s B agar as a basal medium (King et al., 1954). The appearance of a yellow to orange zone around the actinobacterial colony after 7 days incubation in the dark was an indication of siderophore production. Quantitative determination of siderophore was carried out in liquid medium. Actinobacteria were grown in King’s B broth for 7 days. The culture supernatant was mixed with ferric perchlorate solution for hydroxamate siderophore (Atkin et al., 1970), and 0.5 M HCl, nitrite-molybdate reagent and 1 M NaOH for catecholate siderophore (Arnow, 1937). Phosphate solubilization was determined from clear zone formation on Pikovskaya (PVK) agar supplemented with 0.5% (w/v) tricalcium phosphate after 7 days incubation (Nautiyal, 1999). Drought tolerant ability of actinobacteria was also investigated using sorbitol as an osmotic adjustment in water agar medium (Hallsworth et al., 1998).
Taxonomic Characterization
DNA extraction was performed following the method of Kudo et al. (1998). Genomic DNA was used as the template for polymerase chain reaction (PCR) following the method of Lee et al. (2014). 27F (5′ AGAGTTTGATCMTGGCTCAG 3′) and 1492R (5′ TACGGYTACCTTGTTACGACTT 3′) primers were used to amplify 16S rRNA gene. The PCR amplicons were purified by GF-1 AmbiClean Kit (Vivantis®) following the manufacturer instruction. The 16S rRNA sequencing was performed by commercial service at First BASE Laboratories Sdn Bhd, Malaysia. Identification of all actinobacterial isolates was achieved by BLAST analysis of 16S rRNA gene sequences using EzBiocloud database1. Neighbor-joining phylogenetic tree was constructed using BioEdit Sequence Alignment Editor version 7.2 and MEGA7 (Kumar et al., 2016). Tree topology was evaluated using the bootstrap resampling method at 1000 bootstrap. Strain CMU55-4 was also identified based on whole genome sequence analysis. The ANI value was calculated and compared in JSpeciesWS (Ritcher et al., 2016), web server tool, using ANI-Blast (ANIb) and ANI-MUMmer (ANIm) algorithms (Ritcher and Rosselló-Móra, 2009) within the web service. The Genome-to-Genome Distance Calculator (GGDC 2.1) with the BLAST + method (Meier-Kolthoff et al., 2013) was used to evaluate the digital DNA-DNA hybridisation (dDDH).
Growth Promotion on Physcomitrium sphaericum (C. Ludw.) Fürnr.
Physcomitrium sphaericum (C. Ludw.) Fürnr. was used as a moss model to determine beneficial effects of selected actinobacteria on bryophytes during transplantation. P. sphaericum is a rare moss species in Thailand with the risk from extinction. The re-introduction of this moss back to nature is badly needed. Spores of P. sphaericum were picked from healthy plants from the nature under stereo microscope using sterile forceps and placed into Hoagland agar (Hoagland and Arnon, 1950) and incubated for 3 months at 24°C, 35,000–40,000 lux intensity to let spore germinate and form protonema. Then, the moss protonema was transferred to Hoagland agar and incubated in the same condition for 3 months. The moss plantlets were transferred from agar media to soil. Strain CMU55-4 was chosen to be introduced to moss due to its high IAA production in vitro and fast growth within 3 days which is an important trait of PGPA to compete with other soil microbes. Strain CMU55-4 was grown in ISP2 broth for 7 days, 25°C, 150 rpm. Culture broth was centrifuged to collect cells. Cells were washed and resuspended in sterile distilled water and adjusted the concentration to OD600 = 1, which is equivalent to 106 cells/ml. A suspension of strain CMU55-4 (1 ml) was dropped into the autoclaved soil around the plants. Sterile distilled water was used as a control solution. The plants were maintained in the incubator at 24°C, 35,000–40,000 lux for 1 month. Chlorophyll and carotenoid contents were determined from fresh samples using standard method (Chappelle et al., 1992). Dry weight was obtained from plants that were dried in 60°C oven for 7 days.
Genome Mining for Plant Growth Promoting Potential
Whole genome sequencing of Micromonospora sp. CMU55-4 was carried out by an Illumina Miseq platform (Illumina, Inc., San Diego, US-CA) using 2 × 250 bp paired-end reads. Raw reads quality was checked using FASTQC software (Andrews, 2010). Adaptors and poor-quality reads were removed using Trim Galore (Krueger, 2015), and the filtered reads were used as an input for Unicycler (Wick et al., 2017), genome assembly program. Annotation of assembled genome was done using Prokka Version 1.13 (Seemann, 2014), and NCBI Prokaryotic Genome Annotation Pipeline (PGAP). The draft genome sequence of strain CMU55-4 was mined using RAST annotation server (Aziz et al., 2008) and analyzed through SEED viewer (Overbeek et al., 2014) for genes responsible for plant growth promoting properties.
GenBank Accession Number
The accession number of the draft genome sequence is JAAOLH000000000.
Chemotaxonomic, Cultural, and Phenotypic Characterization of Micromonospora sp. CMU55-4
The type strain Micromonospora chalcea DSM 43026T was purchased from Deutsche Sammlung von Mikroorganismen und Zellkulturen (DSM) culture collection. Micromonospora terminaliae TMS7T was kindly given by Dr. Onuma Kaewkla of Department of Biology, Faculty of Science, Mahasarakham University. Cultural characteristics were determined after 2 weeks at 30°C on ISP1-7 media (Shirling and Gottlieb, 1966), Nutrient agar (Difco), Tryptic Soy agar (Difco), Potato Dextrose agar (Difco), Modified Bennett agar (Jones, 1949), and ATCC172 agar. The ISCC-NBS color chart (Kelly, 1964) was used to determine the color of mycelia. pH range for growth (4.0–12.0 at intervals of 1.0 pH unit), tolerant to NaCl concentration (0–9% at 1% intervals) and temperature range for growth (4, 20, 25, 30, 37, 40, 45, 50, 55°C) were determined using ISP2 agar as a basal medium. H2S production, coagulation and peptonization, gelatin liquefaction, starch hydrolysis and nitrate reduction were determined on ISP6 agar, 10% skim milk (Difco), glucose-peptone-gelatin medium (2.0% glucose, 0.5% peptone, 20% gelatin pH 7.0), ISP4 agar and ISP8 broth (0.5% peptone, 0.3% beef extract, 0.1% KNO3, pH 7.0). The production of enzymes was determined using API ZYM kit (bioMérieux). The utilization of carbohydrates as sole carbon sources was obtained using ISP 9 (Nihon Pharmaceutical) as the basal medium supplemented with 1% (w/v) of each carbon source. Spore morphology of 21-day-old culture on ISP2 agar was observed by scanning electron microscope (JSM-IT500HR; JEOL). For chemotaxonomic study, biomass of strain CMU55-4 was obtained from culture grown in ISP2 broth for 7 days at 30°C (160 rpm) and freeze dried. The analysis of whole cell reducing sugar and isomer of diaminopimelic acid on cell wall (A2pm) was determined on thin-layer-chromatography (TLC) following the method of Staneck and Robert (1974). Polar lipids profiles were extracted and identified using 2-dimensional TLC according to the method of Minnikin et al. (1984). Menaquinones were extracted according to the method of Collins et al. (1977) and were analyzed by UPLC (Aligent Technology 1290 Infinity II model: 67116B 1290MCT UV detector) with a μBondapak C18 column (Waters).
Statistical Analysis
Statistical analysis was performed by SPSS statistics 17.0 program.
Results
Selective Isolation
A total of 13 actinobacteria were obtained from all four selective media. The highest number of isolates was recovered on water proline agar (6 isolates, 46.15%) followed by humic acid vitamin B agar (4 isolates, 30.76%), starch casein agar (2 isolates, 15.38%) and R2A gar (1 isolate, 7.69%) (Table 2). Most isolates were from C. involutus (38%). However, no bacteria were obtained from liverwort.
Plant Growth Promoting Activity
All isolates produced indole-3 acetic acid and siderophores at varying amount (Table 1). Isolates CMU51-1 and CMU55-4 produced the highest amount of IAA without L-tryptophan supplement (2.73 μg/ml) and in 2 mg/ml of L-tryptophan (11.35 μg/ml), respectively. For siderophores production, isolates CMU51-1 produced the highest amount of both hydroxamate type (992.50 ± 50.76 μMole/l) and catecholate type siderophores (484.47 ± 27.91 μMole/l) (Table 1). For drought tolerant ability, no growth was observed below aw 0.957 for all isolates. None of the obtained isolates could solubilize tricalcium phosphate on PVK agar.
Taxonomic Characterization
Comparison of 16S rRNA gene sequence similarity of the obtained actinobacteria in EzBiocloud database, assigned them as members of the following genera: Micromonospora, Streptomyces, Mycolicibacterium and Microbispora (Table 2). The majority of isolates were Micromonospora (46%) followed by Streptomyces (38%). The 16S rRNA gene similarity values were ranged from 96.31 to 100%. Phylogenetic analysis confirmed the assignment of these actinobacteria at genus level based on BLAST results (Figure 1). Strain CMU55-5 is closely related to Microbispora rosea subsp. rosea ATCC 12950T with low 16S rRNA gene sequence similarity value of 96.3%. Strain CMU55-4 is closely related to Micromonospora chalcea DSM 43026T with 16S rRNA gene sequence similarity value of 98.2%. The ANIb values of 98.47% and digital DNA-DNA hybridization value of 91.8% were found between strain CMU55-4 and M. chalcea DSM 43026T.
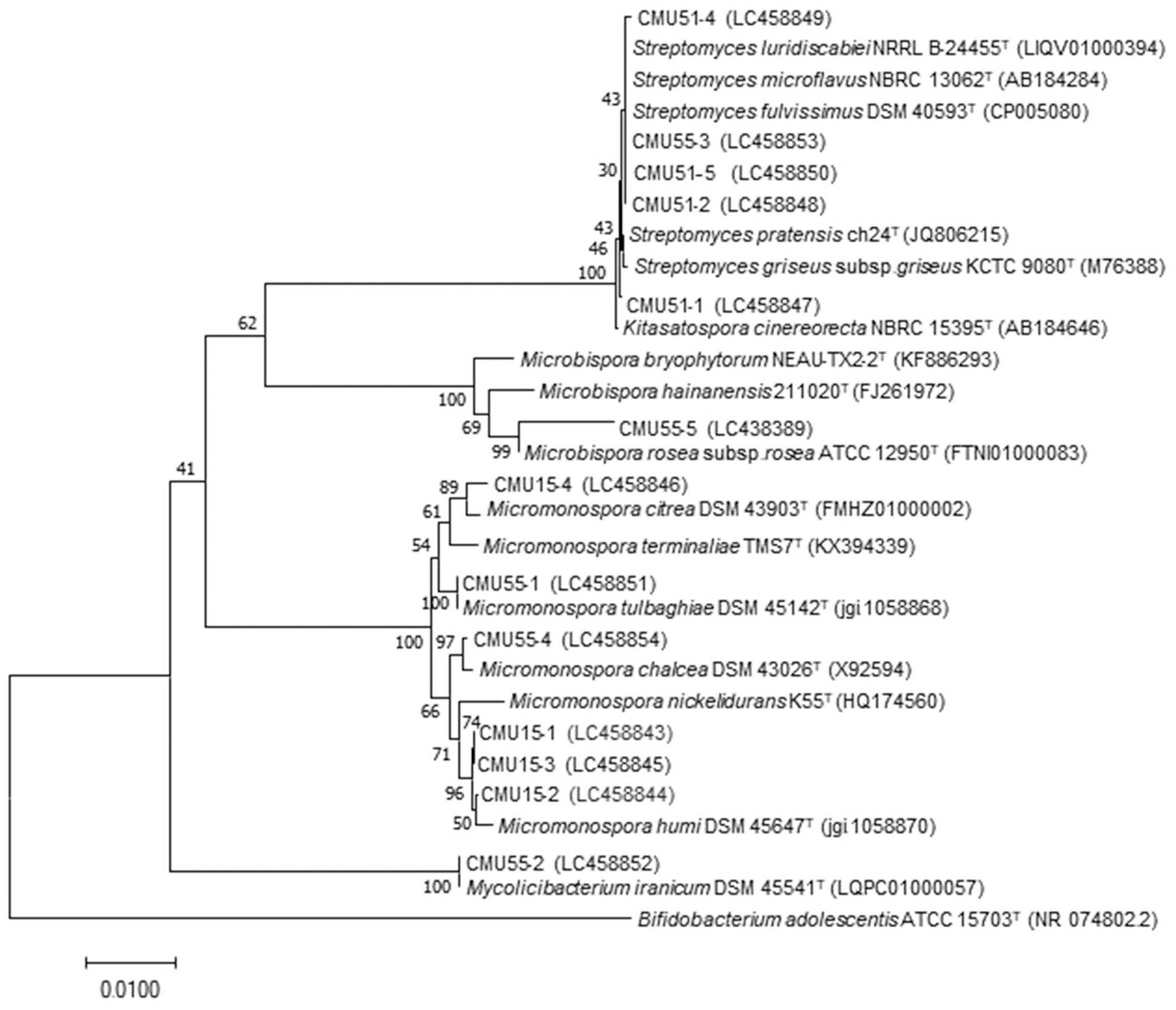
Figure 1. Phylogenetic tree based on neighbor-joining analysis of 16S rRNA gene sequences from bryophytes associated actinobacteria and their nearest neighbors. The numbers at branch nodes indicate bootstrap percentages derived from 1,000 replications. Bar, 0.01 substitutions per nucleotide position.
Growth Promotion on Physcomitrium sphaericum (C. Ludw.) Fürnr.
Physcomitrium sphaericum (C. Ludw.) Fürnr. plantlets that were inoculated with Micromonospora strain CMU55-4 showed better growth appearance compared to control plants. The control plants have more chlorosis leaves and showed growth retardation (Figure 2A). The plants that had been treated with strain CMU55-4 started to develop new leaves and showed the production of capsules (Figure 2B). Carotenoid, fresh weight and dry weight of P. sphaericum were significantly higher than the control. However, total chlorophyll content was not different in control and strain CMU55-4 inoculated plantlets (Figure 3).
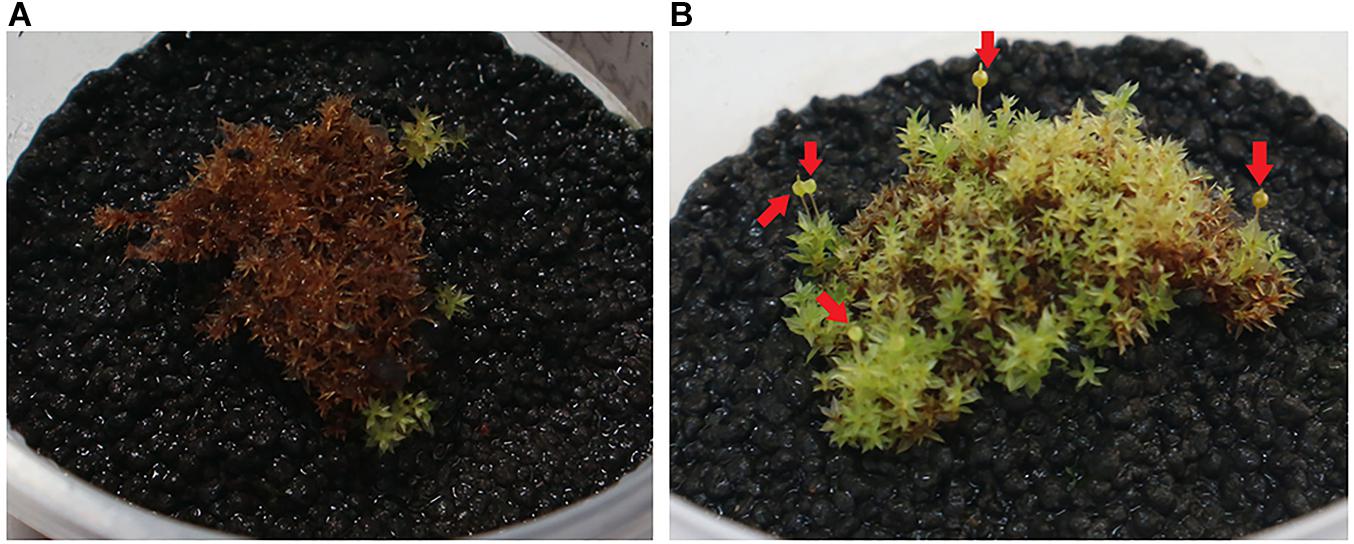
Figure 2. P. sphaericum control plant (A) and P. sphaericum inoculated with CMU55-4 for 1 month (B). Arrows indicated capsule production.
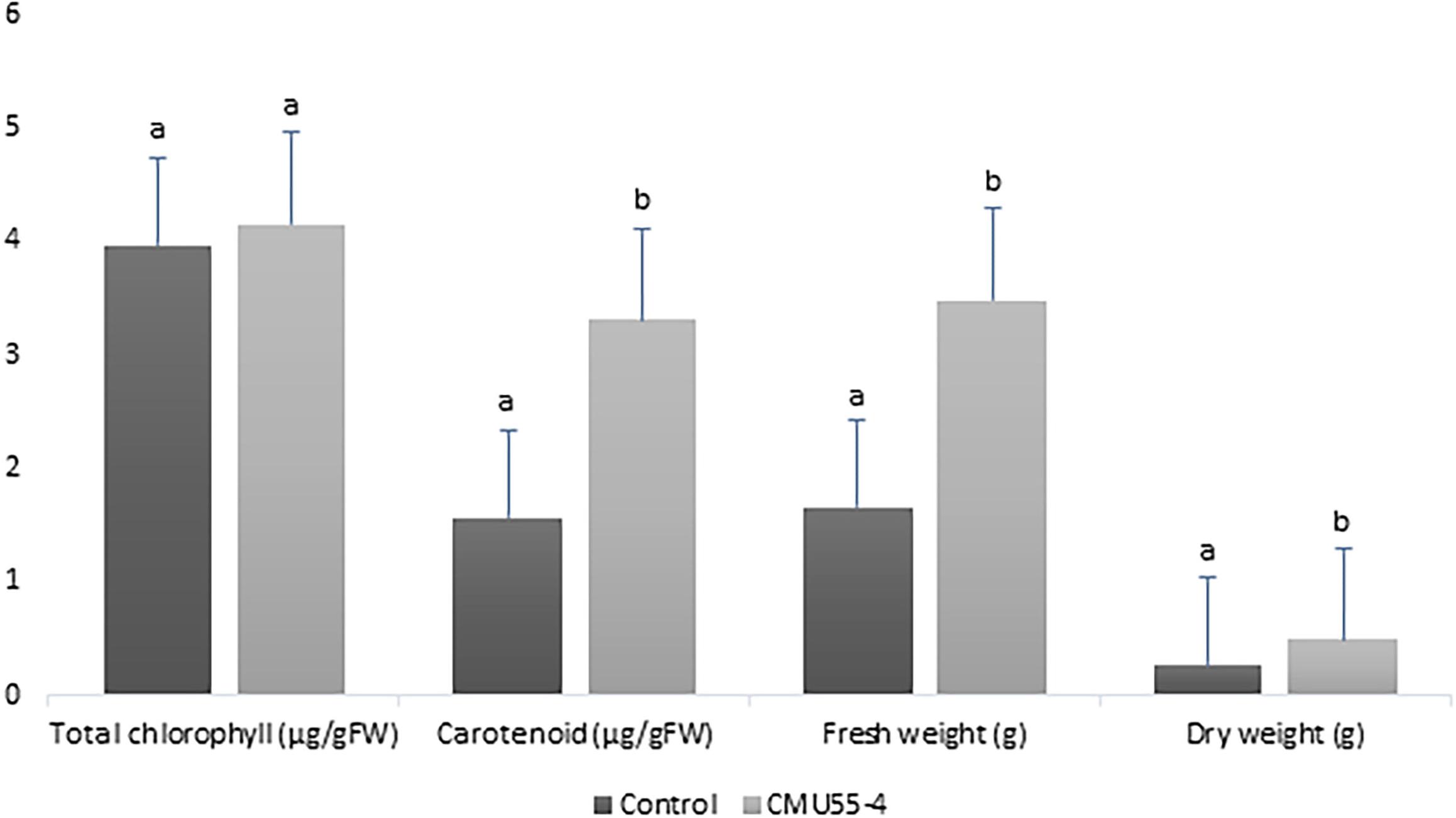
Figure 3. Effects of Micromonospora strain CMU55-4 toward the growth of P. sphaericum. a, b indicated significant difference in statistical analysis tested by SPSS independent T-test (p < 0.05), n = 3.
Genome Mining for Plant Growth Promoting Potential
From whole genome sequence data, the estimated genome size of Micromonospora sp. CMU55-4 was 6.6 Mb. The GC content was 73.0%. The summary of sequence assembly and genome annotation is displayed in Table 3. Phylogenomic tree clearly classified isolate CMU55-4 in the same species and subspecies level with M. chalcea DSM 43026T (Figure 4). Prokka predicted 5,946 coding regions with 6,077 estimated genes (Table 4) contained 67 tRNAs, 3 rRNAs and 2 repeat regions. Gene annotation in RAST server showed that genes were grouped into 25 subsystems (Supplementary Material 1). The majority of genes play roles in amino acids, carbohydrate, and protein metabolisms. From various subsystems, essential roles of protein coding sequences according to their plant growth promoting traits was grouped in Table 5. Twenty-four genes were associated with siderophore production, namely siderophore assembly kit, siderophore desferrioxamine E, and siderophore aerobactin. Indole-3-glycerol phosphate synthase and tryptophan synthase plays role in IAA synthesis. Gene encoding exopolyphosphatase with possible role in phosphate solubilization was also found. In addition, protein coding sequences involved in nitrogen metabolism such as assimilatory nitrate reductase, nitrate/nitrite transporter, and ammonium transporter were also detected. Strain CMU55-4 was well equipped with protein coding sequences related to oxidative stress response such as SoxR, NsrR, organic hydroperoxide reductase, glutathione peroxidases and trehalose synthesis genes. Glycine betaine transporter (OpuD) for osmotic adjustment under osmotic stress was also present. CspA protein family, DnaK and DnaJ chaperones responsible for cold or heat stress were identified. Strain CMU55-4 also exhibited genes involved in the utilization of saccharides found inside plant cells such as xylose, arabinose, mannose and D-galacturonate (Table 5).
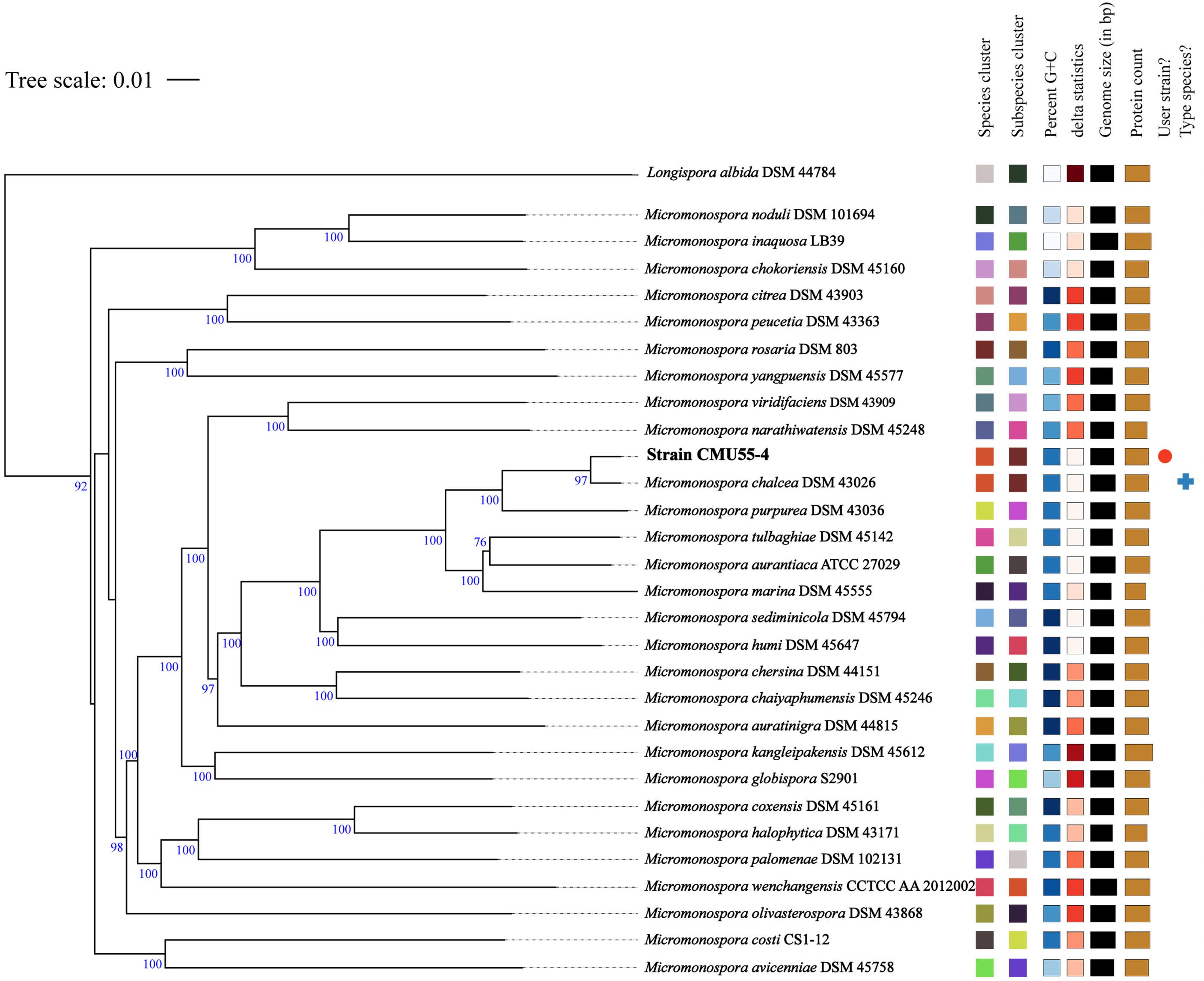
Figure 4. Phylogenomic tree of strain CMU55-4 and their closely related type strains of the genus Micromonospora. Tree inferred with FastME 2.1.6.1 (Lefort et al., 2015) from Genome BLAST Distance Phylogeny (GBDP) distances calculated from genome sequences. The branch lengths are scaled in terms of GBDP distance formula d5. The numbers above branches are GBDP pseudo-bootstrap support values from 100 replications, with an average branch support of 92.6 %. The tree was rooted at the midpoint (Farris, 1972). Leaf labels were annotated by affiliation to species and subspecies clusters, genomic G+C content, δ values, overall genome sequence length, number of proteins, and the kind of strain, respectively.
Chemotaxonomic, Cultural, and Phenotypic Characterizations of Micromonospora sp. CMU55-4
Strain CMU55-4 grew well on TSA, modified Bennett’s, and ATCC172 media. The colors of substrate mycelia were strong orange on ISP2, deep yellowish-brown on ISP1 and ISP3, ISP5, and ISP7, strong orange yellow on ISP4, ISP6 and TSA. Dark olive brown on modified Bennett’s and vivid orange on ATCC172. No growth was observed on NA and PDA. The strain produced globular spores (0.8 μm in size) with warty surface (Figure 5). The strain grew at 20 to 45°C (optimally at 30°C), pH 5.0 to 12.0 (optimally pH at 8.0) and tolerated up to 4% (w/v) NaCl. Strain CMU55-4 utilized various type of carbohydrates such as L-arabinose, dulcitol, D-mannose, and D-mannitol and those found in plant cells including D-glucose, sucrose, cellulose, amygdalin, and starch (Table 6). Cell-wall peptidoglycan of strain CMU55-4 contained meso-diaminopimelic acid. Galactose, arabinose and xylose were detected as diagnostic sugars in the whole-cell hydrolysates. The predominant phospholipids were diphosphatidylglycerol (DPG), phosphatidylethanolamine (PE), and phosphatidylinositol (PI). Major menaquinones were MK-9(H4) (33.46%), MK-9(H6) (13.63%), MK-9(H8) (26.16%), and MK-10(H8) (15.38%).
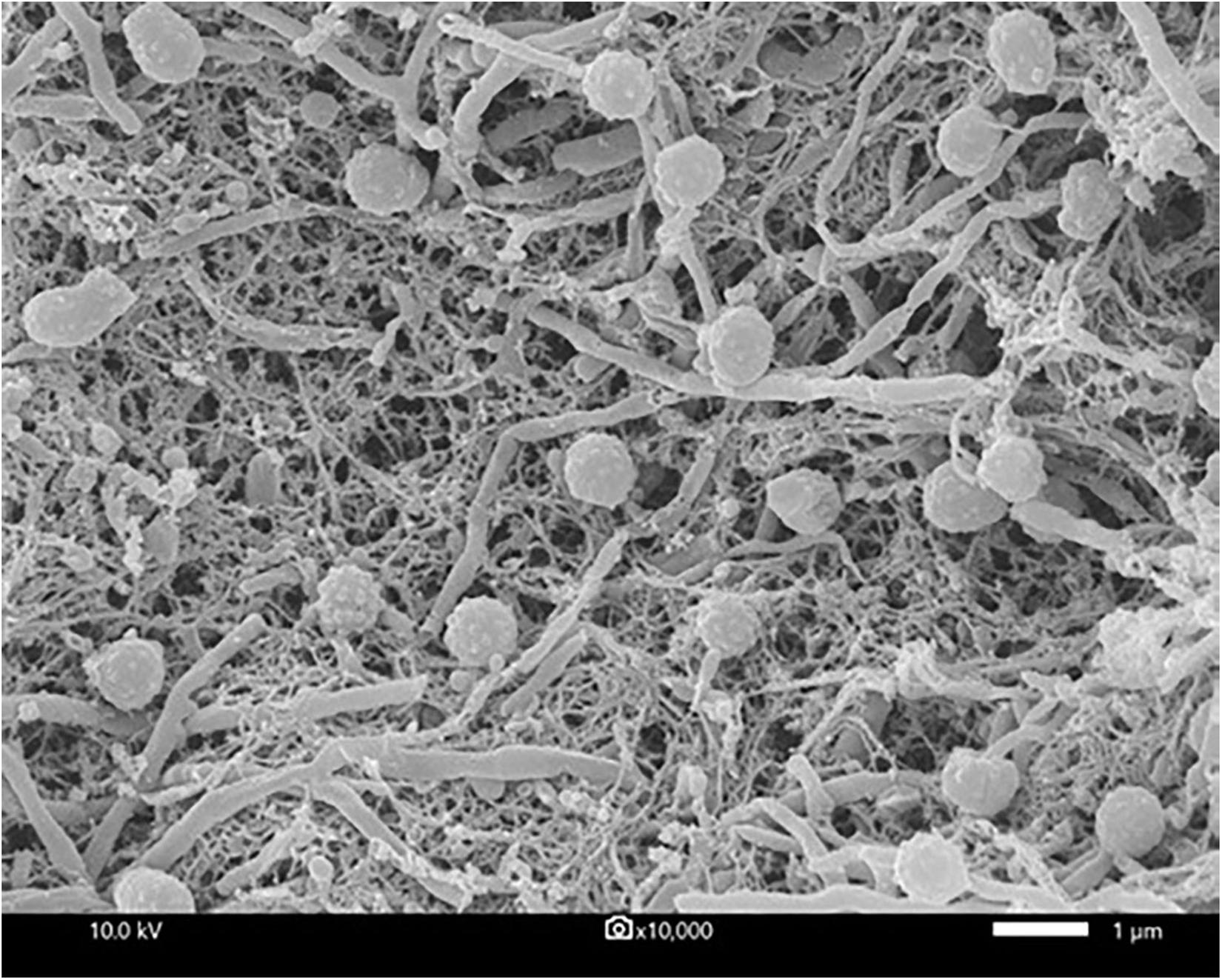
Figure 5. Scanning electron micrograph showing M. chalcea CMU55-4 grown on ISP2 agar medium at 30°C for 21 days. Bar represents 1 μm.
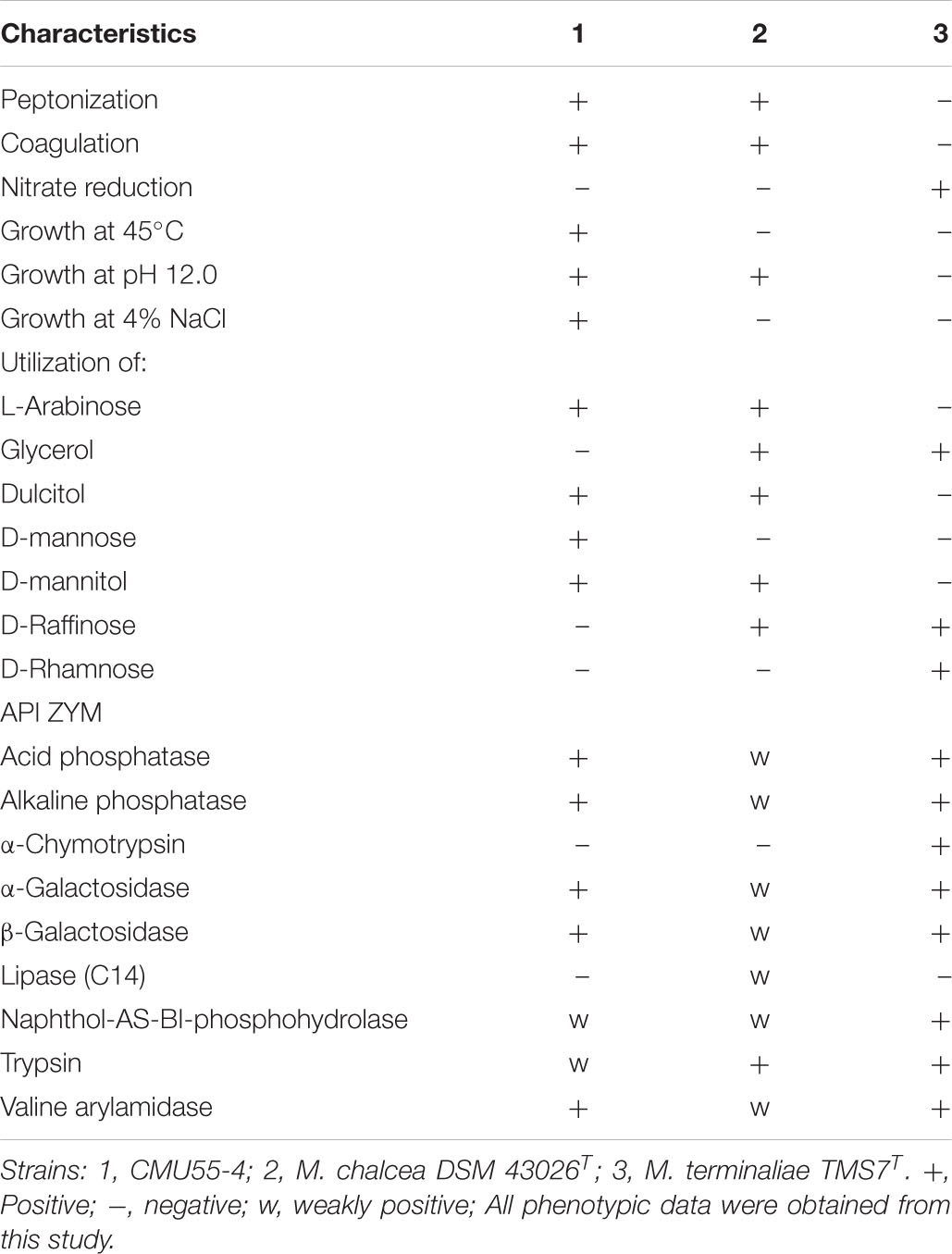
Table 6. Differential phenotypic characteristics of strain CMU55-4 and its phylogenetically closest type strains.
Discussion
Actinobacteria from bryophytes are very rare. In the earliest report in 2007, small number of Rothia, Arthrobacter, Micrococcus, and Plantibacter isolates were reported as endophytic actinobacteria from Sphagnum magellanicum Brid. and Sphagnum fallax H. Klinggr (Opelt et al., 2007). Only three new species of actinobacteria were described from unidentified moss species namely Streptomyces bryophytorum (Li et al., 2016), Actinoallomulus bryophytorum (Li et al., 2015a) and Microbispora bryophytorum (Li et al., 2016). In this study, almost half of actinobacteria (46%) were identified as Micromonospora. Members of this genus have been isolated from various habitats including plants (Trujillo et al., 2010, 2015; Carro et al., 2012, 2013; Genilloud, 2015). Several new species of Micromonospora are endophytes of various plants as exemplified by the description of M. costi (Thawai, 2015), M. globbae (Kuncharoen et al., 2018), M. phytophila (Carro et al., 2018b), M. luteiviridis (Carro et al., 2018b), M. oryzae (Kittiwongwattana et al., 2015), and M. terminaliae (Kaewkla et al., 2017). Streptomyces isolates also dominant represent around 38.5%. Our results provide additional evidence that these two actinobacterial genera are also dominant in bryophytes. Mycolicibacterium stellerae was recently described as endophytic actinobacteria from plant, Stellera chamaejasme L. in Yunnan (Nouioui et al., 2019b). However, this is the first time that member of this genus was found associated with a bryophyte. The remaining isolate, CMU55-5 was closely related to Microbispora rosea subsp. rosea. The low 16S rRNA gene similarity value (96.3%) between CMU55-5 and its closest neighbor, suggests that this strain may represent a new species. However, polyphasic taxonomic characterization is needed for its formal description which will be the subject of future investigation.
No actinobacteria was obtained from liverwort. This may be an effect of “oil bodies,” the highly distinctive organelle uniquely found in liverworts (He et al., 2013). This organelle is responsible for toxic compound accumulation that usually use for protection of liverworts from herbivore (Stahl, 1888), pathogens, low temperature and excessive light (Hieronymus, 1892). We opined that during the isolation of actinobacteria, the liverwort sample was crushed which may cause the release of toxic compounds from oil bodies and inhibit the growth of actinobacteria.
The use of water proline agar yielded the highest number of actinobacterial isolates in this study. Water proline agar is a low nutritional medium that simulate oligotrophic status in natural environments. Proline is also serving as a compatible solute that commonly found in plant cells. The composition of the minimal media is suggested to allow an easier adaptation for endophytes (Alain and Querllou, 2009) and allow slow growing endophytic strains a chance to develop (Eevers et al., 2015). Complex media composed of rich carbon and nitrogen sources are suggested to be unsuitable for the growth of slow-growing endophytic bacteria as they do not resemble the environment inside plant tissues (Eevers et al., 2015). Low nutritional media have been successfully used to isolate actinobacteria from various environmental samples (Janssen et al., 2002; Wang D.-S. et al., 2014; Ruttanasutja and Pathom-aree, 2015).
Strain CMU55-4 was classified into the genus Micromonospora of the family Micromonosporaceae based on the sequence of 16S rRNA gene and its unique phenotypic characteristics. It forms a characteristic orange colony at the early stage, which turns to dark olive brown color with age. The strain produced extensively branched substrate hyphae, lack of aerial mycelia which is a unique morphological characteristic of the genus Micromonospora (Ørskov, 1923). The strain formed a single non-motile warty-globular spore on the vegetative mycelium similar to the closest type strains, M. chalcea DSM 43026T (Ørskov, 1923). The formation of single spores is also the main morphological characteristic of the genus Micromonospora (Trujillo et al., 2015). A meso-diaminopimelic acid was found in the cell wall peptidoglycan of strain CMU55-4 with arabinose, xylose and galactose as diagnostic sugars in the whole-cell hydrolysates corresponding to the cell wall type II and sugar type D (Lechevalier et al., 1971). Strain CMU55-4 exhibited phospholipid type II, comprising of phosphatidylethanolamine (PE), diphosphatidylglycerol (DPG), and phosphatidylinositol (PI) as the major phospholipids (Lechevalier et al., 1977). The strain contained a large amount of MK-9(H4) and MK-9(H6) which generally found in the genus Micromonospora (Nouioui et al., 2018). Strain CMU55-4 shared 98.47% of ANIb values and 91.8% digital DNA-DNA hybridization to M. chalcea DSM 43026T. The value of <95–96% ANI or 70% dDDH is proposed as a cutoff value for delineating of new species (Chun et al., 2018). Therefore, strain CMU55-4 was identified as M. chalcea CMU55-4.
Micromonospora have long been recognized as antibiotic producers (Hirsch and Valdes, 2010). Recently, endophytic Micromonospora have been regarded as plant growth promoting bacteria especially in legumes (Trujillo et al., 2014; Benito et al., 2017). Physcomitrium sphaericum moss is known to be distributed only in the temperate regions; North America, Europe, Russia, China, and Japan. Recently, it has been found in the tropical country and reported as new record to Thailand (Printarakul et al., 2014). The inoculation of M. chalcea CMU55-4 on P. sphaericum moss resulted in an increase of carotenoid content, fresh and dry weight significantly higher than the control (Figure 3). This promotion effect appears to be related to the production of phytohormone IAA as M. chalcea CMU55-4 produced the highest IAA among all tested actinobacteria. Interestingly, P. sphaericum inoculated with M. chalcea CMU55-4 developed new leaves and showed the production of capsules (Figure 2B). Capsule is an important part of bryophyte reproduction as it represent sporophyte stage and benefits in asexual reproduction through spores dispersion (Sundberg et al., 2014). It is likely that the moss-bacterium interaction between M. chalcea CMU55-4 and P. sphaericum may play a role in promote leaves and capsule development though the exact mechanism still required further investigation. Effect of moss associated bacteria on Pylaisiella selwynii moss development was reported that the obtained Gram-negative bacteria could promote protonemal growth and gametophore initiation of P. selwynii (Spiess et al., 1981). Recently, auxin has been shown to involve in the development of moss (Physcomitrella patens) including gametophore and sporophyte development (Thelander et al., 2018). Almost all works regarding plant growth promoting actinobacteria are carried out with vascular plants. For examples, the inoculation of Micromonospora strain SB3 promotes plant biomass, root length and increases the acclimatization rate of Lolium multiflorum plantlets after 4 weeks of in vitro culture (Della Mónica et al., 2018). Recently, an endophytic M. chalcea UAE1 from halophytic crop, Salicornia bigelovii has been reported to promote the growth of S. bigelovii mainly by ACC-deaminase production (El-Tarabily et al., 2019). To the best of our knowledge, this is the first report on potential of Micromonospora species on the growth and development of non-vascular plants such as mosses.
Approximate genome sizes of 40 Micromonospora type strains were reported to be ranged from 6.1 Mbp for Micromonospora marina DSM 45555T to 7.9 Mbp for M. carbonacea DSM 43168T with the average genome size for all of the Micromonospora strains was 7 ± 0.4 Mbp (Carro et al., 2018a). The estimated genome size of our M. chalcea CMU55-4 was within these previously reported ranges (6.6 Mb) with 73.0% GC content. The genome size of M. chalcea DSM 43026T was 7.0 Mb and 72.8% G+C content (Carro et al., 2018a). The slightly bigger genome size of 7.086 Mb was reported from plant growth promoting rhizosphere Micromonospora sp. strain MW-13 with a similar G+C content of 73.3% (Jahanshah et al., 2019).
Omics techniques, including genome sequencing, comparative genomics, microarray, next generation sequencing, metagenomics, and metatranscriptomics can be used to explain plant-endophyte relationship (Kaul et al., 2016). Endophytic lifestyle of M. chalcea CMU55-4 was supported by data from genome mining and phenotypic studies as this strain was able to utilize various kind of saccharides and carbohydrates which found inside plant cells. Saccharides molecules were discharged from plant cell walls during elongation process and served as nutrients to promote the colonization ability of plant-associated bacteria (Etesami et al., 2015). M. chalcea CMU55-4 is also able to produce both catecholate and hydroxamate type siderophores in vitro. In addition, several predictive genes related to siderophore production were also detected in its genome for examples desferrioxamine E and aerobactin and genes encoding transporter proteins. Neilands and Leong (1986) suggested that siderophore systems may play a role in infections of plant tissue. Thus, the ability of M. chalcea CMU55-4 to produce siderophores may help this strain to enter and live inside plant cells. Metallophores producing Micromonospora strains were recently reported to promote the growth of Arabidopsis thaliana under heavy metal condition by playing roles in metal acquisition, iron metabolism and resistance to toxic compounds (Ortúzar et al., 2020). Genes mutually found in Ortúzar et al. (2020) and this study are ferric hydroxamate ABC transporter (FhuB, FhuC, FhuD), siderophore desferrioxamine E (DesA, DesB, DesC, DesD), and siderophore aerobactin (FhuB, FhuC, FhuD).
Previous studies on actinobacterial genome revealed genes related to plant growth promoting activities primarily production of IAA, siderophore, phosphate solubilization, and phytopathogen inhibition (Gupta et al., 2014; Liu et al., 2019; Nouioui et al., 2019a). Predictive genes obtained from genome mining in this study supported the experimental data on IAA and siderophore producing abilities of M. chalcea CMU55-4 in vitro. Tryp-dependent pathway could be a pathway for IAA synthesis in strain CMU55-4 as predictive genes for tryptophan synthase alpha chain (TSA1), tryptophan synthase beta chain (TSB), indole-3-glycerol phosphate synthase (IGS) were found. Indole-3-glycerol phosphate or indole is a branch point for Tryp-dependent pathway that directly leads to IAA synthesis (Spaepen and Vanderleyden, 2011; Di et al., 2016). IAA promotes plant growth by advocating cell division, cell elongation, stimulates primary growth, and plays role in stress resistant. IAA production trait is considered as a useful criterion for selection of endophytic and rhizospheric bacteria in rice growth promotion (Etesami et al., 2015). High IAA production without L-tryptophan was recorded in some isolates such as CMU51-1 and CMU51-5. This observation suggests the possibility that these isolates can produce IAA by Tryp-independent pathway. M. chalcea CMU55-4 failed to solubilize tricalcium phosphate on PVK agar though predictive gene for exopolyphosphatase was detected. Since only tricalcium phosphate was used for phosphate solubilizing property, we cannot rule out the possibility of M. chalcea CMU55-4 to solubilize other types of inorganic phosphates. M. chalcea CMU55-4 also genetically possessed abilities related to nitrogen metabolism in particular nitrate/nitrite ammonification and ammonia assimilation. These abilities could enhance nitrogen uptake system of plant as nitrate (NO3–) and ammonium (NH4+) are the main resources of inorganic nitrogen (N2) absorbed by the roots of higher plants (Wickert et al., 2007).
It is also interesting to note the gene sequences responsible for other functions, such as the production of antioxidants and heat shock/cold shock proteins were detected though these properties had not been investigated under laboratory conditions in this study. M. chalcea CMU55-4 contained predictive genes involved with oxidative stress response such as SoxR, NsrR, organic hydroperoxide resistance protein, trehalose synthesis genes, glutathione S-transferase omega, and carotenoid production, which are the molecules help plant to tolerate to oxidative stress. Reactive oxygen species (ROS) created by the plant cells, are generally neutralized by the production of enzymes such as superoxide dismutases (SOD), catalases (CatA), peroxidases (POD), alkyl hydroperoxide reductases (AhpC), genes conferring nitrosative stress caused by reactive nitrogen species, and glutathione-S-transferases (GSTs) in endophytes (Khare et al., 2018). GST was found in the genome of Streptomyces scabrisporus NF3, an endophyte of Amphipterygium adstringens (Ceapã et al., 2018). The ability to alleviate oxidative stress is one attractive property of plant growth promoting bacteria. Forty-two genomes of Micromonospora strains including the type strain of M. chalcea, were analyzed to determine the presence of PGP genes and other characteristics related with plant growth promotion (Carro et al., 2018a). Genomes of Micromonospora strains contain genes related to stress responses such as cspA, cspC for cold shock response, dnaK and grpE for heat shock responses, and sod for oxidative stress. Similar observation was also found in this study.
Bryophytes require high water for growth. They are more sensitive to water deficit condition than other plants. Accumulation of compatible solute such as glycine betaine therefore can help bryophytes to maintain water balance. Glycine betaine transporter OpuD was found in M. chalcea CMU55-4 genome. The opuD gene product was reported to be essential for glycine betaine uptake and osmoprotection in E. coli (Kappes et al., 1996). Glycine betaine accumulation had been reported to increase the drought tolerant in Streptomyces chatreusis (Wang et al., 2019). Predictive genes involve in trehalose metabolism was also present in M. chalcea CMU55-4 genome including gene encoded for trehalose synthase. Trehalose is another compatible solute that can protect plant proteins and cellular membranes from inactivation or denaturation caused by a variety of stress conditions, including desiccation, dehydration, heat, cold, and oxidation (Elbein et al., 2003).
Bryophytes usually grow in low temperature and high humidity area. Cold shock protein may be essential for the adaptation of actinobacteria to live with bryophytes under such environment. M. chalcea CMU55-4 genome is equipped with CspA family of cold shock protein and several heat shock protein genes. This protein plays an essential role in inhibition of DNA replication during cold-adaptation in Streptomyces sp. AA8321 (Kim et al., 2007). Similarly, heat shock proteins (HSP) can be benefit to bryophytes for survival under high temperature. HSPs remove denatured proteins to prevent formation of large protein aggregates and cell death while DnaK/DnaJ/GrpE or GroEL/GroES chaperones are part of sigma-32 heat shock regulon that regulates cell during heat stress and maintains protein homeostasis in living cells (Schumann, 2016).
In conclusion, this study provides the first evidence of cultivable actinobacteria associated with three high altitude moss species, Bryum apiculatum, Syntrichia gemmascens and Campylopus involutus. These actinobacteria show plant growth promoting ability in vitro. The inoculation of the selected strain, M. chalcea CMU55-4, can promote the growth of rare moss species, P. sphaericum. The response of this moss to M. chalcea CMU55-4 suggested that actinobacteria of the genus Micromonospora might occur naturally in association with the moss and might commonly affect moss development in nature. Genome mining data also support plant growth promotion potential of M. chalcea CMU55-4 as a good candidate for breeding program of P. sphaericum especially those under acclimatization and other moss species.
Data Availability Statement
The datasets generated for this study can be found in online the repositories. The names of the repository/repositories and accession number(s) can be found below: https://www.ncbi.nlm.nih.gov/genbank/, JAAOLH000000000.
Author Contributions
CI performed overall experiments and wrote the manuscript. WP, NC, and ST supervised CI. CI, WP, NK, and NC revised the manuscript. WP conceived the idea and designed research outline. All authors contributed to the article and approved the submitted version.
Funding
The authors would like to acknowledge funding support from the Development and Promotion of Science and Technology Talents Project (DPST), Ministry of Education, Thailand, and Canada-ASEAN Scholarships and Educational Exchanges for Development (SEED) program, supported by the Government of Canada. This work was also partially supported by the Chiang Mai University.
Conflict of Interest
The authors declare that the research was conducted in the absence of any commercial or financial relationships that could be construed as a potential conflict of interest.
Acknowledgments
We thank to Dr. Narin Printarakul, Department of Biology, Faculty of Science, Chiang Mai University for bryophytes identification.
Supplementary Material
The Supplementary Material for this article can be found online at: https://www.frontiersin.org/articles/10.3389/fmicb.2020.563047/full#supplementary-material
Footnotes
References
Alain, K., and Querllou, J. (2009). Cultivating the uncultured: limits, advances and future challenges. Extremophiles 13, 583–594. doi: 10.1007/s00792-009-0261-3
Andrews, S. (2010). FastQC: A Quality Control Tool for High Throughput Sequence Data. Available at: http://www.bioinformatics.babraham.ac.uk/projects/fastqc (accessed March 2, 2020).
Arnow, L. E. (1937). Colorimetric determination of the components of 3,4-di hydroxyphenylalanine-tyrosine mixtures. J. Biol. Chem. 118, 531–537.
Atkin, C. L., Neilands, J. B., and Phaff, H. J. (1970). Rhodotorulic acid from species of Leucosporidium, Rhodosporidium, Rhodotorula, Sporidioholus and Sporoholomyces, and a new alanine-containing ferrichrome from Cryptococcus melibiosum. J. Bacteriol. 103, 722–733. doi: 10.1128/jb.103.3.722-733.1970
Aziz, R. K., Bartels, D., Best, A. A., Matthew, D., Terrence, D., Robert, A. E., et al. (2008). The RAST server: rapid annotations using subsystems technology. BMC Genomics 9:75. doi: 10.1186/1471-2164-9-75
Benito, P., Alonso-Vega, P., Aguado, C., Luján, R., Anzai, Y., Hirsch, A. M., et al. (2017). Monitoring the colonization and infection of legume nodules by Micromonospora in co-inoculation experiments with rhizobia. Sci. Rep. 7:11051. doi: 10.1038/s41598-017-11428-1
Bulgarelli, D., Schlaeppi, K., Spaepen, S., Ver, E., Themaat, L., and Schulze-lefert, P. (2013). Structure and functions of the bacterial microbiota of plants. Annu. Rev. Plant Biol. 64, 807–838. doi: 10.1146/annurev-arplant-050312-120106
Carro, L., Nouioui, I., Sangal, V., Meier-Kolthoff, J. P., Nevzat, S., Darren, L. S., et al. (2018a). Genome-based classification of micromonosporae with a focus on their biotechnological and ecological potential. Sci. Rep. 8:525. doi: 10.1038/s41598-017-17392-0
Carro, L., Pujic, P., Trujillo, M. E., and Normand, P. (2013). Micromonospora is a normal occupant of actinorhizal nodules. J. Biosci. 38, 685–693. doi: 10.1007/s12038-013-9359-y
Carro, L., Spröer, C., Alonso, P., and Trujillo, M. E. (2012). Diversity of Micromonospora strains isolated from nitrogen fixing nodules and rhizosphere of Pisum sativum analyzed by multilocus sequence analysis. Syst. Appl. Microbiol. 35, 73–80. doi: 10.1016/j.syapm.2011.11.003
Carro, L., Veyisoglu, A., Riesco, R., Sproer, C., Klenk, H.-P., Sahin, N., et al. (2018b). Micromonospora phytophila sp. nov. and Micromonospora luteiviridis sp. nov., isolated as natural inhabitants of plant nodules. Int. J. Syst. Evol. Microbiol. 68, 248–253. doi: 10.1099/ijsem.0.002490
Ceapã, C. D., Vázquez-Hernández, M., Rodríguez-Luna, S. D., Cruz Vázquez, A. P., Jiménez Suárez, V., Romina, R. S., et al. (2018). Genome mining of Streptomyces scabrisporus NF3 reveals symbiotic features including genes related to plant interactions. PLoS One 13:e0192618. doi: 10.1371/journal.pone.0192618
Chandra, R. (2014). Potential of bryophytes as therapeutics. Int. J. Pharm. Sci. Res. 5, 3584–3593. doi: 10.13040/IJPSR.0975-8232.5(9).3584-93
Chandra, S., and Bandopadhyay, R. (2010). Acclimatization of tissue cultured plantlets: from laboratory to land. Biotechnol. Lett. 32, 1199–1205. doi: 10.1007/s10529-010-0290-0
Chappelle, E. W., Kin, M. S., and Mcmurtrey, J. E. (1992). Radio analysis of reflectance spectra (RARS): an algorithm for the remote estimation of the concentrations of chlorophyll a, chlorophyll b and carotenoids in soybean leaves. Remote Sens. Environ. 39, 239–247. doi: 10.1016/0034-4257(92)90089-3
Chun, J., Oren, A., Ventosa, A., Christensen, H., Arahal, D. R., da Costa, M. S., et al. (2018). Proposed minimal standards for the use of genome data for the taxonomy of prokaryotes. Int. J. Syst. Evol. Microbiol. 68, 461–466. doi: 10.1099/ijsem.0.002516
Collins, M. D., Pirouz, T., Goodfellow, M., and Minnikin, D. E. (1977). Distribution of menaquinones in actinomycetes and corynebacteria. J. Gen. Microbiol. 100, 221–230. doi: 10.1099/00221287-100-2-221
Della Mónica, I. F., Novas, M. V., Iannone, L. J., Querejeta, G., Scervino, J. M., Pitta Alvarez, S. I., et al. (2018). Infection with Micromonospora strain SB3 promotes in vitro growth of Lolium multiflorum plantlets. Plant Cell Tissue Organ Cult. 134, 445–455. doi: 10.1007/s11240-018-1434-5
Di, D., Zhang, C., Luo, P., An, C. W., and Guo, G. Q. (2016). The biosynthesis of auxin: How many paths truly lead to IAA? Plant Growth Regul. 78, 275–285. doi: 10.1007/s10725-015-0103-5
Eevers, N., Gielen, M., Sánchez-López, A., Jaspers, S., White, J. C., Vangronsveld, J., et al. (2015). Optimization of isolation and cultivation of bacterial endophytes through addition of plant extract to nutrient media. Microb. Biotechnol. 8, 707–715. doi: 10.1111/1751-7915.12291
Elbein, A. D., Pan, Y. T., Pastuszak, I., and Carroll, D. (2003). New insight on trehalose: a multifunctional molecule. Glycobiology 13, 17R–27R. doi: 10.1093/glycob/cwg047
El-Tarabily, K. A., Alkhajeh, A. S., Ayyash, M. M., and Fuentes-ramírez, L. E. (2019). Growth promotion of Salicornia bigelovii by Micromonospora chalcea acid deaminase producing actinobacterial isolate. Front. Microbiol. 10:1694. doi: 10.3389/fmicb.2019.01694
Etesami, H., Alikhani, H. A., and Hosseini, H. M. (2015). Indole-3-acetic acid (IAA) production trait, a useful screening to select endophytic and rhizosphere competent bacteria for rice growth promoting agents. MethodsX 2, 72–78. doi: 10.1016/j.mex.2015.02.008
Farris, J. S. (1972). Estimating phylogenetic trees from distance matrices. Am. Nat. 106, 645–667. doi: 10.1086/282802
Gabriele, M. O. (2016). Bioprospecting plant-associated microbiomes. J. Biotechnol. 235, 171–180. doi: 10.1016/j.jbiotec.2016.03.033
Genilloud, O. (2015). “Micromonospora,” in Bergey’s Manual of Systematics of Archaea and Bacteria, eds W. B. Whitman, F. Rainey, P. Kämpfer, M. Trujillo, and J. P. Chun, (Hoboken, NJ: Wiely). doi: 10.1002/9781118960608.gbm00148
Glickman, E., and Dessaux, Y. (1995). A critical examination of the specificity of the Salkowski reagent for indolic compounds produced by phytopathogenic bacteria. Appl. Environ. Microbiol. 61, 793–796. doi: 10.1128/aem.61.2.793-796.1995
Gupta, A., Gopal, M., Thomas, G. V., Manikandan, V., Gajewski, J., Thomas, G., et al. (2014). Whole genome sequencing and analysis of plant growth promoting bacteria isolate d from the rhizosphere of plantation crops coconut, cocoa and arecanut. PLoS One 9:e104259. doi: 10.1371/journal.pone.0104259
Hallsworth, J. E., Nomura, Y., and Iwahara, M. (1998). Ethanol-induced water stress and fungal growth. J. Biosci. Bioeng. 86, 451–456. doi: 10.1016/s0922-338x(98)80150-5
Hamedi, J., and Mohammadipanah, F. (2014). Biotechnological application and taxonomical distribution of plant growth promoting actinobacteria. J. Ind. Microbiol. Biotechnol. 42, 157–171. doi: 10.1007/s10295-014-1537-x
Hattori, S., Thaithong, O., and Kitagawa, N. (1977). The genus Frullania in Thailand. J. Hattori Bot. Lab. 43, 439–457.
Hayakawa, M., and Nonomura, H. (1987). Humic acid-vitamin Agar, a new medium for selective isolation of soil actinomycetes. J. Ferment. Technol. 65, 501–509. doi: 10.1016/0385-6380(87)90108-7
He, X., Sun, Y., and Zhu, R. (2013). The oil bodies of liverworts: unique and important organelles in land plants. Crit. Rev. Plant Sci. 32, 293–302. doi: 10.1080/07352689.2013.765765
Hieronymus, G. (1892). Beitrage Zur Morphologie und Biologie der Algen. Beitr. Biol. P?anz. 5, 461–495.
Hirsch, A. M., and Valdes, M. (2010). Micromonospora: an important microbe for biomedicine and potentially for biocontrol and biofuels. Soil Biol. Biochem. 42, 536–542. doi: 10.1016/j.soilbio.2009.11.023
Hoagland, D. R., and Arnon, D. I. (1950). The Water-Culture Method FOR Growing Plants Without Soil. Berkeley, CA: University of California.
Jahanshah, G., Miess, H., Busche, T., Rückert, C., Kalinowski, J., Rokni-Zadeh, H., et al. (2019). Draft genome sequence of Micromonospora sp. strain MW-13, a bacterial strain with antibacterial properties and plant growth promotion potential isolated from the rhizosphere of wheat in Iran. Microbiol. Resour. Announc. 8:e01375-18. doi: 10.1128/MRA.01375-18
Janssen, P. H., Yates, P. S., Grinton, B. E., Taylor, P. M., and Sait, M. (2002). Improved culturability of soil bacteria and isolation in pure culture of novel members of the divisions Acidobacteria, Actinobacteria, Proteobacteria, and Verrucomicrobia. Appl. Environ. Microbiol. 68, 2391–2396. doi: 10.1128/aem.68.5.2391-2396.2002
Jones, K. L. (1949). Fresh isolates of actinomycetes in which the presence of sporogenous aerial mycelia is a fluctuating characteristic. J. Bacteriol. 57, 141–145. doi: 10.1128/jb.57.2.141-145.1949
Kaewkla, O., Thamchaipinet, A., and Franco, C. M. M. (2017). Micromonospora terminaliae sp. nov., an endophytic actinobacterium isolated from the surface-sterilized stem of the medicinal plant Terminalia mucronate. Int. J. Syst. Evol. Microbiol. 67, 225–230. doi: 10.1099/ijsem.0.001600
Kappes, R. M., Kempf, B., and Bremer, E. (1996). Three transport systems for the osmoprotectant glycine betaine operate in Bacillus subtilis: characterization of OpuD. J. Bacteriol. 178, 5071–5079. doi: 10.1128/jb.178.17.5071-5079.1996
Kaul, S., Sharma, T., and Dhar, M. K. (2016). “Omics” tools for better understanding the plant–endophyte interactions. Front. Plant Sci. 7:955. doi: 10.3389/fpls.2016.00955
Kelly, K. L. (1964). Inter-Society Color Council - National Bureau of Standards Color Name Charts Illustrated with Centroid Colors. Washington, DC: US Government Printing Office.
Khare, E., Mishra, J., and Arora, N. K. (2018). Multifaceted interactions between endophytes and plant: developments and prospects. Front. Microbiol. 9:2732. doi: 10.3389/fmicb.2018.02732
Kim, M., Lee, Y. K., Lee, H. K., and Hana, I. M. (2007). Characterization of cold-shock protein A of Antarctic Streptomyces sp. AA8321. Protein J. 26, 51–59. doi: 10.1007/s10930-006-9044-1
King, E. O., Ward, M. K., and Rangey, D. E. (1954). Two simple media for demonstrate of pyocyanin and fluorescein. J. Lab. Clin. Med. 44, 301–307.
Kittiwongwattana, C., Thanaboripat, D., Laosinwattana, C., Koohakan, P., Parinthawong, N., and Thawai, C. (2015). Micromonospora oryzae sp. nov., isolated from roots of upland rice. Int. J. Syst. Evol. Microbiol. 65, 3818–3823. doi: 10.1099/ijsem.0.000500
Krueger, F. (2015). Trim Galore: A Wrapper Tool Around Cutadapt and FastQC to Consistently Apply Quality and Adapter Trimming to FastQ Files. Available at: https://www.bioinformatics.babraham.ac.uk/projects/trim_galore/ (accessed March 2, 2020).
Kudo, T., Matsushima, K., Itoh, T., Sasaki, J., and Suzuki, K. (1998). Description of four new species of the genus Kineosporia: Kineosporia succinea sp. nov., Kineosporiarhizophila sp. nov., Kineosporia mikuniensis sp. nov. and Kineosporia rhamnosa sp. nov., isolated from plant samples, and amended description of the genus Kineosporia. Int. J. Syst. Bacteriol. 48, 1245–1255. doi: 10.1099/00207713-48-4-1245
Kumar, S., Stecher, G., and Tamura, K. (2016). MEGA7: Molecular evolutionary genetics analysis version 7.0 for bigger datasets. Mol. Biol. Evol. 33, 1870–1874. doi: 10.1093/molbev/msw054
Kuncharoen, N., Pittayakhajonwut, P., and Tanasupawat, S. (2018). Micromonospora globbae sp. nov., an endophytic actinomycete isolated from roots of Globba winitii C. H. Wright. Int. J. Syst. Evol. Microbiol. 68, 1073–1077. doi: 10.1099/ijsem.0.002625
Küster, E., and Williams, S. T. (1964). Selection of media for isolation of streptomycetes. Nature 202, 928–929. doi: 10.1038/202928a0
Lechevalier, H. A., Lechevalier, M. P., and Gerber, N. N. (1971). Chemical composition as a criterion in the classification of actinomycetes. Adv. Appl. Microbiol. 14, 47–72. doi: 10.1016/s0065-2164(08)70539-2
Lechevalier, M. P., De Bièvre, C., and Lechevalier, H. A. (1977). Chemotaxonomy of aerobic actinomycetes: phospholipid composition. Biochem. Syst. Ecol. 5, 249–260. doi: 10.1016/0305-1978(77)90021-7
Lee, L. H., Zainal, N., Azman, A. S., Eng, S. K., Ab Mutalib, N. S., Yin, W. F., et al. (2014). Streptomyces pluripotens sp. nov., a bacteriocin-producing streptomycete that inhibits meticillin-resistant Staphylococcus aureus. Int. J. Syst. Evol. Microbiol. 64, 3297–3306. doi: 10.1099/ijs.0.065045-0
Lefort, V., Desper, R., and Gascuel, O. (2015). FastME 2.0: a comprehensive, accurate, and fast distance-based phylogeny inference program. Mol. Biol. Evol. 32, 2798–2800. doi: 10.1093/molbev/msv150
Li, C., Jin, P., Liu, C., Ma, Z., Zhao, J., Li, J., et al. (2016). Streptomyces bryophytorum sp. nov., an endophytic actinomycete isolated from moss (Bryophyta). Antonie Van Leeuwenhoek 109, 1209–1215. doi: 10.1007/s10482-016-0722-5
Li, C., Wang, H., Jin, P., Zheng, W., Chu, L., Liu, C., et al. (2015a). Actinoallomurus bryophytorum sp. nov., an endophytic actinomycete isolated from moss (Bryophyta). Antonie van Leeuwenhoek 108, 453–459. doi: 10.1007/s10482-015-0498-z
Li, C., Zhang, Y., and Liu, C. (2015b). Microbispora bryophytorum sp. nov., an actinomycete isolated from moss (Bryophyta). Int. J. Syst. Evol. Microbiol. 65, 1274–1279. doi: 10.1099/ijs.0.000095
Li, X. J., He, S., and Zang, M. (2007). Moss Flora of China, Vol. 4. St. Louis, MO: Missouri Botanical Garden Press.
Liu, D., Yan, R., Fu, Y., Wang, X., Zhang, J., and Xiang, W. (2019). Antifungal, plant growth-promoting, and genomic properties of an endophytic actinobacterium Streptomyces sp. NEAU-S7GS2. Front. Microbiol. 10:2077. doi: 10.3389/fmicb.2019.02077
Meier-Kolthoff, J. P., Auch, A. F., Klenk, H. P., and Göker, M. (2013). Genome sequence- based species delimitation with confidence intervals and improved distance functions. BMC Bioinformatics 14:60. doi: 10.1186/1471-2105-14-60
Minnikin, D. E., O’Donnell, A. G., Goodfellow, M., Alderson, G., Athalye, M., Schaal, A., et al. (1984). An integrated procedure for the extraction of bacterial isoprenoid quinones and polar lipids. J. Microbiol. Methods 2, 233–241. doi: 10.1016/0167-7012(84)90018-6
Nautiyal, C. S. (1999). An efficient microbiological growth medium for screening phosphate solubilizing microorganisms. FEMS Microbiol. Lett. 170, 265–270. doi: 10.1111/j.1574-6968.1999.tb13383.x
Neilands, J. B., and Leong, S. A. (1986). Siderophores in relation to plant growth and disease. Annu. Rev. Plant Physiol. 37, 187–208. doi: 10.1146/annurev.pp.37.060186.001155
Nouioui, I., Carro, L., García-López, M., Meier-Kolthoff, J. P., Woyke, T., Nikos, C. K., et al. (2018). Genome-based taxonomic classification of the phylum Actinobacteria. Front. Microbiol. 9:2007. doi: 10.3389/fmicb.2018.02007
Nouioui, I., Cortés-albayay, C., Carro, L., Castro, J. F., Gtari, M., Ghodhbane-Gtari, F., et al. (2019a). Genomic insights into plant-growth-promoting potentialities of the genus Frankia. Front. Microbiol. 10:1457. doi: 10.3389/fmicb.2019.01457
Nouioui, I., Sangal, V., Cortes-Albayay, C., Jando, M., Igual, J. M., Klenk, H.-P., et al. (2019b). Mycolicibacterium stellerae sp. nov., a rapidly growing scotochromogenic strain isolated from Stellera chamaejasme. Int. J. Syst. Evol. Microbiol. 69, 3465–3471. doi: 10.1099/ijsem.o.003644
Opelt, K., Berg, C., and Berg, G. (2007). The bryophyte genus Sphagnum is a reservoir for powerful and extraordinary antagonists and potentially facultative human pathogens. FEMS Microbiol. Ecol. 61, 38–53. doi: 10.1111/j.1574-6941.2007.00323.x
Ørskov, J. (1923). Investigations into the Morphology of the Ray Fungi. Copenhagen: Levin & Munksgaard, 165–171.
Ortúzar, M., Trujillo, M. E., Román-Ponce, B., and Carro, L. (2020). Micromonospora metallophores: a plant growth promotion trait useful for bacterial-assisted phytoremediation? Sci. Total Environ. 739:139850. doi: 10.1016/j.scitotenv.2020.139850
Overbeek, R., Olson, R., Pusch, G. D., Olsen, G. J., Davis, J. J., Disz, T., et al. (2014). The SEED and the rapid annotation of microbial genomes using subsystems technology (RAST). Nucleic Acids Res. 42, D206–D214. doi: 10.1093/nar/gkt1226
Printarakul, N., Tan, B. C., Wongkuna-Thananoppakun, K., and Santanachote, K. (2014). The Indian connection of the Thailand moss flora, with one new species, Fissidens elizbrowniae. Telopea 17, 195–215. doi: 10.7751/telopea20147808
Ritcher, M., and Rosselló-Móra, R. (2009). Shifting the genomics gold standard for the prokaryotic species definition. Proc. Natl. Acad. Sci. U.S.A. 106, 19126–19131. doi: 10.1073/pnas.0906412106
Ritcher, M., Rosselló-Móra, R., Oliver Glöckner, F., and Peplies, J. (2016). JspeciesWS: a web server for prokaryotic species circumscription based on pairwise genome comparison. Bioinformatics 32, 929–931. doi: 10.1093/bioinformatics/btv681
Ruttanasutja, P., and Pathom-aree, W. (2015). Selective isolation of cultivable actinomycetes from Thai coastal marine sediment. Chiang Mai J. Sci. 42, 88–103.
Schumann, W. (2016). Regulation of bacterial heat shock stimulons. Cell Stress Chaperones 21, 959–968. doi: 10.1007/s12192-016-0727-z
Schwyn, B., and Neilands, J. B. (1987). Universal chemical assay for the detection and determination of siderophores. Anal. Biochem. 160, 47–56. doi: 10.1016/0003-2697(87)90612-9
Seemann, T. (2014). Prokka: rapid prokaryotic genome annotation. Bioinformatics 30, 2068–2069. doi: 10.1093/bioinformatics/btu153
Shirling, E., and Gottlieb, D. (1966). Methods for characterization of Streptomyces species. Int. J. Syst. Bact. 16, 313–340. doi: 10.1099/00207713-16-3-313
Spaepen, S., and Vanderleyden, J. (2011). Auxin and plant-microbe interactions. Cold Spring Harb. Perspect. Biol. 3:a001438. doi: 10.1101/cshperspect.a001438
Spiess, L., Lippincott, B., and Lippincott, J. (1981). Bacteria isolated from moss and their effect on moss development. Bot. Gaz. 142, 512–518. doi: 10.1086/337253
Staneck, J. L., and Robert, G. D. (1974). Simplified approach to identification of aerobic actinomycetes by thin-layer chromatography. Appl. Microbiol. 28, 226–231. doi: 10.1128/aem.28.2.226-231.1974
Sundberg, S., Hylander, K., Johansson, V., and Niklas, L. (2014). Release thresholds for moss spores: the importance of turbulence and sporophyte length. J. Ecol. 102, 721–729. doi: 10.1111/1365-2745.12245
Thawai, C. (2015). Micromonospora costi sp. nov., isolated from a leaf of Costus speciosus. Int. J. Syst. Evol. Microbiol. 65, 1456–1461. doi: 10.1099/ijs.0.000120
Thelander, M., Landberg, K., and Sundberg, E. (2018). Auxin-mediated developmental control in the moss Physcomitrella patens. J. Exp. Bot. 69, 277–290. doi: 10.1093/jxb/erx255
Trujillo, M. E., Alonso-Vega, P., Rodríguez, R., Carro, L., Cerda, E., Alonso, P., et al. (2010). The genus Micromonospora is widespread in legume root nodules: the example of Lupinus angustifolius. ISME J. 4, 1265–1281. doi: 10.1038/ismej.2010.55
Trujillo, M. E., Bacigalupe, R., Pujic, P., Igarashi, Y., Benito, P., Riesco, R., et al. (2014). Genome features of the endophytic actinobacterium Micromonospora lupini strain Lupac 08: On the process of adaptation to an endophytic life style? PLoS One 9:e108522. doi: 10.1371/journal.pone.0108522
Trujillo, M. E., Riesco, R., Benito, P., and Carro, L. (2015). Endophytic actinobacteria and the interaction of Micromonospora and nitrogen fixing plants. Front. Microbiol. 6:1341. doi: 10.3389/fmicb.2015.01341
Wang, D.-S., Xue, Q. H., Ma, Y.-Y., Wei, X.-L., Chen, J., and He, F. (2014). Oligotrophy is helpful for the isolation of bioactive actinomycetes. Indian J. Microbiol. 54, 178–184. doi: 10.1007/s12088-014-0444-1
Wang, W., Qiu, Z., and Tan, H. (2014). Siderophore production by actinobacteria. Biometals 27, 623–631. doi: 10.1007/s10534-014-9739-2
Wang, Z., Solanki, M. K., Yu, Z. X., Yang, L. T., An, Q. L., Dong, D. F., et al. (2019). Draft genome analysis offers insights into the mechanism by which Streptomyces chartreusis WZS021 increases drought tolerance in sugarcane. Front. Microbiol. 9:3262. doi: 10.3389/fmicb.2018.03262
Wick, R. R., Judd, L. M., Gorrie, C. L., and Holt, K. E. (2017). Unicycler: resolving bacterial genome assemblies from short and long sequencing reads. PLoS Comput. Biol. 13:e1005595. doi: 10.1371/journal.pcbi.1005595
Wickert, E., Marcondes, J., Lemos, M. V., and Lemos, E. G. M. (2007). Nitrogen assimilation in Citrus based on CitEST data mining. Genet. Mol. Biol. 30, 810–818. doi: 10.1590/S1415-47572007000500009
Yadav, A. N., Verma, P., Kumar, S., Kumar, V., Kumar, M., and Chellammal, T. (2018). “Actinobacteria from rhizosphere: molecular diversity, distributions, and potential biotechnological applications,” in New and Future Developments in Microbial Biotechnology and Bioengineering, eds B. P. Singh, V. K. Gupta, and A. K. Passari, (Amsterdam: Elsevier), 13–41. doi: 10.1016/B978-0-444-63994-3.00002-3
Keywords: actinobacteria, bryophytes, Physcomitrium sphaericum, plant-microbes interaction, plant-growth promoting bacteria, genome mining, draft genome, whole genome sequencing
Citation: Insuk C, Kuncharoen N, Cheeptham N, Tanasupawat S and Pathom-aree W (2020) Bryophytes Harbor Cultivable Actinobacteria With Plant Growth Promoting Potential. Front. Microbiol. 11:563047. doi: 10.3389/fmicb.2020.563047
Received: 17 May 2020; Accepted: 24 August 2020;
Published: 29 September 2020.
Edited by:
Andrea Genre, University of Turin, ItalyReviewed by:
Lorena Carro, Newcastle University, United KingdomManoj Kumar Solanki, Agricultural Research Organization (ARO), Israel
Joachim Wink, Helmholtz Association of German Research Centers (HZ), Germany
Copyright © 2020 Insuk, Kuncharoen, Cheeptham, Tanasupawat and Pathom-aree. This is an open-access article distributed under the terms of the Creative Commons Attribution License (CC BY). The use, distribution or reproduction in other forums is permitted, provided the original author(s) and the copyright owner(s) are credited and that the original publication in this journal is cited, in accordance with accepted academic practice. No use, distribution or reproduction is permitted which does not comply with these terms.
*Correspondence: Wasu Pathom-aree, d2FzdS5wQGNtdS5hYy50aA==; d2FzdTIxNTc5M0BnbWFpbC5jb20=