- Department of Clinical Laboratory, Peking University People’s Hospital, Beijing, China
Carbapenemase-producing Enterobacterales have become a severe public health concern because of their rapidly transmissible resistance elements and limited treatment options. The most effective antimicrobial combinations against carbapenemase-producing Enterobacterales are currently unclear. Here, we aimed to assess the therapeutic effects of seven antimicrobial combinations (colistin-meropenem, colistin-tigecycline, colistin-rifampicin, colistin-erythromycin, meropenem-tigecycline, meropenem-rifampicin, and meropenem-tigecycline-colistin) against twenty-four carbapenem-producing Enterobacterales (producing blaKPC, blaNDM, coexisting blaNDM and blaIMP, and coexisting mcr-1/8/9 and blaNDM genes) and one carbapenem-susceptible Enterobacterales using the checkerboard assay, time-kill curves, and scanning electron microscopy. None of the combinations were antagonistic. The combination of colistin-rifampicin showed the highest synergistic effect of 76% (19/25), followed by colistin-erythromycin at 60% (15/25), meropenem-rifampicin at 24% (6/25), colistin-meropenem at 20% (5/25), colistin-tigecycline at 20% (5/25), and meropenem-tigecycline at 4% (1/25). The triple antimicrobial combinations of meropenem-tigecycline-colistin had a synergistic effect of 100%. Most double antimicrobial combinations were ineffective on isolates with coexisting blaNDM and blaIMP genes. Meropenem with tigecycline showed no synergistic effect on isolates that produced different carbapenemase genes and were highly resistant to meropenem (92% meropenem MIC ≥ 16 mg/mL). Colistin-tigecycline showed no synergistic effect on Escherichia coli producing blaNDM–1 and Serratia marcescens. Time-kill curves showed that antimicrobial combinations achieved an eradication effect (≥ 3 log10 decreases in colony counts) within 24 h without regrowth, based on 1 × MIC of each drug. The synergistic mechanism of colistin-rifampicin may involve the colistin-mediated disruption of bacterial membranes, leading to severe alterations in their permeability, then causes more rifampicin to enter the cell and induces cell death. In conclusion, the antimicrobial combinations evaluated in this study may facilitate the successful treatment of patients infected with carbapenemase-producing pathogens.
Introduction
During the last decade, carbapenemase-producing Enterobacterales (CPE) have gradually become the main pathogen responsible for significant hospital-acquired infections. Because of limited therapeutic options, infections caused by these “super bacteria” are associated with high mortality rates (Logan and Weinstein, 2017). In 2017, the World Health Organization’s priority pathogens list indicated that development of novel antibiotics to treat carbapenem-resistant Enterobacterales (CRE) was urgently required (Shrivastava et al., 2018). However, in the current post-antibiotic era, novel antibiotics for treating CRE infections are unavailable owing to the lengthy process of drug discovery and low success rate, which has become a serious concern over the past decade (Luepke et al., 2017). Drug-resistant pathogens are resistant to the most frequently used antibiotics and second-line drugs, resulting in an increased burden of infectious diseases (WHO Pathogens Priority List Working Group, 2018). Thus, antimicrobial combinations may offer an alternative for treating CRE pathogens that are resistant to most available therapies.
Among the many mechanisms that mediate CRE resistance, carbapenemase production is the most common (Nordmann et al., 2011). A series of carbapenemases have been identified in Enterobacterales. Three classes of β-lactamases often exist in carbapenem-resistant Enterobacterales: Ambler classes A, B, and D. Class A and D β-lactamases have serine-based hydrolytic activities, and class B consists of metallo-β-lactamases with zinc in their active site (Merie Queenan and Bush, 2007). The β-lactamase inhibitors currently available for clinical use consist only of serine inhibitors. For instance, ceftazidime-avibactam works against CRE strains producing Klebsiella pneumoniae carbapenemases (KPCs) and OXA-48 but shows no activity against class B β-lactamases. So far, there are no alternative drugs to combat the production of metallo-β-lactamase isolates (Shields et al., 2018).
Combination therapy is a method wherein two or more active antibiotics are used together. This method reduces the frequency of drug resistance and minimizes the dosage of toxic drugs, achieving more significant effects than monotherapy in biochemical activity (Fitzgerald et al., 2006). The Chinese XDR Consensus Working Group (Guan et al., 2016) and most retrospective studies (Nabarro and Veeraraghavan, 2015; Bassetti et al., 2016; Trecarichi and Tumbarello, 2017; Wang et al., 2019) have reported that combination therapy is more effective than monotherapy. However, the advantages of combination therapy remain debatable because different infectious pathogens produce different carbapenemase genes and have different levels of resistance. Investigations focusing on antibiotic combinations that are most effective for treating infections caused by these isolates are limited.
In this study, we explored the synergistic effect of seven antimicrobial combinations against 24 CPE (producing blaKPC, blaNDM, both blaNDM and blaIMP, and both mcr-1/8/9 and blaNDM genes) and one carbapenem-susceptible Enterobacterales (CSE) in vitro. In addition, the antibacterial synergistic mechanism of colistin with rifampicin was tested using scanning electron microscopy (SEM). The aim of this study was to study the most effective antimicrobial combinations against carbapenemase-producing Enterobacterales in vitro activity.
Materials and Methods
Microbiological Characteristics of CRE and CSE Isolates
Twenty-five clinical isolates were retrospectively collected from 16 tertiary hospitals in China in 2013–2018 years. The isolates were sent to Peking University People’s Hospital for reappraisal of both resistance mechanisms and antimicrobial susceptibility testing (AST). The isolates were identified by matrix-assisted laser desorption ionization-time of flight mass spectrometry (Bruker Daltonics Inc., Billerica, MA, United States) or a Vitek 2 compact system (BioMérieux Vitek Inc., Hazelwood, MO, United States). Minimum inhibitory concentrations (MICs) were determined by broth microdilution methods according to the CLSI document M100-S30.1 For all CPE isolates, polymerase chain reaction (PCR) was used to detect carbapenemase genes (blaKPC, blaNDM, and blaIMP) as previously described (Yigit et al., 2008; Xiaojuan et al., 2014; Khodadadian et al., 2018). The colistin-resistant genes mcr-1, mcr-8, and mcr-9 were also detected by PCR as previously described (Quan et al., 2017; Wang et al., 2018; Yuan et al., 2019). Multilocus sequence typing (MLST) was confirmed according to the Pasteur Institute MLST website2 for K. pneumoniae and the MLST websites for Escherichia coli3 and Enterobacter cloacae.4
Synergy Testing by Checkerboard Assay
The synergy of double or triple antimicrobial combinations were determined using the standard broth microdilution checkerboard assay as described previously (Berenbaum, 1978; Yoon et al., 2004). In brief, the MICs of antimicrobials were determined before the experiment. Ninety-six-well microtiter plates were arranged with increasing concentrations of one drug, ranging from 0.125 to 8 × MIC on the x-axis and increasing concentrations of the other drug ranging from 0.125 to 8 × MIC on the y-axis. When using triple antimicrobial combinations, fixed concentrations of the drugs were added into 96-well microtiter plates. The final inoculum in each well was approximately 5 × 105 CFU/mL. The 96-well microtiter plates were incubated at 37°C for 24 h, and turbidity was observed by the naked eye to determine growth. The effects of the antimicrobial combinations were defined according to the fractional inhibitory concentration index (FICI). FICI = (MIC drug A/MIC drug A plus drug B) + (MIC drug B/MIC drug A plus drug B), FICI ≤ 0.5, synergism; 0.5 < FICI ≤ 4, no interaction or FICI > 4, antagonistic (Odds, 2003). With the triple antimicrobial combination, FICI < 1, synergistic, FICI = 1, additive, or FICI > 1, antagonistic (Berenbaum, 1978).
Static Time-Kill Assay
A static time-kill assay was conducted for four isolates according to the previously described methodology (Lin et al., 2018). Two Klebsiella pneumoniae (blaKPC–2, blaNDM–1), 1 E. coli (blaNDM–1), and 1 Serratia marcescens were selected to examine the bactericidal effects. The double and triple antimicrobial combinations colistin-meropenem, colistin-rifampicin, colistin-tigecycline, colistin-erythromycin, and colistin-meropenem-tigecycline were tested. Bacteria (1 × 106 CFU/mL) were inoculated in Mueller-Hinton broth containing antibiotics with continuous shaking overnight at 35°C and 200 rpm in an atmospheric environment. One hundred microliter samples were drawn and then serially diluted at 0, 4, 8, 16, and 24 h, and 50 μL aliquots were smeared on Mueller-Hinton agar plates. After incubating the plates overnight at 35°C, the colonies were counted. Synergy was defined as a decrease of ≥2 log10 CFU/mL between the combination and the most efficient agent alone at 24 h. Bactericidal activity was defined as ≥3 log10 CFU/mL reduction in cell numbers compared to the initial inoculum after 24 h (Doern, 2014).
Scanning Electron Microscopy (SEM)
The colistin-sensitive isolate SF-18-09 was selected to explore the synergistic mechanism of colistin with rifampicin on cellular morphology using SEM as per a previously described method (Zhang et al., 2019). Bacteria at the mid-exponential growth phase (1 × 106 CFU/mL) were added to the final drug concentration according to the checkerboard results, and a no-drug group was used as a control. The cells were incubated for 4 h, as described in the static time-kill assay method. After incubation, samples were transferred to 15 mL polypropylene tubes (Corning, United States) and centrifuged at 10,000 × g for 3 min. The supernatants were discarded, and the bacterial pellets were resuspended and washed in 1 mL of 2.5% glutaraldehyde in phosphate-buffered saline (PBS). The tubes were fixed overnight at 4°C. Once fixed, the tubes were centrifuged again at 10,000 × g for 3 min, and the supernatants were removed. Bacterial pellets were resuspended in 1 mL PBS and then observed using a scanning electron microscope (Hitachi SU8020).
Statistical Analysis
Statistical analysis was performed with the software GraphPad Prism version 8.
Ethical Approval
This study was approved by the research ethics board at Peking University People’s Hospital. As this study was retrospective and participants were anonymized, informed consent was not required.
Results
Microbiological Characteristics of CRE Isolates
Genotypic and phenotypic characteristics of CRE and CSE isolates used in this study are displayed in Table 1, including 11 K. pneumoniae (6 blaKPC, 3 blaNDM, 1 coexisting mcr-8 and blaNDM, and 1 coexisting blaNDM and blaIMP), 6 E. coli (4 coexisting blaNDM and mcr-1, 2 blaNDM), 5 E. cloacae (2 blaNDM, 1 coexisting blaNDM and mcr-9, 1 coexisting blaNDM and blaIMP, and 1 non-carbapenemase producer), 2 K. oxytoca (both coexisting blaNDM and blaIMP), and 1 S. marcescens. The antimicrobials had the following MICs (μg/mL) against all isolates: rifampicin, 8–128; colistin, 0.125–256; meropenem, 0.125–256 (most isolates (23/25) ≥ 16); tigecycline, 0.064–8; and erythromycin, 64–256.
In vitro Evaluation of Synergy Using the Checkerboard Method
We used the broth microdilution checkerboard method to test the following seven antimicrobial combinations: colistin-meropenem, colistin-tigecycline, colistin-rifampicin, colistin-erythromycin, meropenem-tigecycline, meropenem-rifampicin, and colistin-meropenem-tigecycline.
The double antimicrobial combinations of colistin-rifampicin had the highest synergistic effect at 76% (19/25), followed by colistin-erythromycin at 60% (15/25), meropenem-rifampicin at 24% (6/25), colistin-meropenem at 20% (5/25), colistin-tigecycline at 20% (5/25), and meropenem-tigecycline at 4% (1/25). The triple antimicrobial combinations of meropenem-tigecycline-colistin showed a synergistic effect of 100% (16/16). Colistin-tigecycline was ineffective on E. coli (Table 2).
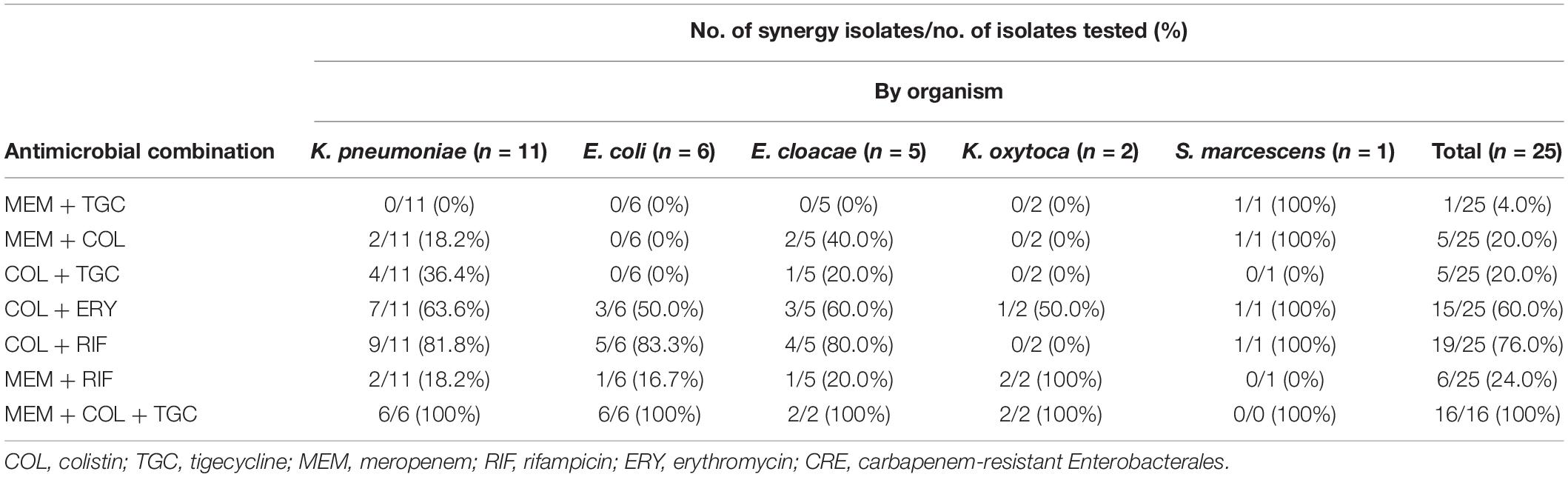
Table 2. Checkerboard results of double and triple antimicrobial combinations for 25 clinical CRE strains.
For CPE isolates, most double antimicrobial combinations were ineffective on the isolates with coexisting blaNDM and blaIMP genes, including colistin-rifampicin, colistin-meropenem, colistin-tigecycline, and meropenem-tigecycline (Table 3). Meropenem-tigecycline had no synergistic effect on CPE with highly resistant to meropenem. In contrast to colistin-rifampicin, meropenem-rifampicin demonstrated the greatest potential synergistic effect on isolates with coexisting blaNDM and blaIMP genes, with a synergistic effect of 75% (3/4). Colistin-meropenem also had a synergistic effect of 14.3% (1/7) against blaKPC-producing isolates and 37.5% (3/8) against blaNDM-producing isolates. Colistin with tigecycline had no synergistic effect on blaNDM–1-producing E. coli and S. marcescens.
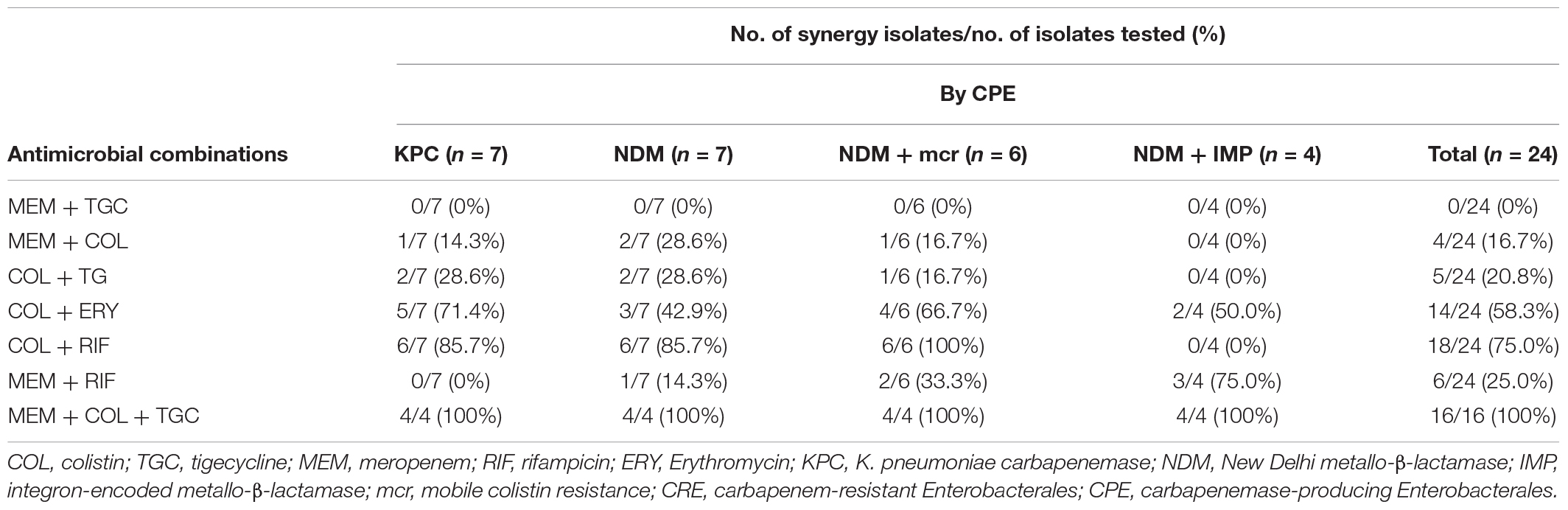
Table 3. Checkerboard results of double and triple antimicrobial combinations for 25 clinical CRE strains by CPE.
Time-Kill Assay of the Antimicrobial Combinations
Time-kill curves of colistin, tigecycline, meropenem, erythromycin, and rifampicin monotherapy or combination therapy against the two K. pneumoniae (SF-18-09, blaKPC–2, C2772, blaNDM–1), one E. coli (C297, blaNDM–1), and one S. marcescens (C261) are shown in Figure 1. The data represent the changes in bacterial density from an initial inoculum. The antimicrobial combinations that demonstrated synergy via the checkerboard assay were evaluated using the time-kill assay. According to the checkerboard synergistic drug concentration, antimicrobial monotherapy showed no bactericidal effect on all isolates within 24 h. Conversely, the majority of antimicrobial combinations therapies resulted in an early synergistic effect (≥ 2 log10 decrease in colony counts) within 4 h. However, the bacteria showed regrowth over 4 h and had the same growth tendency as the control group within 24 h, including those treated with the double (Figures 1A1,B1,C1,D1) and triple antimicrobial combinations (Figure 1D1). Only the colistin-erythromycin combination showed a bactericidal effect on SF-18-09 within 16 h (Figure 1B1).
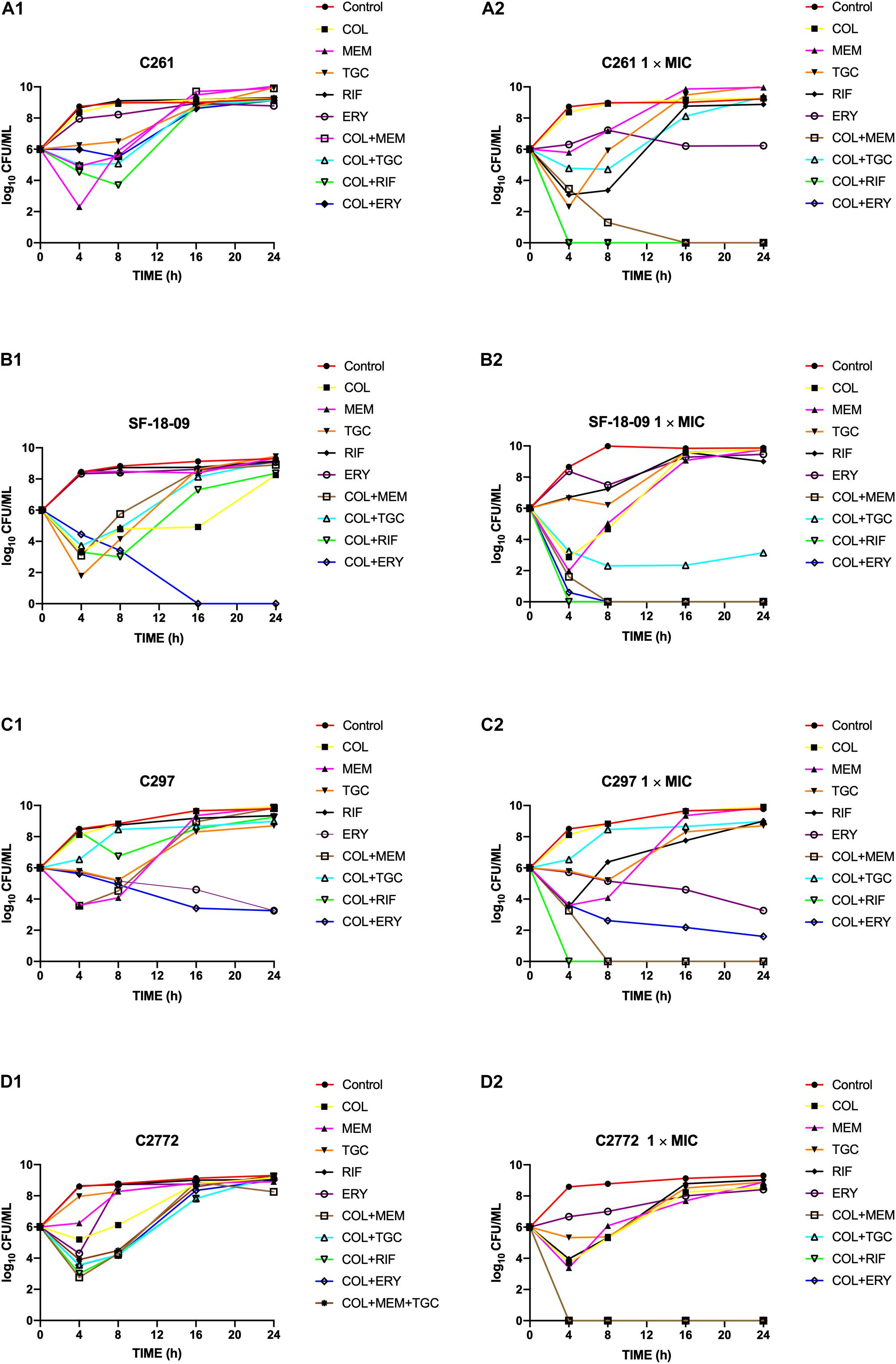
Figure 1. Time-kill curves of colistin alone or in combination with meropenem, tigecycline, rifampicin, and erythromycin against Serratia marcescens C261 (A), Klebsiella pneumoniae SF-18-09 (B), Escherichia coli C297 (C), and K. pneumoniae C2772 (D). According to the checkerboard synergistic drug concentration, monotherapy or combination therapy showed no bactericidal effect on clinical carbapenem-resistant Enterobacterales (CRE) isolates (A1, B1, C1, D1). When using antibiotic concentration 1 × MIC, combination therapy achieved an eradication effect (≥ 3 log10 decrease in colony counts) within 24 h without regrowth (A2, B2, C2, D2).
When using an antimicrobial concentration of 1 × MIC, antimicrobial monotherapy showed no bactericidal effect on all strains within 24 h, whereas the antimicrobial combination therapy achieved an eradication effect (≥ 3 log10 decreases in colony counts) over 24 h without regrowth (Figures 1A2,B2,C2,D2). Colistin-tigecycline only had a bactericidal effect on C2772 (K. pneumoniae, blaNDM–1) isolates (Figure 1D2) and a synergistic effect on SF-18-09 (K. pneumoniae, blaKPC–2) isolates (Figure 1B2), and was ineffective against C297 (E. coli, blaNDM) (Figure 1C2) and C261 (S. marcescens) isolates (Figure 1A2). In addition, 58.8% (10/17) of the time-kill results were consistent with the checkerboard results.
Impact of Combination Therapy on Cellular Morphology
As the colistin-rifampicin combination showed the best synergistic effect on CPE isolates, we examined their potential synergistic mechanism. The colistin-sensitive isolate SF-18-09 (K. pneumoniae, blaKPC) was selected for studying the morphological changes in the bacterial cellular surface using SEM. On treatment with a combination of colistin and rifampicin, the cellular surface showed more micelles and deep craters (Supplementary Figure 2D) than in the control group (Supplementary Figure 2A). The cellular surface appeared to burst, causing excessive leakage of the cellular contents. In contrast, colistin monotherapy (Supplementary Figure 2B) caused only slight asperities and craters on the cellular surface. Rifampicin monotherapy (Supplementary Figure 2C) led to the formation of a biofilm layer around the cells, protecting them from being killed.
Discussion
In this study, we assessed the therapeutic effect of seven antimicrobial combinations (colistin-meropenem, colistin-tigecycline, colistin-rifampicin, colistin-erythromycin, meropenem-tigecycline, meropenem-rifampicin, and colistin-meropenem-tigecycline) against 25 clinical isolates producing different resistance genes (blaKPC, blaNDM, coexisting blaNDM and blaIMP, coexisting mcr-1/8/9 and blaNDM) and preserving highly resistant to meropenem (92% meropenem MIC ≥ 16 μg/mL) using a checkerboard assay, time-kill curves, and SEM.
Antimicrobial combination therapy aims to achieve bactericidal effects at sub-MICs of the concerned isolates and is important for extending life and reducing economic burden. Colistin is a polypeptide antibiotic that causes rapid bacterial killing in a concentration-dependent manner. It acts on the Gram-negative bacterial cell wall, leading to rapid changes in the permeability of the cell membrane and ultimately cell death (Newton, 1956; Schindler and Osborn, 1979). There are major concerns regarding the safety of colistin doses and the prevention of heteroresistant phenotypes (Owen et al., 2007).
Our current study yielded several notable findings. First, the double antimicrobial colistin-rifampicin combinations showed the highest synergistic effect against all the isolates tested, but was ineffective against isolates with coexisting blaNDM and blaIMP. Although colistin combined with rifampicin is generally regarded as safe for multidrug-resistant A. baumannii infections in clinical settings (Bassetti et al., 2008), it is uncertain whether it can be used to treat infections caused by CPE, the in vivo evidence remains insufficient.
Colistin-erythromycin had a suboptimal synergistic effect. The antibacterial spectrum of erythromycin mainly targets Gram-positive cocci, with side effects involving liver toxicity and temporary hearing impairment (Mylonas, 2011). Thus, combining colistin with erythromycin may be a feasible method to alleviate its side effects. Although we identified a significant advantage of combining colistin with erythromycin in vitro, there is incomplete information in the literature regarding its possible therapeutic effect on CPE infections, and there is a lack of prospective clinical trials to confirm this effect. The potential mechanism of the combination of colistin and erythromycin may be that colistin increases the entry of erythromycin into the cell, playing an indirect role in its bactericidal activity (Vaara, 1992; Ofek et al., 1994).
The combination of meropenem with tigecycline had no synergistic effect on CPE with highly resistant to meropenem. This result is consistent with a recently published study, which reported that combination with meropenem is becoming less effective against strains with meropenem MIC > 8 μg/mL (Del Bono et al., 2017).
Triple antimicrobial combinations are being considered as a treatment option against serious CPE infections, and have shown promising results in vitro (Diep et al., 2017). In our study, the triple antimicrobial combinations of meropenem-tigecycline-colistin showed a synergistic effect of 100%. This is the first study demonstrating the effectiveness of triple antimicrobial combinations against coexisting carbapenemase gene isolates, and more clinical trials are required to validate their effectiveness.
We also confirmed the checkerboard results using time-kill assays, which provided dynamic measurements of bactericidal activities over time to explore the in vitro bactericidal effects. Four strains were selected for this analysis. According to the checkerboard synergistic drug concentration, unsatisfactory bactericidal activity was observed against all strains within 24 h when isolates were treated using either monotherapy or combination therapy, and the same growth tendency was observed as in the control group. However, these results are in contrast with those of another study conducted by Soudeiha et al. (2017), showing that antimicrobial combinations at sub-MIC levels can also prevent bacterial regrowth. The discrepancy between these results may be explained by the use of isolates with different carbapenemase-producing and resistant levels. When using antimicrobial concentrations of 1× MIC, the combination of antimicrobials achieved an eradication effect (≥ 3 log10 decreases in colony counts) by 24 h without regrowth compared with monotherapy, which showed regrowth. The combination of colistin with other antimicrobials also show bactericidal effects on S. marcescens strains that are intrinsically non-susceptible to colistin, as several classes of available antibiotics can penetrate the envelope barrier effectively in the presence of colistin (Fajardo et al., 2013). We firstly found that colistin-tigecycline had no synergistic bactericidal effect on blaNDM–1-producing E. coli and S. marcescens.
SEM was used to observe morphological changes in the bacterial cellular surface. Rifampicin monotherapy resulted in the production of a layer of biofilm formation around cells, protecting them from death. Previous reports have demonstrated that the ability of bacteria to form biofilms may contribute to treatment failure as biofilm-forming bacteria are less susceptible to antibiotics (Donlan and Costerton, 2002). Colistin combined with rifampicin caused more micelles and deep craters than monotherapy, which has been shown to be a precursor of cell death according to the carpet model hypothesis (Ciumac et al., 2019). We also observed structural damage via toroidal pore formation, followed by damage to the bacterial membrane and cell death. This phenomenon strongly supports the notion that the synergistic mechanism of colistin with rifampicin may involve changes in the outer cell membrane permeability encoded by colistin, allowing more rifampicin to enter and kill cells. Another possible mechanism is that the combination of colistin with rifampicin reduces the viability of the cell biofilm at low rifampicin concentrations (Geladari et al., 2019).
In conclusions, we found that the double antimicrobial combinations of colistin with rifampicin had the highest synergistic effect on isolates that produced different carbapenemase genes and were highly resistant to meropenem. However, this combination was ineffective on isolates with coexisting blaNDM and blaIMP genes. The triple antimicrobial combinations of meropenem, tigecycline, and colistin had a synergistic effect of 100%. Colistin with tigecycline had no synergistic effect on blaNDM–1-producing E. coli and S. marcescens. The limitations of this study include the lack of in vivo experiments conducted, in addition to its limited sample sizes. Whether these in vitro findings can be applied to a clinical setting needs to be confirmed in further studies, including PK/PD (pharmacokinetics/pharmacodynamics), in vivo experiments, and prospective randomized clinical trials. In general, the antimicrobial combinations evaluated in this study may facilitate the successful treatment of patients infected with Carbapenemase-producing Enterobacterales.
Data Availability Statement
The raw data supporting the conclusions of this article will be made available by the authors, without undue reservation, to any qualified researcher.
Author Contributions
HW conceived and designed the study and revised the draft. CZ, QW, LJ, RW, YY, JZ, and SS performed the experiments described in this study. CZ performed the statistical analysis and wrote the draft. All authors approved the final version of the manuscript.
Funding
This study was financially supported by the Capital’s Funds for Health Improvement and Research (2018-1-4081).
Conflict of Interest
The authors declare that the research was conducted in the absence of any commercial or financial relationships that could be construed as a potential conflict of interest.
Acknowledgments
We thank all the hospitals who provided carbapenemase-producing Enterobacteriaceae isolates (Department of Clinical Laboratory, Peking University People’s Hospital, Beijing; Department of Pulmonary and Critical Care Medicine, Center of Respiratory Medicine, China-Japan Friendship Hospital, and National Clinical Research Center for Respiratory Diseases, Beijing; Department of Infectious Diseases and Clinical Microbiology, Beijing Chao-Yang Hospital, Capital Medical University, Beijing; Department of Infectious Diseases and Clinical Microbiology, Beijing You-yi Hospital, Capital Medical University, Beijing, Department of Clinical Laboratory, Fangshan Liangxiang Hospital, Beijing; and Department of Clinical Laboratory, Chuiyangliu Hospital).
Supplementary Material
The Supplementary Material for this article can be found online at: https://www.frontiersin.org/articles/10.3389/fmicb.2020.533209/full#supplementary-material
Footnotes
- ^ http://www.clsi.org
- ^ http://bigsdb.pasteur.fr/klebsiella/klebsiella.html
- ^ http://mlst.warwick.ac.uk/mlst/dbs/Ecoli
- ^ https://pubmlst.org/ecloacae/
References
Bassetti, M., Peghin, M., and Pecori, D. (2016). The management of multidrug-resistant Enterobacteriaceae. Curr. Opin. Infect. Dis. 29, 583–594. doi: 10.1097/QCO.0000000000000314
Bassetti, M., Repetto, E., Righi, E., Boni, S., Diverio, M., Molinari, M., et al. (2008). Colistin and rifampicin in the treatment of multidrug-resistant Acinetobacter baumannii infections. J. Antimicrob. Chemother. 61, 417–420. doi: 10.1093/jac/dkm509
Berenbaum, M. (1978). A method for testing for synergy with any number of agents. J. Infect. Dis. 137, 122–130. doi: 10.1093/infdis/137.2.122
Ciumac, D., Gong, H., Hu, X., and Lu, J. R. (2019). Membrane targeting cationic antimicrobial peptides. J. Colloid Interface Sci. 537, 163–185. doi: 10.1016/j.jcis.2018.10.103
Del Bono, V., Giacobbe, D. R., Marchese, A., Parisini, A., Fucile, C., Coppo, E., et al. (2017). Meropenem for treating KPC-producing Klebsiella pneumoniae bloodstream infections: should we get to the PK/PD root of the paradox? Virulence 8, 66–73. doi: 10.1080/21505594.2016.1213476
Diep, J. K., Jacobs, D. M., Sharma, R., Covelli, J., Bowers, D. R., Russo, T. A., et al. (2017). Polymyxin B in combination with rifampin and meropenem against polymyxin B-resistant KPC-producing Klebsiella pneumoniae. Antimicrob. Agents Chemother. 61:e02121-16. doi: 10.1128/AAC.02121-16
Doern, C. D. (2014). When does 2 plus 2 equal 5? A review of antimicrobial synergy testing. J. Clin. Microbiol. 52, 4124–4128. doi: 10.1128/jcm.01121-14
Donlan, R. M., and Costerton, J. W. (2002). Biofilms: survival mechanisms of clinically relevant microorganisms. Clin. Microbiol. Rev. 15, 167–193. doi: 10.1128/cmr.15.2.167-193.2002
Fajardo, A., Martínez-Martín, N., Mercadillo, M., Galán, J. C., Ghysels, B., Matthijs, S., et al. (2013). The neglected intrinsic resistome of bacterial pathogens. PLoS One 4:e1619. doi: 10.1371/journal.pone.0001619
Fitzgerald, J. B., Schoeberl, B., Nielsen, U. B., and Sorger, P. K. (2006). Systems biology and combination therapy in the quest for clinical efficacy. Nat. Chem. Biol. 2:458. doi: 10.1038/nchembio817
Geladari, A., Simitsoupoulou, M., and Antachopoulos, C. (2019). Dose-dependent synergistic interactions of colistin with rifampin, meropenem, and tigecycline against carbapenem-resistant Klebsiella pneumoniae biofilms. Antimicrob. Agents Chemother. 63:e02357-18. doi: 10.1128/AAC.02357-18
Guan, X., He, L., and Hu, B. (2016). Laboratory diagnosis, clinical management and infection control of the infections caused by extensively drug-resistant Gram-negative bacilli: a Chinese consensus statement. Clin. Microbiol. Infect. 22, S15–S25. doi: 10.1016/j.cmi.2015.11.004
Khodadadian, R., Rahdar, H. A., Javadi, A., Safari, M., and Khorshidi, A. (2018). Detection of VIM-1 and IMP-1 genes in Klebsiella pneumoniae and relationship with biofilm formation. Microb. Pathog. 115, 25–30. doi: 10.1016/j.micpath.2017.12.036
Lin, Y.-W., Heidi, H. Y., Zhao, J., Han, M.-L., Zhu, Y., Akter, J., et al. (2018). Polymyxin B in combination with enrofloxacin exerts synergistic killing against extensively drug-resistant Pseudomonas aeruginosa. Antimicrob. Agents Chemother. 62:e00028-18. doi: 10.1128/AAC.00028-18
Logan, L. K., and Weinstein, R. A. (2017). The epidemiology of carbapenem-resistant Enterobacteriaceae: the impact and evolution of a global menace. J. Infect. Dis. 215(Suppl._1), S28–S36. doi: 10.1093/infdis/jiw282
Luepke, K. H., Suda, K. J., Boucher, H., Russo, R. L., Bonney, M. W., Hunt, T. D., et al. (2017). Past, present, and future of antibacterial economics: increasing bacterial resistance, limited antibiotic pipeline, and societal implications. Pharmacotherapy 37, 71–84. doi: 10.1002/phar.1868
Merie Queenan, A., and Bush, K. (2007). Carbapenemases: the versatile β-lactamases. Clin. Microbiol. Rev. 20, 440–458. doi: 10.1128/CMR.00001-07
Mylonas, I. (2011). Antibiotic chemotherapy during pregnancy and lactation period: aspects for consideration. Archiv. Gynecol. nd Obstetr. 283, 7–18. doi: 10.1007/s00404-010-1646-3
Nabarro, L., and Veeraraghavan, B. (2015). Combination therapy for carbapenem-resistant Enterobacteriaceae: increasing evidence, unanswered questions, potential solutions. Eur. J. Clin. Microbiol. Infect. Dis. 34, 2307–2311. doi: 10.1007/s10096-015-2486-7
Newton, B. A. (1956). The properties and mode of action of the polymyxins. Bacteriol. Rev. 20, 14–27. doi: 10.1128/mmbr.20.1.14-27.1956
Nordmann, P., Naas, T., and Poirel, L. (2011). Global spread of carbapenemase-producing Enterobacteriaceae. Emerg. Infect. Dis. 17, 1791–1798. doi: 10.3201/eid1710.110655
Odds, F. C. (2003). Synergy, antagonism, and what the chequerboard puts between them. J. Antimicrob. Chemother. 52:1. doi: 10.1093/jac/dkg301
Ofek, I., Cohen, S., Rahmani, R., Kabha, K., Tamarkin, D., Herzig, Y., et al. (1994). Antibacterial synergism of polymyxin B nonapeptide and hydrophobic antibiotics in experimental gram-negative infections in mice. Antimicrob. Agents Chemother. 38, 374–377. doi: 10.1128/aac.38.2.374
Owen, R. J., Li, J., Nation, R. L., and Spelman, D. (2007). In vitro pharmacodynamics of colistin against Acinetobacter baumannii clinical isolates. J. Antimicrob. Chemother. 59, 473–477. doi: 10.1093/jac/dkl512
Quan, J., Li, X., Chen, Y., Jiang, Y., Zhou, Z., Zhang, H., et al. (2017). Prevalence of mcr-1 in Escherichia coli and Klebsiella pneumoniae recovered from bloodstream infections in China: a multicentre longitudinal study. Lancet Infect. Dis. 17, 400–410. doi: 10.1016/S1473-3099(16)30528-X
Schindler, M., and Osborn, M. J. (1979). Interaction of divalent cations and polymyxin B with lipopolysaccharide. Biochemistry 18, 4425–4430. doi: 10.1021/bi00587a024
Shields, R. K., Nguyen, M. H., Hao, B., Kline, E. G., and Clancy, C. J. (2018). Colistin does not potentiate ceftazidime-avibactam killing of carbapenem-resistant Enterobacteriaceae in vitro or suppress emergence of ceftazidime-avibactam resistance. Antimicrob. Agents Chemother. 62:e01018-18. doi: 10.1128/AAC.01018-18
Shrivastava, S. R., Shrivastava, S. P., and Ramasamy, J. (2018). World Health Organization releases global priority list of antibiotic-resistant bacteria to guide research, discovery, and development of new antibiotics. J. Med. Soc. 32:76. doi: 10.4103/jms.jms_25_17
Soudeiha, M. A., Dahdouh, E. A., Azar, E., Sarkis, D. K., and Daoud, Z. (2017). In vitro evaluation of the colistin-carbapenem combination in clinical isolates of A. baumannii using the checkerboard, Etest, and time-kill curve techniques. Front. Cell. Infect. Microbiol. 7:209. doi: 10.3389/fcimb.2017.00209
Trecarichi, E. M., and Tumbarello, M. (2017). Therapeutic options for carbapenem-resistant Enterobacteriaceae infections. Virulence 8, 470–484. doi: 10.1080/21505594.2017.1292196
Vaara, M. (1992). Agents that increase the permeability of the outer membrane. Microbiol. Mol. Biol. Rev. 56, 395–411. doi: 10.1128/mmbr.56.3.395-411.1992
Wang, X., Wang, Q., Cao, B., Sun, S., Zhang, Y., Gu, B., et al. (2019). Retrospective observational study from a Chinese network of the impact of combination therapy versus monotherapy on mortality from carbapenem-resistant Enterobacteriaceae bacteremia. Antimicrob. Agents Chemother. 63:e01511-8. doi: 10.1128/AAC.01511-8
Wang, X., Wang, Y., Zhou, Y., Li, J., Yin, W., Wang, S., et al. (2018). Emergence of a novel mobile colistin resistance gene, mcr-8, in NDM-producing Klebsiella pneumoniae. Emerg. Microb. Infect. 7:122. doi: 10.1038/s41426-018-0124-z
WHO Pathogens Priority List Working Group (2018). Discovery, research, and development of new antibiotics: the WHO priority list of antibiotic-resistant bacteria and tuberculosis. Lancet Infect. Dis. 18, 318–327. doi: 10.1016/S1473-3099(17)30753-3
Xiaojuan, W., Henan, L., Chunjiang, Z., Hongbin, C., Jingbo, L., and Zhanwei, W. (2014). Novel NDM-9 metallo-β-lactamase identified from a Klebsiella pneumoniae strain isolated in China. Int. J. Antimicrob. Agents 44, 90–91. doi: 10.1016/j.ijantimicag.2014.04.010
Yigit, H., Queenan, A., Anderson, G., Domenech-Sanchez, A., Biddle, J., Steward, C., et al. (2008). Novel carbapenem-hydrolyzing beta-lactamase, KPC-1, from a carbapenem-resistant strain of Klebsiella pneumoniae. Antimicrob. Agents Chemother. 45, 1151–1161. doi: 10.1128/AAC.45.4.1151-1161.2001
Yoon, J., Urban, C., Terzian, C., Mariano, N., and Rahal, J. J. (2004). In vitro double and triple synergistic activities of polymyxin B, imipenem, and rifampin against multidrug-resistant. Acinetobacter baumannii. Antimicrob. Agents Chemother. 48, 753–757. doi: 10.1128/aac.48.3.753-757.2004
Yuan, Y., Li, Y., Wang, G., Li, C., Xiang, L., She, J., et al. (2019). Coproduction of MCR-9 and NDM-1 by colistin-resistant Enterobacter hormaechei isolated from bloodstream infection. Infect. Drug Resist. 12, 2979–2985. doi: 10.2147/idr.S217168
Keywords: Carbapenemase-producing Enterobacterales, in vitro synergistic activity, triple antimicrobial combinations, double antimicrobial combinations, highly resistant isolates
Citation: Zhou C, Wang Q, Jin L, Wang R, Yin Y, Sun S, Zhang J and Wang H (2020) In vitro Synergistic Activity of Antimicrobial Combinations Against blaKPC and blaNDM-Producing Enterobacterales With blaIMP or mcr Genes. Front. Microbiol. 11:533209. doi: 10.3389/fmicb.2020.533209
Received: 07 February 2020; Accepted: 20 August 2020;
Published: 21 October 2020.
Edited by:
Jose Ruben Morones-Ramirez, Autonomous University of Nuevo León, MexicoReviewed by:
Jozsef Soki, University of Szeged, HungaryFangyou Yu, Tongji University, China
Shaolin Wang, China Agricultural University, China
Copyright © 2020 Zhou, Wang, Jin, Wang, Yin, Sun, Zhang and Wang. This is an open-access article distributed under the terms of the Creative Commons Attribution License (CC BY). The use, distribution or reproduction in other forums is permitted, provided the original author(s) and the copyright owner(s) are credited and that the original publication in this journal is cited, in accordance with accepted academic practice. No use, distribution or reproduction is permitted which does not comply with these terms.
*Correspondence: Hui Wang, d2h1aWJqQDE2My5jb20=; d2FuZ2h1aUBwa3VwaC5lZHUuY24=