- 1Programa de Maestría en Biotecnología, Centro de Investigación en Biotecnología, Universidad Autónoma del Estado de Morelos, Cuernavaca, Mexico
- 2Programa de Genómica Evolutiva, Centro de Ciencias Genómicas, Universidad Nacional Autónoma de México, Cuernavaca, Mexico
- 3Departamento de Microbiología Molecular, Instituto de Biotecnología, Universidad Nacional Autónoma de México, Cuernavaca, Mexico
- 4CONACYT-Centro de Investigación en Biotecnología, Universidad Autónoma del Estado de Morelos, Cuernavaca, Mexico
The genus Campylobacter groups 32 Gram-negative bacteria species, several being zoonotic pathogens and a major cause of human gastroenteritis worldwide. Antibiotic resistant Campylobacter is considered by the World Health Organization as a high priority pathogen for research and development of new antibiotics. Genetic elements related to antibiotic resistance in the classical C. coli and C. jejuni species, which infect humans and livestock, have been analyzed in numerous studies, mainly focused on local geographical areas. However, the presence of these resistance determinants in other Campylobacter species, as well as in C. jejuni and C. coli strains distributed globally, remains poorly studied. In this work, we analyzed the occurrence and distribution of antibiotic resistance factors in 237 Campylobacter closed genomes available in NCBI, obtained from isolates collected worldwide, in different dates, from distinct hosts and comprising 22 Campylobacter species. Our data revealed 18 distinct genetic determinants, genes or point mutations in housekeeping genes, associated with resistance to antibiotics from aminoglycosides, β-lactams, fluoroquinolones, lincosamides, macrolides, phenicols or tetracyclines classes, which are differentially distributed among the Campylobacter species tested, on chromosomes or plasmids. Three resistance determinants, the blaOXA–493 and blaOXA–576 genes, putatively related to β-lactams resistance, as well as the lnu(AN2) gene, putatively related to lincosamides resistance, had not been reported in Campylobacter; thus, they represent novel determinants for antibiotic resistance in Campylobacter spp., which expands the insight on the Campylobacter resistome. Interestingly, we found that some of the genetic determinants associated with antibiotic resistance are Campylobacter species-specific; e.g., the blaOXA–493 gene and the T86V mutation in gyrA were found only in the C. lari group, whereas genes associated with aminoglycosides resistance were found only in C. jejuni and C. coli. Additional analyses revealed how are distributed the resistance and multidrug resistance Campylobacter genotypes assessed, with respect to hosts, geographical locations, and collection dates. Thus, our findings further expand the knowledge on the factors that can determine or favor the antibiotic resistance in Campylobacter species distributed globally, which can be useful to choose a suitable antibiotic treatment to control the zoonotic infections by these bacteria.
Introduction
The discovery and consequent therapeutic use of antibiotics was a remarkable advance in human medicine, which prevented the mortal outcomes of bacterial infections, saving millions of lives during the last century. However, bacteria have evolved through diverse mechanisms, intrinsic and acquired, to withstand the harmful activity of antibiotics. The antibiotic resistance (AR) is mainly generated by the presence of specific resistance genes or point mutations in some housekeeping genes; likewise, the AR can be transferred between bacteria through different mechanisms of DNA exchange, which has greatly increased the occurrence and spread of antibiotic-resistant bacteria worldwide. The development of AR has progressively compromised the effective use of antibiotics, restricting the therapeutic options available to treat the illness produced by antibiotic-resistant pathogenic bacteria. Nowadays, pathogenic bacteria that show resistance to a great diversity of antibiotics represent a serious threat to health worldwide. It has been estimated that infections produced by these bacteria could cause 10 million annual deaths by 2050 (O’Neill, 2014). Faced with this risk to human health, the World Health Organization (WHO) issued a priority global list of antibiotic-resistant pathogenic bacteria for which there is an urgent need to direct research for discovery and development new antibiotics (WHO, 2017). Importantly, in this WHO report, and also in a published analysis from the United States Centers for Disease Control and Prevention (CDC), antibiotic-resistant Campylobacter spp. were cataloged as a serious health hazard in the world (CDC, 2013; WHO, 2017).
The genus Campylobacter groups biologically diverse species. These are Gram-negative, chemoorganotrophic, non-sporeforming epsilonproteobacteria. Depending on the species, these can be slender, spirally curved-, curved- or straight- rods; with a single polar flagellum, bipolar or multiple flagella, or no flagellum; and microaerobic or anaerobic bacteria (Vandamme et al., 2015). At the time of writing this paper, the genus Campylobacter comprised 32 species and 13 subspecies with validly published names1. Twenty Campylobacter species have been isolated from symptomatic or healthy humans: C. coli, C. concisus, C. curvus, C. fetus, C. gracilis, C. helveticus, C. hominis, C. hyointestinalis, C. insulaenigrae, C. jejuni, C. lanienae, C. lari, C. mucosalis, C. peloridis, C. rectus, C. showae, C. sputorum, C. upsaliensis, C. ureolyticus and C. volucris (Man, 2011; Kweon et al., 2015). Some of these Campylobacter species have also been isolated from the gastrointestinal tract of animals, mainly farm animals (poultry, pigs, cattle, and sheep), where Campylobacter spp. reside usually as commensal microorganisms (Silva et al., 2011).
In humans, Campylobacter spp. can cause campylobacteriosis, which is considered the leading food-borne zoonosis and the most common cause of gastroenteritis in the world (EFSA and ECDC, 2018)2. C. jejuni and C. coli are the Campylobacter species more frequently involved in human gastroenteritis, hence, these two species have been by far the most studied (Man, 2011; Kaakoush et al., 2015). However, other species such as C. concisus, C. lari, C. upsaliensis, and C. ureolyticus, have also begun to be recognized as causative agents of human and animal campylobacteriosis; therefore, they are known as emerging Campylobacter species (Man, 2011).
In 2010, the global burden of Campylobacter infections was 95,613,970 clinical cases; 21,374 deaths and 2,141,926 DALYs (Disability Adjusted Life Years) (Havelaar et al., 2015). Campylobacteriosis may cause mild to severe clinical signs, or even be asymptomatic. The common symptoms of Campylobacter enteric infections include diarrhea (often bloody), fever, abdominal cramps, headache, nausea and/or vomiting. In vulnerable populations, such as very young children, elderly or immunologically compromised patients, this illness can be mortal2. Furthermore, other gastrointestinal manifestations or severe life threatening extragastrointestinal complications may appear (Man, 2011; Kaakoush et al., 2015). Because most Campylobacter enteric infections are self-limiting, antibiotic administration is usually not required. Antibiotic therapy is recommended in patients with severe clinical symptoms, relapses, or a prolonged course of infection (Tang et al., 2017). In these cases, fluoroquinolones such as ciprofloxacin, and macrolides such as erythromycin, are the drugs of choice (Ge et al., 2013).
A rapid and constant increase in the frequency of antibiotic-resistant Campylobacter strains isolated from humans and animals has been recognized worldwide (Luangtongkum et al., 2009; Cody et al., 2010; Tang et al., 2017; Signorini et al., 2018). It has been reported a wide-ranging prevalence of Campylobacter strains resistant to the following antibiotic families: aminoglycosides, β-lactams, cephalosporins, fluoroquinolones, fosfomycins, lincosamides, macrolides, phenicols, quinolones, sulfonamides, and tetracyclines (Ishihara et al., 2004; Karikari et al., 2017; Lee et al., 2017; Premarathne et al., 2017; Agunos et al., 2018; Bailey et al., 2018; Ewers et al., 2018; Iglesias-Torrens et al., 2018; Khan et al., 2018; Signorini et al., 2018; Wei and Kang, 2018; Zhang et al., 2018; Nowaczek et al., 2019; Schiaffino et al., 2019). Moreover, a prevalence of up to 94% of multidrug resistant (MDR; resistant to three or more antibiotic families) Campylobacter isolates, in different parts of the world, has been reported (Zhang et al., 2018). A lot of relevant information about the genetic determinants mediating AR in C. coli and C. jejuni has been reported (Taylor and Courvalin, 1988; Payot et al., 2006; Alfredson and Korolik, 2007; Luangtongkum et al., 2009; Smith and Fratamico, 2010; Iovine, 2013; Tang et al., 2017; Shen et al., 2018); however, the genetic determinants for AR in the rest of the Campylobacter species, including the emerging species, are greatly unknown.
By its importance for public health and food safety, it is necessary to know the resistome of the genus Campylobacter; the resistome is defined as the collection of AR determinants in a specific bacteria or ecological niche (D’Costa et al., 2006; Wright, 2007; Hu et al., 2017). A very high correlation between the genotype and phenotype for AR has been observed in Campylobacter (Zhao et al., 2016; de Vries et al., 2018; Whitehouse et al., 2018). Therefore, the identification of the Campylobacter genotypes associated with AR could help to choose the best antibiotic treatment against infections by Campylobacter species.
The aim of this study was to gain insight into the genetic determinants that constitute the Campylobacter resistome.
Materials and Methods
Bacterial Genomes
A total of 237 closed genomes (chromosome and plasmid) of Campylobacter spp. were retrieved from the open-access RefSeq: NCBI Reference Sequence Database3 in March 2019. NCBI accession numbers of the 237 genomes, the information about the host, collection date and geographic location of the strains from which the DNA was extracted, sequenced and annotated, as well as the number of plasmids annotated as an assembly unit, are registered in Supplementary File S1.
In silico Identification of Genes Associated With Antibiotic Resistance
Datasets from publicly available resistance gene databases Comprehensive Antibiotic Resistance Database (CARD4) (Jia et al., 2017) and the National Database of Antibiotic Resistant Organisms (NDARO5), were downloaded (March 2019) and used to identify the presence of genes associated with AR in the 237 Campylobacter genomes, by following two different approaches. In the first one, the BPGA software (Chaudhari et al., 2016) was applied to clustering the resistance genes from CARD and NDARO with those of the Campylobacter genomes, using the USEARCH clustering tool with default parameters (a cutoff set of 50% amino acid identity and 20 random permutations). In the second approach, a BLASTp search was performed6 with all predicted ORFs from the Campylobacter genomes, against the products of resistance genes from CARD and NDARO, using an E value cutoff of 10E-5, a selected threshold of 50% amino acid identity and minimum coverage of 60% of the query sequence length. Both approaches were compared to ensure the identification of the respective gene associated with AR, on the base of the best hit.
In silico Identification of Point Mutations Associated With Antibiotic Resistance
Specific point mutations known to mediate resistance to fluoroquinolones (gyrA), macrolides (rplD, rplV and 23S rRNA) and streptomycin (rpsL), in C. coli or C. jejuni, were sought in the 237 Campylobacter spp. genomes by using publicly available ResFinder database version 3.27 (April 2019) (Zankari et al., 2012). To confirm the point mutations identified by ResFinder, the amino acid sequence of GyrA or RpsL, as well as the nucleotide sequence of 23S rRNA, were aligned and examined using the MEGA X software version 10.1 with the MUSCLE algorithm8.
Results
Identification of Genetic Determinants Associated With AR in Campylobacter
Genetic elements associated with AR, i.e., specific genes and point mutations in housekeeping genes, were sought by in silico analysis in a total of 237 closed publicly available Campylobacter genomes, as described in the “Materials and Methods” section. The 237 genomes assessed spanned 22 species of Campylobacter: C. avium (1), C. coli (22), C. concisus (3), C. cuniculorum (1), C. curvus (1), C. fetus (11), C. gracilis (1), C. helveticus (1), C. hepaticus (1), C. hominis (1), C. hyointestinalis (2), C. iguaniorum (3), C. insulaenigrae (3), C. jejuni (163), C. lanienae (1), C. lari (8), C. peloridis (1), C. pinnipediorum (5), C. sputorum (4), C. subantarcticus (2), C. ureolyticus (1) and C. volucris (1); the number of genomes tested for each species is indicated between parenthesis. Most genomes analyzed were from C. jejuni (68.8%) and C. coli (9.3%); genomes from the 20 remaining Campylobacter species denoted 21.9% of the total. Important to note, in our analysis, we considered a genome as that including the sequence of both the chromosome and plasmids, when present, from the respective strain.
As depicted in Figure 1, 15 acquired genes associated with resistance to 5 distinct antibiotic classes were identified. Those more frequently found were blaOXA–61 and blaOXA–184, showing a prevalence of 32.5% (77/237 genomes) and 27.8% (66/237 genomes), respectively, both coding class D oxacillinase (OXA)-type β-lactamases (Figure 1). Acquired resistance to some β-lactams antibiotics has been associated with β-lactamases production in many organisms, including Campylobacter (Taylor and Courvalin, 1988; Alfredson and Korolik, 2005; Griggs et al., 2009). Notably, our analysis revealed 2 additional putative OXA-type β-lactamase genes, blaOXA–493 (prevalence of 5.49%; 13/237 genomes) and blaOXA–576 (prevalence of 0.42%; 1/237 genomes) (Figure 1), which are annotated in the respective genomes, but had not been previously reported. All these genes for β-lactamases are located on chromosome (Table 1), which is consistent with the fact that plasmids coding β-lactamases have not been described in Campylobacter.
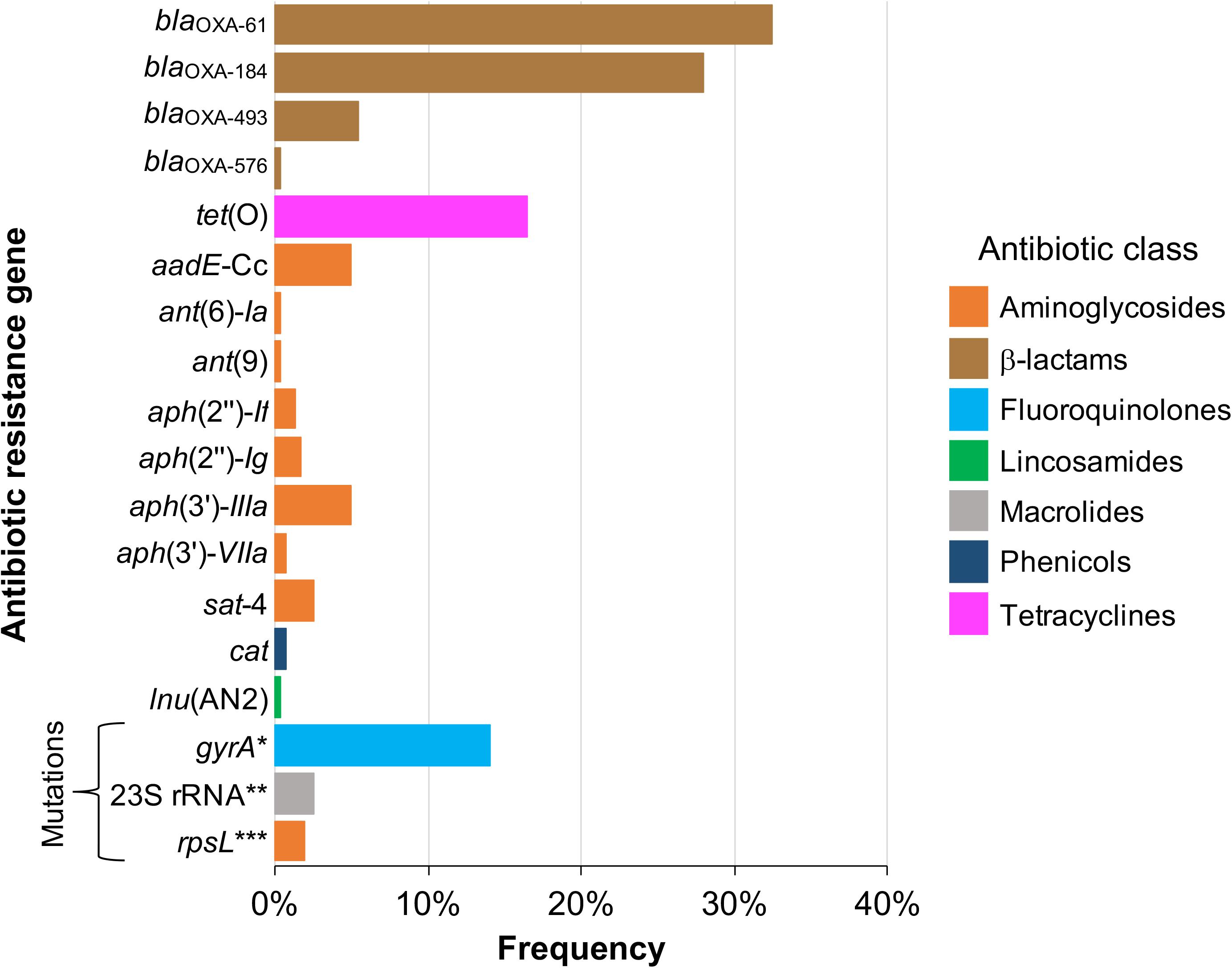
Figure 1. Frequency of genetic determinants associated with antibiotic resistance on Campylobacter genomes. Genetic determinants for antibiotic resistance (resistance genes and mutations in housekeeping genes targets of antibiotics) present in 237 closed publicly available genomes belonging to 22 Campylobacter species, were identified by in silico analysis as described in “Materials and Methods” section. The colors indicate the antibiotic class to which the respective genetic determinant putatively confers resistance. *T86I, T86K, T86V, P104T or T86A/D90Y substitutions; **A2074G or A2074G/A2075G point mutations; ***K43R, K88R or K43R/K88R substitutions.
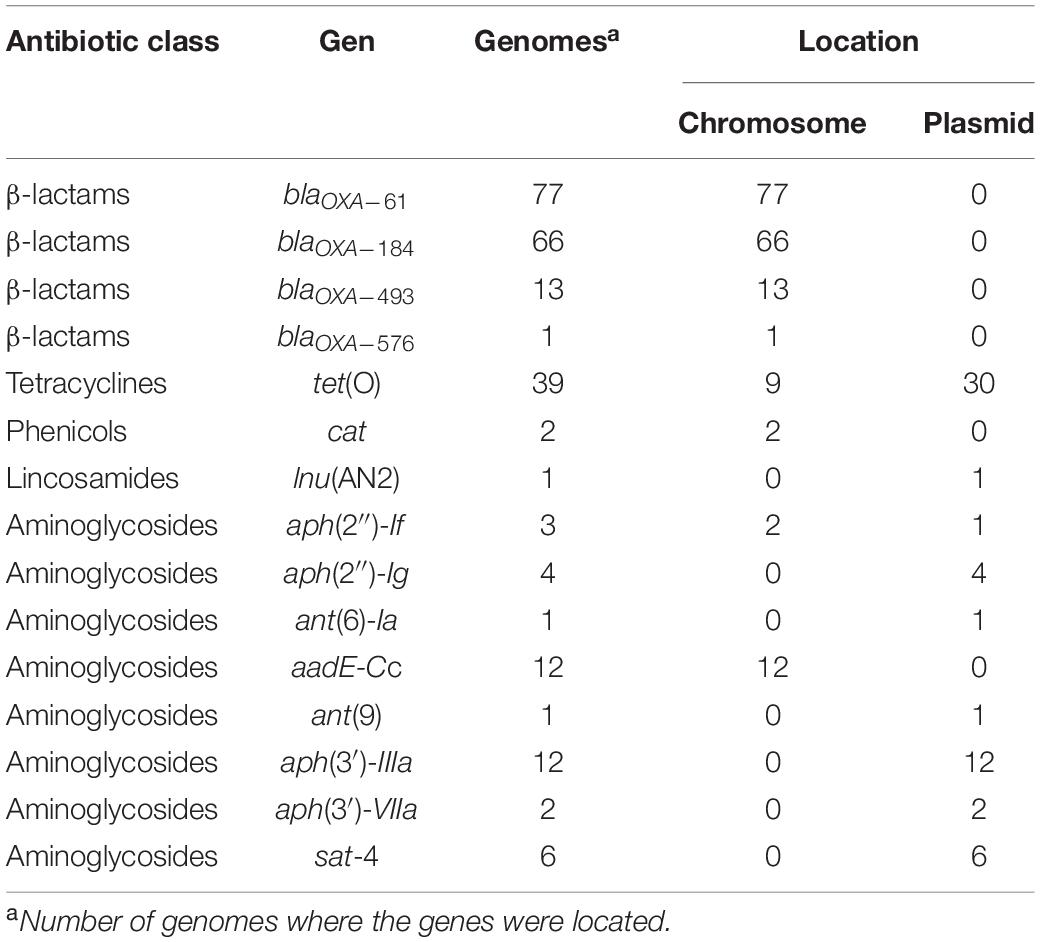
Table 1. Location (chromosome or plasmid) of 15 antibiotic resistance genes detected on Campylobacter closed genomes.
In addition to genes for β-lactamases, we found that 16.5% of analyzed genomes (39/237 genomes) harbor the tet(O) gene (Figure 1), which codes for the ribosomal protection protein involved in resistance to tetracyclines (Sougakoff et al., 1987). Consistent with previous reports indicating the presence of tet(O) either on plasmid or chromosome (Pratt and Korolik, 2005; Dasti et al., 2007), we found that 30 and 9 of the analyzed genomes carry this resistance gene on plasmid and chromosome, respectively (Table 1). It should be noted that Campylobacter plasmids are usually classified according to the genes they carry, those harboring the tet(O) gene have been called pTet plasmids (Marasini et al., 2018). From 87 plasmids annotated as an assembly unit separated from chromosome in the total of Campylobacter genomes tested, 30 were pTet and only one plasmid containing an AR gene other than tet(O) was found; the 31 plasmids harboring AR genes show sizes ranging from 29,115 to 180,543 bp (Figure 2). These pTet plasmids carry the tet(O) gene alone or together with other AR genes; interestingly, all of them, including that no pTet, are present only on genomes from C. coli and C. jejuni (Figure 2).
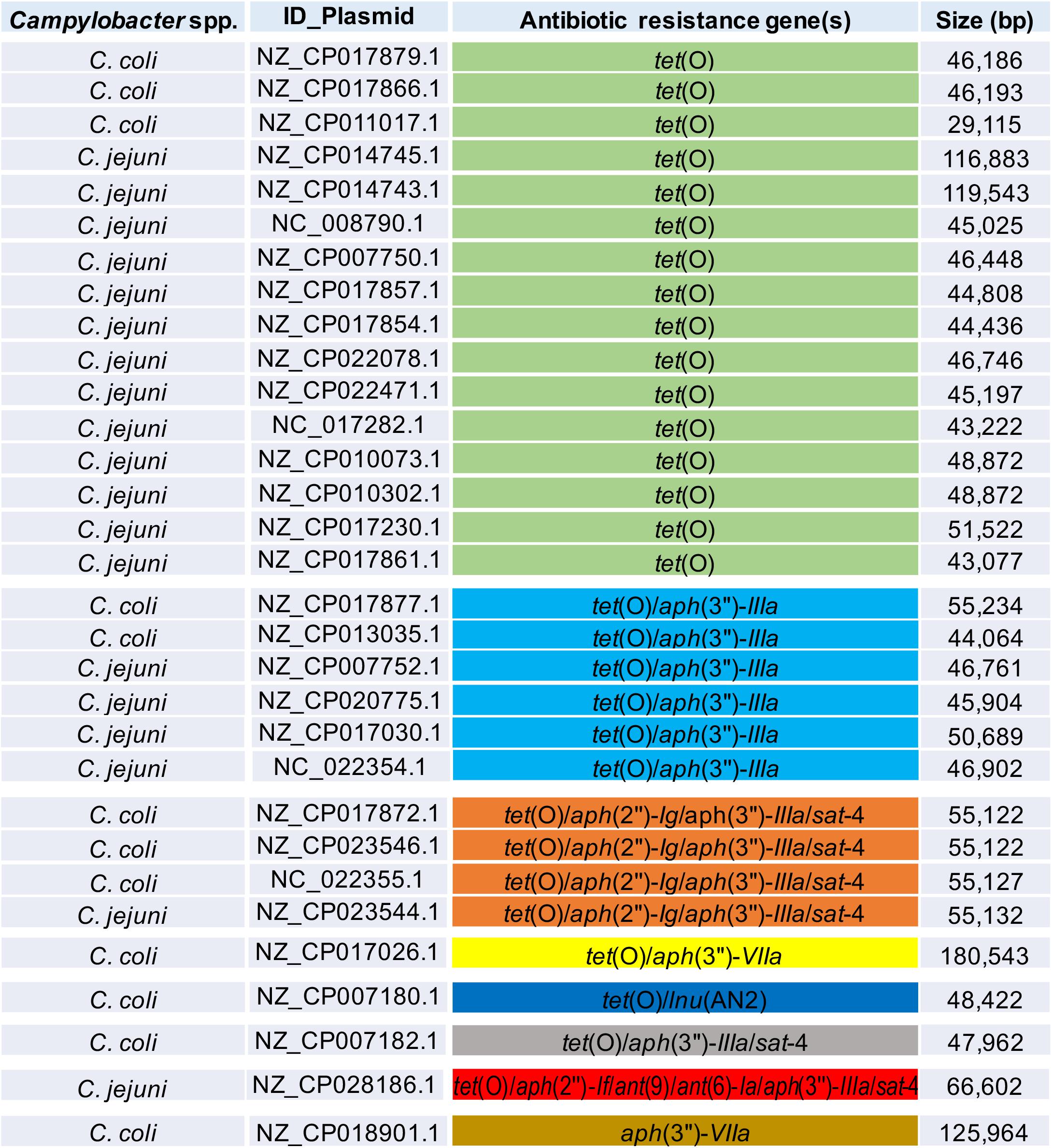
Figure 2. Antibiotic resistance patterns from Campylobacter plasmids. Campylobacter species harboring the respective plasmid, NCBI accession number for each plasmid, genes for antibiotic resistance, and size (bp) for each plasmid, are indicated. Distinct antibiotic resistance patterns are displayed in different color.
On another hand, genes conferring resistance to phenicols or lincosamides were detected in a very small proportion of the analyzed genomes. Only 2 genomes harbor the cat gene (prevalence of 0.84%), which codes for a chloramphenicol acetyltransferase that is associated with resistance to chloramphenicol (Wang and Taylor, 1990); in both cases, this gene was located on chromosome (Figure 1 and Table 1). Regarding lincosamides resistance, a putative lnu(AN2) gene, coding an O-nucleotidyltransferase that mediates resistance to lincomycin (Wang et al., 2000), was only identified in one genome (prevalence of 0.42%) (Figure 1). Previous reports detected the lnu(C) and lnu(D) lincosamides resistance genes in genomes from Campylobacter (Zhao et al., 2016; Fabre et al., 2018), but to the best of our knowledge, this is the first study identifying the lnu(AN2) gene in this genus; specifically in the C. coli strain RM5611. The deduced amino acid sequence from this lnu(AN2) gene shares 64% identity to that from the lnu(AN2) gene of Bacteroides fragilis (NCBI Reference Sequence: NG_047920.1). In B. fragilis, this gene is found on chromosome; in contrast, in C. coli RM5611 the lnu(AN2) gene is located on a plasmid of 48,422 bp, which also contains the tet(O) gene (Figure 3A). Comparative analysis indicated that there is not synteny for the lnu(AN2) gene between C. coli and B. fragilis (data not shown).
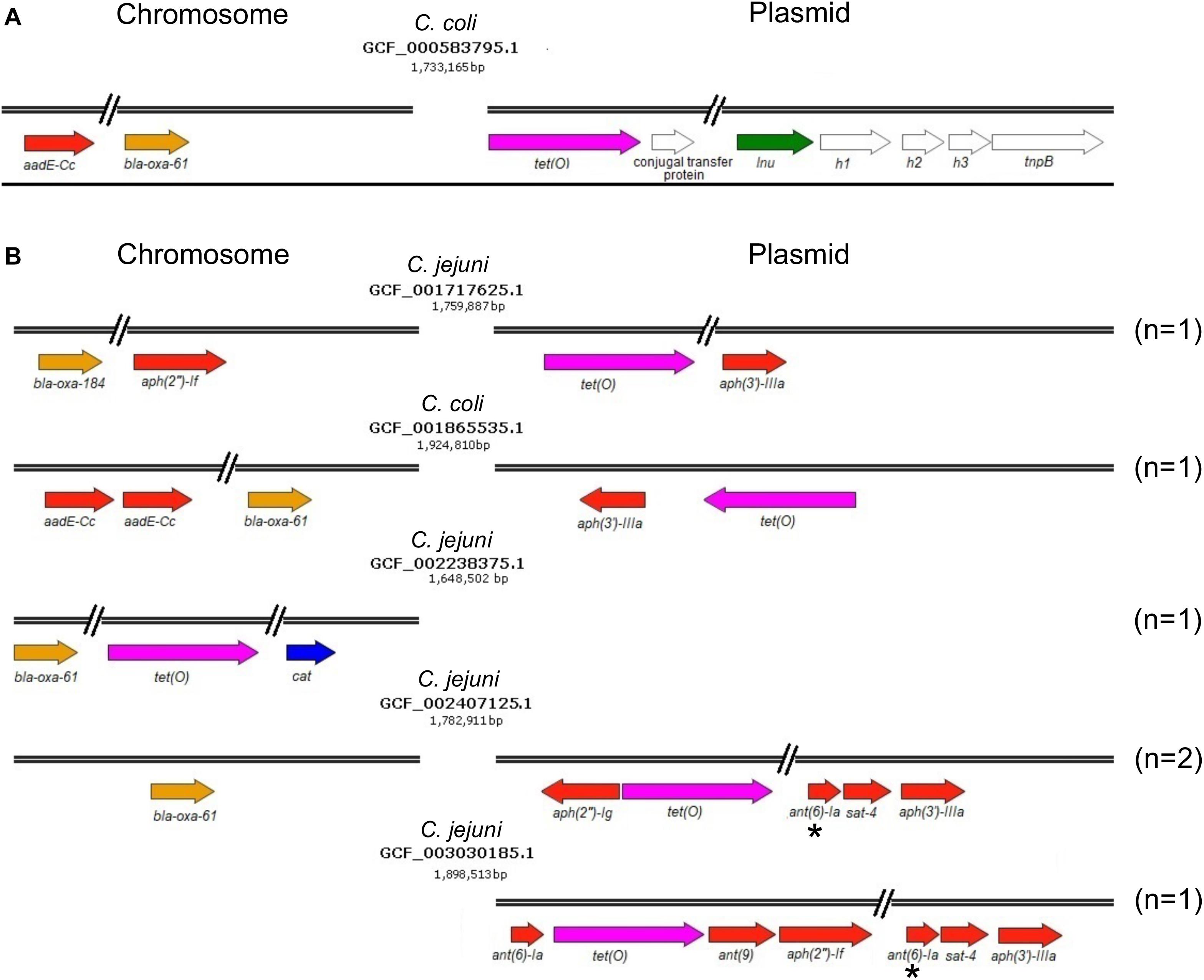
Figure 3. Resistance or multidrug resistance genotypes harbored on genomes of Campylobacter spp. (A) Multidrug resistance genotype containing the lnu(AN2) gene on a 48,422 bp plasmid from the C. coli RM5611 isolate. (B) Resistance or multidrug resistance genotypes containing different combinations of genetic determinants associated with antibiotic resistance on chromosome and/or plasmid. The name of the Campylobacter species from which the respective genome was obtained, as well as the NCBI accession number and size (bp) of genomes are shown. The colors indicate the antibiotic class to which the respective genetic determinant putatively confers resistance. Brown, β-lactams; pink, tetracyclines; blue, phenicols; green, lincosamides; and red, aminoglycosides. The genes shown with white color are not related to antibiotic resistance. The asterisks indicate truncated genes. The number (n) of genomes harboring each antibiotic resistance genotype is specified on the right. Arrows denote the direction of transcription for each gene.
Furthermore, our analysis also identified 8 different genes that code for distinct aminoglycoside-modifying enzymes associated with resistance to diverse aminoglycosides: aph(2″)-If and aph(2″)-Ig (gentamicin); aph(3′)-IIIa and aph(3′)-VIIa (kanamycin); ant(9) (spectinomycin); ant(6)-Ia and aadE-Cc (streptomycin); and sat-4 (streptothricin) (Jacob et al., 1994; Zhao et al., 2015; Fabre et al., 2018). In general, the prevalence of all these aminoglycoside resistance genes was low in the Campylobacter genomes tested, ranging from 0.42% (1/237 genomes) to 5.06% (12/237 genomes), being the aadE-Cc and aph(3′)-IIIa genes those showing the highest prevalence; ant(6)-Ia, ant(9), aph(2″)-If, aph(2″)-Ig, aph(3′)-VIIa and sat-4 were found with a prevalence of 0.42, 0.42, 1.27, 1.69, 0.84, and 2.53%, respectively (Figure 1). It is worth mentioning that 5 additional genomes carried a truncated ant(6)-Ia gene and for this reason they were not considered in the final results; these truncated genes were located on plasmids forming a resistance cluster with the sat-4 and aph(3′)-IIIa genes (Figure 3B). In contrast, the full-length ant(6)-Ia gene was not found close to the sat-4 and aph(3′)-IIIa genes, instead, it was located close to the tet(O) gene (Figure 3B). Regarding the other aminoglycoside resistance genes, most of them were located on plasmids as single units, with exception of aadE-Cc (12/12 genomes) and aph(2″)-If (2/3 genomes) that were situated on chromosome (Table 1).
Additionally, our analysis revealed mutations in 3 housekeeping genes: gyrA, rpsL, and 23S rRNA (Figure 1), which confer resistance to fluoroquinolones (Wang et al., 1993; Charvalos et al., 1996), streptomycin (aminoglycosides) (Olkkola et al., 2010) and macrolides (Jensen and Aarestrup, 2001), respectively. Several point mutations in the gyrA gene have been associated with resistance to fluoroquinolones in Campylobacter spp., the predominant being T86I, caused by the C257T change in gyrA; other mutations include T86A, T86K, T86V, D90N, D90Y, A70T and the double mutation T86I/P104S and T86I/D90N (Wang et al., 1993; Bachoual et al., 2001; Hakanen et al., 2002; Piddock et al., 2003; McIver et al., 2004; Ruiz et al., 2005; Hanninen and Hannula, 2007; Tang et al., 2017). Mutations in gyrA were identified in 13.9% (33/237 genomes) of the Campylobacter genomes analyzed (Figure 1). These mutations are T86I (22/237 genomes), T86K (1/237 genomes), T86V (8/237 genomes), P104T (1/237 genomes) and the combination T86A/D90Y (1/237 genomes). On the other hand, in C. coli and C. jejuni, resistance to macrolides has been attributed to point mutations in domain V of the 23S rRNA gene, at positions 2074 and 2075 (A2074C, A2074G and A2075G), being the A2075G transition the most frequent in Campylobacter (Jensen and Aarestrup, 2001; Vacher et al., 2003; Ren et al., 2011). The bulk of Campylobacter macrolide-resistant isolates have mutations in the 3 copies of the 23S rRNA gene, but some present only 2 mutated copies (Gibreel et al., 2005). Our analysis revealed the presence of point mutations in the 23S rRNA gene in 2.53% (6/237 genomes) of the Campylobacter genomes tested (Figure 1), including A2074G (5/237 genomes) and A2074G/A2075G transitions (1/237 genomes). In 5 genomes, these point mutations were present in the 3 copies of the 23S rRNA gene, whereas in one genome, the A2074G mutation was found only in 2 copies of this gene. Finally, our analysis also revealed mutations in the rpsL gene, which codes for the S12 ribosomal protein, a component of the 30S ribosomal subunit. The K43R, K88E, K88Q and K88R mutations in RpsL have been associated with streptomycin resistance, being the K43R mutation the most commonly found in Campylobacter (Olkkola et al., 2010). We identified some of these mutations in RpsL, in 2.11% (5/237 genomes) of the Campylobacter genomes analyzed: K43R (1/237 genomes), K88R (3/237 genomes) and K43R/K88R (1/237 genomes) (Figure 1).
AR genotypes were identified according to the different combinations of the genetic determinants associated with AR found in the Campylobacter genomes analyzed. A total of 37 AR genotypes were recognized, being the AR genotypes conferring resistance to β-lactams the most predominant found. The different AR genotypes and their prevalence on genomes from C. coli, C. jejuni, and other Campylobacter species, are shown in Supplementary Figures S1–S3. blaOXA–61 and tet(O) were the most common AR genotypes present on genomes from C. coli, with a prevalence of 23.8% (5/21 genomes) and 14.3% (3/21 genomes), respectively; whereas blaOXA–184 (prevalence of 41.1%; 58/141 genomes) and blaOXA–61 (prevalence of 28.4%; 40/141 genomes) were the predominant AR genotypes on genomes from C. jejuni; and blaOXA–493/gyrA T86V and blaOXA–493 were the AR genotypes more common on genomes from other Campylobacter species, with a prevalence of 31.8% (7/22 genomes) and 27.3% (6/22 genomes), respectively (Figure 4). Location (chromosome or plasmid) of the AR determinants for some of the AR genotypes is shown in Figure 3B. All AR genotypes present in the Campylobacter plasmids are displayed in Figure 2.
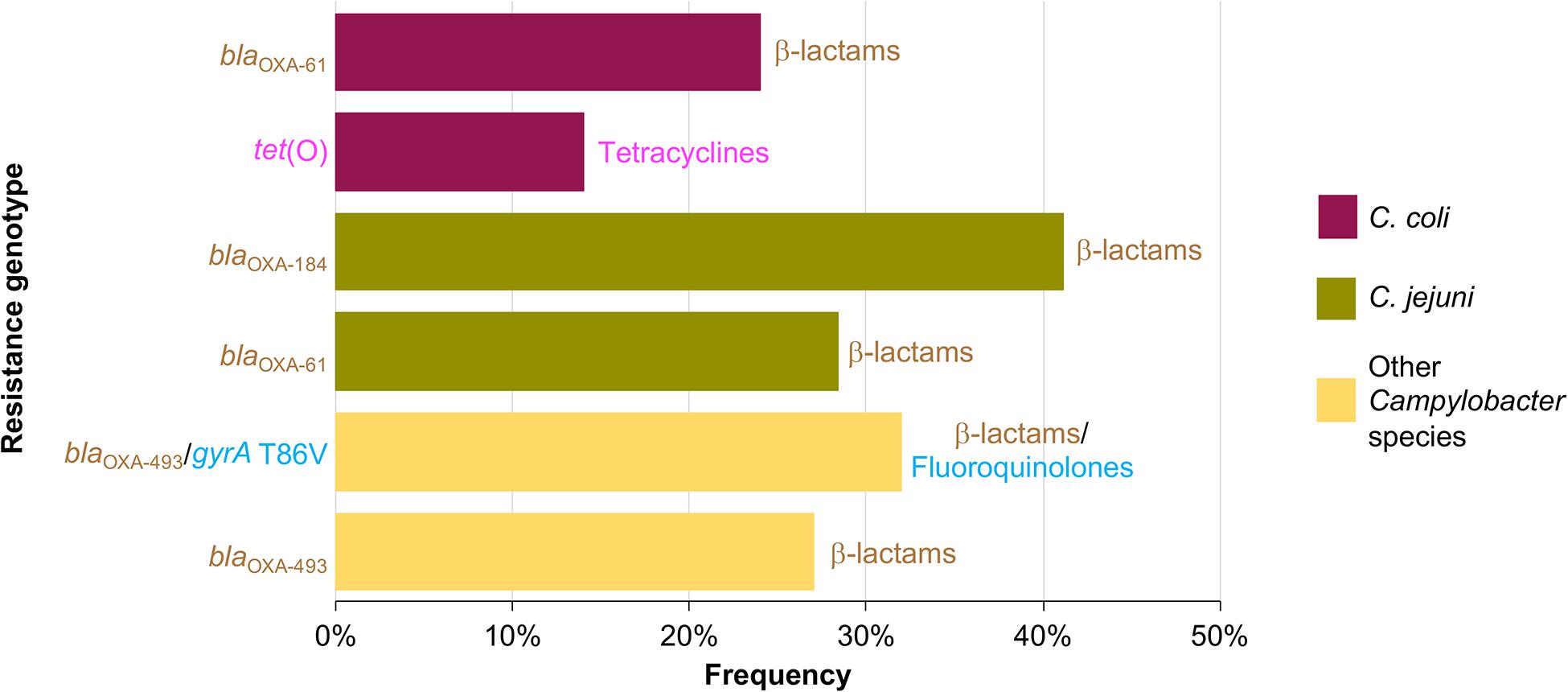
Figure 4. Frequency of the most prevalent antibiotic resistance genotypes on genomes from C. coli, C. jejuni and other Campylobacter species. Other Campylobacter species include: C. avium, C. fetus, C. helveticus, C. hyointestinalis, C. iguaniorum, C. insulaenigrae, C. lanienae, C. lari, C. peloridis, C. sputorum, C. subantarcticus, C. ureolyticus, and C. volucris. For each genotype, the antibiotic class to which they putatively confer resistance is indicated.
Genetic Determinants Associated With AR That Are Campylobacter Species-Specific
Interestingly, our analysis revealed that some genetic determinants for AR are present only in particular Campylobacter species. For instance, despite the great representativeness of genomes from C. jejuni (163 genomes), and with less abundance from C. coli (22 genomes), the blaOXA–493 gene was only found on genomes from 4 of the 6 species that integrate the C. lari group (Miller et al., 2014; Costa and Iraola, 2019): C. insulaenigrae (3/3 genomes), C. lari (7/8 genomes), C. subantarcticus (2/2 genomes) and C. volucris (1/1 genomes) (Supplementary File S1); the number of genomes carrying the blaOXA–493 gene with respect to the total of genomes tested for each species, is indicated between parenthesis. Hence, blaOXA–493 was the predominant gene for β-lactamases that was identified in the C. lari group (prevalence of 92.9%, 13/14 genomes); only one genome from this group presents the blaOXA–184 gene and none carries the blaOXA–61 or blaOXA–576 genes. In contrast, the blaOXA–61 gene was confined to C. jejuni (63/163 genomes) and C. coli (14/22 genomes) species, as well as the blaOXA–184 gene (66 genomes) was mostly present in C. jejuni (65/163 genomes); only one genome of C. helveticus also carries the blaOXA–184 gene (1/1 genomes) (Supplementary File S1). Furthermore, aminoglycoside resistance genes were only detected in C. coli (12/22 genomes) and C. jejuni (11/163 genomes) (Supplementary File S1).
On another hand, the T86V substitution in GyrA (with a prevalence of 24.2% with respect to the total of GyrA substitutions, 8/33 genomes) was detected only in species of the C. lari group: C. insulaenigrae (3/3 genomes), C. lari (1/8 genomes), C. peloridis (1/1 genomes), C. subantarcticus (2/2 genomes) and C. volucris (1/1 genomes) (Supplementary File S1). In contrast, the T86I mutation in GyrA (with a prevalence of 66.7% with respect to the total of GyrA substitutions, 22/33 genomes) was identified mostly in genomes of C. jejuni (18/22), but not in those from the C. lari group (Supplementary File S1).
Prevalence of AR Genotypes in Campylobacter and Its Association With Collection Date, Host and Geographic Location
Genetic factors associated with AR (genes or point mutations) were identified in 77.6% of the Campylobacter genomes analyzed (184/237 genomes; 1 to 8 factors per genome). No genetic determinants associated with AR were found on genomes from C. concisus, C. cuniculorum, C. curvus, C. gracilis, C. hepaticus, C. hominis, and C. pinnipediorum. Genomes carrying factors that putatively confer resistance to 1 or 2 classes of antibiotics were considered to represent a resistance genotype, those carrying factors related with resistance to 3 or more antibiotic classes were considered to be a multidrug resistance (MDR) genotype, and those lacking factors for AR were taken as susceptible genotypes. Most studies on AR in Campylobacter have been performed on the C. coli and C. jejuni species, whereas this phenomenon remains poorly explored for the other Campylobacter species. Thus, to gain more insight about the AR in C. coli and C. jejuni, as well as in the Campylobacter species less studied, we decided to compare the prevalence of resistance or MDR genotypes between genomes from C. coli (22 genomes), C. jejuni (163 genomes) and the other Campylobacter species analyzed (52 genomes). For the genomes from C. coli, the resistance genotype is the most represented (prevalence of 63.6%; 14/22 genomes), followed by the MDR genotype (prevalence of 31.8%; 7/22 genomes) and finally the susceptible genotype (prevalence of 4.6%; 1/22 genomes) (Figure 5). For genomes from C. jejuni, the resistance genotype is also the predominant (prevalence of 78.5%; 128/163 genomes), then the susceptible genotype (prevalence of 13.5%, 22/163 genomes) and at last the MDR genotype (prevalence of 8%; 13/163 genomes) (Figure 5). In contrast, for the genomes from the other Campylobacter species, the susceptible genotype is predominant (prevalence of 57.7%; 30/52 genomes), followed by the resistance genotype (prevalence of 40.4%; 21/52 genomes) and only one MDR genotype was found (prevalence of 1.9%; 1/52 genomes) (Figure 5).
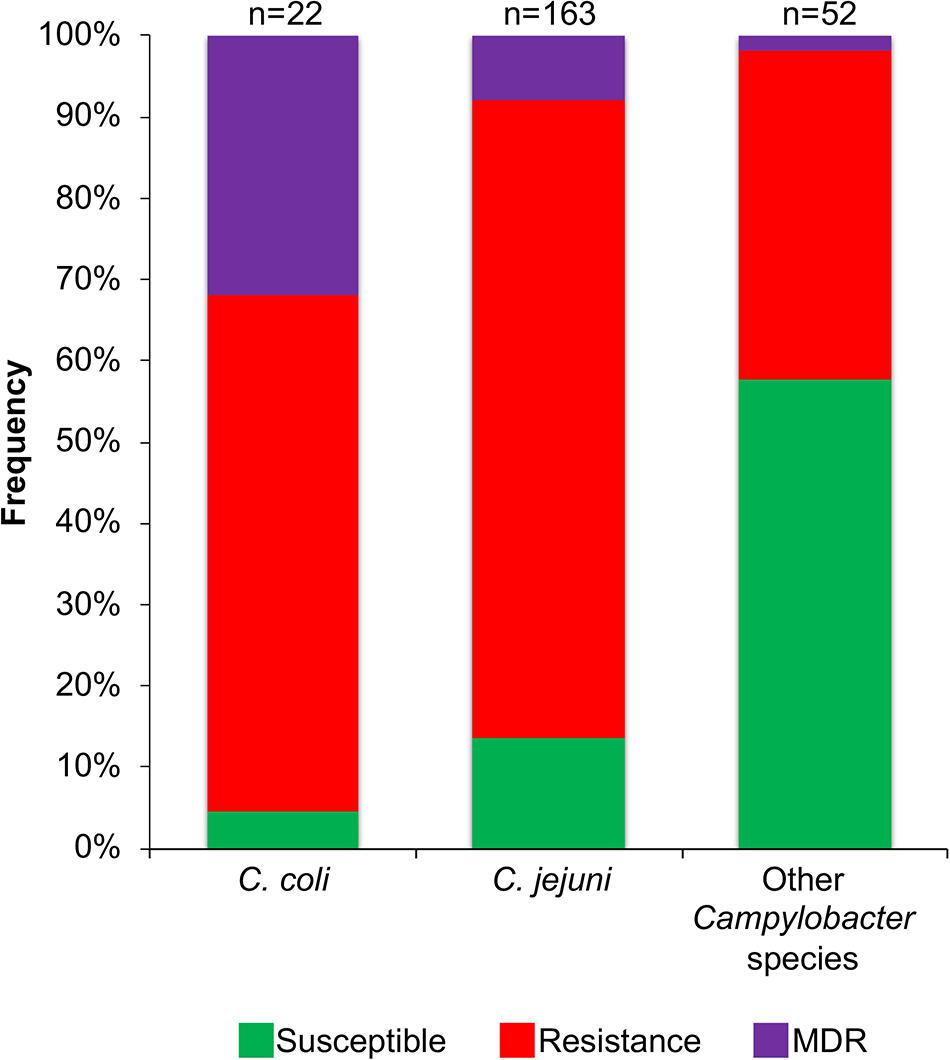
Figure 5. Frequency of susceptible, resistance or multidrug resistance (MDR) genotypes on the 237 Campylobacter genomes tested. Frequencies for genomes from each C. coli, C. jejuni and other Campylobacter species (C. avium, C. concisus, C. cuniculorum, C. curvus, C. fetus, C. gracilis, C. helveticus, C. hepaticus, C. hominis, C. hyointestinalis, C. iguaniorum, C. insulaenigrae, C. lanienae, C. lari, C. peloridis, C. pinnipediorum, C. sputorum, C. subantarcticus, C. ureolyticus, and C. volucris) are indicated. The genotypes were classified as follows: susceptible, lacks genetic determinants for antibiotic resistance; resistance, carries one or more genetic determinants related to resistance to antibiotics belonging to 1 or 2 different classes; MDR, contains one or more genetic determinants related to resistance to antibiotics belonging to 3 or more different classes. The number (n) of the respective genomes analyzed is shown above the columns.
Then, we analyzed the prevalence of the Campylobacter AR genotypes according to the host, collection date and geographical location from where the respective bacteria were isolated (Supplementary File S1). The C. coli isolates from farm animals carry resistance or MDR genotypes, with prevalence of 61.1% (11/18 genomes) and 33.3% (6/18 genomes), respectively, whereas the only 2 C. coli isolates from humans present the resistance genotype (Figure 6A). For C. jejuni, the MDR genotype has a higher prevalence in isolates from farm animals (25.8%; 8/31 genomes) compared with those from humans (3.7%; 4/109 genomes); the opposite was observed for the resistance genotype (Figure 6B). For the other Campylobacter species, the resistance genotype is predominant in bacteria isolated from marine animals (58.3%; 7/12 genomes), whereas the only MDR genotype found in this group is present in the C. lanienae NCTC 13004 strain isolated from a human (6.7%; 1/15 genomes) (Figure 6C). Notably, our analyses show a considerable increase in the presence of MDR genotypes from C. coli (Figure 7A) and C. jejuni (Figure 7B), as well as in the presence of resistance genotypes from the other Campylobacter species group (Figure 7C), in bacteria collected in the last decade (2010–2018), compared with previous decades. Additionally, our results indicate a very high prevalence of AR genotypes in C. coli and C. jejuni isolates from both Europe and North America (88–100%); being the MDR genotype from both C. coli and C. jejuni more predominant in North America (Figures 8A,B). Likewise, our analyses indicate a higher prevalence of AR genotypes from the other Campylobacter species group in Europe (56.3%) with respect to North America (35.7%) (Figure 8C).
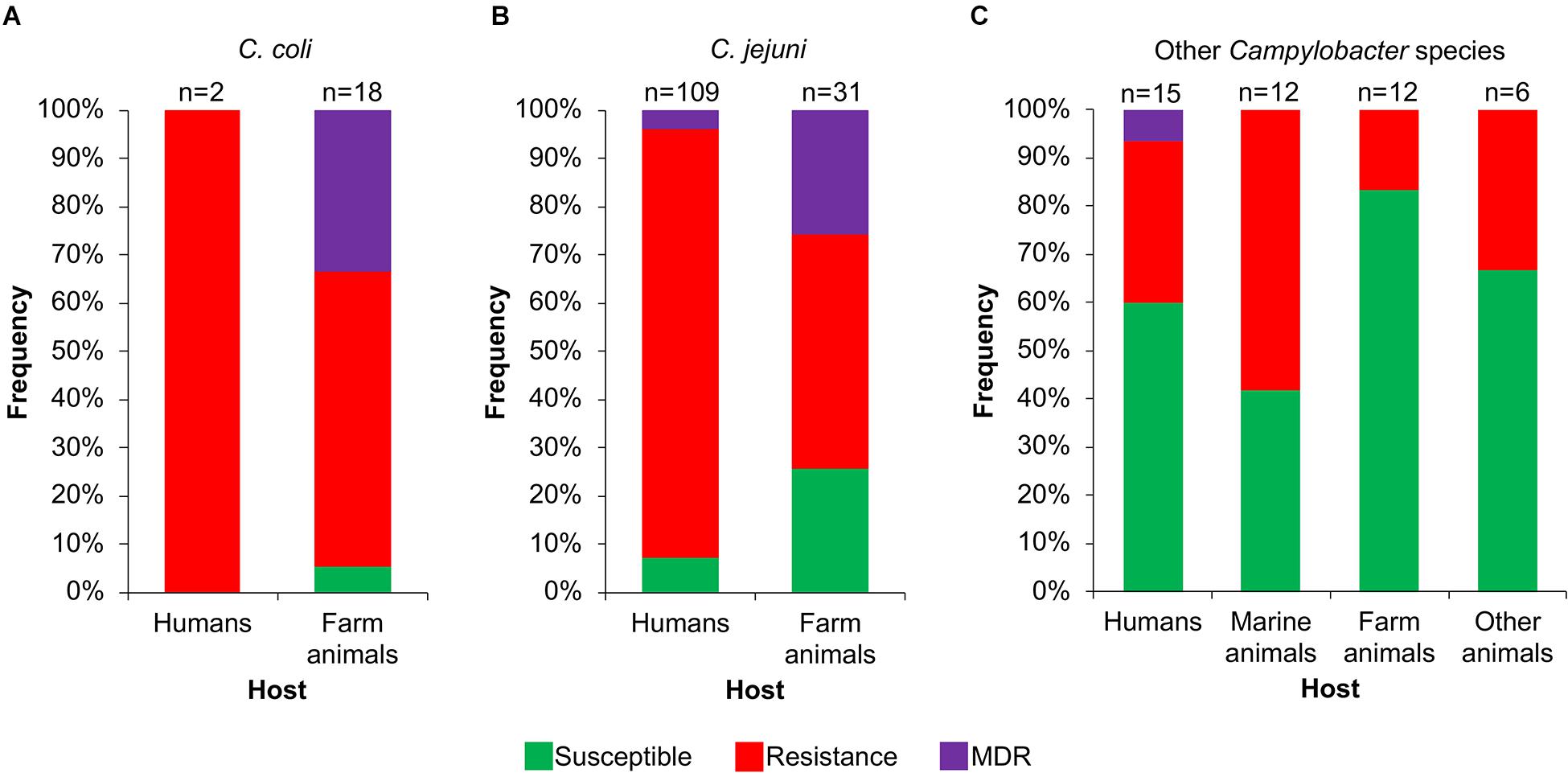
Figure 6. Frequency of susceptible, resistance or multidrug resistance (MDR) genotypes on Campylobacter genomes from isolates collected from different hosts. Genotypes from (A) C. coli, (B) C. jejuni and (C) other 20 Campylobacter species: C. avium, C. concisus, C. cuniculorum, C. curvus, C. fetus, C. gracilis, C. helveticus, C. hepaticus, C. hominis, C. hyointestinalis, C. iguaniorum, C. insulaenigrae, C. lanienae, C. lari, C. peloridis, C. pinnipediorum, C. sputorum, C. subantarcticus, C. ureolyticus, and C. volucris. Farm animals include chicken, bovine, pig, sheep, turkey, and rabbit; marine mammals comprise seal, sea lion, penguin, albatross, gull, and shellfish; other animals encompass iguana, lizard, snake, cat, and alpaca. Only those hosts with more available information are displayed. The number (n) of genomes corresponding to each host is shown above the columns.
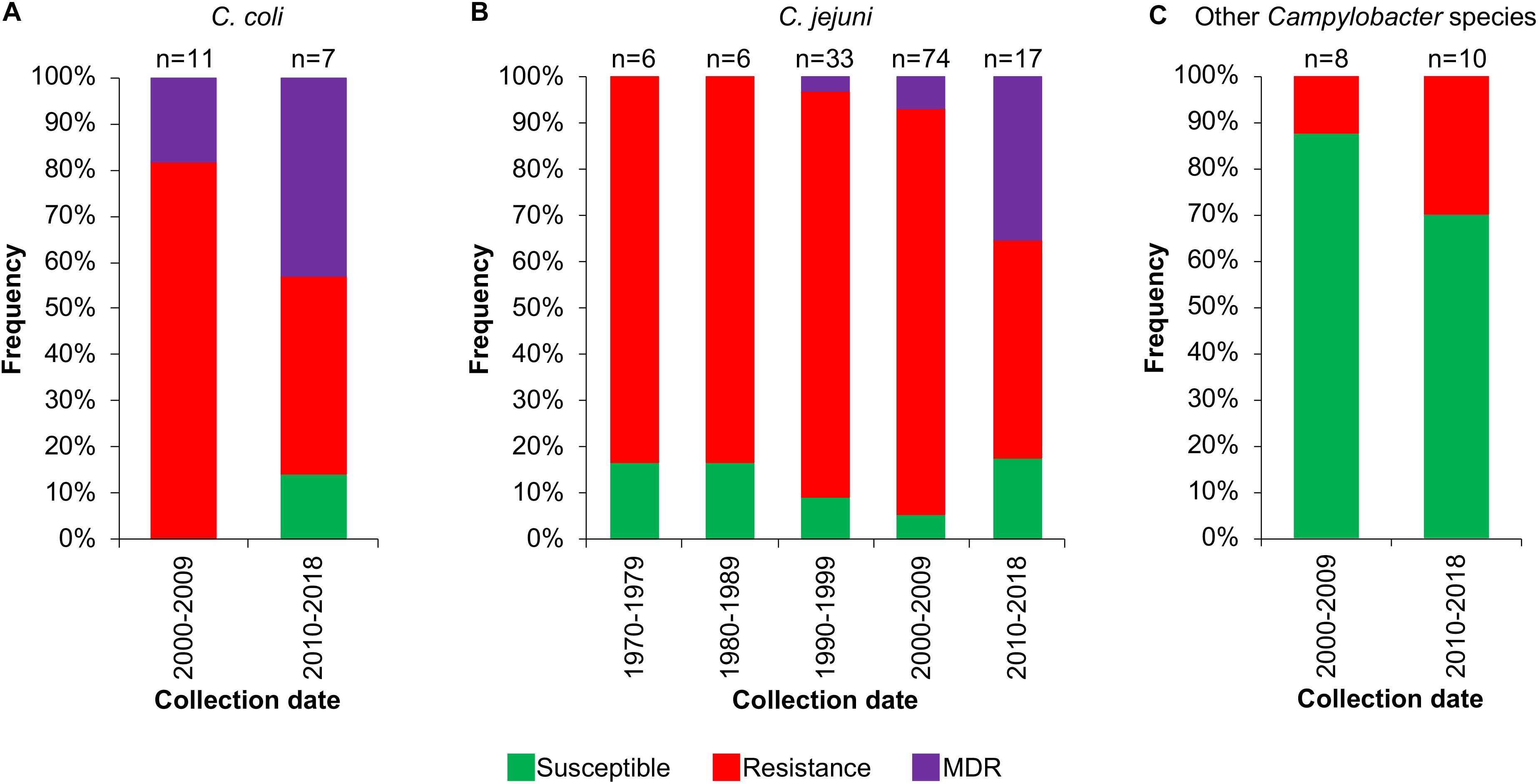
Figure 7. Frequency of susceptible, resistance or multidrug resistance (MDR) genotypes on Campylobacter genomes from isolates collected in different decades. Genotypes from (A) C. coli, (B) C. jejuni and (C) other 9 Campylobacter species: C. avium, C. concisus, C. cuniculorum, C. fetus, C. hepaticus, C. iguaniorum, C. lari, C. pinnipediorum, and C. sputorum. Only those decades with more available information are displayed. The number (n) of genomes corresponding to each decade is shown above the columns.
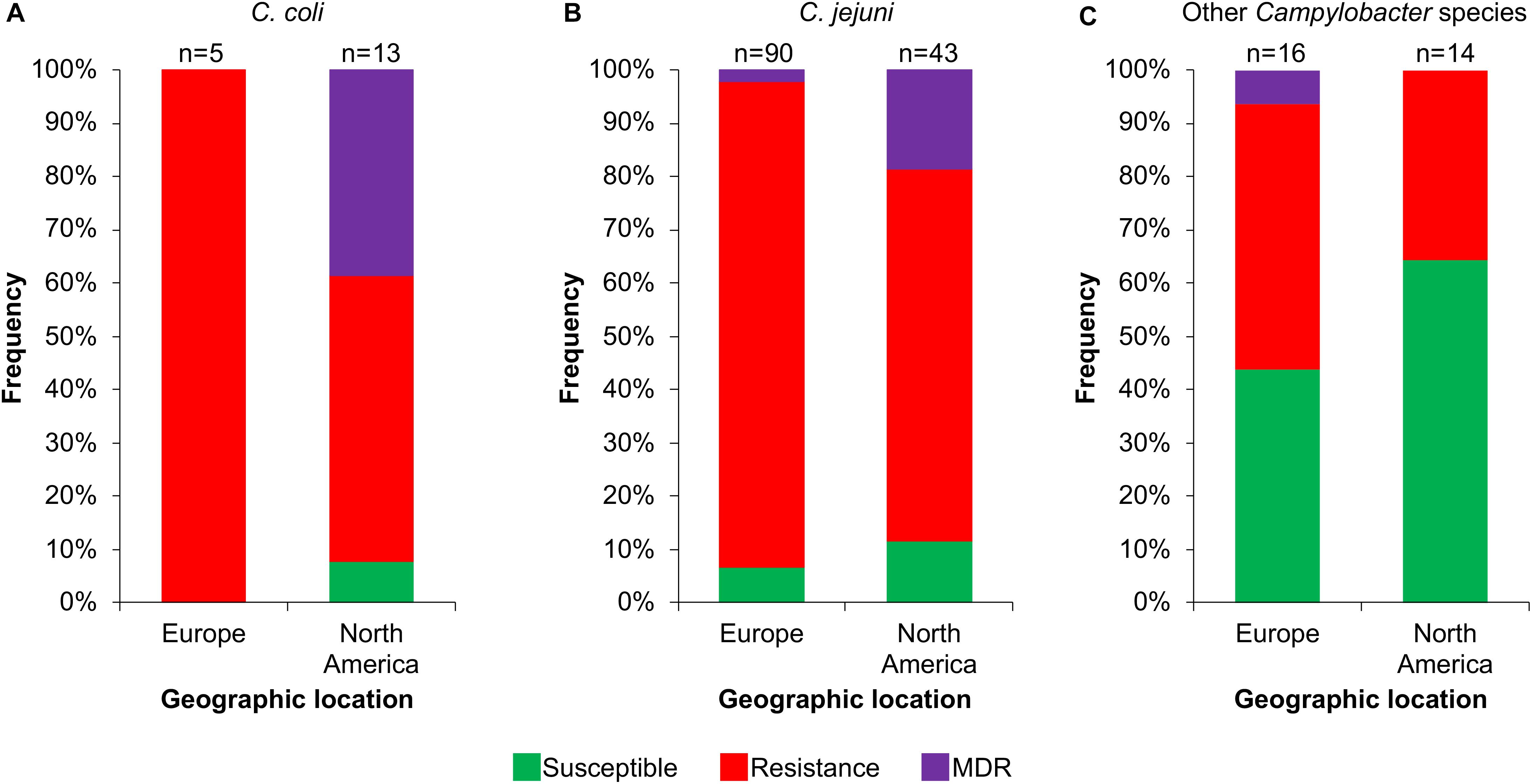
Figure 8. Frequency of susceptible, resistance or multidrug resistance (MDR) genotypes on Campylobacter genomes from isolates collected in different geographic locations. Genotypes from (A) C. coli, (B) C. jejuni and (C) other 18 Campylobacter species: C. avium, C. concisus, C. cuniculorum, C. fetus, C. gracilis, C. helveticus, C. hepaticus, C. hyointestinalis, C. iguaniorum, C. insulaenigrae, C. lanienae, C. lari, C. peloridis, C. pinnipediorum, C. sputorum, C. subantarcticus, C. ureolyticus, and C. volucris. Europe includes Belgium, Denmark, Finland, France, Germany, Italy, Netherlands, Sweden, Switzerland, and United Kingdom; North America comprises Canada and United States. Only those geographic locations with more available information are displayed. The number (n) of genomes corresponding to each geographic location is shown above the columns.
Discussion
Previous studies have analyzed the prevalence of genetic determinants for AR in genomes from C. coli and C. jejuni strains isolated in specific geographic regions (Weis et al., 2016; Zhao et al., 2016; Cantero et al., 2018; de Vries et al., 2018; Fabre et al., 2018; Whitehouse et al., 2018). In the present study, we identified genetic determinants associated with AR in 237 genomes from 22 different Campylobacter species with global distribution.
We found 15 distinct AR genes and point mutations related to AR in 3 housekeeping genes in the Campylobacter genomes tested (Figure 1). Notably, 3 of the AR genes had not been previously reported in Campylobacter: lnu(AN2), putatively associated with lincosamides resistance, and 2 putative β-lactams resistance genes, blaOXA–493 and blaOXA–576. Two β-lactamases coding genes, blaOXA–61 and blaOXA–184, were the most abundant AR genes in the analyzed genomes from C. coli and C. jejuni, which is consistent with the results from previous studies (Griggs et al., 2009; Weis et al., 2016; Zhao et al., 2016; de Vries et al., 2018; Fabre et al., 2018; Whitehouse et al., 2018). In contrast, in the analyzed genomes from species other than C. coli and C. jejuni, the blaOXA–493 β-lactamase coding gene was the predominant (Supplementary File S1). On another hand, even though our study indicates a low occurrence of determinants associated with aminoglycoside resistance in the Campylobacter genomes tested, these determinants displayed a great diversity, since 9 different aminoglycoside resistance genes, as well as point mutations in rpsL, were found (Figure 1). These findings are in agreement with those reported previously (Qin et al., 2012; Zhao et al., 2015, 2016; Cantero et al., 2018; Fabre et al., 2018; Hormeno et al., 2018; Whitehouse et al., 2018).
A high global incidence of Campylobacter isolates resistant to ciprofloxacin (fluoroquinolones) and erythromycin (macrolides) has been reported before (Luangtongkum et al., 2009; Signorini et al., 2018; Sproston et al., 2018). However, our analysis showed a low occurrence of mutations in the gyrA and 23S rRNA genes (Figure 1), which are associated with resistance to fluoroquinolones and macrolides, respectively. Nevertheless, multidrug efflux pumps can also mediate resistance to antibiotics of different classes, such as aminoglycosides, β-lactams, chloramphenicol, fluoroquinolones, macrolides and tetracyclines, among others (Blair et al., 2014). The efflux pumps are ancient elements that play important physiological roles in bacteria; but notably, they can also export antibiotics out of the cell, thus reducing their intracellular concentration and, as consequence, confer AR (Blair et al., 2014; Blanco et al., 2016). CmeABC is the only multidrug efflux pump characterized in Campylobacter; it was shown to be involved in resistance to structurally different antibiotics including β-lactams, fluoroquinolones, macrolides, and tetracyclines (Lin et al., 2002; Luangtongkum et al., 2009; Guo et al., 2010; Shen et al., 2018). In addition to specific genes and point mutations for AR, we also detected genes that code for multiple efflux pumps in Campylobacter (Supplementary File S2). Genes for the CmeABC system, MacB family, macrolide ABC transporter and TolC family efflux pumps, were found in the bulk of analyzed genomes. Besides, genes for other efflux pumps were found only in some genomes, such as those for the AcrB/AcrD/AcrF family, hydrophobe/amphiphile efflux-1 family and multidrug efflux SMR transport efflux pumps. Important to note, given that the efflux pumps can confer resistance to multiple antibiotics, to facilitate interpretation, they were not considered in our analysis as determinants for AR.
A major finding of our work was the Campylobacter species-specific distribution of some genetic determinants for AR. Campylobacter species cluster in 5 discrete phylogenetic clades (Costa and Iraola, 2019). One is the C. lari group, which is composed of six species: C. insulaenigrae, C. lari, C. ornithocola, C. peloridis, C. subantarcticus, and C. volucris (Miller et al., 2014; Costa and Iraola, 2019). These species are highly related at the genome level and have been isolated from similar hosts and environments (Miller et al., 2014). We found the blaOXA–493 gene and the T86V substitution in GyrA, exclusively in species from the C. lari group (Supplementary File S1). The T86V mutation in GyrA had also been previously reported in the C. lari species (Piddock et al., 2003; Weis et al., 2016). In contrast, aminoglycoside resistance genes were only detected in the classical C. coli and C. jejuni species (Supplementary File S1). Previous studies also have indicated the presence of aminoglycoside resistance genes in C. jejuni and C. coli (Qin et al., 2012; Zhao et al., 2015, 2016; Cantero et al., 2018; Fabre et al., 2018; Whitehouse et al., 2018); however, the presence of these genes in species other than C. jejuni and C. coli had not been analyzed. Thus, our data show that the genetic variability between the different clades of Campylobacter also involves the determinants for AR. Due to the limited number of genomes from non-C. coli/C. jejuni species (52 genomes), we cannot discard the possibility that aminoglycoside resistance genes are present in these species, but in very low prevalence.
Consistent with previous reports (NARMS, 2012; de Vries et al., 2018; Whitehouse et al., 2018), we found that the C. coli genomes harbor the highest number of AR determinants, compared with the other Campylobacter species analyzed (Supplementary File S1). Furthermore, in agreement with previous studies (Thakur et al., 2010; Wieczorek et al., 2015), our results show a higher prevalence of MDR genotypes in C. coli (31.8%; 7/22 genomes) with respect to C. jejuni (8%; 13/163 genomes). Notably, 86.4% (19/22 genomes) of the C. coli genomes possess 1–4 plasmids; in contrast, only 14.1% (23/163 genomes) of the C. jejuni genomes carry 1 or 2 plasmids, and 25% (13/52 genomes) of the genomes from the remaining 20 Campylobacter species tested contain 1–5 plasmids (Supplementary File S1). Interestingly, most genes associated with AR in C. coli are located on plasmids, whereas in the other Campylobacter species are placed on chromosome (Supplementary File S1), which could explain, at least in part, the higher number of AR determinants and MDR genotypes in C. coli compared with the other Campylobacter species. Remarkably, in non-C. coli/C. jejuni species, AR genes located on plasmids were not identified (Figure 2 and Supplementary File S1), Campylobacter spp. have mechanisms for conjugation and natural transformation, and transferrable AR has been documented in this genus (Taylor and Courvalin, 1988). Moreover, it has been proposed that Campylobacter gained some AR genes from Gram-positive bacteria (Taylor and Courvalin, 1988; Zilhao et al., 1988; Alfredson and Korolik, 2007). Thus, the AR genes located on plasmids, or on other mobile elements, imply a threat for the appearance of new Campylobacter strains resistant to antibiotics.
We found a reduced number of genetic determinants for AR in Campylobacter, in comparison with the huge number of genetic determinants for AR present in other bacteria such as Enterococcus spp. (Torres et al., 2018), Escherichia coli (Poirel et al., 2018) or Salmonella enterica (McDermott et al., 2018), which share hosts and niches with Campylobacter spp. The reason why Campylobacter spp. maintain in general a low number of genetic determinants for AR remains to be determined.
Our results, together with previous studies, reveal a high prevalence of Campylobacter AR genotypes worldwide, not only from the classical C. jeuni and C. coli species, but also, although still with less extent, from emerging Campylobacter species; these AR genotypes can be present in bacteria residing in different hosts such as humans and different animals (Figures 5,6,8 and Supplementary File S1). Even more worryingly, our data support that the prevalence of these bacteria carrying AR genotypes has been increasing with time (Figure 7).
Although the phenotype conferred by all the resistance genetic determinants identified in this study needs to be tested, a very high correlation between the presence of genetic determinants for AR and the respective phenotype has been reported in Campylobacter spp., which reaches up to 100% of correspondence for some specific antibiotics (Nirdnoy et al., 2005; Chen et al., 2013; Zhao et al., 2015, 2016; Fabre et al., 2018; Whitehouse et al., 2018). Hence, it has been suggested that analysis of genomic data, for the identification of genetic determinants associated with AR, has the potential to reliably predict resistance phenotypes (Zhao et al., 2016; Whitehouse et al., 2018; Feldgarden et al., 2019).
Thus, our study, together with other reports, provide genetic determinants that can be used to predict AR in Campylobacter spp., which could greatly help to select the best antibiotic therapy against infections caused by these bacteria. Additionally, our study further expands the knowledge on the genetic elements that shape the resistome of the genus Campylobacter and on the scattering of these AR genetic determinants between the Campylobacter species.
Data Availability Statement
All datasets generated for this study are included in the article/Supplementary Material.
Author Contributions
DP-M and VB contributed to the conception and design of the study, as well as the discussion of the results. DR-M, IM-F, RS, and LL carried out the bioinformatics work. DP-M analyzed the bioinformatics results, created the figures, and wrote the manuscript. VB edited the manuscript. All authors contributed to the article and approved the submitted version.
Funding
This work was supported by a grant from the Consejo Nacional de Ciencia y Tecnología (CONACYT)/México (2017-01-5182) to VB. DR-M was supported by a master fellowship from CONACYT (grant 771346).
Conflict of Interest
The authors declare that the research was conducted in the absence of any commercial or financial relationships that could be construed as a potential conflict of interest.
Supplementary Material
The Supplementary Material for this article can be found online at: https://www.frontiersin.org/articles/10.3389/fmicb.2020.513070/full#supplementary-material
Footnotes
- ^ www.bacterio.net/campylobacter.html
- ^ https://www.who.int/news-room/fact-sheets/detail/campylobacter
- ^ https://www.ncbi.nlm.nih.gov/refseq/
- ^ https://card.mcmaster.ca/
- ^ https://www.ncbi.nlm.nih.gov/pathogens/antimicrobial-resistance/
- ^ https://blast.ncbi.nlm.nih.gov/Blast.cgi
- ^ https://cge.cbs.dtu.dk/services/ResFinder/
- ^ https://www.megasoftware.net
References
Agunos, A., Arsenault, R. K., Avery, B. P., Deckert, A. E., Gow, S. P., Janecko, N., et al. (2018). Changes in antimicrobial resistance levels among Escherichia coli, Salmonella, and Campylobacter in Ontario broiler chickens between 2003 and 2015. Can. J. Vet. Res. 82, 163–177.
Alfredson, D. A., and Korolik, V. (2005). Isolation and expression of a novel molecular class D beta-lactamase, OXA-61, from Campylobacter jejuni. Antimicrob. Agents Chemother. 49, 2515–2518. doi: 10.1128/AAC.49.6.2515-2518.2005
Alfredson, D. A., and Korolik, V. (2007). Antibiotic resistance and resistance mechanisms in Campylobacter jejuni and Campylobacter coli. FEMS Microbiol. Lett. 277, 123–132. doi: 10.1111/j.1574-6968.2007.00935.x
Bachoual, R., Ouabdesselam, S., Mory, F., Lascols, C., Soussy, C. J., and Tankovic, J. (2001). Single or double mutational alterations of gyrA associated with fluoroquinolone resistance in Campylobacter jejuni and Campylobacter coli. Microb. Drug Resist. 7, 257–261. doi: 10.1089/10766290152652800
Bailey, R. A., Kranis, A., Psifidi, A., Watson, K. A., Rothwell, L., Hocking, P. M., et al. (2018). Colonization of a commercial broiler line by Campylobacter is under limited genetic control and does not significantly impair performance or intestinal health. Poult. Sci. 97, 4167–4176. doi: 10.3382/ps/pey295
Blair, J. M., Richmond, G. E., and Piddock, L. J. (2014). Multidrug efflux pumps in Gram-negative bacteria and their role in antibiotic resistance. Future Microbiol. 9, 1165–1177. doi: 10.2217/fmb.14.66
Blanco, P., Hernando-Amado, S., Reales-Calderon, J. A., Corona, F., Lira, F., Alcalde-Rico, M., et al. (2016). Bacterial multidrug efflux pumps: much more than antibiotic resistance determinants. Microorganisms 4:14. doi: 10.3390/microorganisms4010014
Cantero, G., Correa-Fiz, F., Ronco, T., Strube, M., Cerda-Cuellar, M., and Pedersen, K. (2018). Characterization of Campylobacter jejuni and Campylobacter coli broiler isolates by whole-genome sequencing. Foodborne Pathog. Dis. 15, 145–152. doi: 10.1089/fpd.2017.2325
CDC (2013). Antibiotic Resistance Threats in the United States, 2013. U.S. Department of Health and Human Services. Atlanta, GA: Centers for Disease Control and Prevention.
Charvalos, E., Peteinaki, E., Spyridaki, I., Manetas, S., and Tselentis, Y. (1996). Detection of ciprofloxacin resistance mutations in Campylobacter jejuni gyrA by nonradioisotopic single-strand conformation polymorphism and direct DNA sequencing. J. Clin. Lab. Anal. 10, 129–133. doi: 10.1002/(sici)1098-2825(1996)10:3<129::aid-jcla3>3.0.co;2-6
Chaudhari, N. M., Gupta, V. K., and Dutta, C. (2016). BPGA- an ultra-fast pan-genome analysis pipeline. Sci. Rep. 6:24373. doi: 10.1038/srep24373
Chen, Y., Mukherjee, S., Hoffmann, M., Kotewicz, M. L., Young, S., Abbott, J., et al. (2013). Whole-genome sequencing of gentamicin-resistant Campylobacter coli isolated from U.S. retail meats reveals novel plasmid-mediated aminoglycoside resistance genes. Antimicrob. Agents Chemother. 57, 5398–5405. doi: 10.1128/aac.00669-13
Cody, A. J., Clarke, L., Bowler, I. C., and Dingle, K. E. (2010). Ciprofloxacin-resistant campylobacteriosis in the UK. Lancet 376:1987. doi: 10.1016/s0140-6736(10)62261-1
Costa, D., and Iraola, G. (2019). Pathogenomics of emerging Campylobacter species. Clin. Microbiol. Rev. 32:e00072-18.
Dasti, J. I., Gross, U., Pohl, S., Lugert, R., Weig, M., and Schmidt-Ott, R. (2007). Role of the plasmid-encoded tet(O) gene in tetracycline-resistant clinical isolates of Campylobacter jejuni and Campylobacter coli. J. Med. Microbiol. 56(Pt 6), 833–837. doi: 10.1099/jmm.0.47103-0
D’Costa, V. M., McGrann, K. M., Hughes, D. W., and Wright, G. D. (2006). Sampling the antibiotic resistome. Science 311, 374–377. doi: 10.1126/science.1120800
de Vries, S. P. W., Vurayai, M., Holmes, M., Gupta, S., Bateman, M., Goldfarb, D., et al. (2018). Phylogenetic analyses and antimicrobial resistance profiles of Campylobacter spp. from diarrhoeal patients and chickens in Botswana. PLoS One 13:e0194481. doi: 10.1371/journal.pone.0194481
EFSA and ECDC (2018). The European Union summary report on trends and sources of zoonoses, zoonotic agents and food-borne outbreaks in 2017. EFSA J. 16:5500. doi: 10.2903/j.efsa.2018.5500
Ewers, E. C., Anisowicz, S. K., Ferguson, T. M., Seronello, S. E., Barnhill, J. C., Lustik, M. B., et al. (2018). Antibiotic resistance, molecular characterizations, and clinical manifestations of Campylobacteriosis at a military medical center in Hawaii from 2012-2016: a retrospective analysis. Sci. Rep. 8:11736. doi: 10.1038/s41598-018-29461-z
Fabre, A., Oleastro, M., Nunes, A., Santos, A., Sifre, E., Ducournau, A., et al. (2018). Whole-genome sequence analysis of multidrug-resistant Campylobacter isolates: a focus on aminoglycoside resistance determinants. J. Clin. Microbiol. 56:e00390-18.
Feldgarden, M., Brover, V., Haft, D. H., Prasad, A. B., Slotta, D. J., Tolstoy, I., et al. (2019). Validating the AMRFinder tool and resistance gene database by using antimicrobial resistance genotype-phenotype correlations in a collection of isolates. Antimicrob. Agents Chemother. 63:e00483-19.
Ge, B., Wang, F., Sjolund-Karlsson, M., and McDermott, P. F. (2013). Antimicrobial resistance in Campylobacter: susceptibility testing methods and resistance trends. J. Microbiol. Methods 95, 57–67. doi: 10.1016/j.mimet.2013.06.021
Gibreel, A., Kos, V. N., Keelan, M., Trieber, C. A., Levesque, S., Michaud, S., et al. (2005). Macrolide resistance in Campylobacter jejuni and Campylobacter coli: molecular mechanism and stability of the resistance phenotype. Antimicrob. Agents Chemother. 49, 2753–2759. doi: 10.1128/AAC.49.7.2753-2759.2005
Griggs, D. J., Peake, L., Johnson, M. M., Ghori, S., Mott, A., and Piddock, L. J. (2009). Beta-lactamase-mediated beta-lactam resistance in Campylobacter species: prevalence of Cj0299 (bla OXA-61) and evidence for a novel beta-Lactamase in C. jejuni. Antimicrob. Agents Chemother. 53, 3357–3364. doi: 10.1128/aac.01655-08
Guo, B., Lin, J., Reynolds, D. L., and Zhang, Q. (2010). Contribution of the multidrug efflux transporter CmeABC to antibiotic resistance in different Campylobacter species. Foodborne Pathog. Dis. 7, 77–83. doi: 10.1089/fpd.2009.0354
Hakanen, A., Jalava, J., Kotilainen, P., Jousimies-Somer, H., Siitonen, A., and Huovinen, P. (2002). gyrA polymorphism in Campylobacter jejuni: detection of gyrA mutations in 162 C. jejuni isolates by single-strand conformation polymorphism and DNA sequencing. Antimicrob. Agents Chemother. 46, 2644–2647. doi: 10.1128/aac.46.8.2644-2647.2002
Hanninen, M. L., and Hannula, M. (2007). Spontaneous mutation frequency and emergence of ciprofloxacin resistance in Campylobacter jejuni and Campylobacter coli. J. Antimicrob. Chemother. 60, 1251–1257. doi: 10.1093/jac/dkm345
Havelaar, A. H., Kirk, M. D., Torgerson, P. R., Gibb, H. J., Hald, T., Lake, R. J., et al. (2015). World health organization global estimates and regional comparisons of the burden of foodborne disease in 2010. PLoS Med. 12:e1001923. doi: 10.1371/journal.pmed.1001923
Hormeno, L., Ugarte-Ruiz, M., Palomo, G., Borge, C., Florez-Cuadrado, D., Vadillo, S., et al. (2018). ant(6)-I genes encoding aminoglycoside o-nucleotidyltransferases are widely spread among streptomycin resistant strains of Campylobacter jejuni and Campylobacter coli. Front. Microbiol. 9:2515. doi: 10.3389/fmicb.2018.02515
Hu, Y., Gao, G. F., and Zhu, B. (2017). The antibiotic resistome: gene flow in environments, animals and human beings. Front. Med. 11, 161–168. doi: 10.1007/s11684-017-0531-x
Iglesias-Torrens, Y., Miro, E., Guirado, P., Llovet, T., Munoz, C., Cerda-Cuellar, M., et al. (2018). Population structure, antimicrobial resistance, and virulence-associated genes in Campylobacter jejuni isolated from three ecological niches: gastroenteritis patients, broilers, and wild birds. Front. Microbiol. 9:1676. doi: 10.3389/fmicb.2018.01676
Iovine, N. M. (2013). Resistance mechanisms in Campylobacter jejuni. Virulence 4, 230–240. doi: 10.4161/viru.23753
Ishihara, K., Kira, T., Ogikubo, K., Morioka, A., Kojima, A., Kijima-Tanaka, M., et al. (2004). Antimicrobial susceptibilities of Campylobacter isolated from food-producing animals on farms (1999-2001): results from the Japanese veterinary antimicrobial resistance monitoring program. Int. J. Antimicrob. Agents 24, 261–267. doi: 10.1016/j.ijantimicag.2004.03.017
Jacob, J., Evers, S., Bischoff, K., Carlier, C., and Courvalin, P. (1994). Characterization of the sat4 gene encoding a streptothricin acetyltransferase in Campylobacter coli BE/G4. FEMS Microbiol. Lett. 120, 13–17. doi: 10.1111/j.1574-6968.1994.tb07000.x
Jensen, L. B., and Aarestrup, F. M. (2001). Macrolide resistance in Campylobacter coli of animal origin in Denmark. Antimicrob. Agents Chemother. 45, 371–372. doi: 10.1128/aac.45.1.371-372.2001
Jia, B., Raphenya, A. R., Alcock, B., Waglechner, N., Guo, P., Tsang, K. K., et al. (2017). CARD 2017: expansion and model-centric curation of the comprehensive antibiotic resistance database. Nucleic Acids Res. 45, D566–D573. doi: 10.1093/nar/gkw1004
Kaakoush, N. O., Castano-Rodriguez, N., Mitchell, H. M., and Man, S. M. (2015). Global epidemiology of Campylobacter infection. Clin. Microbiol. Rev. 28, 687–720. doi: 10.1128/cmr.00006-15
Karikari, A. B., Obiri-Danso, K., Frimpong, E. H., and Krogfelt, K. A. (2017). Antibiotic resistance of Campylobacter recovered from faeces and carcasses of healthy livestock. Biomed Res. Int. 2017:4091856. doi: 10.1155/2017/4091856
Khan, J. A., Rathore, R. S., Abulreesh, H. H., Qais, F. A., and Ahmad, I. (2018). Prevalence and antibiotic resistance profiles of Campylobacter jejuni isolated from poultry meat and related samples at retail shops in Northern India. Foodborne Pathog. Dis. 15, 218–225. doi: 10.1089/fpd.2017.2344
Kweon, O. J., Lim, Y. K., Yoo, B., Kim, H. R., Kim, T. H., and Lee, M. K. (2015). First case report of Campylobacter volucris bacteremia in an immunocompromised patient. J. Clin. Microbiol. 53, 1976–1978. doi: 10.1128/jcm.00442-15
Lee, M. H. M., Pan, D. S. T., Huang, J. H., Chen, M. I., Chong, J. W. C., Goh, E. H., et al. (2017). Results from a patient-based health education intervention in reducing antibiotic use for acute upper respiratory tract infections in the private sector primary care setting in Singapore. Antimicrob. Agents Chemother. 61:e02257-16.
Lin, J., Michel, L. O., and Zhang, Q. (2002). CmeABC functions as a multidrug efflux system in Campylobacter jejuni. Antimicrob. Agents Chemother. 46, 2124–2131. doi: 10.1128/aac.46.7.2124-2131.2002
Luangtongkum, T., Jeon, B., Han, J., Plummer, P., Logue, C. M., and Zhang, Q. (2009). Antibiotic resistance in Campylobacter: emergence, transmission and persistence. Future Microbiol. 4, 189–200. doi: 10.2217/17460913.4.2.189
Man, S. M. (2011). The clinical importance of emerging Campylobacter species. Nat. Rev. Gastroenterol. Hepatol. 8, 669–685. doi: 10.1038/nrgastro.2011.191
Marasini, D., Karki, A. B., Buchheim, M. A., and Fakhr, M. K. (2018). Phylogenetic relatedness among plasmids harbored by Campylobacter jejuni and Campylobacter coli isolated from retail meats. Front. Microbiol. 9:2167. doi: 10.3389/fmicb.2018.02167
McDermott, P. F., Zhao, S., and Tate, H. (2018). Antimicrobial resistance in nontyphoidal Salmonella. Microbiol. Spectr. 6:ARBA-0014-2017.
McIver, C., Hogan, T., White, P., and Tapsall, J. (2004). Patterns of quinolone susceptibility in Campylobacter jejuni associated with different gyrA mutations. Pathology 36, 166–169. doi: 10.1080/00313020410001672019
Miller, W. G., Yee, E., Chapman, M. H., Smith, T. P., Bono, J. L., Huynh, S., et al. (2014). Comparative genomics of the Campylobacter lari group. Genome Biol. Evol. 6, 3252–3266. doi: 10.1093/gbe/evu249
Nirdnoy, W., Mason, C. J., and Guerry, P. (2005). Mosaic structure of a multiple-drug-resistant, conjugative plasmid from Campylobacter jejuni. Antimicrob. Agents Chemother. 49, 2454–2459. doi: 10.1128/AAC.49.6.2454-2459.2005
Nowaczek, A., Urban-Chmiel, R., Dec, M., Puchalski, A., Stepien-Pysniak, D., Marek, A., et al. (2019). Campylobacter spp. and bacteriophages from broiler chickens: characterization of antibiotic susceptibility profiles and lytic bacteriophages. Microbiologyopen 8:e00784. doi: 10.1002/mbo3.784
Olkkola, S., Juntunen, P., Heiska, H., Hyytiainen, H., and Hanninen, M. L. (2010). Mutations in the rpsL gene are involved in streptomycin resistance in Campylobacter coli. Microb. Drug Resist. 16, 105–110. doi: 10.1089/mdr.2009.0128
O’Neill, J. (2014). Antimicrobial Resistance: Tackling a Crisis for the Health and Wealth of Nations. London: The Review on Antimicrobial Resistance.
Payot, S., Bolla, J. M., Corcoran, D., Fanning, S., Megraud, F., and Zhang, Q. (2006). Mechanisms of fluoroquinolone and macrolide resistance in Campylobacter spp. Microbes Infect. 8, 1967–1971. doi: 10.1016/j.micinf.2005.12.032
Piddock, L. J., Ricci, V., Pumbwe, L., Everett, M. J., and Griggs, D. J. (2003). Fluoroquinolone resistance in Campylobacter species from man and animals: detection of mutations in topoisomerase genes. J. Antimicrob. Chemother. 51, 19–26. doi: 10.1093/jac/dkg033
Poirel, L., Madec, J. Y., Lupo, A., Schink, A. K., Kieffer, N., Nordmann, P., et al. (2018). Antimicrobial resistance in Escherichia coli. Microbiol. Spectr. 6:ARBA-0026-2017. doi: 10.1016/b978-0-12-801214-7.00002-8
Pratt, A., and Korolik, V. (2005). Tetracycline resistance of Australian Campylobacter jejuni and Campylobacter coli isolates. J. Antimicrob. Chemother. 55, 452–460. doi: 10.1093/jac/dki040
Premarathne, J., Anuar, A. S., Thung, T. Y., Satharasinghe, D. A., Jambari, N. N., Abdul-Mutalib, N. A., et al. (2017). Prevalence and antibiotic resistance against tetracycline in Campylobacter jejuni and C. coli in cattle and beef meat from Selangor, Malaysia. Front. Microbiol. 8:2254. doi: 10.3389/fmicb.2017.02254
Qin, S., Wang, Y., Zhang, Q., Chen, X., Shen, Z., Deng, F., et al. (2012). Identification of a novel genomic island conferring resistance to multiple aminoglycoside antibiotics in Campylobacter coli. Antimicrob. Agents Chemother. 56, 5332–5339. doi: 10.1128/aac.00809-12
Ren, G. W., Wang, Y., Shen, Z., Chen, X., Shen, J., and Wu, C. (2011). Rapid detection of point mutations in domain V of the 23S rRNA gene in erythromycin-resistant Campylobacter isolates by pyrosequencing. Foodborne Pathog. Dis. 8, 375–379. doi: 10.1089/fpd.2010.0676
Ruiz, J., Moreno, A., Jimenez de Anta, M. T., and Vila, J. (2005). A double mutation in the gyrA gene is necessary to produce high levels of resistance to moxifloxacin in Campylobacter spp. clinical isolates. Int. J. Antimicrob. Agents 25, 542–545. doi: 10.1016/j.ijantimicag.2004.10.016
Schiaffino, F., Colston, J. M., Paredes-Olortegui, M., Francois, R., Pisanic, N., Burga, R., et al. (2019). Antibiotic resistance of Campylobacter species in a pediatric cohort study. Antimicrob. Agents Chemother. 63:e01911-18.
Shen, Z., Wang, Y., Zhang, Q., and Shen, J. (2018). Antimicrobial resistance in Campylobacter spp. Microbiol. Spectr. 6:ARBA-0013-2017.
Signorini, M. L., Rossler, E., Diaz David, D. C., Olivero, C. R., Romero-Scharpen, A., Soto, L. P., et al. (2018). Antimicrobial resistance of thermotolerant Campylobacter species isolated from humans, food-producing animals, and products of animal origin: a worldwide meta-analysis. Microb. Drug Resist. 24, 1174–1190. doi: 10.1089/mdr.2017.0310
Silva, J., Leite, D., Fernandes, M., Mena, C., Gibbs, P. A., and Teixeira, P. (2011). Campylobacter spp. as a foodborne pathogen: a review. Front. Microbiol. 2:200. doi: 10.3389/fmicb.2011.00200
Smith, J. L., and Fratamico, P. M. (2010). Fluoroquinolone resistance in Campylobacter. J. Food Prot. 73, 1141–1152. doi: 10.4315/0362-028x-73.6.1141
Sougakoff, W., Papadopoulou, B., Nordmann, P., and Courvalin, P. (1987). Nucleotide sequence and distribution of gene tetO encoding tetracycline resistance in Campylobacter coli. FEMS Microbiol. Lett. 44, 153–159.
Sproston, E. L., Wimalarathna, H. M. L., and Sheppard, S. K. (2018). Trends in fluoroquinolone resistance in Campylobacter. Microb. Genom. 4:e000198. doi: 10.1099/mgen.0.000198
Tang, Y., Fang, L., Xu, C., and Zhang, Q. (2017). Antibiotic resistance trends and mechanisms in the foodborne pathogen, Campylobacter. Anim. Health Res. Rev. 18, 87–98. doi: 10.1017/S1466252317000135
Taylor, D. E., and Courvalin, P. (1988). Mechanisms of antibiotic resistance in Campylobacter species. Antimicrob. Agents Chemother. 32, 1107–1112. doi: 10.1128/aac.32.8.1107
Thakur, S., Zhao, S., McDermott, P. F., Harbottle, H., Abbott, J., English, L., et al. (2010). Antimicrobial resistance, virulence, and genotypic profile comparison of Campylobacter jejuni and Campylobacter coli isolated from humans and retail meats. Foodborne Pathog. Dis. 7, 835–844. doi: 10.1089/fpd.2009.0487
Torres, C., Alonso, C. A., Ruiz-Ripa, L., Leon-Sampedro, R., Del Campo, R., and Coque, T. M. (2018). Antimicrobial resistance in Enterococcus spp. of animal origin. Microbiol. Spectr. 6:ARBA-0032-2018.
Vacher, S., Menard, A., Bernard, E., and Megraud, F. (2003). PCR-restriction fragment length polymorphism analysis for detection of point mutations associated with macrolide resistance in Campylobacter spp. Antimicrob. Agents Chemother. 47, 1125–1128. doi: 10.1128/aac.47.3.1125-1128.2003
Vandamme, P., Dewhirst, F. E., Paster, B. J., and On, S. L. W. (2015). “Campylobacter,” in Bergey’s Manual of Systematics of Archaea and Bacteria, Online, ed. W. B. Whitman (Hoboken, NJ: John Wiley & Sons, Inc).
Wang, J., Shoemaker, N. B., Wang, G. R., and Salyers, A. A. (2000). Characterization of a Bacteroides mobilizable transposon, NBU2, which carries a functional lincomycin resistance gene. J. Bacteriol. 182, 3559–3571. doi: 10.1128/jb.182.12.3559-3571.2000
Wang, Y., Huang, W. M., and Taylor, D. E. (1993). Cloning and nucleotide sequence of the Campylobacter jejuni gyrA gene and characterization of quinolone resistance mutations. Antimicrob. Agents Chemother. 37, 457–463. doi: 10.1128/aac.37.3.457
Wang, Y., and Taylor, D. E. (1990). Chloramphenicol resistance in Campylobacter coli: nucleotide sequence, expression, and cloning vector construction. Gene 94, 23–28. doi: 10.1016/0378-1119(90)90463-2
Wei, B., and Kang, M. (2018). In vitro activity of fosfomycin against Campylobacter isolates from poultry and wild birds. PLoS One 13:e0200853. doi: 10.1371/journal.pone.0200853
Weis, A. M., Storey, D. B., Taff, C. C., Townsend, A. K., Huang, B. C., Kong, N. T., et al. (2016). Genomic comparison of Campylobacter spp. and their potential for zoonotic transmission between birds, primates, and livestock. Appl. Environ. Microbiol. 82, 7165–7175. doi: 10.1128/aem.01746-16
Whitehouse, C. A., Young, S., Li, C., Hsu, C. H., Martin, G., and Zhao, S. (2018). Use of whole-genome sequencing for Campylobacter surveillance from NARMS retail poultry in the United States in 2015. Food Microbiol. 73, 122–128. doi: 10.1016/j.fm.2018.01.018
WHO (2017). Global Priority List of Antibiotic-Resistant Bacteria to Guide Research, Discovery, and Development of New Antibiotics. Geneva: WHO.
Wieczorek, K., Denis, E., and Osek, J. (2015). Comparative analysis of antimicrobial resistance and genetic diversity of Campylobacter from broilers slaughtered in Poland. Int. J. Food Microbiol. 210, 24–32. doi: 10.1016/j.ijfoodmicro.2015.06.006
Wright, G. D. (2007). The antibiotic resistome: the nexus of chemical and genetic diversity. Nat. Rev. Microbiol. 5, 175–186. doi: 10.1038/nrmicro1614
Zankari, E., Hasman, H., Cosentino, S., Vestergaard, M., Rasmussen, S., Lund, O., et al. (2012). Identification of acquired antimicrobial resistance genes. J. Antimicrob. Chemother. 67, 2640–2644. doi: 10.1093/jac/dks261
Zhang, X., Tang, M., Zhou, Q., Zhang, J., Yang, X., and Gao, Y. (2018). Prevalence and characteristics of Campylobacter throughout the slaughter process of different broiler batches. Front. Microbiol. 9:2092. doi: 10.3389/fmicb.2018.02092
Zhao, S., Mukherjee, S., Chen, Y., Li, C., Young, S., Warren, M., et al. (2015). Novel gentamicin resistance genes in Campylobacter isolated from humans and retail meats in the USA. J. Antimicrob. Chemother. 70, 1314–1321. doi: 10.1093/jac/dkv001
Zhao, S., Tyson, G. H., Chen, Y., Li, C., Mukherjee, S., Young, S., et al. (2016). Whole-genome sequencing analysis accurately predicts antimicrobial resistance phenotypes in Campylobacter spp. Appl. Environ. Microbiol. 82, 459–466. doi: 10.1128/aem.02873-15
Keywords: Campylobacter, zoonotic bacteria, antibiotic resistance, resistance genes, genomic analysis, resistome
Citation: Rivera-Mendoza D, Martínez-Flores I, Santamaría RI, Lozano L, Bustamante VH and Pérez-Morales D (2020) Genomic Analysis Reveals the Genetic Determinants Associated With Antibiotic Resistance in the Zoonotic Pathogen Campylobacter spp. Distributed Globally. Front. Microbiol. 11:513070. doi: 10.3389/fmicb.2020.513070
Received: 19 November 2019; Accepted: 21 August 2020;
Published: 11 September 2020.
Edited by:
Octavio Luiz Franco, Catholic University of Brasilia (UCB), BrazilReviewed by:
Jonathan Gray Frye, US National Poultry Research Center (USDA-ARS), United StatesMónica Oleastro, Instituto Nacional de Saúde Doutor Ricardo Jorge (INSA), Portugal
Copyright © 2020 Rivera-Mendoza, Martínez-Flores, Santamaría, Lozano, Bustamante and Pérez-Morales. This is an open-access article distributed under the terms of the Creative Commons Attribution License (CC BY). The use, distribution or reproduction in other forums is permitted, provided the original author(s) and the copyright owner(s) are credited and that the original publication in this journal is cited, in accordance with accepted academic practice. No use, distribution or reproduction is permitted which does not comply with these terms.
*Correspondence: Víctor H. Bustamante, dmljdG9yQGlidC51bmFtLm14; Deyanira Pérez-Morales, ZGV5YXBlbW9AZ21haWwuY29t
†These authors have contributed equally to this work