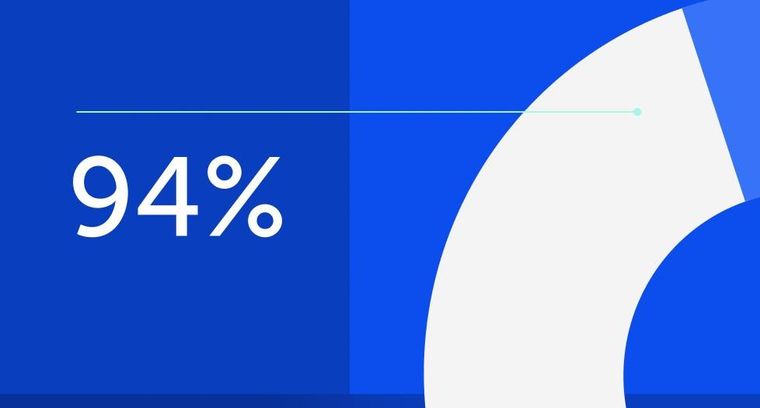
94% of researchers rate our articles as excellent or good
Learn more about the work of our research integrity team to safeguard the quality of each article we publish.
Find out more
ORIGINAL RESEARCH article
Front. Microbiol., 19 November 2020
Sec. Food Microbiology
Volume 11 - 2020 | https://doi.org/10.3389/fmicb.2020.530906
This article is part of the Research TopicDevelopments in Campylobacter, Helicobacter & Related Organisms Research – CHRO 2019View all 18 articles
Campylobacter is the leading cause of the human bacterial foodborne infections in the developed countries. The perception cues from biotic or abiotic environments by the bacteria are often related to bacterial surface and membrane proteins that mediate the cellular response for the adaptation of Campylobacter jejuni to the environment. These proteins function rarely as a unique entity, they are often organized in functional complexes. In C. jejuni, these complexes are not fully identified and some of them remain unknown. To identify putative functional multi-subunit entities at the membrane subproteome level of C. jejuni, a holistic non a priori method was addressed using two-dimensional blue native/Sodium dodecyl sulfate (SDS) polyacrylamide gel electrophoresis (PAGE) in strain C. jejuni 81–176. Couples of acrylamide gradient/migration-time, membrane detergent concentration and hand-made strips were optimized to obtain reproducible extraction and separation of intact membrane protein complexes (MPCs). The MPCs were subsequently denatured using SDS-PAGE and each spot from each MPCs was identified by mass spectrometry. Altogether, 21 MPCs could be detected including multi homo-oligomeric and multi hetero-oligomeric complexes distributed in both inner and outer membranes. The function, the conservation and the regulation of the MPCs across C. jejuni strains were inspected by functional and genomic comparison analyses. In this study, relatedness between subunits of two efflux pumps, CmeABC and MacABputC was observed. In addition, a consensus sequence CosR-binding box in promoter regions of MacABputC was present in C. jejuni but not in Campylobacter coli. The MPCs identified in C. jejuni 81–176 membrane are involved in protein folding, molecule trafficking, oxidative phosphorylation, membrane structuration, peptidoglycan biosynthesis, motility and chemotaxis, stress signaling, efflux pumps and virulence.
Campylobacter is a Gram-negative spiral-shaped bacterium. It has emerged as the leading cause of foodborne bacterial gastroenteritis in humans (Epps et al., 2013; Kaakoush et al., 2015; EFSA and ECDC, 2018). The number of campylobacteriosis cases has been increasing in Europe since 2005 and has reached an incidence of 65 per 100,000 people with 246–158 confirmed cases in 2018 (EFSA and ECDC, 2018). Most cases were attributed to Campylobacter jejuni, an invasive microorganism causing gastroenteritis associated with fever and frequent watery bloody diarrhea, abdominal pains and occasionally nausea (Moore et al., 2005; Silva et al., 2011; Epps et al., 2013). It is also associated with post-infection complications including the immune-mediated neurological disease Guillain-Barré Syndrome (Nachamkin, 2002; Alshekhlee et al., 2008), its variant Miller Fisher Syndrome (Ang et al., 2001) or reactive arthritis (Altekruse et al., 1999). Notably, the infectious dose is considered to be lower than the one for other foodborne pathogens as only 500–800 bacteria trigger human infection (Robinson, 1981; Black et al., 1988; Boyanova et al., 2004; Castano-Rodriguez et al., 2015). Campylobacter cost of illness was estimated at 2.4 billion euros per year in Europe (EFSA, 2016). C. jejuni infections are mainly associated with consumption of poultry and cross-contamination from poultry products (Hue et al., 2010; Guyard-Nicodeme et al., 2013; Hald et al., 2016). For the first time, the European Commission regulation has amended the regulation (EC) No 2073/2005 in 2017 on the hygiene of foodstuffs as regards Campylobacter on broiler carcasses stating a limit of 1000 CFU/g applied from January 2018. This microaerophilic, capnophilic and thermophilic microorganism requires fastidious growth conditions and it growth is rapidly hampered by several environmental stress conditions. Optimal growth is obtained using a modified atmosphere limited in dioxygen and enriched in carbon dioxide, a temperature between 37 and 45 C and a pH between 6.5 and 7.5 (Mace et al., 2015). Nonetheless, C. jejuni is able to survive harmful conditions by developing adaptation mechanisms in response to stress conditions throughout the food chain (Atack and Kelly, 2009; Rodrigues et al., 2016). Living as biofilms is also a phenotypical feature that was demonstrated for C. jejuni, indicating multiple surviving ways outside hosts (Turonova et al., 2015).
Proteomic techniques have been applied to Campylobacter to better understand how changes in genetic expression, bacterial state, nutrient limitation, food plant processing and environmental conditions could affect C. jejuni at the protein level (Tresse, 2017). Natural compartmentalization has facilitated subfraction proteome analyses of Campylobacter such as the cytosolic proteome (Kalmokoff et al., 2006; Bieche et al., 2012; Asakura et al., 2016), the membrane proteome (Seal et al., 2007; Cordwell et al., 2008; Scott et al., 2014; Watson et al., 2014), the inner or outer membrane proteome (Sulaeman et al., 2012) and the exoproteome (Kaakoush et al., 2010). In addition, the genomic and computational era have brought exciting and challenging prospects for proteomics like assigning a function to each protein and subsequently its relationship to other proteins in the cell. Functional genomics and protein structural modeling approaches can predict protein-protein interactions (PPIs), which constitutes the theoretical protein interactome of an organism. Predicted interactomes, including potential stable or transient PPIs, are limited to databases content but PPIs already demonstrated to be biologically functional, specific genetic organizations (operons, gene clusters and regulons) or structural features (domains and loops) (Planas-Iglesias et al., 2013; Wetie et al., 2013). Genomic analyses of the main pathogenic species of Campylobacter, revealed a lack of some of the well-described organizations into operons or gene clusters in Gram-negative bacteria (Parkhill et al., 2000). For instance, genes involved in the amino-acid biosynthesis are scattered in distinct loci across the genome of C. jejuni whereas they are organized into operons in other bacteria. In H. pylori, the closest specie relative to Campylobacter, the presence of some genetic elements organized into operons, gene clusters or islands could have contributed to the specialization of this pathogen (Sohn and Lee, 2011; You et al., 2012). In C. jejuni, the virulence variation among strains could not be assigned to any specific genetic organization other than point mutations in the virulence-associated genes or indels in individual loci (Bell et al., 2013). A reduced genetic organization has probably participated to the idiosyncrasy of C. jejuni.
The alternative method to identify PPIs, which does not result necessary from a specific genetic organization, is to detect complexes of proteins using non-hypothesis driven methods. When these complexes are composed of only protein subunits, the global approach is called proteocomplexomic. This is the case of the two-dimensional (2-D) blue native (BN)/SDS-PAGE which aims at highlighting intact protein complexes using mild non-ionic and non-denaturing detergents (Dresler et al., 2011; Lasserre and Menard, 2012; Wohlbrand et al., 2016). This method consists in separating native protein complexes according to their molecular mass during the first dimension and subsequently in separating protein subunits of each complex in SDS-denaturated conditions in an orthogonal second dimension. It has been applied with success to monitor oligomeric state, stoichiometry and protein subunit composition of protein complexes.
This study aimed at exploring protein machineries of C. jejuni at the membrane level. The bacterial membrane as a hydrophobic lipid structure is a suitable site for protein complex organization. Numerous well-characterized proteins embedded in the membrane are organized into functional units involved in various cellular processes. These membrane protein complexes (MPCs) could be also influenced by the membrane structural integrity and their molecular environment (Sachs and Engelman, 2006). In didermata such as C. jejuni, MPCs could be either organized throughout both membranes and the periplasmic space or specifically in the inner or in the outer membrane. The first objective was to apply and to optimize 2-D BN/SDS-PAGE technique on the C. jejuni membrane proteins to obtain reproducible gels. The second goal was to identify MPCs present in C. jejuni during optimal growth. As this analysis was conducted on the membrane compartment, it was called membrane proteocomplexomic analysis.
The virulent C. jejuni strain 81–176 (NC_008787), whose whole genome is available in Genoscope Platform (MicroScope Vallenet et al., 2017), was selected for the experiments. A loopful of frozen 81–176 cells culture, conserved at −80°C in Brain-Heart Infusion (BHI) broth (Biokar, Beauvais, France) containing 20% sterile glycerol, was cultured on fresh Karmali agar plates (Oxoid, Dardilly, France) (Air Liquid, Paris, France) at 42°C for 48 h in microaerobic conditions (MAC) generated using gas replacement jars operated by MACSmics gassing system (BioMérieux, France) with a gas blend composed of 5% O2, 10% CO2, and 85% N2 (Air Liquid, Paris, France) and 4 filled/flushed cycles at −50 kPa as described in Mace et al. (2015). Cultures were obtained by inoculating 500 mL of BHI broth in a 1-L flask and incubating them for 16 h under MAC at 42°C in a rotary shaker.
The cells were harvested by centrifugation for 20 min at 4°C at 6,000 × g. The supernatant was discarded and about 3 g of dry pellet was obtained. The cells were washed twice with lysis buffer containing 50 mM Tris, 750 mM 6-amino-n-caproic acid as a zwitterionic salt, with each wash followed by centrifugation at 6,000 × g for 20 min at 4°C. The cells were then resuspended in 5.5 mL of lysis buffer supplemented with 60 μL phenylmethylsulfonyl fluoride (PMSF) and sonicated at 50 kHz for 6 × 30 s with 5 min intervals on ice (Vibracell 72434, Bioblock Scientific, Illkirch, France) as previously described by Bieche et al. (2012). The proteins present in the supernatant were then collected and centrifuged twice at 10,000 × g for 30 min at 4°C in order to remove the cellular debris. The whole protein lysate was treated with 0.2 mg/ml DNase I for 1 h at 25°C and then ultra centrifuged at 100,000 × g for 1 h at 4°C. The pellet containing membrane complexes was resuspended in 10 mL of lysis buffer with 50 μL PMSF supplemented with the mild detergent Dodecyl-β-D-Maltoside (DDM) (Sigma, France) at concentrations ranging from 1 to 5% (w/v) to maintain the integrity of protein complexes and limiting dissociation or denaturation as previously recommended by Bernarde et al. (2010). After 15 min on ice, each sample solubilized with DDM was directly ultra-centrifuged at 100,000 × g for 1 h at 4°C. The MPC extraction was performed in triplicate from three independent cultures. Aliquots of the supernatant containing the membrane protein complexes were stored at −80°C. The protein concentration of membrane complexes was determined using the Micro BCATM Protein Assay Kit (Perbio Science, Brebieres, France) according to the manufacturer protocol.
The first dimension was performed in a blue native polyacrylamide gel (BN-PAGE) according to Schagger (Schagger and von Jagow, 1991) with the following modifications. The MCP separation using BN-PAGE gels (15 cm × 16 cm × 0.1 cm) was assayed on linear acrylamide gradients: 4–14% (w/v), 4–18% (w/v), 8–18% (w/v) or 10–20% (w/v) using a gradient forming unit and Protean II cell (Bio-Rad, Hercules, CA, United States). Each separating gel was overlaid with a 3% stacking BN-PAGE. Both anode and cathode buffers contained 50 mM Tris and 75 mM Glycine. Only the cathode buffer was supplemented with 0.002% (w/v) Coomassie Blue G250 (Serva Biochemicals, Heidelberg, Germany). The assembly of gels were embedded with anode and cathode buffers and maintained at 4°C for 3 h before loading the protein sample. A volume of 1–5 μL of sample buffer (500 mM 6-amino-n-caproic acid and 5% Serva blue G) was added to DDM-solubilized membrane protein complex samples. Thyroglobulin (669 kDa), ferritin (440 kDa), catalase (232 kDa), lactate dehydrogenase (140 kDa) and BSA (67 kDa) were used as high molecular weight native protein marker mixture (GE Healthcare, Buckinghamshire, United Kingdom). The migration was run at 4°C with 1 W per gel and limited at 150 V and 90 mA during 4 to 48 h according to the assays.
To check the optimal solubilization of the protein complexes using DDM, migration through BN-PAGE was performed as described above with 3% stacking gel. Samples of 10 or 20 μg of protein complexes solubilized in 1, 2 or 5% (w/v) DDM were prepared as described above and loaded for each lane of the BN-PAGE. For protein complex analyses, gels were silver stained and scanned with a GS-800 densitometer (Bio-Rad) operated with the Quantity One® software (Bio-Rad) at the resolution of 42.3 microns as described previously by Sulaeman et al. (2012). For the protein identification, the gels were loaded with 50 μg of protein complexes. Following the 1-D migration, the protein complexes in the BN-PAGE were fixed using the kit Bio SafeTM Coomassie G-250 Stain (Bio-Rad) according to the manufacturer’s instructions.
The second dimension was performed under denaturing conditions using 10% (w/v) acrylamide SDS-PAGE (15 cm × 16 cm × 0.15 cm). An individual lane was cut off from the first dimension BN-PAGE using a glass plate. Gel lane was equilibrated for 5 min in a buffer containing 1% (w/v) SDS and 125 mM Tris. Then, the proteins were reduced for 15 min into equilibrating buffer supplemented with 50 mM dithiothreitol (DTT) (Sigma, France), and subsequently alkylated for 15 min in equilibrating buffer supplemented with 125 mM iodoacetamide (Bio-Rad). An ultimate washing step lasting 5 min was performed in the equilibrating buffer without supplement. After polymerization of the separating SDS-PAGE and equilibration, the gel lane was laid on a plastic support and introduced between the gel glass plates over the separation gel and embedded with low-melting agarose. Migration was carried out for 4 h at 16°C at 300 V maximum and 10 mA/gel for the first 45 min and then at 20 mA/gel. After migration, proteins were silver stained and scanned as described above.
The silver-stained spots separated by SDS-PAGE were excised manually. At first, the spots were discolored, then washed and reduced/alkylated using an automated system (MultiProbe II, Perkin Elmer, France) as following: each spot was washed several times in water, once in 25 mM ammonium carbonate and dehydrated with acetonitrile (ACN). After drying the gel pieces, the reduction was achieved by incubation for 1 h with 10 mM DTT at 55°C. The alkylation was achieved by incubation the samples with 25 mM iodoacetamide for 1 h at room temperature. Finally, the gel spots were washed three times in water for 10 min, again alternating between ammonium carbonate and ACN. The gel pieces were completely dried before trypsin digestion and rehydrated by trypsin addition. The digestion was carried out overnight at 37°C. The gel fragments were subsequently incubated twice for 15 min in a H2O/ACN solution and in ACN to allow extraction of peptides from the gel pieces. The peptide extracts were then pooled, dried and dissolved in 10 μL starting buffer for chromatographic elution, consisting of 3% (v/v) ACN and 0.1% (v/v) formic acid in water.
The peptides were enriched and separated using a lab-on-a-chip technology (Agilent, Massy, France) and fragmented using an on-line XCT mass spectrometer (Agilent). The fragmentation data were interpreted using the Data Analysis program (version 3.4, Bruker Daltonic, Billerica, MA, United States). For the protein identification, the MS/MS peak lists were extracted, converted into mgf-format files and compared to the C. jejuni, strain 81–176 protein database (UniprotKB, CP000538 for the chromosome, CP000549 for plasmid pTet and CP000550 for plasmid pVir) with the MASCOT Daemon search engine (version 2.6.0; Matrix Science, London, United Kingdom). The following search parameters were used: trypsin was used as the cutting enzyme, the mass tolerance for monoisotopic peptide window was set to ±1.0 Da and the MS/MS tolerance window was set to ±0.5 Da. Two missed cleavages were allowed. Carbamidomethylation, oxidized methionine, acetylation and pyroglutamate in Nt and amidation in Ct were chosen as variable modifications. Generally, the peptides with individual ions scores higher than the score indicated for p < 0.05 were selected. The proteins with two or more unique peptides matching the protein sequence were automatically considered as a positive identification. The main raw data are presented in Supplementary Data Sheet S1. Other raw data are available upon request.
The western blots of 2-D BN/SDS PAGE were performed according to Sulaeman et al. (2012). Briefly, prior to transfer, the 2-D SDS gels were cut into two horizontal sections and each section was soaked for 15 min in transfer buffer. Then, the proteins of each gel section were transferred to a nitrocellulose membrane by electrophoresis using Mini Trans Blot (Bio-Rad). The transferred proteins were then probed with a 1/2000 dilution of antibody anti-PorA or antibody anti-CadF. The immunoreactive proteins were detected using a 1/2000 dilution goat-anti-rabbit alkaline phosphatase antibody [Anti-Rabbit IgG, F(avb)2 fragment-Alkaline Phosphatase, Sigma, Saint-Quentin-Fallavier, France], followed by BCIP/NBT staining (Bio-Rad). Gels were scanned using the GS-800 Imaging densitometer (Bio-Rad).
The presence and organization of genes encoded protein subunits of identified complexes in 81–176 and other Campylobacter complete genomes was explored using the Platform MicroScope described in Vallenet et al., 2017. The biological function of these proteins and complexes were inferred using KEGG and Microcyc (Kanehisa et al., 2016). The conformation analyses of the proteins alone or in a complex were performed using UniProt (Zommiti et al., 2016), RCSB (Stephen et al., 2018), RCSB PDB (Young et al., 2018), Swiss-model (Biasini et al., 2014) and OPM (Lomize et al., 2012). The functional links between partners of complex was explored using STRING (Szklarczyk et al., 2017). If the complex protein subunits were not identified in Campylobacter, homologous genes were searched using conventional gene alignment tools (BlatN).
The protein sequences of CmeA, CmeB, CmeC and MacA, MacB, putMacC (for putative MacC) on fourteen complete genomes of C. jejuni strains and six complete genomes of Campylobacter coli strains were recovered (Supplementary Data Sheet S1). MAFFT alignments (reference) and Fast Tree (reference) phylogenetic trees were performed using Geneious R9 and visualized using FigTree V1.4.3 software1. The proteins structures predictions were determined using Philus transmembrane prediction server (reference,)2.
BlastP analyses were performed for each protein sequence of the components of these efflux pumps in C. jejuni 81–176 against domain bacteria in RefSeq (NCBI Reference Sequence Database) protein database. The general parameters applied for similarity validation were Max target sequences at 20000, automatically adjust parameters for short input sequences, expect threshold at 10, word size at 6 and max matches in a query range at 0. Scoring parameters were obtained from the blosum62 matrix, gap costs with existence at 11 and extension at one with a conditional compositional score matrix adjustment.
The sequence logo of CosR-binding box previously defined by Turonova et al. (2017) was used to check the presence of cosR-binding box in the promoter regions of the operons encoding the efflux pumps cmeABC and macABputC among the complete genomes of C. jejuni and C. coli (Supplementary Data Sheet S1). For that, a sequence length of maximum 120 pb in the intergenic regions upstream to cmeA and macA was recovered. Based on these sequences, a new sequence logo of CosR DNA-binding box with sequences was drawn using WebLogo platform3 (Crooks et al., 2004).
The BN-PAGE was applied first to check the solubilization efficiency of the extracted MPCs. In order to approach a useful detergent concentration for protein complex solubilization for BN-PAGE, Dodecyl-β-D-Maltoside (DDM) has turned out to be suitable (Schägger, 2002). The solubilization of the membrane was tested in a concentration of 1, 2, or 5% of DDM. The loading quantities of 10 μg or 20 μg of MPCs on BN-PAGE were found appropriate to determine the reliable concentration of DDM detergent for C. jejuni (Figure 1). In native conditions, MPCs migrate according to their mass and their form in space (steric hindrance). The bands detected in BN-PAGE in the range of 20 kDa to 670 kDa indicate that MCPs were stable after membrane solubilization and during the separation. All tested concentrations of DDM (1, 2, and 5%) seem to be suitable to solubilize MPCs from C. jejuni. However, over the three independent extractions, less reproducibility was obtained with 5% DDM for the lower molecular mass complexes (not shown). Consequently, only 2% DDM were selected to explore MPCs of C. jejuni 81–176. In addition, the better separation of MPCs was obtained by extending the migration time until 40 h (Supplementary Figure S1). Acrylamide gradients of BN-PAGE were also optimized by testing different couples of lower concentrations (ranging from 4 to 10%) and higher concentration (ranging from 14 to 20%). Even though some protein complexes were more distinct on some gradients, the linear gradient which gives more detectable complexes was 4 to 18% of acrylamide. In general, a lack of reproducibility of the 2-D gels and vertical smearing altering the resolution of MPCs subunits on SDS-PAGE were frequently reported. To circumvent these biases, many gels were run in previous studies until obtaining at least three similar replicates. In the present study, obtaining gel repeatability in a consecutive manner was the first goal. This goal was reached by decreasing vertical smearing, adjusting the thickness of the gels, fixing the supports and isolating the homemade strip from glass using a five-step protocol (Supplementary Figure S1). All these optimization steps resulted in performing consecutive reproducible gels. The reproducibility was validated once three consecutive profiles could be aligned with the same number of detected spots. The results of optimization steps during the first and second dimensions are presented in Supplementary Figure S2.
Figure 1. Separation of membrane protein complexes from C. jejuni Strain 81–176 by BN-PAGE. 10 μg (A) and 20 μg (B) of membrane protein complexes were solubilized with DDM at concentrations ranging from 1, 2, and 5% (w/v). Mass marker are indicated in the central lane (MW in kDa).
Using the criteria mentioned by Reisinger and Eichacker (2006), all protein spots that are located vertically below each other with a similar shape on the 2-D were considered as subunits of one MPC. Consequently, the complexes were numbered from the left side to the right side of the 2-D gel and the detected subunits for each complex with a second number starting from the top of the gel (Figure 2). The spots, which were located side by side in a horizontal row, could potentially be an identical subunit in protein complexes of different molecular masses. If we assume that the molecular mass of a protein complex is increased during assembly of its structural subunits, the analysis of the protein pattern allows to determine the stepwise subunit assembly. The lower toward the higher molecular mass should correspond to complexes located from the right side of the gel toward its left side (Reisinger and Eichacker, 2008). When a complex was composed of subunits from the same gene product, they were called multihomooligomeric complexes and when they were composed of different subunits, they were named multi hetero-oligomeric complexes as described before (Bernarde et al., 2010). Some complexes could not be detected due to a relatively low abundance in the membrane, solubilization parameters or separation parameters. In addition, depending on the solubilization and separation parameters more than one protein complex could run at the same molecular mass during BN-PAGE. This was observed more frequently for smaller protein complexes. In this case, the spot identification helps to separate different complexes when the biological function was previously described. The solubilization, the migration parameters, the interaction between subunits and the subunit organization in or associated with the membrane could also result in partial identification of complexes.
Figure 2. 2D-BN/SDS-PAGE separation of membrane protein complexes of C. jejuni 81–176. Acrylamide gradient was 4–18% for BN-PAGE and acrylamide concentration was 10% for SDS-PAGE. Mass markers for BN-PAGE and SDS-PAGE are respectively, indicated at the top left side of the gel. Proteins identified by LC-MS/MS are indicated by blue arrows. Proteins that do not reach identification criteria after LC-MS/MS analysis are indicated with black arrows. First numbers correspond to complexes and second numbers to subunits of these complexes.
The identification of the spots was achieved by nanoLC MS/MS and validated using Mascot score (Table 1 and Supplementary Data Sheet S1). The western blots using polyclonal antibodies anti-PorA and anti-CadF were used to target specific outer membrane proteins (Supplementary Figure S3). Three spots of PorA and one spot of CadF could be identified using Western-blot confirming the identification performed by LC MS/MS. In addition, the LC MS/MS identified two supplementary spots of PorA indicating that it has a probable higher sensitivity than Western-blot according to the protein abundance. As expected, PorA, also named major outer membrane protein (MOMP) is among the predominant proteins. It was previously reported that PorA could account for 45% of the total visible membrane proteins of C. jejuni (Cordwell et al., 2008).
Table 1. Description of membrane protein complexes identified in C. jejuni strain 81–176 using two-dimensional BN/SDS-PAGE.
Overall, 55 spots were submitted to LC-MS/MS analysis (Figure 2 and Table 1). Among them, nine isolated spots and all spots of complex 7 could not be identified, although attempts using different MS technologies were performed. No contamination with exogenous protein, like keratin, was detected. The spectrograms seem to show noise-to-signal trouble shootings. Two proteins identified with a low scoring (Mascot score <30) were discarded from the analysis. The remaining spots could be grouped into 20 complexes according to their location in the gel, identification and biological functions when available. Overall, 39 proteins predicted as membrane proteins or membrane associated proteins were identified indicating the efficiency of the extraction of the protein complexes from C. jejuni 81–176. These complexes were grouped according to biological functions of KEGG classification (Table 1). Four complexes are involved in oxidative phosphorylation, two in the respiration process, seven in molecules trafficking, three in protein biosynthesis and folding, one in motility and three with unknown functions in the membrane. Altogether, 6 multi hetero-oligomeric and fourteen multihomooligomeric complexes were identified (Table 1). Certain identified complexes were already described in C. jejuni such as efflux pump CmeABC (Gibreel et al., 2007), validating this technique to identify MPC in this bacteria. However, novel complexes are presented, such as complexes 2 and 8 comprised of FrdABC and FdhAB, respectively. These complexes were already described in Helicobacter pylori by Bernarde et al. (2010) and Eubacterium acidaminophilum (Graentzdoerffer et al., 2003), respectively.
Among the 20 identified complexes, subunits belonging to two efflux pumps were detected in complex 6 with CmeA, CmeB and CmeC and complex 20 with MacB (Figure 2 and Table 1). The subunits of these two pumps were further investigated in this study. CmeABC is a multridrug efflux system in Campylobacter working as an RND efflux pump (Lin et al., 2002; Akiba et al., 2006; Grinnage-Pulley and Zhang, 2015). It contributes to the resistance acquisition of Campylobacter to various antimicrobials including macrolides and fluoroquinolones (Yan et al., 2006; Gibreel et al., 2007; Jeon and Zhang, 2009). This efflux pump has also an important role in the resistance to bile (Lin et al., 2003). It includes the inner membrane drug transporter CmeB, the periplasmic membrane fusion protein CmeA and the outer membrane channel CmeC. These proteins can be glycosylated at various sites (Scott et al., 2011) which could explain two CmeB proteins identified with a different molecular weight (Figure 2). The bioinformatics analysis confirmed the organization in operon of the two efflux pumps amongst both C. jejuni and C. coli. For the other efflux pump, the subunit MacB, previously identified to be associated with MacA, was detected in the multihomooligomeric complex 20 which belongs to the efflux pump specific to macrolides (Yum et al., 2009; Bogomolnaya et al., 2013). Using Platform MicroScope, STRING, blastp and blastn, both DNA and protein sequences of these efflux pump partners were analyzed across C. jejuni and C. coli. Genes cmeA and macA are homologous and cmeC is homologous to a gene encoding a putative outer membrane protein (CJJ81176_0637) located downstream to macB. This putative outer membrane protein contains the same functional domain TolC as the one described in CmeC suggesting that this putative protein is probably the third partner of MacAB efflux pump. Further experimental assays will be required to validate the biological function of this putative protein for macrolide efflux pump operation. The phylogenetic and functional analyses confirm the similarities between proteins sequences of subunits of these two efflux pumps across Campylobacter strains (Figure 3 and Supplementary Table S1). CmeA and MacA belong to the HlyD superfamily showing a structure composed of the signal peptide and the non-cytoplasmic domain. The phylogenic analysis of the putative outer membrane protein CJJ81176_0637 confirmed it similarly to CmeC which likely is the third subunit of the efflux pump MacAB (Figure 3). Considering this data, this putative outer membrane protein was named putative MacC (putMacC) and the system MacABputC. In contrast to the similarly between sequences and structural functions of CmeC and putMacC in one hand and CmeA and MacA in the other hand, differences observed between CmeB and MacB is probably at the origin of the restriction of MacB to macrolides efflux.
Figure 3. Phylogenetic tree of proteins belonging to efflux pumps CmeABC and MacABputC. Alignment was performed using MAFFT (Geneious R9) with functional domain and protein structure (Philus) in 14 C. jejuni strains and 6 C. coli strains. Bootstraps percentages were added to internal branches for 1000 replicates. Strains used in this study are listed in Supplementary Table S1.
The efflux pump CmeABC was previously shown to be regulated by CmeR and CosR (Lin et al., 2005; Hwang et al., 2012; Grinnage-Pulley et al., 2016) while no regulation was identified for MacABC. Using the CosR binding box sequence (5′-wdnnhdwnwhwwTTwnhhTTd- 3′) previously described by Turonova et al. (2017), in silico analysis revealed the presence of a CosR-like DNA-binding box upstream to macA in C. jejuni 81–176. Screening for the presence of the CosR binding box in the promoter region of macA in the complete genomes of C. jejuni and C. coli, this consensus sequence was found in C. jejuni but not in C. coli. All the binding box DNA sequences of CosR in the cmeA promoter region of both C. jejuni and C. coli strains and the macA promoter region of C. jejuni strains were compared so as to propose a consensus sequence logo refined for CosR-binding box (Figure 4).
Figure 4. Consensus sequence logo of CosR-binding box of the upstream sequences of genes with CosR-binding capacity (cmeA and macA). Consensus sequence logo of CosR-binding box defined by Turonova et al. (2017) (A) alignment of upstream sequences of cmeA and macA by Geneious 9.1.8 (B) Consensus sequence logo of CosR-binding box redefined (C). w-A or T; y-C or T; m-A or C and k-G or T.
Analyses of genes encoding proteins belonging to these two efflux pumps using across Bacteria domain Blastp analysis indicates that they are mainly observed in proteobacteria (Figure 5 and Supplementary Table S2). CmeA, CmeB, and CmeC were mainly found in the delta/epsilon proteobacteria groups while MacABputC also highly more represented in beta and gamma proteobacteria. Proteins of MacABputC were also found in the fusobacteriaceae family belonging to the fusobacterial phylum (Figure 5 and Supplementary Table S2). The presence of this third subunit in outer membrane (putMaC and CmeC) is probably crucial for the functionality of these complexes.
Figure 5. Distribution of CmeABC and MacABputC subunits in bacteria using Blastp with C. jejuni 81–176 protein sequences of each subunit as queries in RefSeq_protein database. The data are based on the hits presented in Supplementary Table S2.
Beyond the protein mapping at the organism or a biological compartment scale using holistic approaches, identifying functional multi-subunit entities at the proteome level is a real challenge. Many cellular processes are carried out by sophisticated multi-subunit protein machineries, i.e., different protein complexes maintained by stable protein interactions. These functional entities could be defined as protein complexes composed of a minimal biologically structure of assembled protein subunits necessary for a specific cellular process (Reisinger and Eichacker, 2007). Membrane proteocomplexome of C. jejuni 81–176 cultivated in optimal growth conditions was explored using 2-D BN/SDS PAGE. The prerequisite goal was to obtain reproducible profiles after optimizing and stabilizing homemade strips. The objective was reached when three consecutive gels with resolved spots could be performed. Twenty-one MPCs were found with this method in C. jejuni 81–176 (Figure 6). We found incomplete complexes suggesting that subunits were probably lost during MPC extraction or unidentified by LC MS/MS.
Figure 6. Membrane protein complexes identified by 2-D BN/SDS-PAGE in C. jejuni 81–176. Color of complexes corresponds to their function in cell: Green for molecules trafficking, Red for protein biosynthesis and folding, Blue for respiration, Yellow for motility, and Orange for the oxidative phosphorylation.
Oxidative phosphorylation is the metabolic pathway in which bacteria use enzymatic complexes to re-oxidize cofactors and produce ATP. It ensures the electron transfer between electron donors and the final electron acceptor which is oxygen for aerobic and microaerobic bacteria such as C. jejuni. The redox reactions are carried out by a series of four protein complexes (I, II, III, and IV) located in the inner membrane. Membrane proteocomplexomic profiling revealed the presence of complexes involved in oxidative phosphorylation. Complex 19 corresponds to the NADH ubiquinone oxidoreductase, the first complex of the oxidative phosphorylation chain. This complex catalyzes the transfer of two electrons from NADH to quinone with the translocation of four protons across the inner membrane: NADH + H+ + Q + 4H+in → NAD+ + QH2 + 4H+out (Baranova et al., 2007; Weerakoon and Olson, 2008; Efremov and Sazanov, 2011; Baradaran et al., 2013). Genomic analysis revealed fourteen genes nuo organized in operon in C. jejuni and C. coli genomes with a highly conserved synteny. NuoFEGDCBI are involved in the hydrophilic domain of the NADH dehydrogenase complex while NuoAHJKLMN is localized into the membrane (Baradaran et al., 2013). The partners of this complex NuoC, NuoD and NuoG detected from our MPC fingerprinting are predicted to be localized on the basal part of the hydrophilic domain, close to the inner membrane. In Escherichia coli, NuoC and NuoD are fused and NuoG is close to them (Baranova et al., 2007; Baradaran et al., 2013). If these subunits are similarly organized in C. jejuni, this would indicate that 2% DMM MPC extraction, detection and subunit identification mainly selected this part of NADH dehydrogenase complex. The complex 2 corresponds to complex II of the oxidative phosphorylation chain. The fumarate reductase complex is generally composed of FrdA, FrdB and FrdC (Weingarten et al., 2009; Guccione et al., 2010; Jardim-Messeder et al., 2017). All three subunits were detected and identified on all the proteocomplexomic finger printings performed in the present study. In C. jejuni, this inner membrane system is bifunctional being able to catalyze both succinate oxidation and fumarate reduction (Guccione et al., 2010; Hofreuter, 2014). The succinate oxidation is favored under microaerobic conditions while the fumarate reduction is operated under oxygen-limited conditions. All three genes are close located on the genome of both C. jejuni and C. coli. FrdA is the fumarate reductase flavoprotein, FrdB is the iron-sulfur subunit and FrdC is the cytochrome B-556 subunit. This fumarate reductase complex FrdABC was also described in H. pylori (Pyndiah et al., 2007). A fourth partner, FrdD is described in E. coli (Rothery et al., 2005). However, the bioinformatic analyses did not reveal any homologous gene to FrdD in C. jejuni and C. coli genomes. This would indicate that complex 2 does exist and might be functional in C. jejuni and H. pylori without FrdD subunit. Any subunit of the ubiquinol-cytochrome c reductase, complex III of the oxidative phosphorylation, was not detected in this study. However, predicted functional partners of this proton pump were detected in C. jejuni genome: petA encoding the iron sulfur subunit, petB encoding the cytochrome b subunit and petC encoding the cytochrome c1 subunit which indicates the absence of this complex in optimal growth conditions or DMM limitations to extract all MPCs. Partners, function and pathways of cytochrome c oxidase (complex IV) in Campylobacter remain elusive. It is encoded by ccoNOPQ. The protein CcoO (Complex 9) was detected in our study. It corresponds to the subunit II of cytochrome c oxidase complex with a high affinity for O2 (Cosseau and Batut, 2004; Hofreuter, 2014). In complex V, the ATP synthase is usually composed of nine subunits, AtpABCDEFFGH often identified as subunit α, A, ε, β, C, B, B’, γ and δ (Rastogi and Girvin, 1999; Altendorf et al., 2000; Cingolani and Duncan, 2011; Okuno et al., 2011). The bioinformatics analyses revealed that genes atpF, atpF’, atpH, atpA, atpG, atpD and atpC are close located in C. jejuni and C. coli genomes while genes atpB and atpE are present in different loci. The proteocomplexomic analysis identified AptD (also called subunit β in complex 11). The membrane-bound ATP synthase is a key energy carrier in bacteria using the energy of an electrochemical ion gradient and the synthesis of ATP from ADP with inorganic phosphate (Rastogi and Girvin, 1999; Altendorf et al., 2000; Cingolani and Duncan, 2011; Okuno et al., 2011).
The complex formate dehydrogenase (complex 8) contributes to the respiration process by producing CO2 from formate oxidation (Hofreuter, 2014). This complex is composed of two FdhA and FdhB, two subunits detected on complexomic fingerprinting. As an asaccharolytic microorganism, carbon supply in Campylobacter is ensured from amino and organic acids and formate is one of the preferred substrate when hosted in poultry. The second detected complex involved the respiration process is hydrogenase complex HydAB complex 12. Two HydB with different weights (around 75 kDa and 130 kDa) were identified in complex 12 indicating the possible presence of a dimeric form of HydB (spot 12.1) in C. jejuni membrane. For these two complexes, single conserved copies of the encoding genes were observed in all analyzed genomes except for C. jejuni 4031 where a second copy of FdhA was found. Two other subunits for each complex (FdhC/FdhD and HydC/HydD) were previously identified in C. jejuni (Andreesen and Makdessi, 2008; Smart et al., 2009; Weerakoon et al., 2009; Pryjma et al., 2012; Shaw et al., 2012; Hofreuter, 2014). These two other partners interact with the main ones only under environmental stress conditions. The genes encoding these environment dependent conditions are present on C. jejuni and C. coli genomes. As MPCs of C. jejuni 81–176 were explored under optimal growth in our study, it is not a surprise not having detected them.
Several proteins are biosynthesized and folded by different complexes localized in membrane. Different membrane protein complexes were extracted and identified in this study.
The chaperonin GroEL (complex 0) is a cylindrical complex with two stacked heptameric rings with ATPase activity that binds non-native substrate protein (SP). GroEL is associated with cofactor GroES and form a nano-cage where SP can be folded up (Klancnik et al., 2006; Chi et al., 2015; Haldar et al., 2015; Motojima and Yoshida, 2015; Hayer-Hartl et al., 2016). As its cofactor GroES has a too small molecular weight (10 kDa), it could not be detected on our profiling fingerprinting. Another complex (complex 4) playing a role in protein folding was detected. DsbB-DsbA is a disulfide bond generation system operating in the oxidative pathway (Inaba et al., 2006; Inaba and Ito, 2008; Ito and Inaba, 2008; Grabowska et al., 2011; Sperling et al., 2013). In this study, only DsbB subunit was identified although two dsbA genes with 51% homology were present in C. jejuni 81–176 genome. DsbB localized in inner-membrane interacts with the periplasmic dithiol oxidase DsbA (Inaba et al., 2006; Inaba and Ito, 2008).
Several membrane protein complexes identified in this study have a function in molecule trafficking through the C. jejuni 81–176 membrane. These complexes play an important role in adaptation and virulence capabilities. For instance, the major Campylobacter porin PorA was extracted and identified in the complexes 3 and 13. This porin corresponding to MOMP, involved in the adaptation of Campylobacter to host environments (De et al., 2000; Zhang et al., 2000; Clark et al., 2007; Ferrara et al., 2016), is a multihomooligomeric porin with three PorA subunits (Zhang et al., 2000; Ferrara et al., 2016). This porin can be identified in three conformational forms including the folded monomer (35 kDa), the denatured monomer (40 to 48 kDa), and the native trimer (120 to 140 kDa) (Huyer et al., 1986; Zhang et al., 2000). In our gel, two complexes corresponding to native trimer MOMP at two different weights were identified. The complex 13 was estimated between 120–140 kDa and after subunit separation proteins corresponding to the folded monomer at 35 kDa and the denatured monomer between 40 and 48 kDa could be detected as previously described by Zhang et al. (2000). In the complex 3, the spot with apparent weight between 350 kDa and 400 kDa could correspond to the fusion of two trimeric MOMPs. The associated forms of MOMP in the complex 3 with likely a folded monomer, denatured monomer and a truncated form at 16 kDa was not previously described.
In this work, units of complexes corresponding to two efflux pumps CmeABC and MacAB were detected (complex 6 and 20). These two efflux pumps were known to play a role in antimicrobials resistance. The multidrug efflux pump CmeABC was more studied compared to the macrolide efflux pump MacABputC in Campylobacter (Lin et al., 2002, 2003; Akiba et al., 2006; Yan et al., 2006; Gibreel et al., 2007; Jeon and Zhang, 2009; Grinnage-Pulley and Zhang, 2015). In our study, the genetic and proteomic similarities between the constitutive proteins of these efflux pumps were highlighted by bioinformatics analyses. We were able to identify a potential third partner of the macrolide efflux pump, putMacC with a functional domain TolC similarly to CmeC. Subunits composing these two efflux pumps were mainly found in the proteobacteria phylum. Furthermore, the presence of CosR-binding box of the upstream sequences of macA was found in C. jejuni strains. However, this CosR-binding box could not be detected upstream macA in C. coli strains. Further biological analyses are required to explore the potential rule of CosR to regulate expression of transcripts of this macrolide efflux pump and to state its presumptive species specificity.
Two complexes (complex 1 and 14) were extracted and their subunits could be identified. The subunits of the complex 1, IlvC a ketol-acid reductoisomerase and Ftn a non-heme iron-containing ferritin were detected on the same horizontal line. IlvC is involved in L-isoleucine and L-valine biosynthesis (Pyndiah et al., 2007; Wu et al., 2016) while Ftn has a role in storing available cytosolic iron and to reduce cellular toxicity under conditions of intermittent or constant iron excess during infection (Wai et al., 1996). There are few information concerning these two proteins in Campylobacter and no interactions were reported. Complexes 10 and 14 are made of two isoforms of the methyl accepting chemotaxis protein CJJ_0180. This protein plays a role in chemotaxis and colonization of the gastrointestinal tract (Gonzalez et al., 1998; Zautner et al., 2012; Li et al., 2014; Chandrashekhar et al., 2015). However, weight of complexes 10 and 14 are different, indicating that these multihomooligomeric complex might be composed of different forms of the subunit as it was described for MOMP complex.
To conclude, this study is the first 2-D BN/SDS-PAGE method applied to identify membrane proteocomplexome of Campylobacter jejuni. Although not all the subunits of functional complexes in the membrane of C. jejuni could be detected, functional genomics analyses assisted us in reconstituting probable functional complexes. For instance, we were able to pinpoint a potential third partner in the macrolide efflux pump and raised hypothesis concerning its regulation by CosR. The 2-D BN/SDS-PAGE raised also limitations for studying bacterial proteocomplexomes. Assignment of spots to independent membrane protein complexes in low molecular weight areas is less easy. In certain cases, protein complexes were probably too weakly expressed as compared to others, or absent in optimal conditions. The tune up of DDM concentration, the conformation of complexes and their location, as full or part of the membrane, might contribute to the extraction of entire and stable complexes. Altogether, this study has allowed to better described the membrane proteocomplexome of C. jejuni providing new focus for further studies of protein complexes previously annotated with unknown functions.
The raw data supporting the conclusions of this article will be made available by the authors, without undue reservation, to any qualified researcher.
OT conceived the work and contributed to finishing the manuscript writing and preparing the figures. SS, LC, and AG performed the experiments. AG prepared the manuscript and the figures. OT, ED, LC, FB-H, and AM revised the manuscript. All authors contributed to the article and approved the submitted version.
AG was financed by INRAE through the program RFI “Food for Tomorrow” in region Pays de la Loire. This study was supported by CompCamp project. This work was presented in part at the 19th International Workshop on Campylobacter, Helicobacter and Related Organisms: CHRO, 2017.
The authors declare that the research was conducted in the absence of any commercial or financial relationships that could be construed as a potential conflict of interest.
The authors are grateful to Pr Mégraud (University of Bordeaux, INSERM, UMR1053 Bordeaux Research in Translational Oncology, BaRITOn, Bordeaux) for helping us to develop complexomic analyses. Thank you to Lucile Bougro for her technical assistance.
The Supplementary Material for this article can be found online at: https://www.frontiersin.org/articles/10.3389/fmicb.2020.530906/full#supplementary-material
Supplementary Figure 1 | Homemade strips in 5 steps to separate the subunits of protein complexes in a reproducing manner. (A) Cut the strip with a clean glass plate following the well where the sample was deposited, equilibrate the gel lane in DTT and iodoacetamide buffers as indicated in materials and methods; (B) lay the gel strip on the wet plastic support using clean forceps; (C) Stick the plastic face of the strip on the glass behind; (D) Slide the strip until touching the precast polyacrylamide gel; (E) Fill the gap and overlaid the strip with low melting agarose using a sterile syringe.
Supplementary Figure 2 | 2D-BN-SDS-PAGE profiling before (A) and after optimization (B) of protein complexes and their subunit separation. The first dimension for complex separation was performed on 4 to 18% acrylamide gradient gels with 2% Dodecyl-β-D-Maltoside (DDM).
Supplementary Figure 3 | 2D-BN/SDS-PAGE western blot, using antibody anti-MOMP (A) and antibody anti-CadF (B). The image in the middle corresponds to the identified proteins complexes before the Western-blot the black arrow indicate the identified proteins with anti-PorA antibody and the white arrow the identify protein with anti-CadF antibody on the 2D-BN/SDS-PAGE.
Supplementary Table 1 | Campylobacter strains with complete genome sequencing used in this study.
Supplementary Table 2 | Number of hits corresponding to the protein sequence of each three subunits of efflux pumps Cme and Mac of C. jejuni 81–176 in RefSeq_protein database. One hit is the result of sequence similarity using comparison tool Blastp with the parameters indicated in materials and methods section. The number of the different occurrences is defined by the threshold of word size.
Akiba, M., Lin, J., Barton, Y. W., and Zhang, Q. J. (2006). Interaction of CmeABC and CmeDEF in conferring antimicrobial resistance and maintaining cell viability in Campylobacter jejuni. J. Antimicrob. Chemother. 57, 52–60. doi: 10.1093/jac/dki419
Alshekhlee, A., Hussain, Z., Sultan, B., and Katirji, B. (2008). Guillain-Barre syndrome - Incidence and mortality rates in US hospitals. Neurology 70, 1608–1613. doi: 10.1212/01.wnl.0000310983.38724.d4
Altekruse, S. F., Stern, N. J., Fields, P. I., and Swerdlow, D. L. (1999). Campylobacter jejuni - An emerging foodborne pathogen. Emerg. Infect. Dis 5, 28–35. doi: 10.3201/eid0501.990104
Altendorf, K., Stalz, W. D., Greie, J. C., and Deckers-Hebestreit, G. (2000). Structure and function of the F-o complex of the ATP synthase from Escherichia coli. J. Exp. Biol. 203, 19–28.
Andreesen, J. R., and Makdessi, K. (2008). “Tungsten, the surprisingly positively acting heavy metal element for prokaryotes,” in Incredible Anaerobes: From Physiology to Genomics to Fuels, eds J. Wiegel, R. J. Maier, and M. W. W. Adams (Oxford: Blackwell Publishing), 215–229. doi: 10.1196/annals.1419.003
Ang, C. W., De Klerk, M. A., Endtz, H. P., Jacobs, B. C., Laman, J. D., Van Der Meche, F. G. A., et al. (2001). Guillain-Barre syndrome- and Miller Fisher syndrome-associated Campylobacter jejuni lipopolysaccharides induce anti-GM(1) and anti-GQ(1b) antibodies in rabbits. Infect. Immun. 69, 2462–2469. doi: 10.1128/iai.69.4.2462-2469.2001
Asakura, H., Kawamoto, K., Murakami, S., Tachibana, M., Kurazono, H., Makino, S., et al. (2016). Ex vivo proteomics of Campylobacter jejuni 81-176 reveal that FabG affects fatty acid composition to alter bacterial growth fitness in the chicken gut. Res. Microbiol. 167, 63–71. doi: 10.1016/j.resmic.2015.10.001
Atack, J. M., and Kelly, D. J. (2009). Oxidative stress in Campylobacter jejuni: responses, resistance and regulation. Future Microbiol. 4, 677–690. doi: 10.2217/fmb.09.44
Baradaran, R., Berrisford, J. M., Minhas, G. S., and Sazanov, L. A. (2013). Crystal structure of the entire respiratory complex I. Nature 494, 443–448. doi: 10.1038/nature11871
Baranova, E. A., Holt, P. J., and Sazanov, L. A. (2007). Projection structure of the membrane domain of Escherichia coli respiratory complex I at 8 angstrom resolution. J. Mol. Biol. 366, 140–154. doi: 10.1016/j.jmb.2006.11.026
Bell, J., Jerome, J., Plovanich-Jones, A., Smith, E., Gettings, J., Kim, H., et al. (2013). Outcome of infection of C57BL/6 IL-10(-/-) mice with Campylobacter jejuni strains is correlated with genome content of open reading frames up- and down-regulated in vivo. Microb. Pathog. 54, 1–19. doi: 10.1016/j.micpath.2012.08.001
Bernarde, C., Lehours, P., Lasserre, J. P., Castroviejo, M., Bonneu, M., Megraud, F., et al. (2010). Complexomics study of two Helicobacter pylori strains of two pathological origins: potential targets for vaccine development and new insight in bacteria metabolism. Mol. Cell. Proteomics 9, 2796–2826. doi: 10.1074/mcp.m110.001065
Biasini, M., Bienert, S., Waterhouse, A., Arnold, K., Studer, G., Schmidt, T., et al. (2014). SWISS-MODEL: modelling protein tertiary and quaternary structure using evolutionary information. Nucleic Acids Res. 42, W252–W258.
Bieche, C., De Lamballerie, M., Chevret, D., Federighi, M., and Tresse, O. (2012). Dynamic proteome changes in Campylobacter jejuni 81-176 after high pressure shock and subsequent recovery. J. Proteom. 75, 1144–1156. doi: 10.1016/j.jprot.2011.10.028
Black, R. E., Levine, M. M., Clements, M. L., Hughes, T. P., and Blaser, M. J. (1988). Experimental Campylobacter jejuni Infection in Humans. J. Infect. Dis. 157, 472–479. doi: 10.1093/infdis/157.3.472
Bogomolnaya, L. M., Andrews, K. D., Talamantes, M., Maple, A., Ragoza, Y., Vazquez-Torres, A., et al. (2013). The ABC-Type Efflux Pump MacAB Protects Salmonella enterica serovar Typhimurium from Oxidative Stress. mBio 4:11.
Boyanova, L., Gergova, G., Spassova, Z., Koumanova, R., Yaneva, P., Mitov, I., et al. (2004). Campylobacter infection in 682 Bulgarian patients with acute enterocolitis, inflammatory bowel disease, and other chronic intestinal diseases. Diagn. Microbiol. Infect. Dis 49, 71–74. doi: 10.1016/j.diagmicrobio.2003.12.004
Castano-Rodriguez, N., Kaakoush, N. O., Lee, W. S., and Mitchell, H. M. (2015). Dual role of Helicobacter and Campylobacter species in IBD: a systematic review and meta-analysis. Gut 66, 235–249.
Chandrashekhar, K., Gangaiah, D., Pina-Mimbela, R., Kassem, I. I., Jeon, B. H., and Rajashekara, G. (2015). Transducer like proteins of Campylobacter jejuni 81-176: role in chemotaxis and colonization of the chicken gastrointestinal tract. Front. Cell. Infect. Microbiol. 5:46. doi: 10.3389/fcimb.2015.00046
Chi, H. X., Wang, X. Q., Li, J. Q., Ren, H., and Huang, F. (2015). Folding of newly translated membrane protein CCR5 is assisted by the chaperonin GroEL-GroES. Sci. Rep. 5:11.
Cingolani, G., and Duncan, T. M. (2011). Structure of the ATP synthase catalytic complex (F-1) from Escherichia coli in an autoinhibited conformation. Nat. Struct. Mol. Biol. 18, 701–U100.
Clark, C. G., Beeston, A., Bryden, L., Wang, G. H., Barton, C., Cuff, W., et al. (2007). Phylogenetic relationships of Campylobacter jejuni based on porA sequences. Can. J. Microbiol. 53, 27–38.
Cordwell, S. J., Alice, C. L. L., Touma, R. G., Scott, N. E., Falconer, L., Jones, D., et al. (2008). Identification of membrane-associated proteins from Campylobacter jejuni strains using complementary proteomics technologies. Proteomics 8, 122–139. doi: 10.1002/pmic.200700561
Cosseau, C., and Batut, J. (2004). Genomics of the ccoNOQP-encoded cbb(3) oxidase complex in bacteria. Arch. Microbiol. 181, 89–96. doi: 10.1007/s00203-003-0641-5
Crooks, G. E., Hon, G., Chandonia, J. M., and Brenner, S. E. (2004). WebLogo: a sequence logo generator. Genome Res. 14, 1188–1190. doi: 10.1101/gr.849004
De, E., Jullien, M., Labesse, G., Pages, J. M., Molle, G., and Bolla, J. M. (2000). MOMP (major outer membrane protein) of Campylobacter jejuni; a versatile pore-forming protein. FEBS Lett. 469, 93–97. doi: 10.1016/s0014-5793(00)01244-8
Dresler, J., Klimentova, J., and Stulik, J. (2011). Bacterial protein complexes investigation using blue native PAGE. Microbiol. Res. 166, 47–62. doi: 10.1016/j.micres.2010.01.005
Efremov, R. G., and Sazanov, L. A. (2011). Structure of the membrane domain of respiratory complex I. Nature 476, 414–U462.
EFSA (2016). Scientific report of EFSA and ECDC - The European Union summary report on trends and sources of zoonoses, zoonotic agents and food-borne outbreaks in 2014. EFSA J. 13:4329.
EFSA, and ECDC (2018). European Food Safety Authority, European Centre for Disease Prevention and Control: The European Union Summary Report on Trends and Sources of Zoonoses, Zoonotic Agents and Food-borne Outbreaks in 2017. EFSA J. 16:5500.
Epps, S. V. R., Harvey, R. B., Hume, M. E., Phillips, T. D., Anderson, R. C., and Nisbet, D. J. (2013). Foodborne campylobacter: infections, metabolism, pathogenesis and reservoirs. Int. J. Environ. Res. Public Health 10, 6292–6304. doi: 10.3390/ijerph10126292
Ferrara, L. G. M., Wallat, G. D., Moynié, L., Dhanasekar, N. N., Aliouane, S., Acosta-Gutiérrez, S., et al. (2016). MOMP from Campylobacter jejuni Is a Trimer of 18-Stranded β-Barrel Monomers with a Ca2 + Ion Bound at the Constriction Zone. J. Mol. Biol. 428, 4528–4543. doi: 10.1016/j.jmb.2016.09.021
Gibreel, A., Wetsch, N. M., and Taylor, D. E. (2007). Contribution of the CmeABC efflux pump to macrolide and tetracycline resistance in Campylobacter jejuni. Antimicrob. Agents Chemother. 51, 3212–3216. doi: 10.1128/aac.01592-06
Gonzalez, I., Richardson, P. T., Collins, M. D., and Park, S. F. (1998). Identification of a gene encoding a methyl-accepting chemotaxis-like protein from Campylobacter coli and its use in a molecular typing scheme for campylobacters. J. Appl. Microbiol. 85, 317–326. doi: 10.1046/j.1365-2672.1998.00510.x
Grabowska, A. D., Wandel, M. P., Lasica, A. M., Nesteruk, M., Roszczenko, P., Wyszynska, A., et al. (2011). Campylobacter jejuni dsb gene expression is regulated by iron in a Fur-dependent manner and by a translational coupling mechanism. BMC Microbiol. 11:16. doi: 10.1186/1471-2180-11-166
Graentzdoerffer, A., Rauh, D., Pich, A., and Andreesen, J. R. (2003). Molecular and biochemical characterization of two tungsten- and selenium-containing formate dehydrogenases from Eubacterium acidaminophilum that are associated with components of an iron-only hydrogenase. Arch. Microbiol. 179, 116–130. doi: 10.1007/s00203-002-0508-1
Grinnage-Pulley, T., Mu, Y., Dai, L., and Zhang, Q. J. (2016). Dual Repression of the Multidrug Efflux Pump CmeABC by CosR and Cmer in Campylobacter jejuni. Front. Microbiol. 7:1097. doi: 10.3389/fmicb.2016.01097
Grinnage-Pulley, T., and Zhang, Q. J. (2015). Genetic basis and functional consequences of differential expression of the CmeABC Efflux Pump in Campylobacter jejuni Isolates. PLoS One 10:e131534. doi: 10.1371/journal.pone.0131534
Guccione, E., Hitchcock, A., Hall, S. J., Mulholland, F., Shearer, N., Van Vliet, A. H. M., et al. (2010). Reduction of fumarate, mesaconate and crotonate by Mfr, a novel oxygen-regulated periplasmic reductase in Campylobacter jejuni. Environ. Microbiol. 12, 576–591. doi: 10.1111/j.1462-2920.2009.02096.x
Guyard-Nicodeme, M., Tresse, O., Houard, E., Jugiau, F., Courtillon, C., El Manaa, K., et al. (2013). Characterization of Campylobacter spp. transferred from naturally contaminated chicken legs to cooked chicken slices via a cutting board. Int. J. Food Microbiol. 164, 7–14. doi: 10.1016/j.ijfoodmicro.2013.03.009
Hald, B., Skov, M. N., Nielsen, E. M., Rahbek, C., Madsen, J. J., Waino, M., et al. (2016). Campylobacter jejuni and Campylobacter coli in wild birds on Danish livestock farms. Acta Vet. Scand. 58:11.
Haldar, S., Gupta, A. J., Yan, X., Miličiæ, G., Hartl, F. U., and Hayer-Hartl, M. (2015). Chaperonin-assisted protein folding: relative population of asymmetric and symmetric GroEL:GroES Complexes. J. Mol. Biol. 427, 2244–2255. doi: 10.1016/j.jmb.2015.04.009
Hayer-Hartl, M., Bracher, A., and Hartl, F. U. (2016). The GroEL-GroES Chaperonin Machine: a Nano-Cage for Protein Folding. Trends Biochem. Sci. 41, 62–76. doi: 10.1016/j.tibs.2015.07.009
Hofreuter, D. (2014). Defining the metabolic requirements for the growth and colonization capacity of Campylobacter jejuni. Front. Cell. Infect. Microbiol. 4:137. doi: 10.3389/fcimb.2014.00137
Hue, O., Le Bouquin, S., Laisney, M.-J., Allain, V., Lalande, F., Petetin, I., et al. (2010). Prevalence of and risk factors for Campylobacter spp. contamination of broiler chicken carcasses at the slaughterhouse. Food Microbiol. 27, 992–999. doi: 10.1016/j.fm.2010.06.004
Huyer, M., Parr, T. R., Hancock, R. E. W., and Page, W. J. (1986). Outer-membrane porin protein of Campylobacter-jejuni. FEMS Microbiol. Lett. 37, 247–250. doi: 10.1111/j.1574-6968.1986.tb01803.x
Hwang, S., Zhang, Q. J., Ryu, S., and Jeon, B. (2012). Transcriptional Regulation of the CmeABC Multidrug Efflux Pump and the KatA Catalase by CosR in Campylobacter jejuni. J. Bacteriol. 194, 6883–6891. doi: 10.1128/jb.01636-12
Inaba, K., and Ito, K. (2008). Structure and mechanisms of the DsbB-DsbA disulfide bond generation machine. Biochim. Biophys. Acta 1783, 520–529. doi: 10.1016/j.bbamcr.2007.11.006
Inaba, K., Murakami, S., Suzuki, M., Nakagawa, A., Yamashita, E., Okada, K., et al. (2006). Crystal structure of the DsbB-DsbA complex reveals a mechanism of disulfide bond generation. Cell 127, 789–801. doi: 10.1016/j.cell.2006.10.034
Ito, K., and Inaba, K. (2008). The disulfide bond formation (Dsb) system. Curr. Opin. Struct. Biol. 18, 450–458. doi: 10.1016/j.sbi.2008.02.002
Jardim-Messeder, D., Cabreira-Cagliari, C., Rauber, R., Turchetto-Zolet, A. C., Margis, R., and Margis-Pinheiro, M. (2017). Fumarate reductase superfamily: a diverse group of enzymes whose evolution is correlated to the establishment of different metabolic pathways. Mitochondrion 34, 56–66. doi: 10.1016/j.mito.2017.01.002
Jeon, B., and Zhang, Q. J. (2009). Sensitization of Campylobacter jejuni to fluoroquinolone and macrolide antibiotics by antisense inhibition of the CmeABC multidrug efflux transporter. J. Antimicrob. Chemother. 63, 946–948. doi: 10.1093/jac/dkp067
Kaakoush, N. O., Castano-Rodriguez, N., Mitchell, H. M., and Man, S. M. (2015). Global Epidemiology of Campylobacter Infection. Clin. Microbiol. Rev. 28, 687–720. doi: 10.1128/cmr.00006-15
Kaakoush, N. O., Man, S. M., Lamb, S., Raftery, M. J., Wilkins, M. R., Kovach, Z., et al. (2010). The secretome of Campylobacter concisus. FEBS J. 277, 1606–1617. doi: 10.1111/j.1742-4658.2010.07587.x
Kalmokoff, M., Lanthier, P., Tremblay, T. L., Foss, M., Lau, P. C., Sanders, G., et al. (2006). Proteomic analysis of Campylobacter jejuni 11168 biofilms reveals a role for the motility complex in biofilm formation. J. Bacteriol. 188, 4312–4320. doi: 10.1128/jb.01975-05
Kanehisa, M., Sato, Y., Kawashima, M., Furumichi, M., and Tanabe, M. (2016). KEGG as a reference resource for gene and protein annotation. Nucleic Acids Res. 44, D457–D462.
Klancnik, A., Botteldoorn, N., Herman, L., and Mozina, S. S. (2006). Survival and stress induced expression of groEL and rpoD of Campylobacter jejuni from different growth phases. Int. J. Food Microbiol. 112, 200–207. doi: 10.1016/j.ijfoodmicro.2006.03.015
Lasserre, J. P., and Menard, A. (2012). Two-dimensional blue native/SDS gel electrophoresis of multiprotein complexes. Methods Mol. Biol. 869, 317–337. doi: 10.1007/978-1-61779-821-4_27
Li, Z. F., Lou, H. Q., Ojcius, D. M., Sun, A. H., Sun, D., Zhao, J. F., et al. (2014). Methyl-accepting chemotaxis proteins 3 and 4 are responsible for Campylobacter jejuni chemotaxis and jejuna colonization in mice in response to sodium deoxycholate. J. Med. Microbiol. 63, 343–354. doi: 10.1099/jmm.0.068023-0
Lin, J., Akiba, M., Sahin, O., and Zhang, Q. J. (2005). CmeR functions as a transcriptional repressor for the multidrug efflux pump CmeABC in Campylobacter jejuni. Antimicrob. Agents Chemother. 49, 1067–1075. doi: 10.1128/aac.49.3.1067-1075.2005
Lin, J., Michel, L. O., and Zhang, Q. J. (2002). CmeABC functions as a multidrug efflux system in Campylobacter jejuni. Antimicrob. Agents Chemother. 46, 2124–2131. doi: 10.1128/aac.46.7.2124-2131.2002
Lin, J., Sahin, O., Michel, L. O., and Zhang, O. J. (2003). Critical role of multidrug efflux pump CmeABC in bile resistance and in vivo colonization of Campylobacter jejuni. Infect. Immun. 71, 4250–4259. doi: 10.1128/iai.71.8.4250-4259.2003
Lomize, M. A., Pogozheva, I. D., Joo, H., Mosberg, H. I., and Lomize, A. L. (2012). OPM database and PPM web server: resources for positioning of proteins in membranes. Nucleic Acids Res. 40, D370–D376.
Mace, S., Haddad, N., Zagorec, M., and Tresse, O. (2015). Influence of measurement and control of microaerobic gaseous atmospheres in methods for Campylobacter growth studies. Food Microbiol. 52, 169–176. doi: 10.1016/j.fm.2015.07.014
Moore, J. E., Corcoran, D., Dooley, J. S. G., Fanning, S., Lucey, B., Matsuda, M., et al. (2005). Campylobacter. Vet. Res. 36, 351–382.
Motojima, F., and Yoshida, M. (2015). Productive folding of a tethered protein in the chaperonin GroEL–GroES cage. Biochem. Biophys. Res. Commun. 466, 72–75. doi: 10.1016/j.bbrc.2015.08.108
Nachamkin, I. (2002). Chronic effects of Campylobacter infection. Microbes Infect. 4, 399–403. doi: 10.1016/s1286-4579(02)01553-8
Okuno, D., Iino, R., and Noji, H. (2011). Rotation and structure of FoF1-ATP synthase. J. Biochem. 149, 655–664. doi: 10.1093/jb/mvr049
Parkhill, J., Wren, B. W., Mungall, K., Ketley, J. M., Churcher, C., Basham, D., et al. (2000). The genome sequence of the food-borne pathogen Campylobacter jejuni reveals hypervariable sequences. Nature 403, 665–668. doi: 10.1038/35001088
Planas-Iglesias, J., Bonet, J., Garcia-Garcia, J., Marin-Lopez, M. A., Feliu, E., and Oliva, B. (2013). Understanding protein-protein interactions using local structural features. J. Mol. Biol. 425, 1210–1224. doi: 10.1016/j.jmb.2013.01.014
Pryjma, M., Apel, D., Huynh, S., Parker, C. T., and Gaynor, E. C. (2012). FdhTU-modulated formate dehydrogenase expression and electron donor availability enhance recovery of Campylobacter jejuni following host cell infection. J. Bacteriol. 194, 3803–3813. doi: 10.1128/jb.06665-11
Pyndiah, S., Lasserre, J. P., Menard, A., Claverol, S., Prouzet-Mauleon, V., Megraud, F., et al. (2007). Two-dimensional blue native/SDS gel electrophoresis of multiprotein complexes from Helicobacter pylori. Mol. Cell. Proteom. 6, 193–206. doi: 10.1074/mcp.m600363-mcp200
Rastogi, V. K., and Girvin, M. E. (1999). Structural changes linked to proton translocation by subunit c of the ATP synthase. Nature 402, 263–268. doi: 10.1038/46224
Reisinger, V., and Eichacker, L. A. (2006). Analysis of membrane protein complexes by blue native PAGE. Proteomics 6, 6–15. doi: 10.1002/pmic.200600553
Reisinger, V., and Eichacker, L. A. (2007). How to analyze protein complexes by 2D blue native SDS-PAGE. Proteomics 7(Suppl. 1), 6–16. doi: 10.1002/pmic.200700205
Reisinger, V., and Eichacker, L. A. (2008). Solubilization of membrane protein complexes for blue native PAGE. J. Proteom. 71, 277–283. doi: 10.1016/j.jprot.2008.05.004
Robinson, D. A. (1981). Infective dose of Campylobacter jejuni in milk. Br. Med. J. 282, 1584–1584. doi: 10.1136/bmj.282.6276.1584
Rodrigues, R. C., Haddad, N., Chevret, D., Cappelier, J. M., and Tresse, O. (2016). Comparison of Proteomics Profiles of Campylobacter jejuni Strain Bf under microaerobic and aerobic conditions. Front. Microbiol. 7:1596. doi: 10.3389/fmicb.2016.01596
Rothery, R. A., Seime, A. M., Spiers, A. M. C., Maklashina, E., Schroder, I., Gunsalus, R. P., et al. (2005). Defining the Q(P)-site of Escherichia coli fumarate reductase by site-directed mutagenesis, fluorescence quench titrations and EPR spectroscopy. FEBS J. 272, 313–326. doi: 10.1111/j.1742-4658.2004.04469.x
Sachs, J. N., and Engelman, D. M. (2006). “Introduction to the membrane protein reviews: the interplay of structure, dynamics, and environment in membrane protein function,” in Annual Review of Biochemistry, ed. R. D. Kornberg (Palo Alto, CA: Annual Reviews), 707–712. doi: 10.1146/annurev.biochem.75.110105.142336
Schägger, H. (2002). Respiratory chain supercomplexes of mitochondria and bacteria. Biochim. Biophys. Acta 1555, 154–159. doi: 10.1016/s0005-2728(02)00271-2
Schagger, H., and von Jagow, G. (1991). Blue native electrophoresis for isolation of membrane protein complexes in enzymatically active form. Anal. Biochem. 199, 223–231. doi: 10.1016/0003-2697(91)90094-a
Scott, N. E., Marzook, N. B., Cain, J. A., Solis, N., Thaysen-Andersen, M., Djordjevic, S. P., et al. (2014). Comparative Proteomics and Glycoproteomics Reveal Increased N-Linked Glycosylation and Relaxed Sequon Specificity in Campylobacter jejuni NCTC11168 O. J. Proteome Res. 13, 5136–5150. doi: 10.1021/pr5005554
Scott, N. E., Parker, B. L., Connolly, A. M., Paulech, J., Edwards, A. V. G., Crossett, B., et al. (2011). Simultaneous Glycan-Peptide Characterization Using Hydrophilic Interaction Chromatography and Parallel Fragmentation by CID, Higher Energy Collisional Dissociation, and Electron Transfer Dissociation MS Applied to the N-Linked Glycoproteome of Campylobacter jejuni. Mol. Cell. Proteom. 10,
Seal, B. S., Hiett, K. L., Kuntz, R. L., Woolsey, R., Schegg, K. M., Ard, M., et al. (2007). Proteomic analyses of a robust versus a poor chicken gastrointestinal colonizing isolate of Campylobacter jejuni. J. Proteome Res. 6, 4582–4591. doi: 10.1021/pr070356a
Shaw, F. L., Mulholland, F., Le Gall, G., Porcelli, I., Hart, D. J., Pearson, B. M., et al. (2012). Selenium-Dependent Biogenesis of Formate Dehydrogenase in Campylobacter jejuni is controlled by the fdhTU accessory genes. J. Bacteriol. 194, 3814–3823. doi: 10.1128/jb.06586-11
Silva, J., Leite, D., Fernandes, M., Mena, C., Gibbs, P. A., and Teixeira, P. (2011). Campylobacter spp. as a foodborne pathogen: a review. Front. Microbiol. 2:200. doi: 10.3389/fmicb.2011.00200
Smart, J. P., Cliff, M. J., and Kelly, D. J. (2009). A role for tungsten in the biology of Campylobacter jejuni: tungstate stimulates formate dehydrogenase activity and is transported via an ultra-high affinity ABC system distinct from the molybdate transporter. Mol. Microbiol. 74, 742–757. doi: 10.1111/j.1365-2958.2009.06902.x
Sohn, S. H., and Lee, Y. C. (2011). The genome-wide expression profile of gastric epithelial cells infected by naturally occurring cagA isogenic strains of Helicobacter pylori. Environ. Toxicol. Pharmacol. 32, 382–389. doi: 10.1016/j.etap.2011.08.006
Sperling, L. J., Tang, M., Berthold, D. A., Nesbitt, A. E., Gennis, R. B., and Rienstra, C. M. (2013). Solid-State NMR Study of a 41 kDa Membrane Protein Complex DsbA/DsbB. J. Phys. Chem. B 117, 6052–6060. doi: 10.1021/jp400795d
Stephen, K. B., Helen, M. B., Cole, C., Jose, M. D., Zukang, F., John, W., et al. (2018). RCSB Protein Data Bank: sustaining a living digital data resource that enables breakthroughs in scientific research and biomedical education. Protein Sci. 27, 316–330. doi: 10.1002/pro.3331
Sulaeman, S., Hernould, M., Schaumann, A., Coquet, L., Bolla, J. M., De, E., et al. (2012). Enhanced adhesion of Campylobacter jejuni to abiotic surfaces is mediated by membrane proteins in oxygen-enriched conditions. PLoS One 7:e46402. doi: 10.1371/journal.pone.0046402
Szklarczyk, D., Morris, J. H., Cook, H., Kuhn, M., Wyder, S., Simonovic, M., et al. (2017). The STRING database in 2017: quality-controlled protein-protein association networks, made broadly accessible. Nucleic Acids Res. 45, D362–D368.
Tresse, O. (2017). Proteomics Analyses Applied to the Human Foodborne Bacterial Pathogen Campylobacter spp.
Turonova, H., Briandet, R., Rodrigues, R., Hernould, M., Hayek, N., Stintzi, A., et al. (2015). Biofilm spatial organization by the emerging pathogen Campylobacter jejuni: comparison between NCTC 11168 and 81-176 strains under microaerobic and oxygen-enriched conditions. Front. Microbiol. 6:709. doi: 10.3389/fmicb.2015.00709
Turonova, H., Haddad, N., Hernould, M., Chevret, D., Pazlarova, J., and Tresse, O. (2017). Profiling of Campylobacter jejuni proteome in exponential and stationary phase of growth. Front. Microbiol. 8:913. doi: 10.3389/fmicb.2017.00913
Vallenet, D., Calteau, A., Cruveiller, S., Gachet, M., Lajus, A., Josso, A., et al. (2017). MicroScope in 2017: an expanding and evolving integrated resource for community expertise of microbial genomes. Nucleic Acids Res. 45, D517–D528.
Wai, S. N., Nakayama, K., Umene, K., Moriya, T., and Amako, K. (1996). Construction of a ferritin-deficient mutant of Campylobacter jejuni: contribution of ferritin to iron storage and protection against oxidative stress. Mol. Microbiol. 20, 1127–1134. doi: 10.1111/j.1365-2958.1996.tb02633.x
Watson, E., Sherry, A., Inglis, N. F., Lainson, A., Jyothi, D., Yaga, R., et al. (2014). Proteomic and genomic analysis reveals novel Campylobacter jejuni outer membrane proteins and potential heterogeneity. EuPA Open Proteom. 4, 184–194. doi: 10.1016/j.euprot.2014.06.003
Weerakoon, D. R., Borden, N. J., Goodson, C. M., Grimes, J., and Olson, J. W. (2009). The role of respiratory donor enzymes in Campylobacter jejuni host colonization and physiology. Microb. Pathog. 47, 8–15. doi: 10.1016/j.micpath.2009.04.009
Weerakoon, D. R., and Olson, J. W. (2008). The Campylobacter jejuni NADH : Ubiquinone oxidoreductase (complex I) utilizes flavodoxin rather than NADH. J. Bacteriol. 190, 915–925. doi: 10.1128/jb.01647-07
Weingarten, R. A., Taveirne, M. E., and Olson, J. W. (2009). The dual-functioning fumarate reductase is the sole succinate: quinone reductase in Campylobacter jejuni and is required for full host colonization. J. Bacteriol. 191, 5293–5300. doi: 10.1128/jb.00166-09
Wetie, A., Sokolowska, I., Woods, A., Roy, U., Loo, J., and Darie, C. (2013). Investigation of stable and transient protein-protein interactions: past, present, and future. Proteomics 13, 538–557. doi: 10.1002/pmic.201200328
Wohlbrand, L., Ruppersberg, H. S., Feenders, C., Blasius, B., Braun, H. P., and Rabus, R. (2016). Analysis of membrane-protein complexes of the marine sulfate reducer Desulfobacula toluolica Tol2 by 1D blue native-PAGE complexome profiling and 2D blue native-/SDS-PAGE. Proteomics 16, 973–988. doi: 10.1002/pmic.201500360
Wu, W. H., Tran-Gyamfi, M. B., Jaryenneh, J. D., and Davis, R. W. (2016). Cofactor engineering of ketol-acid reductoisomerase (IlvC) and alcohol dehydrogenase (YqhD) improves the fusel alcohol yield in algal protein anaerobic fermentation. Algal Res.-Biomass Biofuels Bioproducts 19, 162–167. doi: 10.1016/j.algal.2016.08.013
Yan, M. G., Sahin, O., Lin, J., and Zhang, Q. J. (2006). Role of the CmeABC efflux pump in the emergence of fluoroquinolone-resistant Campylobacter under selection pressure. J. Antimicrob. Chemother. 58, 1154–1159. doi: 10.1093/jac/dkl412
You, Y., He, L., Zhang, M., Fu, J., Gu, Y., Zhang, B., et al. (2012). Comparative genomics of Helicobacter pylori strains of China associated with different clinical outcome. PLoS One 7:e38528. doi: 10.1371/journal.pone.0038528
Young, J. Y., Westbrook, J. D., Feng, Z. K., Peisach, E., Persikova, I., Sala, R., et al. (2018). Worldwide Protein Data Bank biocuration supporting open access to high-quality 3D structural biology data. Database J. Biol. Databases Curation
Yum, S., Xu, Y., Piao, S., Sim, S., Kim, H., Jo, W., et al. (2009). Crystal structure of the periplasmic component of a tripartite macrolide-specific efflux pump. J. Mol. Biol. 387, 1286–1297. doi: 10.1016/j.jmb.2009.02.048
Zautner, A. E., Tareen, A. M., Groß, U., and Lugert, R. (2012). Chemotaxis in Campylobacter jejuni. Eur. J. Microbiol. Immunol. 2, 24–31.
Zhang, Q. J., Meitzler, J. C., Huang, S. X., and Morishita, T. (2000). Sequence polymorphism, predicted secondary structures, and surface-exposed conformational epitopes of Campylobacter major outer membrane protein. Infect. Immun. 68, 5679–5689. doi: 10.1128/iai.68.10.5679-5689.2000
Keywords: foodborne pathogen, proteomics, functional genomics, complexes, membrane proteins, efflux pumps, regulation, blue native electrophoresis
Citation: Guérin A, Sulaeman S, Coquet L, Ménard A, Barloy-Hubler F, Dé E and Tresse O (2020) Membrane Proteocomplexome of Campylobacter jejuni Using 2-D Blue Native/SDS-PAGE Combined to Bioinformatics Analysis. Front. Microbiol. 11:530906. doi: 10.3389/fmicb.2020.530906
Received: 30 January 2020; Accepted: 14 October 2020;
Published: 19 November 2020.
Edited by:
Ozan Gundogdu, University of London, United KingdomReviewed by:
David Smith, Heriot-Watt University, United KingdomCopyright © 2020 Guérin, Sulaeman, Coquet, Ménard, Barloy-Hubler, Dé and Tresse. This is an open-access article distributed under the terms of the Creative Commons Attribution License (CC BY). The use, distribution or reproduction in other forums is permitted, provided the original author(s) and the copyright owner(s) are credited and that the original publication in this journal is cited, in accordance with accepted academic practice. No use, distribution or reproduction is permitted which does not comply with these terms.
*Correspondence: Odile Tresse, b2RpbGUudHJlc3NlQGlucmFlLmZy
Disclaimer: All claims expressed in this article are solely those of the authors and do not necessarily represent those of their affiliated organizations, or those of the publisher, the editors and the reviewers. Any product that may be evaluated in this article or claim that may be made by its manufacturer is not guaranteed or endorsed by the publisher.
Research integrity at Frontiers
Learn more about the work of our research integrity team to safeguard the quality of each article we publish.