- 1Section Geomicrobiology, GFZ German Research Centre for Geosciences, Potsdam, Germany
- 2Institute of Geosciences, University of Potsdam, Potsdam, Germany
- 3Section Organic Geochemistry, GFZ German Research Centre for Geosciences, Potsdam, Germany
- 4Section Applied Geochemistry, Institute of Applied Geosciences, Technische Universität Berlin, Berlin, Germany
- 5Section Climate Dynamics and Landscape Evolution, GFZ German Research Centre for Geosciences, Potsdam, Germany
The Hartoušov mofette system is a natural CO2 degassing site in the central Cheb Basin (Eger Rift, Central Europe). In early 2016 a 108 m deep core was obtained from this system to investigate the impact of ascending mantle-derived CO2 on indigenous deep microbial communities and their surrounding life habitat. During drilling, a CO2 blow out occurred at a depth of 78.5 meter below surface (mbs) suggesting a CO2 reservoir associated with a deep low-permeable CO2-saturated saline aquifer at the transition from Early Miocene terrestrial to lacustrine sediments. Past microbial communities were investigated by hopanoids and glycerol dialkyl glycerol tetraethers (GDGTs) reflecting the environmental conditions during the time of deposition rather than showing a signal of the current deep biosphere. The composition and distribution of the deep microbial community potentially stimulated by the upward migration of CO2 starting during Mid Pleistocene time was investigated by intact polar lipids (IPLs), quantitative polymerase chain reaction (qPCR), and deoxyribonucleic acid (DNA) analysis. The deep biosphere is characterized by microorganisms that are linked to the distribution and migration of the ascending CO2-saturated groundwater and the availability of organic matter instead of being linked to single lithological units of the investigated rock profile. Our findings revealed high relative abundances of common soil and water bacteria, in particular the facultative, anaerobic and potential iron-oxidizing Acidovorax and other members of the family Comamonadaceae across the whole recovered core. The results also highlighted the frequent detection of the putative sulfate-oxidizing and CO2-fixating genus Sulfuricurvum at certain depths. A set of new IPLs are suggested to be indicative for microorganisms associated to CO2 accumulation in the mofette system.
Introduction
The Hartoušov mofette system is located in the center of the Cheb Basin (Eger Rift) at the central part of the Počatky-Plesná Fault Zone (PPZ) (Bankwitz et al., 2003a,b; Flechsig et al., 2008, 2010; Nickschick et al., 2019; Figure 1). The region is known for periodically occurring earthquake swarms and widely distributed natural cold gas exhalation systems in form of mofette sites and mineral water springs releasing CO2-rich gas into the atmosphere (Fischer et al., 2014). The CO2 originates from active magma chambers at the crust-mantle boundary and from lithospheric mantle depths of about 65 km (Heuer et al., 2006; Bräuer et al., 2009). The CO2 preferentially migrates to the surface as component of supercritical fluids in the lower crust or either dissolved in water or as a free gas phase along deep-seated faults in the upper crust (Weinlich et al., 1999; Weise et al., 2001; Bräuer et al., 2011; Kämpf et al., 2019). The PPZ started to develop at the boundary from Mid to Late Pleistocene (Bankwitz et al., 2003a,b). CO2-rich nephelinitic magma (Seifert and Kämpf, 1994; Geissler et al., 2007; Brandl et al., 2015) erupted in the Mid Pleistocene (Mrlina et al., 2007; Rohrmüller et al., 2018; Krmíček et al., 2020; Lied et al., 2020). Age determinations of the hydrothermal activity of Karlovy Vary spa with travertine deposits go back to 0.23 Ma (Vylita et al., 2007). The age of the Hartoušov mofette system is unknown so far, but in this study assigned to the onset of PPZ development and the occurrence of volcanism. Previous investigations from sediments of the upper 9 m at the Hartoušov mofette system revealed that ascending CO2-containing fluids cause sediment fluidization, hydrofracturing, and geochemical alterations e.g., sediment bleaching, mobilization of metals and the preservation of organic matter (Flechsig et al., 2008; Rennert et al., 2011; Mehlhorn et al., 2016, 2018; Rennert and Pfanz, 2016; Bussert et al., 2017; Liu et al., 2018). At the surface, CO2 exhalation occurs in form of diffuse degassing structures (DDS, namely dry mofettes) and localized water filled, pool-like structures (wet mofettes) (Flechsig et al., 2008; Kämpf et al., 2013, 2019; Nickschick et al., 2015, 2017).
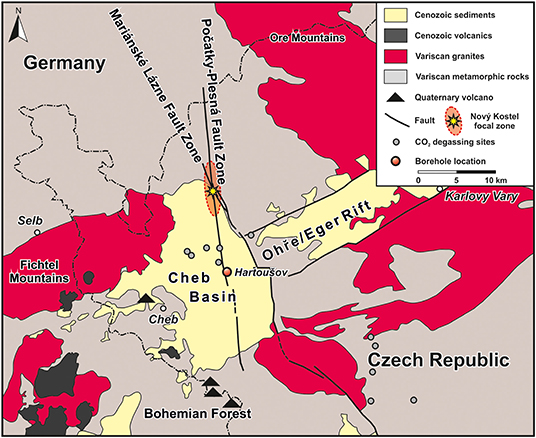
Figure 1. Map of the study site in NW Czech Republic showing the position of the Hartoušov mofette system in the center of the Cheb Basin that evolved at the intersection of the Eger Rift and Regensburg-Leipzig-Rostock Fault Zone, here represented by the Mariánské Lázně and the Počatky-Plesná Fault Zones (modified after Liu et al., 2018).
Dry mofette areas display high CO2 soil gas concentrations, low soil pH, accelerated silicate weathering, leaching of base cations, anomalous vegetation patterns, low taxonomic, and functional biodiversity of soil biota and a limited microbial degradation of soil organic matter (SOM) (Rennert et al., 2011; Hohberg et al., 2015; Beulig et al., 2016; Rennert and Pfanz, 2016; Kämpf et al., 2019). In comparison to reference sites, the microbial community differs in composition and is dominated by anaerobic chemolithoautotrophic microorganisms, e.g., acidophilic, methanogenic, and sulfur-cycling organisms (Beulig et al., 2015, 2016; Liu et al., 2018). Additionally, a higher microbial abundance was observed even in deeper parts of the sedimentological profile (Liu et al., 2018) and estimates for microbial fixation of ascending geogenic CO2 reach up to 27% of the total SOM (Nowak et al., 2015). Similar characteristics were also found at other mofette sites, e.g., the Laacher See in Germany (Krüger et al., 2009; Frerichs et al., 2013), the Latera caldera in Italy (Oppermann et al., 2010), and the Stavešinci mofette in Slovenia (Šibanc et al., 2014).
Hydrogeochemical investigations of waters from wet mofettes and mineral springs in the Cheb Basin by Krauze et al. (2017) and in the adjacent areas (Wagner et al., 2007; Schuessler et al., 2016) unraveled different water sources, with surface water at some locations being co-sourced by a deep saline aquifer. The microbial communities in all of these CO2-influenced waters were generally dominated by chemolithoautotrophic microorganisms (iron- and sulfur-cycling organisms) and methanogenic archaea. Similar to dry mofettes, the microbial degradation of complex dissolved organic carbon (DOC) is also restricted in these anaerobic environments (Krauze et al., 2017), suggesting that upstreaming CO2 is also one of the main carbon sources for microorganisms. The connection to a deep saline aquifer at some mofettes (e.g., Bublak, ~1.5 km NNE of the Hartoušov mofette) was indicated by the occurrence of specific microorganisms from the deep subsurface biosphere and marine paleoenvironments pointing to a widespread deep saline aquifer as a general deep microbial habitat in this region (Krauze et al., 2017).
In addition, other deep biosphere habitats may exist that are associated with CO2 reservoirs in geological trapping structures, as indicated by an increase in gas flow rates after swarm earthquakes pointing to a gas release after seismically induced fracking of sealing layers (Sandig et al., 2014; Sauer et al., 2014; Schuessler et al., 2016; Fischer et al., 2017). Additionally, Kämpf and Bankwitz (2005) described dm- to m-sized cavities in sediments of the nearby Nová Ves II open-cast mine at 50 mbs (meter below surface), which developed along fluid migration pathways. This suggests the presence of restricted gas-filled cavities, which may function as distinct habitats for the deep biosphere. An important indication for a CO2-related deep biosphere was recognized by Bräuer et al. (2005) after a swarm earthquake activity in 2000. They detected an increase in methane concentrations at the Wettin spring (Bad Brambach, Germany) about 20 km north of the Hartoušov mofette system, where a significant decrease of δ13Cmethane was attributed to microbial methane production from magmatic CO2 and pre- or co-seismically released hydrogen from the granitic basement. After a swarm earthquake event in 2011, higher methane concentrations were also detected at the Bublak mofette (Bräuer et al., 2018).
These previous investigations show that ascending geogenic CO2-containing fluids locally alter the sedimentary overburden and thus change the environmental conditions for microbial life. Additionally, there is evidence of subsurface structures that may host CO2-influenced deep microbial habitats, which could function as deep microbial hotspots. However, studies investigating the potential for CO2-related deep microbial life in the Cheb Basin and the Eger Rift are still missing. Thus, in early 2016 the German Research Centre for Geosciences (GFZ) drilled a 108.5 m deep borehole as a test case for the International Continental Scientific Drilling Program (ICDP) project “Drilling the Eger Rift” (Dahm et al., 2013). The borehole was positioned in the Hartoušov mofette system (HJB-1) (50°07′58″N, 12°27′46″E) and described in detail by Bussert et al. (2017). During drilling, CO2-rich sediments were recovered between 71 and 81 mbs. At a depth of 78.5 mbs a CO2 blow out occurred, suggesting the presence of a subsurface CO2 accumulation. This CO2 reservoir is associated to a basal low-permeable CO2-saturated and saline aquifer (1,892 mg L−1 of free dissolved CO2) that occurs between 79 and 85 mbs at the transition from Early Miocene terrestrial to overlying lacustrine sediments. Hydrogeochemically, the aquifer is characterized by a Na-Ca-HCO3-SO4-type water with a high Fe content of up to 13.7 mg L−1 and a pH of 6.4 (Bussert et al., 2017). Due to the potential of the CO2-saturated aquifer to host a very specialized microbial community we focussed on the core interval between 65 and 95 mbs. Our aim was to identify the impact of mantle-derived CO2 on deep microbial communities and to find out whether the low-permeable CO2-saturated and saline aquifer might act as a hotspot for present deep microbial life. The methodological approach to characterize the microbial community included lipid biomarker analysis of past and living microbial biomass (hopanoids, GDGTs and intact polar lipids) as well as DNA analysis such as quantitative Polymerase Chain Reaction (qPCR) and Illumina 16S rRNA gene amplicon sequencing. Furthermore, the microbial signals were compared to lithological background information and sedimentological bulk parameters.
Methods
Drilling, Coring, and Pump Test
A detailed description of the field work including drilling, coring and a pump test was published by Bussert et al. (2017). The drilling was performed with a Drillmec G-25 device installed on a Tatra 815 drilling lorry which discovered core material in PVC liners with a length of 3 m and a diameter of 0.1 m. The drilling mud consisted of homogeneously blended pure bentonite. In order to monitor potential drill mud contamination of the retrieved core material, sodium fluorescein was added to the drill mud with a concentration of 5 mg L−1 (Supplementary Figure 1D) according to Pellizzari et al. (2013). Subsamples for further analysis were taken about every 0.5 m and stored in gasbags flushed with nitrogen at −80°C directly after core recovery in the field. After the drilling campaign a 24 h pump test within the deep low-permeable CO2-saturated saline aquifer was performed. The groundwater was filtered, the obtained water samples geochemically analyzed and the obtained filters stored at −20°C, respectively.
Sample Processing and Contamination Control
The initial lithological description of the sample material and the drill mud contamination control were performed in the lab. The frozen core segments were stored over night at 5°C to initiate thawing of the external sample layer and to avoid fluid migration from the rim to the center of the samples. The thawed rim (~1 cm) was removed (inner coring), the still frozen inner core described (e.g., Supplementary Figure 1), material from the removed rim (outer rim) tested in triplicates for fluorescein (Pellizzari et al., 2013) and the samples again stored at −80°C. To ensure that the samples are not contaminated by external DNA the inner coring technique was repeated in a clean bench (Thermo Scientific, Waltham, USA). The removed material and the outside of the inner core were again tested in triplicates for fluorescein (inner rim). Inner core samples (sample) exceeding the background fluorescence were excluded from further analysis (Supplementary Figure 1). The fluorescein concentration was measured with a CLARIO star® plate reader (BMG LABTECH GmbH, Ortenberg, Germany). The background fluorescence signal was obtained from samples of a shallow drilling campaign (3 m) drilled in 2015 adjacent to our study side without the application of drill mud and fluorescein (Liu et al., 2018).
Bulk Carbon and Nitrogen Analyses
Total carbon (TC), total organic carbon (TOC), total nitrogen (TN), and the bulk δ13Corg were all analyzed with the same equipment consisting of a NC2500 Carlo Erba elemental analyser coupled with a ConFlo_III interface on a DELTAplusXL isotope ratio mass spectrometer (IRMS) (Thermo Fischer Scientific). Prior to analysis the sample material was freeze-dried, powdered and homogenized. In order to determine the TC and TN ~25 mg of sample material was loaded into tin capsules and the content was calibrated against acetanilide. For investigation of TOC and bulk δ13Corg the carbonate content was removed using in situ decalcification. Therefore, depending on the TOC content, 3–10 mg sample material were loaded into Ag-capsules and decalcified by drops of 3% HCl followed by 20% HCl and heated for 3 h at 75°C. The calibration was performed using elemental urea and certified isotope standards (USGS24, IAEA-CH-7) and proofed with an internal soil reference sample (Boden3, HEKATECH). All isotope compositions are given relative to the VPDB (Vienna Pee Dee Belemnite) standard in the conventional delta notation. The total inorganic carbon (TIC) was calculated by subtraction of TOC from TC.
Lipid Biomarker Extraction and Chromatographic Column Separation
The freeze-dried, powdered and homogenized sediment samples (about 80 g) were extracted with a modified extraction method after Bligh and Dyer (1959) using methanol:dichloromethane (DCM):ammonium acetate buffer (pH 7.5) (2:1:0.8) as initial extraction solvent mixture. The sample material was admixed with the extraction solvent (4x sample mass in mL, ~320 mL), stirred with a flow-blending rod for 5 min and afterwards centrifuged for 10 min with 2,500 rpm. The supernatant was transferred to a separation funnel and the remaining sample 2 times re-extracted in an ultrasonic bath for 10 min, followed by centrifugation and transfer of the supernatant into the separation funnel. To achieve phase separation, the solvent ratio in the separation funnel was changed to 1:1:0.9 (methanol:DCM:ammonium acetate buffer). Afterwards the organic phase containing the lipid extract was collected in a turbovap glas and the solvent removed (TurboVap 500). Each fifth sample was a blank. After extraction 5α-Androstane and deuterium-labeled phosphatidylcholine (PCd54 = 1,2-dimyristoyl-d54-sn-glycero-3-phosphocholine) were added as standards for compound quantification in the aliphatic and intact polar lipid fractions, respectively. The obtained total extracts were chromatographically separated into a low polar lipid (20 mL chloroform), free fatty acid (50 mL methyl formiate with 0.025% glacial acetic acid), glycolipid (20 mL acetone), and intact polar lipid (IPLs, 25 mL methanol) fraction using two glass syringe columns filled with dried pure silica (1 g silica gel 63–200 μm, dried at 110°C for 2 h) and Florisil (1 g magnesium silica gel 150–250 μm) with the silica column on top of the Florisil column. The IPL fraction was only eluted from the silica column (Zink and Mangelsdorf, 2004). To improve IPL recovery the silica column was eluted with 25 mL methanol:water (60:40) for a second time. Phase separation was conducted as described above. Finally the IPL fractions were combined and the solvent removed. Afterwards, the IPL fraction was split into two halves: one for the direct detection of IPLs and one for the detection of polar lipid fatty acids (PLFAs) after saponification (Müller et al., 1993).
After removal of asphaltenes the low polar lipid fraction was further subdivided by Medium Pressure Liquid Chromatography (MPLC) into an aliphatic, aromatic, and Nitrogen-Sulfur-Oxygen-containing compound (NSO) fraction (Radke et al., 1980). The aliphatic fraction was analyzed for hopanoids and the NSO fraction for glycerol dialkyl glycerol tetraethers (GDGTs). GDGTs have been quantified with regard to an external archaeol standard.
Determination of the Lipid Biomarkers
Analysis of IPLs was performed on a Thermo Scientific Ultimate 3000 RS Ultra high performance liquid chromatograph (UHPLC) coupled to a Q Exactive Plus Orbitrap mass spectrometer (MS) with a heated electrospray (H-ESI II) probe. Samples were separated with a LiChrospher 100 diol column (2 × 125 mm, 5 μm; CS-Chromatographie Service) equipped with a pre-column filter. The eluents used for compound separation were (A) n-hexane:isopropanol:formic acid:ammonia (25% in water) 79:20:1.2:0.04 v/v and (B) isopropanol:water:formic acid:ammonia (25% in water) 88:10:1.2:0.04 v/v (solvent gradients: 1 min 100% A, linear increase of B to 65% within 20 and 40 min for reconditioning). The flow rate was set to 0.35 mL/min (modified after Rütters et al., 2001). ESI source conditions were as follows: spray voltage −2.2 kV; capillary temperature 300°C; nitrogen sheath gas at 49 and auxiliary gas at 12 arbitrary units at a temperature of 419°C, S-Lens 65 V. The obtained data were acquired in negative and positive ion mode with dependent MS/MS acquisition at ranges of m/z 400–2,000. The full scan and fragment spectra were collected at a resolution of 280,000 and 70,000 (at m/z 200), respectively.
The aliphatic fraction and PLFAs were determined on a Thermo Trace GC Ultra equipped with a Thermo PTV injection system and a SGE BPX5 fused silica capillary column (50 m length, 0.22 mm ID, 0.25 μm film thickness) coupled to a Thermo Trace DSQ Quadrupole MS. Helium was used as carrier gas. The temperature of the GC oven was programmed from 50°C (hold 1 min) to 310°C at a rate of 3°C min−1, followed by an isothermal phase of 30 min. The injector temperature was programmed from 50 to 300°C at a rate of 10°C s−1. The MS was operated in electron impact ionization mode (EI) at 70 eV. Full scan mass spectra for compound identification were recorded from m/z 50 to 600 at a scan rate of 1.5 scans s−1.
GDGT analysis was conducted on a Shimadzu LC10AD HPLC instrument coupled to a Finnigan Triple Stage Quadrupole (TSQ) 7000 MS with an atmospheric pressure chemical ionization (APCI) interface. Samples were separated at 30°C with a Prevail Cyano column (2.1 × 150 mm, 3 μm; Alltech) equipped with a pre-column filter. The mobile phase consisted of (A) n-hexane and (B) isopropanol and compound separation was achieved using the following solvent gradients: 5 min 99% A and 1% B, linear gradient to 1.8% B within 40 min, increase to 10% B within 1 min and holding time for 5 min to clean the column, back to initial solvent conditions within 1 min and 16 min for column equilibration (Schouten et al., 2007). The flow rate was set to 200 μL min−1. The APCI adjustments were: corona current 5 μA giving a voltage of around 5 kV, vaporizer temperature 350°C, capillary temperature 200°C and nitrogen sheath gas at 60 psi (no auxiliary gas). Mass spectra were generated by selected ion monitoring in the positive ion mode for the masses 1295.0, 1302.1, 1049.5, 1035.5, 1021.5, and 654.2 each with a width of 7 amu (to also obtain neighboring masses) representing major core GDGTs at a scan rate of 0.33 s.
Compound specific δ13C values of the aliphatic fraction (hopanoids) were determined with a GC-isotope ratio monitoring (IR)-MS system consisting of an Agilent 7890 GC (USA) connected with an open split GC-C/TCIII-Interface for compound-specific carbon and hydrogen isotope analysis to a Delta V Plus IRMS (Thermo Fischer Scientific, Germany). The GC-separated organic substances were oxidized to CO2 in a combustion furnace at a temperature of 940°C on a CuO/Ni/Pt catalyst. CO2 was transferred to the mass spectrometer to determine carbon isotope ratios. Three microliter of the aliphatic fraction were injected with a split ratio of 1:2 and an initial temperature of 230°C to a programmable temperature vaporization inlet (PTV, Agilent Technology, USA). The injector was heated to 300°C with a heating rate of 12°C s−1. The separation of the aliphatic fractions was attained by a fused silica capillary column (HP Ultra 1, 50 m × 0.2 mm ID, 0.33 μm FT, Agilent Technology, Germany) with a temperature program starting from 40 to 300°C, with a heating rate of 4°C min−1 and the maximum temperature held for 45 min. The carrier gas was Helium with a flow rate of 1.0 mL min−1. All samples were measured in triplicates with a usual standard deviation of ≤ 0.5%0. The quality of the results was checked by measuring n-alkane standards (n-C15, n-C20, and n-C25) with known isotopic composition (Campro Scientific, Germany). Isotopic compositions are given in the delta notation relative to the Vienna Pee Dee Belemnite (VPDB) standard.
DNA Extraction and Purification
Due to the extremely low amount of biomass in the core samples, 10 g of powdered sample material was used to extract the total genomic DNA with the DNeasy® PowerMax® Soil Kit (QIAGEN, Venlo, Netherlands). Afterwards, the obtained DNA was dissolved in 5 mL DNA-free water (Carl Roth, Karlsruhe, Germany). For each sampling depth, three independent samples were taken from different positions of the core horizon as technical triplicate. The 5 mL DNA solution was concentrated to 100 μL by an Eppendorf Concentrator Plus (Eppendorf AG, Hamburg, Germany). The Genomic DNA Clean & Concentrator™-10 (Zymo Research, Irvine, CA) was utilized to remove humic acids and other substances that may inhibit the polymerase chain reaction (PCR). Two DNA extractions were done from separated sample duplicates. DNA from 1 mL DNA-free water (Carl Roth, Karlsruhe, Germany) was extracted as a negative control using the same DNA extraction approach.
In addition to the core material, ~1 L of the fluid samples from the pump test were filtered (0.2 μm) to collect insoluble particles. The total genomic DNA trapped on the filters was extracted by the FastDNA™ SPIN Kit for Soil and the FastPrep® Instrument (MP Biomedicals, Santa Ana, CA) with standard protocols. The FastPrep® Instrument homogenizing time and the homogenizing speed were modified to 30 s and 5.5 m s−1 according to Liu et al. (2018).
Quantitative PCR
Total microbial abundance was estimated by determining the number of bacterial 16S rRNA gene copies using quantitative polymerase chain reaction (qPCR) targeting the V3 region of the 16S gene with the primer pairs 341F (5′-CCTACGGGAGGCAGCAG−3′) and 534R (5′-ATTACCGCGGCTGCTGG-3′) (Degelmann et al., 2010). The qPCR Master Mix consisted of 10 μL SYBR® FAST qPCR Master Mix (2X) Universal (KAPA Biosystems, Wilmington, Massachusetts, USA), 5.92 μL PCR water, 0.04 μL forward primer (100 μM), 0.04 μL reverse primer (100 μM), and 4 μL template. The qPCR was programmed as 3 min at 95°C, 40 cycles of 3 s at 95°C, 20 s at 60°C, 30 s at 72°C, and 3 s at 80°C for the plate read. A cloned 16S rRNA gene fragment from Escherichia coli was used as standard. The qPCR was conducted on a CFX96 real-time thermal cycler (Bio-Rad Laboratories Inc., USA) and the analysis of the quantification data was performed with the CFX Manager™ software (Bio-Rad Laboratories Inc., USA). The concentration range of the standard was optimized and set from 103 to 107 16S rRNA gene copies. The R2-value of the standard curve line was 0.994–0.997.
Illumina MiSeq Amplicon Sequencing
The 16S rRNA gene was amplified with OptiTaq™ polymerase (Roboklon, Berlin, Germany) which has a proofreading capability due to the extremely low concentration of extracted total genomic DNA. The PCR reaction solution consisted of 2.5 μL 10x Buffer Pol C, 0.125 μL OptiTaq™ polymerase, 1 μL dNTP Mix (5 mM each), 1 μL MgCl2 (25 mM), 17.075 μL PCR water, 0.3 μL bovine serum albumin, 0.25 μL forward primer (20 μM), 0.25 μL reverse primer (20 μM) and 2.5 μL template. Unique combinations of barcode-tagged 515F (5′-GTGCCAGCMGCCGCGGTAA-3′) and 806R (5′-GGACTACHVGGGTWTCTAAT-3′) (Caporaso et al., 2011) primers were assigned to each sample. PCR amplifications were performed in volumes of 25 μL on a T100™ thermal cycler (Bio-Rad Laboratories Inc., USA) under the following conditions: 5 min at 95°C, 35 cycles of 30 s at 95°C, 45 s at 56°C, 60 s at 72°C, and a final extension step of 7 min at 72°C. A cloned 16S rRNA gene fragment from E. coli was used as positive control. Non-template controls were included with each PCR run. The PCR products were cleaned up with AMPure XP magnetic beads (Beckman Coulter GmbH, Krefeld, Germany). After measuring the DNA concentration with a CLARIO star® plate reader (BMG LABTECH GmbH, Ortenberg, Germany) PCR products were pooled in equimolar amounts. The pooled DNA solution was concentrated with Eppendorf Concentrator plus (Eppendorf AG, Hamburg, Germany) to meet the requirement of the Illumina MiSeq high-throughput sequencing. The final pooled DNA concentration was 77.05 ng μL−1.
Bioinformatics and Statistical Analysis
Sequencing was performed by Eurofins Scientific SE (Luxembourg) on an Illumina MiSeq (2 × 250 bp). Dual-indexed reads were demultiplexed using CutAdapt (Martin, 2011) allowing for 10% errors in the primer and no errors in the barcodes. Individual samples were processed according to the DADA2 pipeline (Callahan et al., 2016). This includes an initial sequence truncation (250 bp forward reads; 200 bp reverse reads). The quality-filtered reads were used to generate an error model that was applied for dereplication, sample inference, and merging of the paired-end reads. All final sequences had a standardized read-orientation and a minimum length of 200 bp. The sequence table was created and potential chimera were filtered using a de novo approach. The resulting amplicon sequence variants (ASVs) were imported into the QIIME2 framework (Bolyen et al., 2019) which facilitated the SILVA taxonomy database (v132) (Quast et al., 2013) and VSEARCH (Rognes et al., 2016) to assign taxonomic units. Singletons and OTUs assigned to chloroplasts and mitochondria were removed from the obtained OTU table. After the filtering processes, 11,063,679 sequences were obtained in the 16S rRNA gene library in total. The read numbers ranged between 12,999 and 243,092 with a mean value of 99,985. The resulting ASV table was manually scanned for potential contaminant taxa based on the negative control, resulting in the removal of ASVs belonging to the taxa Escherichia, Undibacterium, Methylophilaceae, Comamonadaceae, Ralstonia, and Novosphingobium. Due to the low biomass environment and the high susceptibility of contamination introduction via the Power Soil Max kit (Sheik et al., 2018), comparison of drill core and pump test data was used as an additional screening approach and resulted in the removal of additional contamination ASVs (including Lawsonella and Staphylococcus). The final ASV table was rarified to a sequencing depth of 5,541 sequences (lowest available sequencing count) for alpha diversity estimation. Microbial diversity in each sample was assessed by calculating Shannon H and Shannon EH indices using the phyloseq package in R (McMurdie and Holmes, 2013). Beta diversity was determined by the non-metric multidimensional scaling (NMDS) using Bray Curtis distances with PAST3 (Hammer et al., 2001). Sequencing data was deposited at the European Nucleotide Archive (http://www.ebi.ac.uk/ena) under the accession numbers PRJEB22478 (ERS4382097 to ERS4382146 and ERS4382395 to ERS4382400).
Correlation and PCA of Microbial Genera and Lipid Biomarkers
The correlation and Principal Component Analysis (PCA) were performed using the software PAST3 (Hammer et al., 2001). Statistical examinations considered samples investigated both DNA and lipid biomarkers. More specifically, correlations between TOC, TIC divided into TIC-Dolomite restricted to the Cypris Fm. and TIC-Siderite restricted to the Main Seam Fm., TN, the TOC/TN-ratio, all microbial genera with a relative abundance >5%, detected functional genera (<5%) related to the methane-, sulfur- and iron-cycles and all detected lipid biomarkers were investigated. The resulting correlation coefficients (r) are based on linear regression (Pearson) and statistical significance was reported as a p-value. Correlations with a p > 0.05 were considered to be non-significant. The PCA was carried out for TOC, TIC-Dolomite, TIC-Siderite, TN, TOC/TN-ratio, all genera >5%, additional detected functional genera (<5%) and all predominating or relatively constantly distributed lipid biomarkers. The parameters were normalized to 1 with the PAST3 function “row normalized length” and the PCA calculated on the correlation matrix. Compounds not detected were treated as zero.
Results
Stratigraphy and Sample Material
The core section between 65 and 95 mbs lithologically consisted of three different units which were from the bottom to the top: (i) a weathered Paleozoic mica schist (95–91.5 mbs, Paleozoic basement), (ii) sandy to peaty Early Miocene mudstones of the Main Seam Formation (Fm.) with lignite fragments and root structures suggesting paleosol horizons in the lower and upper sections (91.5–78.5 mbs), and (iii) laminated, calcareous, sandy or peaty Early Miocene mudstones interbedded with bioclastic carbonates, dolomite to ankerite beds, and gypsum layers of lacustrine origin (78.5–65 mbs) belonging to the Cypris Fm. (Bussert et al., 2017; Figure 2A). Identified macrofossils in the Cypris Fm. were bark fragments, seeds, plant debris, and ostracod shells (Cypris angusta Rss.) (Figure 2A and Supplementary Figure 1). Sediments from the Main Seam and Cypris Fm. revealed vein-like structures indicating potential CO2 ascending pathways with associated mineral alteration and precipitation. These features were siderite-rich veins and bubble structures in the Main Seam Fm. and small fractures, dykes and sills with sediment color changes in the Cypris Fm. The CO2 blow out during the drilling campaign occurred at the transition from the Cypris Fm. to the underlying Main Seam Fm. This indicates that at this transition a dolomite-rich layer (about 30 cm thick) that is widely distributed in the Cheb basin (Smejkal, 1984; Pešek, 2014) or the lacustrine sediments themselves act as a sealing layer for the low-permeable CO2-saturated saline aquifer in the upper Main Seam Fm., resulting in a zone characterized by high CO2 pore pressure (Figure 2A).
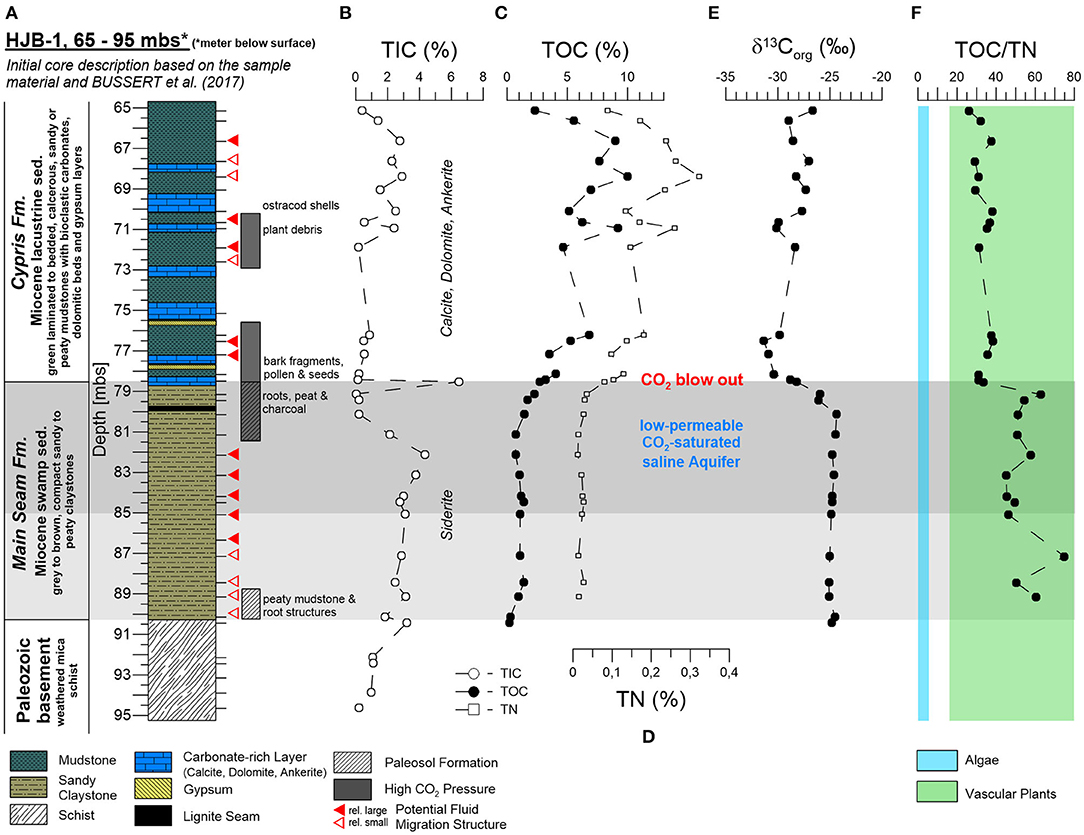
Figure 2. Investigated core section of the Hartoušov mofette core HJB-1 (2016) between 65 and 95 mbs with depth profiles of bulk sedimentological parameters. (A) Stratigraphical and lithological description based on visual inspection of the sample material and on data from Bussert et al. (2017), (B) total inorganic carbon (TIC), (C) total organic carbon (TOC), (D) total nitrogen (TN), (E) bulk δ13Corg, and (F) the TOC/TN ratio.
Bulk Carbon and Nitrogen
Carbonates were detected in all three lithological units and are expressed in total inorganic carbon (TIC) (Figure 2B). In the Paleozoic basement and in the Main Seam Fm. the carbonates were mainly represented by zoned siderite spheres and veins that could have been precipitated from the low-permeable CO2-saturated saline aquifer. At the transition from the Main Seam Fm. to the Cypris Fm. carbonates were essentially absent, except of the thick (30 cm) dolomite-rich layer at 78.5 mbs. In the Cypris Fm., calcite, dolomite, and ankerite predominated. Their occurrence together with evaporitic layers indicates a lacustrine origin (Smejkal, 1984; Pešek, 2014). However, a possible early diagenetic alteration cannot be excluded.
Organic matter was not detected in the Paleozoic basement. The TOC contents of the Main Seam Fm. ranged between 0.2 and 2.3%. After a small increase the TOC contents remained relatively constant at ca. one percent before increasing to 2.3% at the top of the Main Seam Fm. (Figure 2C). In the overlying lacustrine Cypris Fm. the TOC contents were significantly higher and show strong fluctuations between 2.3 and 10%. Bulk δ13Corg data also changed with the lithological transition from the Main Seam to the Cypris Fm. showing relative constant values around −24%0 in most parts of the Main Seam Fm. and a strong decrease down to −30%0 at the top (Figure 2E). In the Cypris Fm. the organic carbon isotope signals fluctuate between −31 and −27%0.
The total nitrogen content (TN, Figure 2D) was mainly positively correlated with the TOC content (r = 0.99) (Supplementary Table 1). Values were low in the Main Seam Fm. ranging between 0.01 and 0.04% and increase at the top. In the Cypris Fm. TN values were significantly higher ranging between 0.08 and 0.32%. The TOC/TN ratio ranged between 45 and 75 in the Main Seam Fm. and between 26 and 41 in the Cypris Fm. (Figure 2F).
Microbial Lipid Biomarker Signals
Hopanoids, representing stabilizing and ordering components in bacterial cell membranes (Sáenz et al., 2015), could be detected as unsaturated hopenes [22,23,30-trisnor-17(21)-ene, 30-norhop-17(21)-ene, hop-17(21)-ene, neohop-13(18)-ene] and saturated hopanes with the steric 17β(H), 21β(H)-, 17β(H), 21α(H)-, and 17α(H)β(H)-configuration (ββ–C27, ββ–C29, βα–C29, ββ–C30, αβ–C30, ββ–C31, βα–C31, αβ–C31R, αβ–C31S) (Figure 3B). In the Paleozoic basement hopanoids were essentially absent. In the Main Seam Fm. the hopanoid signal was usually low (<6 μg gSed−1) and dominated by the αβ-C31R-hopane. However, at the top to the Main Seam Fm. the hopanoid concentrations especially the αβ-C31R signal distinctly increased to the highest values in the entire investigated core interval (134 μg gSed−1) (Figure 3B). In the Cypris Fm., hopanoids were generally more abundant (14–62 μg gSed−1) than in the Main Seam Fm. (excepting the top of the Main Seam Fm.) and hop-17(21)-ene supplemented by ββ-hopanes were the predominating hopanoid compounds (Figure 3B). The δ13Chopanoid values in the Main Seam Fm. plotted in a range between −22 and −37%0 and shifted to significantly lower values between −42 and −60%0 in the Cypris Fm. (Figure 3C).
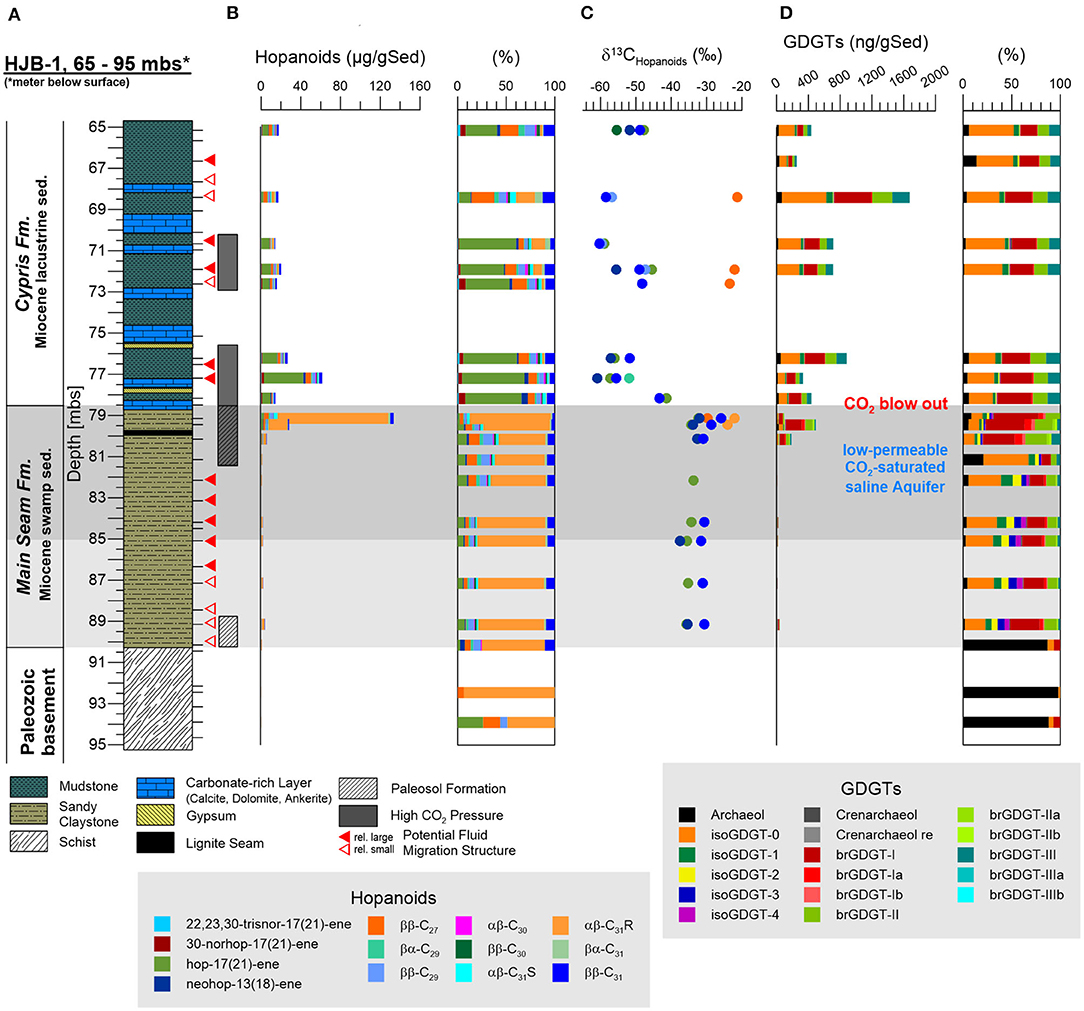
Figure 3. Investigated core section of the Hartoušov mofette core HJB-1 (2016) between 65 and 95 mbs with depth profiles of investigated lipid biomarkers. (A) Stratigraphical and lithological description, (B) the hopanoid distribution and its relative abundance (%), (C) δ13Chopanoids of different hopanoids, and (D) the (core) GDGT distribution and its relative abundance.
In general, GDGTs, also referred to as core GDGTs, are degradation products of membrane-spanning intact microbial lipids (IPL-GDGTs), which contain the GDGT core unit linked to one or two head groups. In contrast to the intact polar lipids (IPL-GDGTs) the GDGT core lipids are stable over geological times. Structural differences lead to a subdivision into branched GDGTs (brGDGTs) and isoprenoid GDGTs (isoGDGTs) (Schouten et al., 2013). The brGDGTs are known to originate from bacteria in terrestrial environments (soils, peats, rivers, and lakes) (Weijers et al., 2007, 2010; Tierney et al., 2012; Dirghangi et al., 2013; DeJonge et al., 2014; Freymond et al., 2017; Huguet et al., 2017; Naafs et al., 2017) and the isoGDGTs are from archaea within aquatic (marine and lacustrine) and terrestrial systems (Pancost et al., 2011; Tierney et al., 2012; Dirghangi et al., 2013; Bischoff et al., 2014; Naeher et al., 2014; Schubotz et al., 2018; Bale et al., 2019; Evans et al., 2019). Both GDGT types occurred across the whole lithological profile with isoGDGT-0, isoGDGT-1, brGDGT-I, brGDGT-II, and brGDGT-III being the most prominent (Figure 3D). In addition to the GDGTs, the archaeal membrane degradation product archaeol was also present in notable amounts. Except isoGDGT-3, all detected GDGTs showed a positive correlation with TOC (Supplementary Table 1). In the Paleozoic basement and lower part of the Main Seam Fm., GDGTs were either absent or identified at low concentrations (1–20 ng gSed−1). The top of the Main Seam Fm. revealed a clear predominance of brGDGTs with a dominating brGDGT-I (~200 ng gSed−1) and subordinated brGDGT-Ia (~37 ng gSed−1), brGDGT-II (~90 ng gSed−1), and isoGDGT-0 (~55 ng gSed−1). Archaeol occurs in lower amounts (~28 ng gSed−1). In the Cypris Fm., isoGDGT-0 ranging between 91 and 559 ng gSed−1 became the most predominant GDGT and brGDGT-I was found to be the second most abundant GDGT (50–462 ng gSed−1). Slightly increased concentrations were also detected for brGDGT-III (26–219 ng gSed−1) and isoGDGT-1 (11–82 ng gSed−1). Interestingly, brGDGT-II (28–252 ng gSed−1) and brGDGT-III appeared in similar amounts from this point to the top of the Cypris Fm. (78–65 mbs). Archaeol contents were low and could be positively correlated to the TOC (8–67 ng gSed−1; r = 0.88). Crenarchaeol appeared across the whole profile but in very low amounts ranging between 0 and 10 ng gSed−1 (Figure 3D).
In contrast to the hopanoids and GDGTs, both commonly interpreted to represent necrotic microbial biomass, intact polar lipids (IPLs) provide information on present microbial life, since these biomarkers degrade relatively rapid after cell death (White et al., 1979; Zink et al., 2003). The chromatogram of IPLs revealed no common phospholipids, but a double peak which represented, to the best of our knowledge, unknown lipid compound groups. These groups were tentatively referred to as compound group A and B (Figure 4A). The mass spectra of these two compound groups showed a cluster of six individual mass peaks with a maximum at m/z 631 or 617, respectively (Figures 4B,C). The masses differed by 14 mass units indicating an increase of the lipid side chain length by a CH2-group. The individual masses of the two compound groups were essentially the same, indicating the same elemental composition. This and the close vicinity of the chromatographic signals suggested that these compounds bear the same head group but show different configurations in their side chains (e.g., OH-group vs. ether-group), causing slightly different elution behaviors. Microbial membrane lipids usually consist of a polar head group and two long-chain ether or ester side chains linked to a glycerol backbone (Mangelsdorf et al., 2019). To elucidate whether ester bond fatty acids formed the lipid side chains, MS-MS and saponification experiments [polar lipid fatty acid (PLFA) analysis] were conducted indicating that the side chains are not ester bound fatty acids and that alkyl and ether bound side chains are more likely. High resolution analysis using an Orbitrap MS provided insights on the elemental formular of the unknown compounds. This approach, together with the isotope patterns of the individual molecular masses, indicated the presence of sulfur (32S and 34S-isotope) presumably a sulfonic acid (R-SO2-OH) as the head group part. An example for the suggested lipid structure for the mass peak at 617 m/z within compound group A is shown in Figure 4D and further experiments have to be conducted to determine the full structure of compound groups A and B. Both lipid groups were almost absent in the basement and Main Seam Fm. (0–7.6 μg/gSed; Figure 5G). However, they were most abundant (475.2 μg/gSed) at the top of the Main Seam Fm. with its high CO2 pressure. In the Cypris Fm. the signal decreased significantly again but was still a bit higher in CO2 influenced core intervals (up to 116 μg/gSed; Figures 5A,G).
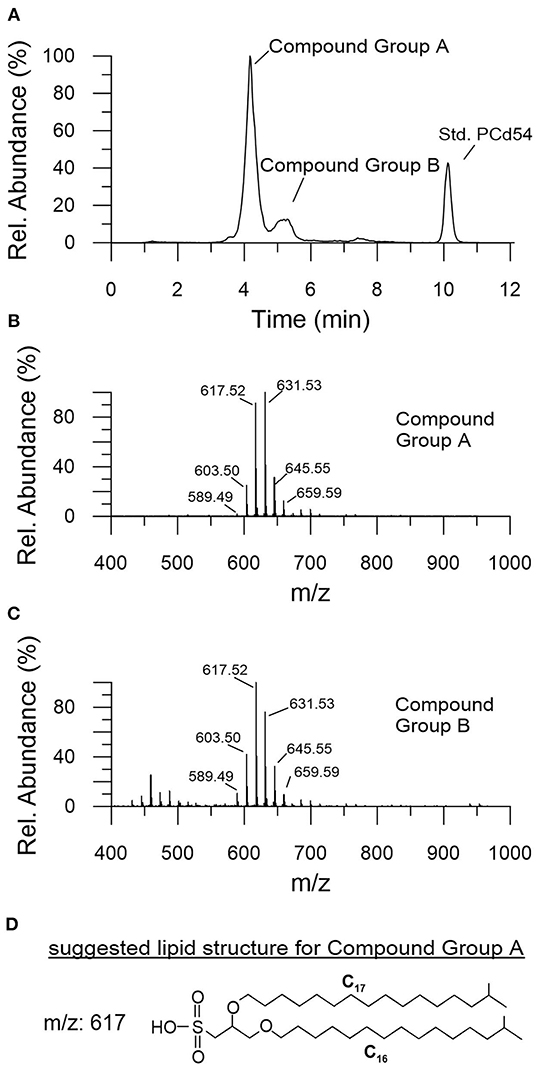
Figure 4. Description of the yet unknown groups of intact polar lipids (IPLs) referred to as compound group A and B. (A) HPLC chromatogram, (B,C) associated mass spectra, both showing a cluster of six individual mass peaks differing by 14 mass units with a maximum at m/z 631 or 617, respectively. (D) Suggested lipid structure for the mass peak at m/z 617 of compound A.
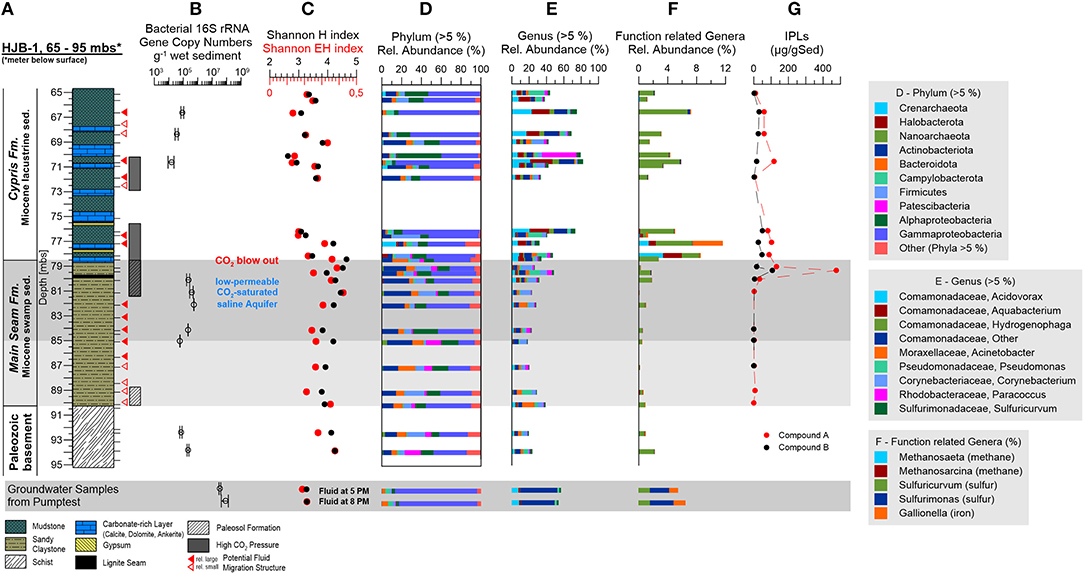
Figure 5. Investigated core section of the Hartoušov mofette core HJB-1 (2016) between 65 and 95 mbs with depth profiles of investigated geomicrobiological parameters and intact lipid biomarkers. (A) Stratigraphical and lithological description of the Hartoušov mofette core HJB-1 (2016), (B) bacterial 16S rRNA gene copy numbers, (C) the Shannon H and Shannon EH indices, (D) the community structure on phylum (>10%) and (E) genus (>5%) levels, (F) specific genera with respect to their metabolism, and (G) intact polar lipids (IPLs).
Abundance of Microorganisms
Total microbial abundance was assessed by measuring the number of bacterial 16S rRNA gene copies per g−1 wet sediment across the evaluated core section. Microbial abundance ranged between of 104 and 105 16S rRNA gene copies g−1 wet sediment in the Paleozoic basement (93.9 and 92.4 mbs), the low-permeable CO2-saturated saline aquifer in the upper Main Seam Fm. (85.1, 84.2, 82.2, 81.1, and 80.2 mbs) and in the upper part of the investigated Cypris Fm. showing fluid migration structures (70.7, 68.4, and 66.7 mbs) (Figures 5A,B). No data was obtained for the other samples due to low biomass or the presence of inhibitors. The fluid filter samples from the pump test contained gene copy numbers in a range of 107 16S rRNA gene copies L−1 (Figure 5B).
Microbial Community Composition
Alpha diversity of the microbial community for the whole lithological profile was assessed by calculating the Shannon H and evenness indices (diversity) per 5,541 sequences. The Shannon H index ranged between 2.6 and 4.7 and the Shannon EH index (evenness) ranged between 0.1 and 0.4 (Figure 5C). Shannon H and Shannon EH indices were found to be higher in the Paleozoic basement, the Main Seam Fm. and at the bottom of the Cypris Fm. (3.8–4.7 and 0.2–0.4) and lower in most of the upper investigated Cypris Fm. (2.6–3.6 and 0.1–0.2) (Figure 5C). Shannon H and EH indices for the fluid filter samples ranged around 3.3 and 0.2 (Figure 5C).
Microbial communities across the analyzed core section were found to be dominated by Bacteria (78–99%) (Figure 5D). The bacterial community in the Paleozoic basement and Main Seam Fm. was characterized by large abundances of Proteobacteria, specifically Gammaproteobacteria (16–45%) and Alphaproteobacteria (8–25%). In addition, Actinobacteria (8–27%), Bacteroidota (4–17%), and Firmicutes (2–20%) were found to be enriched in these layers. Gammaproteobacteria (37–85%) and Alphaproteobacteria (3–45%) were even more abundant in the Cypris Fm., while the detection of Actinobacteriota, Bacteroidota, and Firmicutes varied depending on the evaluated core section. Core sections, where these taxa were identified at abundances similar to the Main Seam Fm., will be in the following text referred to as major Cypris Fm. (65.2, 65.7, 69.1, 71.0, 71.9, and 76.5 mbs), while core sections where Actinobacteriota, Bacteroidota, and Firmicutes were discovered at low relative abundances (66.7, 68.4, 70.7, and 76.2 mbs) will be referred to as intercalated zones.
Archaeal abundances were generally low (0–3%), but appeared to be elevated at a depth of 65.2 mbs (4%), 71.0 mbs (6%), 77.2 mbs (18%), 78.2 mbs (6%), and 85.1 mbs (6%). Archaeal communities were dominated by the phyla Crenarchaeota (average 1.3%, up to 14.8%) and Halobacterota (up to 6.0%). In addition, Nanoarchaeota (3.0–5.5%) were enriched in two deeper core sections (85.1 and 92.5 mbs) (Figure 5D).
The community of the groundwater filter samples from the pump test was dominated by Gammaproteobacteria (81%) with low amounts of Bacteroidota (5%) and Campylobacterota (5%) and showed similarities to the community structure of the intercalated zones in the Cypris Fm. (Figure 5D).
The microbial community was evaluated in more detail by taking a closer look at the genera with a relative abundance of more than 5%. Members belonging to the family Comamonadaceae known from soil and aquatic environments represented the largest fraction of Proteobacteria across the entire analyzed core. This group includes the facultative anaerobic and potentially iron-oxidizing taxon Acidovorax, the biofilm-forming Aquabacterium, the putative facultative autotrophic genus Hydrogenophaga, and several uncharacterized Comamonadaceae genera (Figure 5E). Other genera identified at relative abundance of >5% were Acinetobacter, Pseudomonas, Corynebacterium, Paracoccus, and Sulfuricurvum (Figure 5E).
In the Paleozoic basement and Main Seam Fm. most dominant genera (>5%) did not exceed 50% of the total relative abundance and were represented by Acinetobacter (0.2–15%), Pseudomonas (0.6–13%), Corynebacterium (1–13%), unknown Comamonadaceae (3–7%), Paracoccus (0.1–6%), and Acidovorax (1–5%). The taxa Acidovorax, Aquabacterium, Hydrogenophaga, Pseudomonas, and Sulfuricurvum were enriched at the transition from the Main Seam to the Cypris Fm. (Figure 5E). The major Cypris Fm. was characterized by the occurrence of genera also found in the Paleozoic basement and Main Seam Fm. [i.e., Acinetobacter (0.5–6%), Pseudomonas (1–17%), Corynebacterium (1–14%), unknown Comamonadaceae genera (3–10%), Paracoccus (0.1–40%), and Acidovorax (4–9%)]. However, this was supplemented by an increased occurrence of Aquabacterium (2–13%), Hydrogenophaga (0.4–4%), and Sulfuricurvum (0.4–5%). The intercalated zones were dominated by Aquabacterium (15–25%), Acidovorax (17–23%), unknown Comamonadaceae (15–18%), Hydrogenophaga (5–8%), and Sulfuricurvum (3–7%) whereas the other genera were significantly decreased. The groundwater filter samples were dominated by unknown Comamonadaceae genera (41%) and contained low amounts of Acidovorax (8%), Aquabacterium (2%), Pseudomonas (2%), and Sulfuricurvum (2%) (Figure 5E).
Due to the paleo-environmental conditions of the investigated lithological profile, the hydro-geochemical composition of the low-permeable CO2-saturated saline aquifer with high CO2, , and Fe2− concentrations (Bussert et al., 2017), and the previous microbiological surface investigations from the Hartoušov and Bublák mofette systems (Beulig et al., 2015; Krauze et al., 2017; Liu et al., 2018), the presence of microorganisms involved in methane-, sulfur-, and iron- cycling were expected. However, only small fractions belonging to these microbial groups could be detected. Methanogens were represented by Methanosarcina (0–3%) and Methanosaeta (0–3%) and were mainly detected at the base of the Cypris Fm. (Figure 5F). In spite of their comparably low abundance, sulfur-cycling genera formed the most abundant group of functional taxa and consisted of the sulfur-oxidizing genera Sulfuricurvum and Sulfurimonas. Sulfuricurvum was frequently detected across the evaluated core sections (0.4–8%) and was especially abundant at the transition from the Main Seam to the Cypris Fm. and in other intervals of the Cypris Fm. (10%). Sulfurimonas was most abundant in the groundwater filter samples (3%) (Figure 5F). A notable iron-cycling genus was the putative iron-oxidizer Gallionella. While Gallionella abundances were generally low (<0.3%), sequences belonging to this genus were frequently detected at the base of the Cypris Fm. (4%) and in the groundwater filter samples (1%) (Figure 5F).
Comparison of the microbial distribution across the lithological profile using non-metric multidimensional scaling (NMDS) based on the Bray-Curtis (stress 0.18) identified two major clusters associated with the Main Seam and Cypris Fm. (Figure 6). Samples from the Paleozoic basement clustered closer to the Main Seam Fm. and the groundwater filter samples clustered closer to the intercalated zones of the Cypris Fm. (Figure 6).
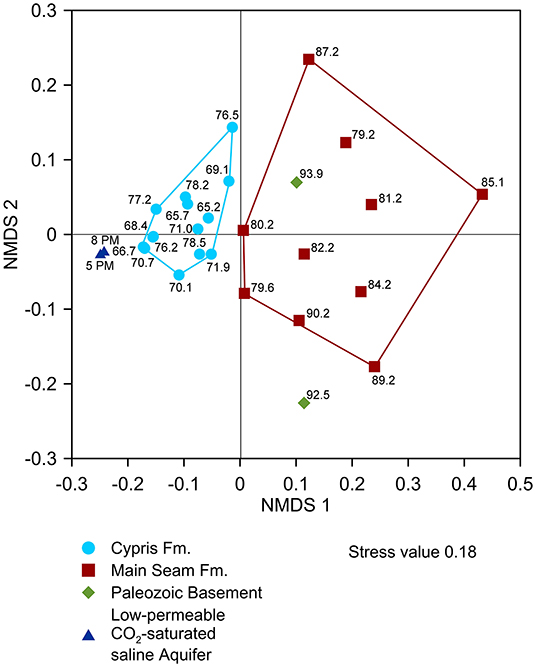
Figure 6. Beta diversity obtained by non-metric multidimensional scaling (NMDS) based on the Bray-Curtis dissimilarity to compare the relative abundance of microorganisms within the samples with respect to their distribution within the lithological profile, i.e., Paleozoic basement, Main Seam Fm., and lacustrine Cypris Fm. as well as the groundwater of the deep CO2-saturated aquifer.
Statistical Analysis of Environmental Parameters, Lipid Biomarkers, and the Microbial Community Structure
Correlation analysis of the bulk elemental parameters, the most abundant microbial genera (>5%), detected functional genera (<5%) and all identified lipid biomarkers revealed various positive correlations (Supplementary Table 1). Within the environmental parameters TIC-Dolomite was found to correlate with TOC and TN. These environmental parameters were found to be positively correlated to the occurrence and relative abundance of the taxa Comamonadacceae, Acidovorax, Aquabacterium, Hydrogenophaga, and Sulfuricurvum, all predominating GDGTs (isoGDGT-0, isoGDGT-1, brGDGT-I, brGDGT-II, and brGDGT-III), most of the rarely detected isoGDGTs (isoGDGT-2, isoGDGT-4, Crenarchaeol, and Crenarchaeol region-isomer) and archaeol (Supplementary Table 1). Most importantly the above mentioned taxa and lipids were also positively correlated with each other (Supplementary Table 1). Since, the correlated genera belong to the domain of bacteria and isoGDGTs are known to be produced by archaea (Schouten et al., 2013) the results evidently represent a spurious correlation, meaning that the investigated lipids cannot be directly assigned to the identified microorganisms. In general, all of these parameters increase at the top of the Main Seam Fm. and exhibit all around higher abundances in the Cypris Fm. (Figures 2C,D, 3B,D, 5D–G). Hence, it is likely that the occurrence pattern of both lipid and microbial abundances is not correlated to each other, but rather driven by the TOC content.
The conducted principal component analysis (PCA) identified one predominating and several minor environmental factors, which may influence the distribution and relation of the identified microbial genera and lipid biomarkers (Figure 7 and Supplementary Figure 2A). In the process, the first two principal components (PCs) explained a cumulative variance of 50.4% with an outstanding explained variance of 34.9% for PC1 and 15.5% for PC2 (Figure 7). TIC-Siderite together with the taxa Acinetobacter, Pseudomonas, Corynebacterium, and Paracoccus (all of which represent the Paleozoic basement and Main Seam Fm. characterized by a low TOC content) plotted on the positive PC1 axis. In contrast, the environmental parameters TIC-Dolomite, TOC and TN (which are closely associated with the Cypris Fm.) as well as the genera Aquabacterium, Hydogenophaga, Acidovorax, Sulfuricurvum, Methanosaeta, Methanosarcina, and Gallionella and all detected lipid biomarkers plotted on or near the negative PC1 axis (Figure 7). The principal component analysis resulted into four small clusters referred to as cluster W, X, Y, and Z (Figure 7). Cluster W was represented by a negative PC1 and a positive PC2 value range and was comprised of TOC, TN, all GDGTs (isoGDGT-0, iso-GDGT-1, brGDGT-I, br-GDGT-II, and brGDGT-III) and the genus Aquabacterium. In addition, archaeol and the genera Hydogenophaga plotted close to cluster W (Figure 7). Clusters X and Y plotted both in the negative ranges of PC1 and PC2. Cluster X contained the putative unknown lipid biomarker compound groups A and B (Figure 7) and Cluster Y included the genera Methanosaeta, Methanosarcina, and Gallionella (Figure 7). All parameters related to these two clusters were abundant at the top of the Main Seam or at the bottom of the Cypris Fm. (Figures 3B,D, 5F). Cluster Z was located near cluster W and comprised of frequently identified taxa (both in the core and the groundwater), namely the unknown genera of the family Comamonadaceae, Acidovorax, Sulfurimonas, and Sulfuricurvum, but did not include any environmental parameters or lipid biomarkers (Figure 7).
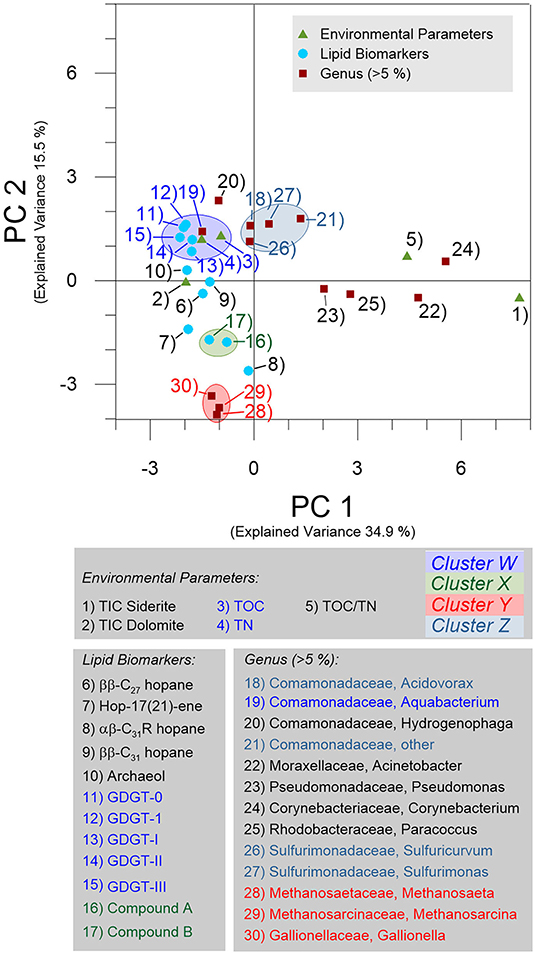
Figure 7. Biplot showing the principal component (PC) loadings for PC1 and PC2 obtained from principal component analysis (PCA) of the environmental parameters, selected microbial genera and predominating microbial lipid biomarkers.
The PCA clustering suggests that the parameters, taxa, and lipids aggregating in and around clusters W and Z are positively correlated to TOC. The positive correlation of these parameters with the TOC content (Figure 7 and Supplementary Table 1) supports the TOC as major environmental shaping factor represented by PC1 and becomes obvious when comparing the depth distributions of the TOC content (Figure 2C) with the PC1 loadings (Supplementary Figure 2C).
Discussion
Differentiation Into Present and Past Microbial Signals
Data analysis identified no meaningful correlation between the present microbial community and the lipid biomarkers but a positive correlation of both with the TOC content. This is shown by the PCA analysis plotting biomarker signals and microbial community data essentially into different clusters (Figure 7).
Nevertheless, in order to further prove that the occurrence of core GDGTs is not linked to the ascending CO2, we compared our GDGT results to the findings of Oppermann et al. (2010), who investigated the distribution of intact GDGTs in a surface interval of a natural CO2 vent in comparison to a non-influenced reference site. They found a 10 times higher amount of intact GDGTs (branched and isoprenoid) in the CO2 vent compared to the reference site and the vent site was dominated by brGDGT-I, -II, and -III as well as significantly increased amounts of isoGDGTs with 1, 2, 3, and 4 cyclopentyl rings relative to isoGDGT-0 (no ring) and crenarchaeol (Oppermann et al., 2010). However, a similar core GDGT distribution was not detected in the intervals of high CO2 pressure between 70 to 81 mbs in the Hartoušov core (Figures 3A,D). While brGDGTs are also dominated by brGDGT-I, -II, and –III, isoprenoid GDGTs are dominated by isoGDGT-0 and isoGDGT-1,−2,−3, and−4 are in general very low or absent (Figure 3D). This finding and the strong correlation of GDGTs to the TOC indicates that brGDGTs are more likely restricted to surficial environments like soils, peats, and lakes and therefore represent a past microbial signal (Pancost et al., 2011; Tierney et al., 2012; Dirghangi et al., 2013) and that the isoGDGTs are mainly of syn-sedimentary origin rather than from archaea related to the ascending mantle-derived CO2. In addition, the occurrence of archaea at the bottom of the Cypris Fm. (Figures 5D,F) does not result in increased amounts of archaeol and isoGDGT-0 and−1 at this interval (Figure 3D). Since these lipids are reported to represent methanogens in subaquatic (lakes) and subaerial (peats, soils, permafrost) systems (Pancost et al., 2011; Tierney et al., 2012; Dirghangi et al., 2013; Bischoff et al., 2014), the assumption of a syn-sedimentary origin for most of these isoGDGTs seem to be very likely. However, a minor production of archaeol or isoGDGT-0 by Methonasaeta and Methanosarcina at the base of the Cypris Fm. cannot be ruled out (Bischoff et al., 2014; Evans et al., 2019).
The lack of meaningful correlations between hopanoids and bacterial genera (Supplementary Table 1) and the compositional change of the dominating hopanoid types from the Main Seam Fm. (αβ–C31R-hopane dominated) to the Cypris Fm. [Hop-17(21)-ene dominated] (Figure 3B) suggests that the hopanoid distribution is also the result of compound preservation during time of deposition. This suggestion is confirmed by the strong shift of the δ13Chopanoid values (Figure 3C) showing a clear decrease related to the transition from the Main Seam to the Cypris Fm. and not to the occurrence of increased CO2 concentrations (Figures 3A,B).
In contrast to the GDGTs and hopanoids intact polar lipids (IPLs) are usually interpreted as markers for living microorganisms (White et al., 1979). Two uncommon and putative unknown IPL groups A and B were detected in the Hartoušov core material (Figure 5G). First structural assessment suggests that they bear a sulfur-containing head group (presumably a sulfonic acid group) and ether- or alkyl-linked long hydrophobic side chains (Figure 4D). This side chain structure would explain why they were not detected during the PLFA analysis, only targeting ester-linked side chains. The fact that two clusters of up to six compounds representing individual lipids with the same head group but different side chain lengths (-CH2- differences) were detected, point to the origin of these lipids from bacteria or a single bacterium. Archaea also contain ether-linked side chains, but they do not show this side chain length variability (Mangelsdorf et al., 2019). Due to the ether-linked side chains with its higher stability against initial degradation, it might be argued that the potential of these IPLs to act as a life marker is restricted (Logemann et al., 2011). However, these compounds show their highest concentration in the sediments with increased CO2 abundance especially below the interface between the Main Seam and the Cypris Fm. (Figure 5G). This suggests that the source bacteria are related to the high CO2 concentrations in the deep subsurface of the Hartoušov mofette and that these uncommon lipids act here as life markers, because their intact preservation since millions of years is not very likely. It can only be speculated whether these lipids (Figure 4D) are derived from sulfur-cycling genera, which were significantly detected in the same intervals although in different amounts than the lipid markers (Figures 5F,G). Further analysis has to be conducted to elucidate the exact structure and origin of these uncommon membrane lipids.
In summary, the investigation of the microbial community structure and the microbial lipid biomarkers in the deep sediments of the Hartoušov mofette system reveals a community distribution that does not significantly correlate to the profiles of the identified lipid biomarkers (Figures 3B–D, 5D–G and Supplementary Table 1). Thus, our findings suggest that GDGTs and hopanoids are not remnants of a currently living microbial community, but represent a signal of syn-sedimentary past microbial communities. Hopanoids more likely represent necrotic remains of bacteria (Ourisson et al., 1979; Summons et al., 2006; Naeher et al., 2014; Talbot et al., 2016) and GDGTs were produced by microorganisms living in soils (Blaga et al., 2009; Weijers et al., 2009, 2010) or lacustrine systems (Blaga et al., 2009). Two groups of unknown IPLs point to the present of living microorganisms related to the ascending CO2. Overall, our results indicate that present microbial life in the Eger Rift subsurface is limited, as low microbial abundances (from below the detection limit to amounts between 104 and 105 copy numbers g−1 wet sediment), low microbial diversity (low Shannon H indices) and the absence of common IPLs suggest low turnover rates. Hence, the limited microbial activity is not able to overprint the past biomarker signal. Nevertheless, the recovery of microbial DNA over the whole lithological profile, the albeit limited, but persistent presence of bacterial 16S rRNA genes, the identification of a specific microbial community and the occurrence of two uncommon IPL groups support the existence of a distinct deep biosphere. An explanation for the identification of DNA, while no common IPLs could be detected, are the different detection limits regarding these two analytical approaches. The DNA amplicon sequencing approach is able to amplify low amounts of DNA and in combination with the higher than usual amount of sample material used for DNA extraction here (10 g), this results into a significant lowering of the detection limit for the DNA analysis compared to the lipid analysis. In contrast, the lipid analysis does not contain an amplification step, resulting in lower sensitivity even when increasing the sample amount for the lipid biomarker extraction to 80 g.
Depositional Environment and Past Microbial Signatures
Based on the lithological description by Bussert et al. (2017), the Early Miocene Main Seam Fm. is characterized by terrestrial sediments deposited in a swamp environment. This is confirmed by the low TN values, the high TOC/TN ratio and the bulk δ13Corg values around −26%0 indicating that vascular C3 plants are the major source of organic matter (OM) (Meyers, 1997, 2003; Figure 2). The abundance of roots, peat, and charcoal in the sample material (Figure 2A) as well as increases in the TOC contents suggests two intervals of paleosol and peat formation at the bottom and the top of the Main Seam Fm. (Figure 2C).
The Early Miocene Cypris Fm. is described as lacustrine deposit, which is lithologically more heterogenous with phases in which carbonate precipitation interrupted the predominant siliciclastic sedimentation (Bussert et al., 2017). The high and fluctuating TOC contents point to changes in OM production related to changing environmental conditions (Figure 2C). Fossil ostracod shells and higher TN values indicate planktonic biomass (Meyers, 1997, 2003; Figures 2A,D). Compared to the Main Seam Fm., the TOC/TN ratio is indeed significantly lower, but with values between 25 and 40 still in the range of land plant material (Figure 2F). This indicates that the lacustrine OM is a mixture of autochthonous aquatic and allochthonous terrestrial biomass. The depletion in bulk δ13Corg (Figure 2E) points to an increased input and/or preservation of 13C-depleted OM, such as lignins, lipids, or their degradation products (Gleixner et al., 1993; Werth and Kuzyakov, 2010), the preservation of isotopic lighter freshwater plankton (Gaines et al., 2009) and/or to early diagenetic microbially induced carbon isotope fractionation, e.g., by methanogenesis, methanotrophy, or acetogenesis (Whiticar, 1999; Boetius et al., 2000; Conrad, 2005; Heuer et al., 2009).
The hopanoids, representing syn-sedimentary necrotic microbial biomass as outlined above, show in the Main Seam Fm. a 13C-depleted but relatively similar distributed δ13Chopanoid signal around −30%0 (Figure 3C) compared to the bulk δ13Corg (around −26%0) (Figure 2E), pointing to a heterotrophic degradation of terrestrial OM by their source organisms (Summons et al., 2006; Talbot et al., 2016). The dominance of αβ-C31R-hopane is associated with bacteria known from terrestrial and more specific peat environments (Quirk et al., 1984; Huang et al., 2015; Inglis et al., 2018). In contrast, the shift to a domination of Hop-17(21)-ene and the decrease of the δ13Chopanoid signal to values ranging between −50 and −60%0 in the Cypris Fm. (Figures 3B,C) illustrates that planktonic freshwater bacteria or benthic aerobic methanotrophs dwelling on microbially-derived methane at the water-sediment interface might have formed a significant part of the source bacteria for the deposited hopanoids (Nealson, 1997; Whiticar, 1999; Summons et al., 2006; Naeher et al., 2014; Davies et al., 2016; Hoefs, 2018).
A similar picture, resulting from the depositional environment can be seen from the depth distribution of the GDGTs. The upper Main Seam Fm. is dominated by brGDGTs characteristic for soil and swamp environments (Weijers et al., 2007, 2010; Dirghangi et al., 2013; Schouten et al., 2013; Freymond et al., 2017; Huguet et al., 2017; Naafs et al., 2017; Figure 3D). The lacustrine Cypris deposits show high but fluctuating amounts of isoGDGTs and brGDGTs representing a mixture of aquatic microbial biomass produced in the lake and terrestrial biomass from the catchment area (Weijers et al., 2007, 2010; Tierney et al., 2012; Schouten et al., 2013; Freymond et al., 2017), respectively. An aquatic in situ production (lacustrine or fluvial) of brGDGTs cannot be excluded, although such in situ productions generally seem to appear only to minor amounts (Tierney et al., 2012; DeJonge et al., 2014; Freymond et al., 2017). The distribution pattern of br-GDGT-II and –III, showing relatively similar amounts, was also investigated by Huguet et al. (2017) for bog and fen sediments and may confirm a palustrine OM input. Therefore, compared to the Main Seam Fm., the relatively higher brGDGT content might reflect a combination of in situ lake production and OM input from the catchment area as well as good preservation conditions within the lake sediments. Abundant isoGDGT-0 and the occurrence of archaeol and isoGDGT-1 with an initial appearance in the paleosol formation section of the Main Seam Fm. and higher occurrence in the Cypris Fm. point to the presence of methanogenic and methanotrophic archaea that have adapted from a palustrine to a lacustrine environment (Pancost et al., 2011; Dirghangi et al., 2013; Schouten et al., 2013; Bischoff et al., 2014; Naeher et al., 2014; Bale et al., 2019). In addition, an origin from archaea living in the water column cannot be excluded (Buckles et al., 2013; Schubotz et al., 2018). However, comparing the results from the hopanoid and GDGT analysis, we assume that methane cycling processes particularly of methanogenic and methanotrophic archaea and methanotrophic bacteria (hopanoid signal) played a significant role within the Early Miocene lake environment (Figure 3C).
Deep Biosphere Structure and CO2-Migration Model of the Deep Hartoušov Mofette System
Our results for the present microbial community structure revealed a domination of genera from the family Comamonadaceae and low amounts of archaea (Figures 5D–F). Surface investigations from the Hartoušov mofette system (Beulig et al., 2015; Liu et al., 2018) and a CO2 vent within the Latera Caldera (Central Italy) (Oppermann et al., 2010) show relatively high abundances of acidophilic, methanogenic and iron- and sulfur-cycling microorganisms [e.g., methanogenic archaea, Acidobacteria, Chloroflexi, sulfate reducing bacteria (SRB), and Geobacteraceae]. These species either appear only to minor amounts or are completely absent in the deep subsurface sediments (Figures 5D–F), displaying the occurrence of different microbial communities in the surface and deep sections of the Hartoušov mofette system.
Considering the Early Miocene age of the investigated sediments, we suggest several community shaping selection processes during subsidence and early diagenetic transformation (Orsi et al., 2020) prior to the onset of CO2-migration in Mid Pleistocene. These selection processes formed a basic microbial community, which became further shaped by the ascending mantle-derived CO2. This assumption is based on the finding of a major microbial community composition that is in parts related to the lithological profile (Figure 6) and shows some modifications related to the occurrence of higher CO2 pressures and the presence of CO2-saturated groundwater. The major community is composed of Actinobacteriota, Bacteroidota, Firmicutes, Patescibacteria, Alphaproteobacteria, and dominating Gammaproteobacteria (Figure 5D) and appears in the Main Seam and Cypris Fm. with the difference, that the Gammaproteobacteria are more abundant in the Cypris Fm. (Figure 5D). A high relative abundance of Gammaproteobacteria and a low Shannon H index of the groundwater filter samples from the CO2-saturated saline aquifer seem to indicate a microbial selection and adaptation related to a high influence of ascending CO2-saturated groundwater, which is expressed in the upper Cypris Fm. as intercalated zones (66.7, 68.4, 70.7, and 76.2 mbs) (Figures 5C,D). As a consequence, Shannon H indices <3.2 in the lithological profile could be indicative for a high CO2 influence (Figure 5C).
On the genus level, the occurrence of unknown genera from the family Comamonadaceae in both, the groundwater and lithological samples as well as the abundance of other Comamonadaceae genera, namely Acidovorax, Aquabacterium, and Hydrogenophaga at the top of the Main Seam Fm., at the bottom of the Cypris Fm. and especially in the intercalated zones seem to be correlated to a mantle-derived CO2 influence as well (Figures 5A,E). The genera Acidovorax and Hydrogenphaga are amongst others comprised of facultative anaerobic, iron-oxidizing, and autotrophic hydrogen-oxidizing and CO2-fixing bacteria (Yoon et al., 2008; Byrne-Bailey et al., 2010; Willems and Gillis, 2015; Li et al., 2017). The occurrence of increased Comamonadaceae genera related to high CO2 concentrations was also reported by Ham et al. (2017), who found a predomination of Comamonadaceae in a natural CO2-dominated aquifer in South Korea as well as by Krauze et al. (2017), who detected members of Comamonadaceae in wet mofettes of the Cheb Basin close to the Hartoušov site. Furthermore, Mu et al. (2014) observed an increase of Comamonadaceae after the injection of CO2 into the Paaratte sandstone aquifer (Southern Australia) and a similar result was reported by Gulliver et al. (2018) for the CO2 injection into an aquifer at the freshwater Plant Daniel in Escatawpa (Massachusetts, USA). Although, Comamonadaceae have been found in non-CO2 influenced subsurface environments such as the Sanford Underground Research Facility (Jangir et al., 2019) and the Fennoscandian shield (Nyyssönen et al., 2014), the relative abundances in these environments were lower compared to our results. Thus, the dominance of members from the family Comamonadaceae in the deep sediments of the Hartoušov mofette seem to reflect a CO2 influenced microbial community with a good adaptation potential to the prevailing conditions. Hence, our study assumes that some members of Comamonadaceae, especially the determined sequences of unknown genera as well as Acidovorax and Aquabacterium are very adaptive to CO2-dominated ecosystems and can be suggested as indicator for such environments.
The occurrence of Sulfuricurvum and Sulfurimonas is linked to the high content of the CO2-saturated saline aquifer (Bussert et al., 2017) and shows an increase of Sulfuricurvum at the transition from the Main Seam to the Cypris Fm. and in the intercalated zones within the Cypris Fm. (Figure 5F). An abundant occurrence of the genus Sulfuricurvum in highly CO2-influenced subsurface environments was also reported by Gulliver et al. (2018). Moreover, the genus Sulfurimonas was found in surficial pools of several mofette systems within the Cheb Basin (Krauze et al., 2017) and in a CO2-driven geyser on the Colorado Plateau (Utah, USA) (Probst et al., 2018). Thus, Sulfuricurvum and Sulfurimonas might represent indicator organisms occurring in CO2 influenced ecosystems with a high concentration.
The relationship of the investigated genera from the family Comamonadaceae as well as Sulfuricurvum and Sulfurimonas to the ascending CO2-saturated saline groundwater indicate, that the groundwater acts both, as transport mechanism and main community shaping factor for the deep biosphere. As a result, we assume the following CO2 migration model for the deep sediments of the Hartoušov mofette system. The CO2-saturated groundwater or the CO2 migrates from the Paleozoic basement into the low-permeable CO2-saturated saline aquifer and is trapped by the overlaying Cypris Fm. (Figure 8B). Thereby, related to buoyancy and the permanent CO2 supply from the mantle, the CO2 pressure increases to the top of the Main Seam Fm. with the highest concentrations occurring between 80.5 and 78.5 mbs (Figure 8C), indicated by the CO2 blow out during the drilling campaign (Figure 8A) and an increase in Sulfuricurvum and the uncommon lipid compound groups A and B (Figures 5F,G). This high CO2 pressure causes a widespread diffuse groundwater migration into the lower part of the Cypris Fm. (between 78.5 and 75 mbs) (Figure 8B). Therein both, potentially produced acetate from OM degradation related to a higher TOC content (Figures 2A,C) and the ascending CO2 itself might act as substrates for methanogenic archaea, namely Methanosaeta, and Methanosarcina (Zinder et al., 1985; Patel and Sprott, 1990; Figure 5F). Subsequently, in this core interval part of the isoGDGT signal representing methanogenic archaeal biomass (Schouten et al., 2013; Naeher et al., 2014) might also derive from the current deep biosphere (Figure 3D). This diffuse migration may also be accompanied by a subordinate migration through small fractures (Figure 8B). Afterwards, the groundwater migration seem to change into a more channelized migration (Figure 8B), indicated by the occurrence of the intercalated zones with higher abundances of Acidovorax, Aquabacterium, Hydrogenophaga, and Sulfuricurvum which correlate with fluid migration structures mentioned in the lithological description from the subsampling (73–65 mbs) (Figures 5A,E and Supplementary Figure 1C) and thus point to a CO2 migration related to small subordinate fault zones in decimeter-size. A possible syntrophy within this environmental setup could be based on an anaerobic, heterotrophic lifestyle of Acidovorax, Aquabacterium, and other genera from the family Comamonadaceae (Willems et al., 1990; Kalmbach et al., 1999). The fermentation of OM provides hydrogen and sulfur as substrate for the hydrogen-oxidizing Hydrogenophaga and the sulfur-oxidizer Sulfuricurvum, which both additionally utilize the ascending CO2 for their metabolism (Willems et al., 1989; Kodama and Watanabe, 2004).
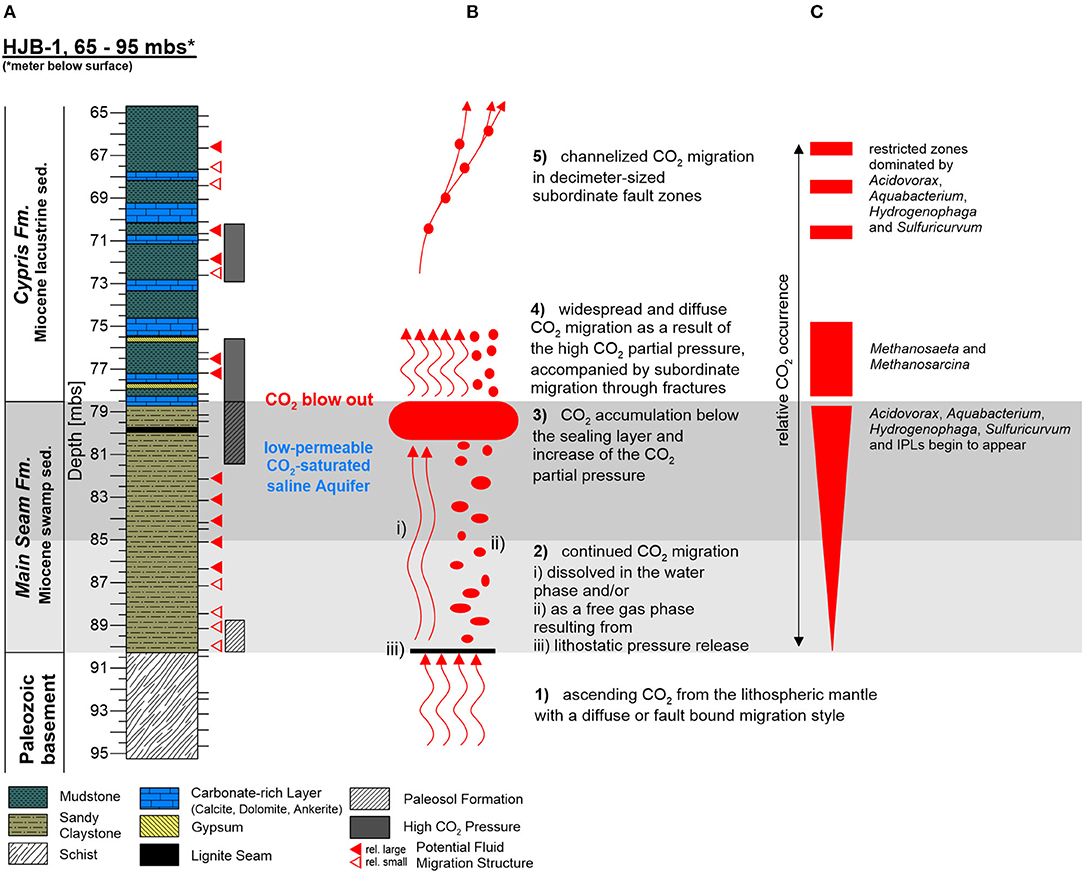
Figure 8. Investigated core section of the Hartoušov mofette core HJB-1 (2016) between 65 and 95 mbs showing (A) the stratigraphical and lithological description, (B) a CO2-migration model with the migration characteristics, and (C) the relative CO2 concentration with the distribution of CO2-related microbial genera.
Conclusion
The lithological setup of the deep Hartoušov mofette system (65–95 mbs) represents a paleoenvironmental change from an Early Miocene terrestrial swamp-like (Main Seam Fm.) to a lacustrine ecosystem (Cypris Fm.). Since Mid Pleistocene time, this system became overprinted by migration and accumulation of mantle-derived CO2 which forms a potential habitat shaping and stimulating deep microbial life.
The necrotic microbial lipid biomarkers essentially reflect the environmental conditions during time of deposition and are therefore unsuitable for tracing the deep biosphere at the Hartoušov mofette site. This already indicates that the current biosphere signal in the deep mofette system is rather small compared to the paleo-microbial biomass.
The overall low abundance of microbial signatures from the deep biosphere in the Hartoušov mofette system suggests that the low-permeable CO2-saturated aquifer interval does not represent a hotspot for deep microbial life as might be expected from a substrate point of view. However, our data indicate that the availability of organic matter as microbial feedstock and CO2 migration are the main community shaping factors in the deep part of the mofette system. In the process, CO2 migration and accumulation occur heterogeneous leading to the formation of niche habitats for CO2-adapted microbial communities independent from the single lithological units of the explored core interval. In addition, our results imply that the high relative abundance of Acidovorax, Aquabacterium, Hydrogenophaga, and unknown genera of the family Comamonadaceae as well as the occurrence of Sulfuricurvum together with high sulfate contents in the CO2-saturated groundwater may be indicative for CO2-dominated deep subsurface ecosystems.
A cluster of yet unknown intact polar membrane lipids displays the presence of microbial life associated to higher accumulations of CO2 in the deep subsurface and show potential to act as lipid biomarkers for such environmental settings.
Data Availability Statement
The datasets generated for this study can be found in the European Nucleotide Archive (http://www.ebi.ac.uk/ena), accession numbers PRJEB22478 (ERS4382097 to ERS4382146 and ERS4382395 to ERS4382400). Lipid data can be found in the Supplementary Material.
Author Contributions
QL and KA wrote the manuscript, performed subsampling, and initial description of the core material as well as PCA analysis in equal manner. QL processed the geomicrobiological analysis, i.e., DNA extractions and purification, gene quantification, and bioinformatical based statistical analyses. KA performed the analysis of the intact and past lipid membrane biomarkers, and conducted together with BP the bulk elemental and bulk stable isotope analysis. FH and DL were involved in 16S rRNA sequencing data processing. PK and DL were involved in statistical analyses. DW, KM, HK, RB, and H-MS gave essential technical advice and contributed to the interpretation of the results and valuable discussion. MA and KM supervised the study and led the writing of the present manuscript. All authors have taken part in the manuscript revisions, interpretation of the results, writing of the manuscript, and agreed with its scientific content.
Funding
This study was supported by the Deutsche Forschungsgemeinschaft (DFG) within the framework of the priority program 1006 International Continental Drilling Program (ICDP) by a grant to MA (AL 1898/1) and KM (MA 2470/5). The drilling of the Hartoušov mofette site and the 16S rRNA gene amplicon (MiSeq) sequencing was financed through the Helmholtz Research Programme Geosystem—the Changing Earth of the GFZ (German Research Centre for Geosciences—Helmholtz Centre Potsdam).
Conflict of Interest
The authors declare that the research was conducted in the absence of any commercial or financial relationships that could be construed as a potential conflict of interest.
Acknowledgments
QL gratefully acknowledges financial support from the China Scholarship Council. KA and KM are grateful to the Deutsche Forschungsgemeinschaft (DFG) for funding the project MA 2470/5 and MA for funding AL 1898/1. The authors would like to thank all involved scientists, technicians, and student research assistants of the GFZ—German Research Centre for Geosciences for support and guidance through the sample preparation, analysis, and interpretation. These important people, who kept the system running are in alphabetical order; Oliver Burckhardt, Anke Kaminsky, Cornelia Karger, Axel Kitte, Sebastian Kreutz, Hartmut Liep, Joana MacLean, Doreen Noak, Sylvia Pinkerneil, Anke Saborowski, and Andrea Vieth-Hillebrand. Finally, we would like to thank the Editor Cassandre Lazar and four reviewers for taking the time to intensely revise our manuscript, which helped us to significantly improve it.
Supplementary Material
The Supplementary Material for this article can be found online at: https://www.frontiersin.org/articles/10.3389/fmicb.2020.543260/full#supplementary-material
References
Bale, N. J., Sorokin, D. Y., Hopmans, E. C., Koenen, M., Rijpstra, W. I. C., Villanueva, L., et al. (2019). New insights into the polar lipid composition of extremely halo(alkali)philic euryarchaea from hypersaline lakes. Front. Microbiol. 10:377. doi: 10.3389/fmicb.2019.00377
Bankwitz, P., Bankwitz, E., Brauer, K., Kampf, H., and Storr, M. (2003a). Deformation structures in plio-and pleistocene sediments (NW Bohemia, central Europe). Spec. Public. Geol. Soc. Lond. 216, 73–93. doi: 10.1144/GSL.SP.2003.216.01.06
Bankwitz, P., Schneider, G., Kämpf, H., and Bankwitz, E. (2003b). Structural characteristics of epicentral areas in Central Europe: study case Cheb Basin (Czech Republic). J. Geodyn. 35, 5–32. doi: 10.1016/S0264-3707(02)00051-0
Beulig, F., Heuer, V. B., Akob, D. M., Viehweger, B., Elvert, M., Herrmann, M., et al. (2015). Carbon flow from volcanic CO2 into soil microbial communities of a wetland mofette. ISME J. 9, 746–759. doi: 10.1038/ismej.2014.148
Beulig, F., Urich, T., Nowak, M., Trumbore, S. E., Gleixner, G., Gilfillan, G. D., et al. (2016). Altered carbon turnover processes and microbiomes in soils under long-term extremely high CO2 exposure. Nat. Microbiol. 1:15025. doi: 10.1038/nmicrobiol.2015.25
Bischoff, J., Mangelsdorf, K., Schwamborn, G., and Wagner, D. (2014). Impact of lake-level and climate changes on microbial communities in a terrestrial permafrost sequence of the el'gygytgyn crater, far east russian arctic. Permaf. Periglac. Process. 25, 107–116. doi: 10.1002/ppp.1807
Blaga, C. I., Reichart, G.-J., Heiri, O., and Sinninghe Damsté, J. S. (2009). Tetraether membrane lipid distributions in water-column particulate matter and sediments: a study of 47 European lakes along a north–south transect. J. Paleolimnol. 41, 523–540. doi: 10.1007/s10933-008-9242-2
Bligh, E. G., and Dyer, W. J. (1959). A rapid method of total lipid extraction and purification. Can. J. Biochem. Physiol. 37, 911–917. doi: 10.1139/y59-099
Boetius, A., Ravenschlag, K., Schubert, C. J., Rickert, D., Widdel, F., Gieseke, A., et al. (2000). A marine microbial consortium apparently mediating anaerobic oxidation of methane. Nature 407, 623–626. doi: 10.1038/35036572
Bolyen, E., Rideout, J. R., Dillon, M. R., Bokulich, N. A., Abnet, C. C., Al-Ghalith, G. A., et al. (2019). Reproducible, interactive, scalable and extensible microbiome data science using QIIME 2. Nat.Biotechnol. 37, 852–857. doi: 10.1038/s41587-019-0209-9
Brandl, P. A., Genske, F. S., Beier, C., Haase, K. M., Sprung, P., and Krumm, S. H. (2015). Magmatic evidence for carbonate metasomatism in the lithospheric mantle underneath the Ohre (Eger) rift. J. Petrol. 56, 1743–1774. doi: 10.1093/petrology/egv052
Bräuer, K., Kämpf, H., Faber, E., Koch, U., Nitzsche, H.-M., and Strauch, G. (2005). Seismically triggered microbial methane production relating to the Vogtland NW bohemia earthquake swarm period 2000, Central Europe. Geochem. J. 39, 441–450. doi: 10.2343/geochemj.39.441
Bräuer, K., Kämpf, H., Koch, U., and Strauch, G. (2011). Monthly monitoring of gas and isotope compositions in the free gas phase at degassing locations close to the Nový Kostel focal zone in the western Eger Rift, Czech Republic. Chem. Geol. 290, 163–176. doi: 10.1016/j.chemgeo.2011.09.012
Bräuer, K., Kämpf, H., Niedermann, S., and Strauch, G. (2018). Monitoring of helium and carbon isotopes in the western Eger Rift area (Czech Republic): relationships with the 2014 seismic activity and indications for recent (2000–2016) magmatic unrest. Chem. Geol. 482, 131–145. doi: 10.1016/j.chemgeo.2018.02.017
Bräuer, K., Kämpf, H., and Strauch, G. (2009). Earthquake swarms in non-volcanic regions: what fluids have to say. Geophys. Res. Lett. 36:L17309. doi: 10.1029/2009GL039615
Buckles, L. K., Villanueva, L., Weijers, J. W. H., Verschuren, D., and Damsté, J. S. S. (2013). Linking isoprenoidal GDGT membrane lipid distributions with gene abundances of ammonia-oxidizing Thaumarchaeota and uncultured crenarchaeotal groups in the water column of a tropical lake (Lake Challa, East Africa). Environ. Microbiol. 15, 2445–2462. doi: 10.1111/1462-2920.12118
Bussert, R., Kämpf, H., Flechsig, C., Hesse, K., Nickschick, T., Liu, Q., et al. (2017). Drilling into an active mofette: pilot-hole study of the impact of CO2-rich mantle-derived fluids on the geo–bio interaction in the western Eger Rift (Czech Republic). Sci. Dril. 23, 13–27. doi: 10.5194/sd-23-13-2017
Byrne-Bailey, K. G., Weber, K. A., Chair, A. H., Bose, S., Knox, T., Spanbauer, T. L., et al. (2010). Completed genome sequence of the anaerobic iron-oxidizing bacterium Acidovorax ebreus strain TPSY. J. Bacteriol. 192, 1475–1476. doi: 10.1128/JB.01449-09
Callahan, B. J., McMurdie, P. J., Rosen, M. J., Han, A. W., Johnson, A. J. A., and Holmes, S. P. (2016). DADA2: High-resolution sample inference from Illumina amplicon data. Nat. Methods 13, 581–583. doi: 10.1038/nmeth.3869
Caporaso, J. G., Lauber, C. L., Walters, W. A., Berg-Lyons, D., Lozupone, C. A., Turnbaugh, P. J., et al. (2011). Global patterns of 16S rRNA diversity at a depth of millions of sequences per sample. Proc. Natl. Acad. Sci. U.S.A. 108(Suppl. 1), 4516–4522. doi: 10.1073/pnas.1000080107
Conrad, R. (2005). Quantification of methanogenic pathways using stable carbon isotopic signatures: a review and a proposal. Organ. Geochem. 36, 739–752. doi: 10.1016/j.orggeochem.2004.09.006
Dahm, T., Hrubcov,á, P., Fischer, T., Horálek, J., Korn, M., Buske, S., et al. (2013). Eger Rift ICDP: an observatory for study of non-volcanic, mid-crustal earthquake swarms and accompanying phenomena. Sci. Dril. 16, 93–99. doi: 10.5194/sd-16-93-2013
Davies, K. L., Pancost, R. D., Edwards, M. E., Walter-Antony, K. M., Langdon, P. G., and Chaves Torres, L. (2016). Diploptene δ13C values from contemporary thermokarst lake sediments show complex spatial variation. Biogeosciences 13, 2611–2621. doi: 10.5194/bg-13-2611-2016
Degelmann, D. M., Borken, W., Drake, H. L., and Kolb, S. (2010). Different atmospheric methane-oxidizing communities in European beech and Norway spruce soils. Appl. Environ. Microbiol. 76, 3228–3235. doi: 10.1128/AEM.02730-09
DeJonge, C., Stadnitskaia, A., Hopmans, E. C., Cherkashov, G., Fedotov, A., and Sinninghe Damsté, J. S. (2014). In situ produced branched glycerol dialkyl glycerol tetraethers in suspended particulate matter from the Yenisei River, Eastern Siberia. Geochim. Cosmochim. Acta 125, 476–491. doi: 10.1016/j.gca.2013.10.031
Dirghangi, S. S., Pagani, M., Hren, M. T., and Tipple, B. J. (2013). Distribution of glycerol dialkyl glycerol tetraethers in soils from two environmental transects in the USA. Organ. Geochem. 59, 49–60. doi: 10.1016/j.orggeochem.2013.03.009
Evans, T. W., Coffinet, S., Könneke, M., Lipp, J. S., Becker, K. W., Elvert, M., et al. (2019). Assessing the carbon assimilation and production of benthic archaeal lipid biomarkers using lipid-RIP. Geochim. Cosmochim. Acta 265, 431–442. doi: 10.1016/j.gca.2019.08.030
Fischer, T., Horálek, J., Hrubcová, P., Vavryčuk, V., Bräuer, K., and Kämpf, H. (2014). Intra-continental earthquake swarms in West-Bohemia and Vogtland: a review. Tectonophysics 611, 1–27. doi: 10.1016/j.tecto.2013.11.001
Fischer, T., Matyska, C., and Heinicke, J. (2017). Earthquake-enhanced permeability – evidence from carbon dioxide release following the M L 3.5 earthquake in West Bohemia. Earth Planet. Sci. Lett. 460, 60–67. doi: 10.1016/j.epsl.2016.12.001
Flechsig, C., Bussert, R., Rechner, J., Schütze, C., and Kämpf, H. (2008). The Hartoušov Mofette field in the Cheb Basin, Western Eger Rift (Czech Republic): a comperative geoelectric, sedimentologic and soil gas study of a magmatic diffuse CO2-degassing structure. Z. Geol. Wiss 36, 177–193.
Flechsig, C., Fabig, T., Rücker, C., and Schütze, C. (2010). Geoelectrical investigations in the cheb basin/W-bohemia: an approach to evaluate the near-surface conductivity structure. Stud. Geophys. Geod. 54, 443–463. doi: 10.1007/s11200-010-0026-6
Frerichs, J., Oppermann, B. I., Gwosdz, S., Möller, I., Herrmann, M., and Krüger, M. (2013). Microbial community changes at a terrestrial volcanic CO2 vent induced by soil acidification and anaerobic microhabitats within the soil column. FEMS Microbiol. Ecol. 84, 60–74. doi: 10.1111/1574-6941.12040
Freymond, C. V., Peterse, F., Fischer, L. V., Filip, F., Giosan, L., and Eglinton, T. I. (2017). Branched GDGT signals in fluvial sediments of the Danube River basin: method comparison and longitudinal evolution. Organ. Geochem. 103, 88–96. doi: 10.1016/j.orggeochem.2016.11.002
Gaines, S. M., Eglinton, G., and Rullkötter, J. (2009). Echoes of Life: What Fossil Molecules Reveal about Earth History. Oxford: Oxford University Press.
Geissler, W. H., Kämpf, H., Seifert, W., and Dulski, P. (2007). Petrological and seismic studies of the lithosphere in the earthquake swarm region Vogtland/NW Bohemia, central Europe. J. Volcanol. Geotherm. Res. 159, 33–69. doi: 10.1016/j.jvolgeores.2006.06.011
Gleixner, G., Danier, H. J., Werner, R. A., and Schmidt, H. L. (1993). Correlations between the 13C content of primary and secondary plant products in different cell compartments and that in decomposing basidiomycetes. Plant Physiol. 102, 1287–1290.
Gulliver, D., Lipus, D., Ross, D., and Bibby, K. (2018). Insights into microbial community structure and function from a shallow, simulated CO2-leakage aquifer demonstrate microbial selection and adaptation. Environ. Microbiol. Rep. 11, 338–351. doi: 10.1111/1758-2229.12675
Ham, B., Choi, B.-Y., Chae, G.-T., Kirk, M. F., and Kwon, M. J. (2017). Geochemical influence on microbial communities at CO2-leakage analog sites. Front. Microbiol. 8:2203. doi: 10.3389/fmicb.2017.02203
Hammer, Ø., Harper, D. A. T., and Ryan, P. D. (2001). PAST-palaeontological statistics, ver. 1.89. Palaeontol. Electron 4, 1–9.
Heuer, B., Geissler, W. H., Kind, R., and Kämpf, H. (2006). Seismic evidence for asthenospheric updoming beneath the western Bohemian Massif, central Europe. Geophys. Res. Lett. 33:L05311. doi: 10.1029/2005GL025158
Heuer, V. B., Pohlman, J. W., Torres, M. E., Elvert, M., and Hinrichs, K.-U. (2009). The stable carbon isotope biogeochemistry of acetate and other dissolved carbon species in deep subseafloor sediments at the northern Cascadia Margin. Geochim. Cosmochim. Acta 73, 3323–3336. doi: 10.1016/j.gca.2009.03.001UR
Hohberg, K., Schulz, H.-J., Balkenhol, B., Pilz, M., Thomalla, A., Russell, D. J., et al. (2015). Soil faunal communities from mofette fields: effects of high geogenic carbon dioxide concentration. Soil Biol. Biochem. 88, 420–429. doi: 10.1016/j.soilbio.2015.06.017
Huang, X., Meyers, P. A., Xue, J., Gong, L., Wang, X., and Xie, S. (2015). Environmental factors affecting the low temperature isomerization of homohopanes in acidic peat deposits, central China. Geochim. Cosmochim. Acta 154, 212–228. doi: 10.1016/j.gca.2015.01.031
Huguet, A., Meador, T. B., Laggoun-Défarge, F., Könneke, M., Wu, W., Derenne, S., et al. (2017). Production rates of bacterial tetraether lipids and fatty acids in peatland under varying oxygen concentrations. Geochim. Cosmochim. Acta 203, 103–116. doi: 10.1016/j.gca.2017.01.012
Inglis, G. N., Naafs, B. D. A., Zheng, Y., McClymont, E. L., Evershed, R. P., and Pancost, R. D. (2018). Distributions of geohopanoids in peat: implications for the use of hopanoid-based proxies in natural archives. Geochim. Cosmochim. Acta 224, 249–261. doi: 10.1016/j.gca.2017.12.029
Jangir, Y., Karbelkar, A. A., Beedle, N. M., Zinke, L. A., Wanger, G., Anderson, C. M., et al. (2019). In situ electrochemical studies of the terrestrial deep subsurface biosphere at the sanford underground research facility, South Dakota, USA. Front. Energy Res. 7:121. doi: 10.3389/fenrg.2019.00121
Kalmbach, S., Manz, W., Wecke, J., and Szewzyk, U. (1999). Aquabacterium gen. nov., with description of Aquabacterium citratiphilum sp. nov., Aquabacterium parvum sp. nov. and Aquabacterium commune sp. nov., three in situ dominant bacterial species from the Berlin drinking water system. Int. J. Syst. Bacteriol. 49(Pt. 2), 769–777. doi: 10.1099/00207713-49-2-769
Kämpf, H., and Bankwitz, P. (2005). “Active processes of the western Eger Graben area, in the KTB deep crustal laboratory and the western Eger Graben,” in Schriftreihe der Deutschen Gesellschaft für Geowissenschaften, Vol. 40, eds H. Kämpf, A. Petrek, J. Rohrmüller, H. J. Kümpel, W. H. Geissler, 78–82.
Kämpf, H., Bräuer, K., Schumann, J., Hahne, K., and Strauch, G. (2013). CO2 discharge in an active, non-volcanic continental rift area (Czech Republic): characterisation (δ13C, 3He/4He) and quantification of diffuse and vent CO2 emissions. Chem. Geol. 339, 71–83. doi: 10.1016/j.chemgeo.2012.08.005
Kämpf, H., Broge, A. S., Marzban, P., Allahbakhshi, M., and Nickschick, T. (2019). Nonvolcanic carbon dioxide emission at continental rifts: the bublak Mofette Area, Western Eger Rift, Czech Republic. Geofluids 2019:4852706. doi: 10.1155/2019/4852706
Kodama, Y., and Watanabe, K. (2004). Sulfuricurvum kujiense gen. nov., sp. nov., a facultatively anaerobic, chemolithoautotrophic, sulfur-oxidizing bacterium isolated from an underground crude-oil storage cavity. Int. J. Systemat. Evol. Microbiol. 54, 2297–2300. doi: 10.1099/ijs.0.63243-0
Krauze, P., Kämpf, H., Horn, F., Liu, Q., Voropaev, A., Wagner, D., et al. (2017). Microbiological and geochemical survey of CO2-dominated mofette and mineral waters of the Cheb Basin, Czech republic. Front. Microbiol. 8:2446. doi: 10.3389/fmicb.2017.02446
Krmíček, L., Timmerman, M. J., Ziemann, M. A., Sudo, M., and Ulrych, J. (2020). 40Ar/39Ar step-heating dating of phlogopite and kaersutite megacrysts from the Železná hurka (Eisenbühl) Pleistocene scoria cone, Czech Republic. Geol. Carpathica 71, 382–387. doi: 10.31577/GeolCarp.71.4.6
Krüger, M., West, J., Frerichs, J., Oppermann, B., Dictor, M.-C., Jouliand, C., et al. (2009). Ecosystem effects of elevated CO2 concentrations on microbial populations at a terrestrial CO2 vent at Laacher See, Germany. Energy Procedia 1, 1933–1939. doi: 10.1016/j.egypro
Li, C., Zhong, S., Zhang, F., Wang, Z., Jiang, F., and Wan, Y. (2017). Response of microbial communities to supercritical CO2 and biogeochemical influences on microbially mediated CO2-saline-sandstone interactions. Chem. Geol. 473, 1–9. doi: 10.1016/j.chemgeo.2017.09.026
Lied, P., Kontny, A., Nowaczyk, N., Mrlina, J., and Kämpf, H. (2020). Cooling rates of pyroclastic deposits inferred from mineral magnetic investigations: a case study from the Pleistocene Mýtina Maar (Czech Republic). Int. J. Earth Sci. 109, 1707–1725. doi: 10.1007/s00531-020-01865-1
Liu, Q., Kämpf, H., Bussert, R., Krauze, P., Horn, F., Nickschick, T., et al. (2018). Influence of CO2 degassing on the microbial community in a dry mofette field in hartoušov, czech republic (Western Eger Rift). Front. Microbiol. 9:2787. doi: 10.3389/fmicb.2018.02787
Logemann, J., Graue, J., Köster, J., Engelen, B., Rullkötter, J., and Cypionka, H. (2011). A laboratory experiment of intact polar lipid degradation in sandy sediments. Biogeosciences 8, 2547–2560. doi: 10.5194/bg-8-2547-2011
Mangelsdorf, K., Karger, C., and Zink, K.-G. (2019). “Phospholipids as life markers in geological habitats,” in Hydrocarbons, Oils and Lipids: Diversity, Origin, Chemistry and Fate, ed H. Wilkes (Cham: Springer), 1–29.
Martin, M. (2011). Cutadapt removes adapter sequences from high-throughput sequencing reads. EMBnet J. 17:10. doi: 10.14806/ej.17.1.200
McMurdie, P. J., and Holmes, S. (2013). phyloseq: an R package for reproducible interactive analysis and graphics of microbiome census data. PLoS ONE 8:e61217. doi: 10.1371/journal.pone.0061217
Mehlhorn, J., Besold, J., Lezama Pacheco, J. S., Gustafsson, J. P., Kretzschmar, R., and Planer-Friedrich, B. (2018). Copper mobilization and immobilization along an organic matter and redox gradient-insights from a mofette site. Environ. Sci. Technol. 52, 13698–13707. doi: 10.1021/acs.est.8b02668
Mehlhorn, J., Byrne, J. M., Kappler, A., and Planer-Friedrich, B. (2016). Time and temperature dependency of carbon dioxide triggered metal(loid) mobilization in soil. Appl. Geochem. 74, 122–137. doi: 10.1016/j.apgeochem.2016.09.007
Meyers, P. A. (1997). Organic geochemical proxies of paleoceanographic, paleolimnologic, and paleoclimatic processes. Organ. Geochem. 27, 213–250. doi: 10.1016/S0146-6380(97)00049-1
Meyers, P. A. (2003). Applications of organic geochemistry to paleolimnological reconstructions: a summary of examples from the Laurentian Great lakes. Organ. Geochem. 34, 261–289. doi: 10.1016/S0146-6380(02)00168-7
Mrlina, J., Kämpf, H., Geissler, W., and van den Bogaard, P. (2007). Assumed quaternary maar structure at the Czech/German border between Mytina and Neualbenreuth (western Eger Rift, Central Europe): geophysical, petrochemical and geochronological indications. Z. Geol. Wiss. 35, 213–230. doi: 10.3989/mc.2004.v54.i276.257
Mu, A., Boreham, C., Leong, H. X., Haese, R. R., and Moreau, J. W. (2014). Changes in the deep subsurface microbial biosphere resulting from a field-scale CO2 geosequestration experiment. Front. Microbiol. 5:209. doi: 10.3389/fmicb.2014.00209
Müller, K.-D., Nalik, H. P., Schmid, E. N., Husmann, H., and Schomburg, G. (1993). Fast identification of mycobacterium species by GC analysis with trimethylsulfonium hydroxide (TMSH) for transesterification. J. High Resol. Chromatogr. 16, 161–165. doi: 10.1002/jhrc.1240160306
Naafs, B. D. A., Inglis, G. N., Zheng, Y., Amesbury, M. J., Biester, H., Bindler, R., et al. (2017). Introducing global peat-specific temperature and pH calibrations based on brGDGT bacterial lipids. Geochim. Cosmochim. Acta 208, 285–301. doi: 10.1016/j.gca.2017.01.038
Naeher, S., Niemann, H., Peterse, F., Smittenberg, R. H., Zigah, P. K., and Schubert, C. J. (2014). Tracing the methane cycle with lipid biomarkers in Lake Rotsee (Switzerland). Organ. Geochem. 66, 174–181. doi: 10.1016/j.orggeochem.2013.11.002
Nealson, K. H. (1997). Microorganisms and biogeochemical cycles:what can we learn from layered microbial communities? Rev. Mineral. 35, 3–34.
Nickschick, T., Flechsig, C., Meinel, C., Mrlina, J., and Kämpf, H. (2017). Architecture and temporal variations of a terrestrial CO2 degassing site using electric resistivity tomography and self-potential. Int. J. Earth Sci. 106, 2915–2926. doi: 10.1007/s00531-017-1470-0
Nickschick, T., Flechsig, C., Mrlina, J., Oppermann, F., and Günther, T. (2019). Large-scale electrical resistivity tomography in the Cheb Basin (Eger Rift) at an ICDP monitoring drill site to image fluid-related structures. Solid Earth 10, 1951–1969. doi: 10.5194/se-2019-38
Nickschick, T., Kämpf, H., Flechsig, C., Mrlina, J., and Heinicke, J. (2015). CO2 degassing in the Hartoušov mofette area, western Eger Rift, imaged by CO2 mapping and geoelectrical and gravity surveys. Int. J. Earth Sci. 104, 2107–2129. doi: 10.1007/s00531-014-1140-4
Nowak, M. E., Beulig, F., Fischer, J., von Muhr, J., Küsel, K., and Trumbore, S. E. (2015). Autotrophic fixation of geogenic CO2 by microorganisms contributes to soil organic matter formation and alters isotope signatures in a wetland mofette. Biogeosciences 12, 7169–7183. doi: 10.5194/bg-12-7169-2015
Nyyssönen, M., Hultman, J., Ahonen, L., Kukkonen, I., Paulin, L., Laine, P., et al. (2014). Taxonomically and functionally diverse microbial communities in deep crystalline rocks of the Fennoscandian shield. ISME J. 8, 126–138. doi: 10.1038/ismej.2013.125
Oppermann, B. I., Michaelis, W., Blumenberg, M., Frerichs, J., Schulz, H. M., Schippers, A., et al. (2010). Soil microbial community changes as a result of long-term exposure to a natural CO2 vent. Geochim. Cosmochim. Acta 74, 2697–2716. doi: 10.1016/j.gca.2010.02.006
Orsi, W. D., Schink, B., Buckel, W., and Martin, W. F. (2020). Physiological limits to life in anoxic subseafloor sediment. FEMS Microbiol. Rev. 44, 219–231. doi: 10.1093/femsre/fuaa004
Ourisson, G., Albrecht, P., and Rohmer, M. (1979). Paleochemistry and biochemistry of a group of natural products: the hopanoids. Pure Appl. Chem. 51, 709–729. doi: 10.1351/pac197951040709
Pancost, R. D., McClymont, E. L., Bingham, E. M., Roberts, Z., Charman, D. J., Hornibrook, E. R. C., et al. (2011). Archaeol as a methanogen biomarker in ombrotrophic bogs. Organ. Geochem. 42, 1279–1287. doi: 10.1016/j.orggeochem.2011.07.003
Patel, G. B., and Sprott, G. D. (1990). Methanosaeta concilii gen. nov., sp. nov. (“Methanothrix concilii”) and Methanosaeta thermoacetophila nom. rev., comb. nov. Int. J. Syst. Bacteriol. 40, 79–82. doi: 10.1099/00207713-40-1-79
Pellizzari, L., Neumann, D., Alawi, M., Voigt, D., Norden, B., and Würdemann, H. (2013). The use of tracers to assess drill-mud penetration depth into sandstone cores during deep drilling: method development and application. Environ. Earth Sci. 70, 3727–3738. doi: 10.1007/s12665-013-2715-2
Pešek, J. (2014). Tertiary Basins and Lignite Deposits of the Czech Republic. Prague: Czech Geological Survey.
Probst, A. J., Ladd, B., Jarett, J. K., Geller-McGrath, D. E., Sieber, C. M. K., Emerson, J. B., et al. (2018). Differential depth distribution of microbial function and putative symbionts through sediment-hosted aquifers in the deep terrestrial subsurface. Nat. Microbiol. 3, 328–336. doi: 10.1038/s41564-017-0098-y
Quast, C., Pruesse, E., Yilmaz, P., Gerken, J., Schweer, T., Yarza, P., et al. (2013). The SILVA ribosomal RNA gene database project: improved data processing and web-based tools. Nucleic Acids Res. 41, D590–D596. doi: 10.1093/nar/gks1219
Quirk, M. M., Wardroper, A. M. K., Wheatley, R. E., and Maxwell, J. R. (1984). Extended hopanoids in peat environments. Chem. Geol. 42, 25–43. doi: 10.1016/0009-2541(84)90003-2
Radke, M., Willsch, H., and Welte, D. H. (1980). Preparative hydrocarbon group type determination by automated medium pressure liquid chromatography. Anal. Chem. 52, 406–411. doi: 10.1021/ac50053a009
Rennert, T., Eusterhues, K., Pfanz, H., and Totsche, K. U. (2011). Influence of geogenic CO2 on mineral and organic soil constituents on a mofette site in the NW Czech Republic. Eur. J. Soil Sci. 62, 572–580. doi: 10.1111/j.1365-2389.2011.01355.x
Rennert, T., and Pfanz, H. (2016). Hypoxic and acidic — soils on mofette fields. Geoderma 280, 73–81. doi: 10.1016/j.geoderma.2016.06.019
Rognes, T., Flouri, T., Nichols, B., Quince, C., and Mahé, F. (2016). VSEARCH: a versatile open source tool for metagenomics. PeerJ 4:e2584. doi: 10.7717/peerj.2584
Rohrmüller, J., Kämpf, H., Geiß, E., Großmann, J., Grun, I., Mingram, J., et al. (2018). Reconnaissance study of an inferred Quaternary maar structure in the western part of the Bohemian Massif near Neualbenreuth, NE-Bavaria (Germany). Int. J. Earth Sci. 107, 1381–1405. doi: 10.1007/s00531-017-1543-0
Rütters, H., Sass, H., Cypionka, H., and Rullkötter, J. (2001). Monoalkylether phospholipids in the sulfate-reducing bacteria Desulfosarcina variabilis and Desulforhabdus amnigenus. Arch. Microbiol. 176, 435–442. doi: 10.1007/s002030100343
Sáenz, J. P., Grosser, D., Bradley, A. S., Lagny, T. J., Lavrynenko, O., Broda, M., et al. (2015). Hopanoids as functional analogues of cholesterol in bacterial membranes. Proc. Natl. Acad. Sci. U.S.A. 112, 11971–11976. doi: 10.1073/pnas.1515607112
Sandig, C., Sauer, U., Bräuer, K., Serfling, U., and Schütze, C. (2014). Comparative study of geophysical and soil–gas investigations at the Hartoušov (Czech Republic) natural CO2 degassing site. Environ. Earth Sci. 72, 1421–1434. doi: 10.1007/s12665-014-3242-5
Sauer, U., Watanabe, N., Singh, A., Dietrich, P., Kolditz, O., and Schütze, C. (2014). Joint interpretation of geoelectrical and soil-gas measurements for monitoring CO 2 releases at a natural analogue. Near Surface Geophys. 12, 165–178. doi: 10.3997/1873-0604.2013052
Schouten, S., Hopmans, E. C., and Sinninghe Damsté, J. S. (2013). The organic geochemistry of glycerol dialkyl glycerol tetraether lipids: a review. Organ. Geochem. 54, 19–61. doi: 10.1016/j.orggeochem.2012.09.006
Schouten, S., Huguet, C., Hopmans, E. C., Kienhuis, M. V. M., and Damsté, J. S. S. (2007). Analytical methodology for TEX86 paleothermometry by high-performance liquid chromatography/atmospheric pressure chemical ionization-mass spectrometry. Anal. Chem. 79, 2940–2944. doi: 10.1021/ac062339v
Schubotz, F., Xie, S., Lipp, J. S., Hinrichs, K.-U., and Wakeham, S. G. (2018). Intact polar lipids in the water column of the eastern tropical North Pacific: abundance and structural variety of non-phosphorus lipids. Biogeosciences 15, 6481–6501. doi: 10.5194/bg-15-6481-2018
Schuessler, J. A., Kämpf, H., Koch, U., and Alawi, M. (2016). Earthquake impact on iron isotope signatures recorded in mineral spring water. J. Geophys. Res. Solid Earth 121, 8548–8568. doi: 10.1002/2016JB013408
Seifert, W., and Kämpf, H. (1994). Ba-enrichment in phlogopite of a nephelinite from Bohemia. Eur. J. Mineral. 497–502. doi: 10.1127/ejm/6/4/0497
Sheik, C. S., Reese, B. K., Twing, K. I., Sylvan, J. B., Grim, S. L., Schrenk, M. O., et al. (2018). Identification and removal of contaminant sequences from ribosomal gene databases: lessons from the census of deep life. Front. Microbiol. 9:840. doi: 10.3389/fmicb.2018.00840
Šibanc, N., Dumbrell, A. J., Mandić-Mulec, I., and Maček, I. (2014). Impacts of naturally elevated soil CO2 concentrations on communities of soil archaea and bacteria. Soil Biol. Biochem. 68, 348–356. doi: 10.1016/j.soilbio.2013.10.018
Smejkal, V. (1984). Isotopic Composition of Carbonates and Differences in Deposition Environment During the Miocene Lacustrine Sedimentation in the Krusne Hory Graben. 0323-8776, 372-379UR. Available online at: https://pascal-francis.inist.fr/vibad/index.php?action=getRecordDetail&idt=9271524
Summons, R. E., Bradley, A. S., Jahnke, L. L., and Waldbauer, J. R. (2006). Steroids, triterpenoids and molecular oxygen. Philos. Trans. R. Soc. B Biol. Sci. 361, 951–968. doi: 10.1098/rstb.2006.1837
Talbot, H. M., McClymont, E. L., Inglis, G. N., Evershed, R. P., and Pancost, R. D. (2016). Origin and preservation of bacteriohopanepolyol signatures in Sphagnum peat from Bissendorfer Moor (Germany). Organ. Geochem. 97, 95–110. doi: 10.1016/j.orggeochem.2016.04.011
Tierney, J. E., Schouten, S., Pitcher, A., Hopmans, E. C., and Sinninghe Damsté, J. S. (2012). Core and intact polar glycerol dialkyl glycerol tetraethers (GDGTs) in Sand Pond, Warwick, Rhode Island (USA): insights into the origin of lacustrine GDGTs. Geochim. Cosmochim. Acta 77, 561–581. doi: 10.1016/j.gca.2011.10.018
Vylita, T., Žák, K., Cílek, V., Hercman, H., and Mikšíková, L. (2007). Evolution of hot-spring travertine accumulation in Karlovy Vary/Carlsbad (Czech Republic) and its significance for the evolution of Teplá valley and Ohre/Eger rift. Zeitschrift für Geomorphologie 51, 427–442. doi: 10.1127/0372-8854/2007/0051-0427
Wagner, C., Mau, M., Schlömann, M., Heinicke, J., and Koch, U. (2007). Characterization of the bacterial flora in mineral waters in upstreaming fluids of deep igneous rock aquifers. J. Geophys. Res. 112:1583. doi: 10.1029/2005JG000105
Weijers, J. W. H., Panoto, E., van Bleijswijk, J., Schouten, S., Rijpstra, W. I. C., Balk, M., et al. (2009). Constraints on the biological source(s) of the Orphan branched tetraether membrane lipids. Geomicrobiol. J. 26, 402–414. doi: 10.1080/01490450902937293
Weijers, J. W. H., Schouten, S., van den Donker, J. C., Hopmans, E. C., and Sinninghe Damst,é, J. S. (2007). Environmental controls on bacterial tetraether membrane lipid distribution in soils. Geochim. Cosmochim. Acta 71, 703–713. doi: 10.1016/j.gca.2006.10.003
Weijers, J. W. H., Wiesenberg, G. L. B., Bol, R., Hopmans, E. C., and Pancost, R. D. (2010). Carbon isotopic composition of branched tetraether membrane lipids in soils suggest a rapid turnover and a heterotrophic life style of their source organism(s). Biogeosciences 7, 2959–2973. doi: 10.5194/bg-7-2959-2010
Weinlich, F. H., Bräuer, K., Kämpf, H., Strauch, G., Tesar, J., and Weise, S. M. (1999). An active subcontinental mantle volatile system in the western Eger rift, Central Europe: gas flux, isotopic (He, C, and N) and compositional fingerprints. Geochim. Cosmochim. Acta 63, 3653–3671. doi: 10.1016/S0016-7037(99)00187-8
Weise, S. M., Bräuer, K., Kämpf, H., Strauch, G., and Koch, U. (2001). Transport of mantle volatiles through the crust traced by seismically released fluids: a natural experiment in the earthquake swarm area Vogtland/NW Bohemia, Central Europe. Tectonophysics 336, 137–150. doi: 10.1016/S0040-1951(01)00098-1
Werth, M., and Kuzyakov, Y. (2010). 13C fractionation at the root–microorganisms–soil interface: a review and outlook for partitioning studies. Soil Biol. Biochem. 42, 1372–1384. doi: 10.1016/j.soilbio.2010.04.009
White, D. C., Davis, W. M., Nickels, J. S., King, J. D., and Bobbie, R. J. (1979). Determination of the sedimentary microbial biomass by extractible lipid phosphate. Oecologia 40, 51–62. doi: 10.1007/BF00388810
Whiticar, M. J. (1999). Carbon and hydrogen isotope systematics of bacterial formation and oxidation of methane. Chem. Geol. 161, 291–314. doi: 10.1016/S0009-2541(99)00092-3
Willems, A., Falsen, E., Pot, B., Jantzen, E., Hoste, B., Vandamme, P., et al. (1990). Acidovorax, a new genus for Pseudomonas facilis, Pseudomonas delafieldii, E. Falsen (EF) group 13, EF group 16, and several clinical isolates, with the species Acidovorax facilis comb. nov., Acidovorax delafieldii comb. nov., and Acidovorax temperans sp. nov. Int. J. Syst. Bacteriol. 40, 384–398. doi: 10.1099/00207713-40-4-384
Willems, A., and Gillis, M. (2015). “Hydrogenophaga,” in Bergey's Manual of Systematics of Archaea and Bacteria, ed Whitman (Chichester: John Wiley & Sons, Inc.), 1–15.
Willems, A.J, Busse, M., Goor, B., Pot, E., Falsen, E., et al. (1989). Hydrogenophaga, a new genus of hydrogen-oxidizing bacteria that includes Hydrogenophaga flava comb. nov. (Formerly Pseudomonas flava), Hydrogenophaga palleronii (Formerly Pseudomonas palleronii), Hydrogenophaga pseudoflava (Formerly Pseudomonas pseudoflava and “Pseudomonas carboxydoflava”), and Hydrogenophaga taeniospiralis (Formerly Pseudomonas taeniospiralis). Int. J. Syst. Evol. Microbiol. 39, 319–333. doi: 10.1099/00207713-39-3-319
Yoon, K.-S., Tsukada, N., Sakai, Y., Ishii, M., Igarashi, Y., and Nishihara, H. (2008). Isolation and characterization of a new facultatively autotrophic hydrogen-oxidizing Betaproteobacterium, Hydrogenophaga sp. AH-24. FEMS Microbiol. Lett. 278, 94–100. doi: 10.1111/j.1574-6968.2007.00983.x
Zinder, S. H., Sowers, K. R., and Ferry, J. G. (1985). NOTES: Methanosarcina thermophila sp. nov., a thermophilic, acetotrophic, methane-producing bacterium. Int. J. Syst. Bacteriol. 35, 522–523. doi: 10.1099/00207713-35-4-522
Zink, K.-G., and Mangelsdorf, K. (2004). Efficient and rapid method for extraction of intact phospholipids from sediments combined with molecular structure elucidation using LC–ESI-MS–MS analysis. Anal. Bioanal. Chem. 380, 798–812. doi: 10.1007/s00216-004-2828-2
Keywords: geo-bio interaction, CO2, mofette systems, Eger Rift, microbial lipid biomarker, microbial diversity, deep biosphere, saline groundwater
Citation: Liu Q, Adler K, Lipus D, Kämpf H, Bussert R, Plessen B, Schulz H-M, Krauze P, Horn F, Wagner D, Mangelsdorf K and Alawi M (2020) Microbial Signatures in Deep CO2-Saturated Miocene Sediments of the Active Hartoušov Mofette System (NW Czech Republic). Front. Microbiol. 11:543260. doi: 10.3389/fmicb.2020.543260
Received: 16 March 2020; Accepted: 20 November 2020;
Published: 14 December 2020.
Edited by:
Cassandre Sara Lazar, Université du Québec à Montréal, CanadaReviewed by:
William D. Orsi, Ludwig Maximilian University of Munich, GermanyAndreas Teske, University of North Carolina at Chapel Hill, United States
Copyright © 2020 Liu, Adler, Lipus, Kämpf, Bussert, Plessen, Schulz, Krauze, Horn, Wagner, Mangelsdorf and Alawi. This is an open-access article distributed under the terms of the Creative Commons Attribution License (CC BY). The use, distribution or reproduction in other forums is permitted, provided the original author(s) and the copyright owner(s) are credited and that the original publication in this journal is cited, in accordance with accepted academic practice. No use, distribution or reproduction is permitted which does not comply with these terms.
*Correspondence: Kai Mangelsdorf, ay5tYW5nZWxzZG9yZkBnZnotcG90c2RhbS5kZQ==
†These authors share first authorship