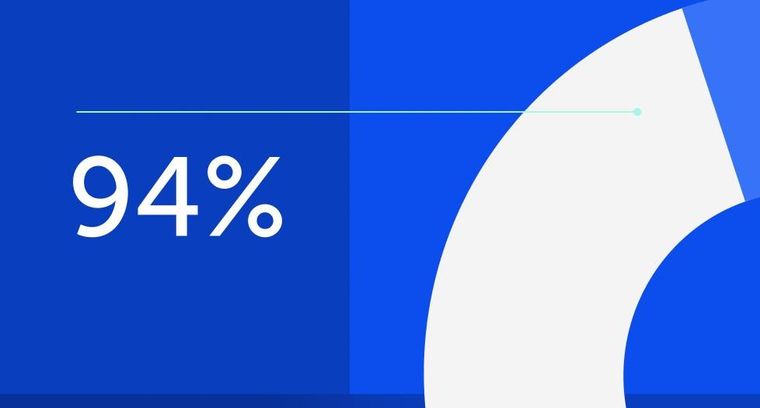
94% of researchers rate our articles as excellent or good
Learn more about the work of our research integrity team to safeguard the quality of each article we publish.
Find out more
ORIGINAL RESEARCH article
Front. Microbiol., 02 October 2020
Sec. Microbiotechnology
Volume 11 - 2020 | https://doi.org/10.3389/fmicb.2020.02019
This article is part of the Research TopicSalt Tolerant Rhizobacteria: For Better Productivity And Remediation Of Saline SoilsView all 14 articles
Soil salinity has emerged as a major obstacle to meet world food demands. Halo-tolerant plant growth promoting rhizobacteria (PGPR) are potential bioinoculants to enhance crop productivity in saline agriculture. Current work was aimed at studying individual or synergetic impact of salt tolerant PGPR on wheat growth and yield under saline conditions. A pot experiment was conducted on two wheat genotypes (Aas-11; salt tolerant and Galaxy-13; salt sensitive) inoculated with Pseudomonas fluorescence, Bacillus pumilus, and Exiguobacterium aurantiacum alone and in consortium. The salt tolerant variety (Aas-11) exhibited maximum root fresh (665.2%) and dry biomass (865%), free proline (138.12%) and total soluble proteins (155.9%) contents, CAT (41.7%) activity and shoot potassium uptake (81.08%) upon inoculation with B. pumilus, while improved shoot dry weight (70.39%), water (23.49%) and osmotic (29.65%) potential, POD (60.51%) activity, enhanced root potassium (286.36%) and shoot calcium (400%) were manifested by E. aurantiacum. Highest shoot length (14.38%), fresh weight (72.73%), potassium (29.7%) and calcium (400%) acquisition as well as glycinebetaine (270.31%) content were found in plants treated with PGPR consortium. On the other hand, in the salt sensitive variety (Galaxy-13), P. fluorescens treated plants showed significantly improved leaf-water relations, glycinebetaine (10.78%) content, shoot potassium (23.07%), root calcium (50%) uptake, and yield parameters, respectively. Plant root length (71.72%) and potassium content (113.39%), root and shoot fresh and dry biomass, turgor potential (231.02%) and free proline (317.2%) content were maximum upon PGPR inoculation in consortium. Overall, Aas-11 (salt tolerant variety) showed significantly better performance than Galaxy-13 (salt sensitive variety). This study recommends B. pumilus and E. aurantiacum for the salt tolerant (Aas-11) and P. fluorescens for the salt sensitive (Galaxy-13) varieties, as potential bioinoculants to augment their growth and yield through modulation of morpho-physiological and biochemical attributes under saline conditions.
Climate change, a hot topic of the current era, has affected planet earth in different ways and a rapid increase in saline landscapes is one of them that ultimately leads to global food insecurity and reduced agricultural productivity (Bharti et al., 2016). Around the globe, 20% of irrigated land is severely damaged by salt accumulation (Selvakumar et al., 2014). This land deterioration is expected to reach up to 50% by the year 2050 (Hossain, 2019). Almost 70% yield loss has been reported among cereal crops including wheat, rice, maize, and barley due to soil contamination by salinity and sodicity (Rajendran et al., 2009; Hussain et al., 2019).
So far, reclamation of such soils is being done by utilizing a variety of inorganic (gypsum, limestone, sulfuric acid and derivatives of sulfur, synthetic fertilizers), and organic (green and farm yard manure, industrial waste like press mud) measures (Qayyum et al., 2016). Similarly, Plant breeders and biotechnologists are in a constant struggle for the development of salt tolerant crop varieties either through natural selection, QTL mapping, marker assisted selection or by genetic manipulation via introduction of salt tolerant genes obtained from other organisms (Qadir et al., 2017). However, at field level, due to multiple factors, satisfactory outcomes have not been observed by such biological means for stress tolerance enhancement among the agro-economical significant crops (Khare et al., 2018).
Recently, exploitation of root adhering plant growth promoting rhizobacteria (PGPR) inhabiting hyper- saline conditions has gained attention as an alternative eco-friendly biological approach to get better crop productivity from salt deteriorating lands (Talaat, 2015). Improvement in plant growth aided by these microbes is well documented (Barnawal et al., 2012; Bharti et al., 2014). These halophilic/halotolerant plant growth-promoting rhizobacteria employ their key mechanisms by colonizing the plant rhizosphere to combat brutal environmental stresses and subsequent ruinous yield penalties. Strategies adopted by these microbes include de novo synthesis of osmolytes for cellular osmotic adjustment, regulation of ionic transporters and maintenance of homeostasis to reduce toxic effects of Na+ and Cl– ions, activation of reactive oxygen species scavenging defense system of plants to cope with deleterious effects of oxidative stress, respectively (Munns and Tester, 2008). Moreover, these microbes synthesize phytohormones, ACC deaminase, biological nitrogen fixation, siderophores, exopolysaccharides, volatile compounds and antifungal or antibacterial metabolites, mobilization of mineral ions, enhancement of photosynthesis, osmotic adjustment through accumulation of osmotically active metabolites like amino acids, sugars, polyols and betaines and detoxification of reactive oxygen species by antioxidants (Talaat and Shawky, 2013; Shabani and Sabzalian, 2016). Hence, these tiny creatures, by using different direct and indirect mechanisms, support plants to combat many biotic and abiotic challenges (Talaat, 2015; Subramanian et al., 2016).
By simple definition, salinity is a form of chemical (abiotic) factor that causes accumulation of soluble salts in the rhizospheric system. This condition adversely affects plant metabolism in two ways. Initially, high concentration of salts induces hyperosmotic and hyperionic situations which damage root architecture consequently leading to impaired water and nutritional acquisition. This eventually triggers secondary stress, i.e., oxidative stress, ultimately resulting in denaturation of DNA and proteins, and membrane instability due to lipid peroxidation. All these phenomena lead to programmed cell death and the collapse of the entire plant (Meloni et al., 2003; Kim et al., 2014).
Wheat (Triticum aestivum L.) is a staple food for 35% of the human population (Agriculture statistics of Pakistan 2017–19). Different plant species have acquired various ranges of stress tolerance. Some wheat varieties, for example, can sustain up to 10 dS/cm salinity level with minor yield losses and fall under the salt tolerant category (Munns et al., 2006). However, there is an immense need to enhance crop productivity up to 57% to meet ever increasing food demands by the year 2050 in parallel with continuous 1 to 2% land loss caused by salinity per year (Hossain, 2019).
It was hypothesized that PGPR residing in hyper-saline ecological conditions have the potential to modulate plant physiology by induced systemic tolerance to promote growth in salt degraded lands. Therefore, the present study was designed to evaluate the role of halotolerant PGP microbes by using two commonly used strategies (single strains and consortium) on two contrasting genotypes of wheat plant (salt tolerant and salt susceptible) and parameters used to investigate their role in plant growth and yield improvement include morpho-physiological and biochemical characteristics. This study will be helpful to explore the potential of native salt tolerant strains of PGPR and further their utilization as biofertilizer for wheat crop to minimize yield losses due to salt stress. In the future, this could lead to developing an effective bioformulation for such problematic soils.
This experimental work was conducted in the greenhouse, located at University of Agriculture, Faisalabad, Pakistan during wheat growing season November 2015–April 2016. Seeds of wheat (salt tolerant variety; Aas-11 and salt susceptible variety; Galaxy-13) were obtained from Ayub Agriculture Research Institute, Faisalabad, Pakistan. Three pre-characterized halotolerant PGPR strains, Pseudomonas fluorescens (Accession # KX644132), Bacillus pumilus (Accession # KX580768) and Exiguobacterium aurantiacum (Accession # KX580769) were collected from NBRC, Microbial Physiology Lab, SEBD, NIBGE, Faisalabad. The salt tolerance profiling on the basis of minimal inhibitory concentration and PGP characteristics (phosphate solubilization, IAA production and ACC metabolism) of these strains were assessed (qualitatively and quantitatively) based on selective media in a previous study (Ullah, 2019). Ullah and Bano (2019) demonstrated that these PGPR have significantly improved the growth and yield of maize grown in saline sodic soil as well physico-chemical properties of soil. Compatibility of these strains was also assessed using a cross streak method (Semenov et al., 2007) on NaCl supplemented LB-medium prior to seed inoculation. Pure saline soil used for the experiment was brought from field area of Biosaline Research Station, Pakka Anna, Faisalabad, Pakistan. The soil contained ECe: 13.41, pH: 9.1, organic matter: 1.39%, available nitrogen: 1.4 mg kg–1, available phosphorus: 19.6 mg kg–1, extractable potassium: 2.1 mg kg–1, sodium: 55 mg kg–1, chloride: 999.96 mg kg–1 and soil texture was clay loam.
Seeds were surface sterilized with 10% sodium hypochlorite solution and subsequent washing with autoclaved distilled water prior to inoculation with PGPR (Cheng et al., 1997). Inocula were prepared by transferring an 24 h old bacterial colony into Luria Bertani broth, kept on shaker at 120 rpm overnight at 28°C, centrifuged to get pellets, re-suspended in distilled water and optical density (at 660 nm) was adjusted to be 1, which was equal to 10–6 cells/ml. Then, surface sterilized wheat seeds were soaked in bacterial inocula containing single PGPR strains, and the consortium of three bacterial cultures for 2 h, while for control treatment seeds were soaked in autoclaved distilled water, respectively. Seeds were sown in plastic pots each containing 8.5 kg sterilized soil. Sowing was done at the rate of 20 seeds pot–1 at the depth of 1.5 inch. Thinning was done at the two-leaf stage up to 10 plants per pot–1. Irrigation was carried out with tap water (pH 7) following sufficient intervals. The experiment was based on completely randomized factorial design comprised of five treatments for each variety with five replications (Table 1). Data was recorded 70 days after sowing at vegetative stage to evaluate the impact of PGPR on growth and physiochemical attributes. Yield data was collected at crop maturity level.
Data regarding root and shoot length of randomly selected plants was recorded right after sampling by using a field meter rod. Fresh weight of root and shoot was measured by electric balance. Dry biomass of 7 days oven dried samples at 115°C was recorded using the same electric balance.
Leaf-water potential was measured according to the method of Scholander et al. (1964). For that purpose, a fully expanded young leaf was excised from each plant. At dawn leaf water potential was measured by using Scholander type pressure chamber (Arimad-2-Japan).
The same leaves were used for osmotic potential determination and stored in a freezer at −20°C for at least 7 days. After 7 days, the sap was extracted by pressing them with glass rod. The sap was placed on an osmometer [Wescor Vapor Pressure Osmometer (Model VAPRO 5520, El Cajon, CA, United States)] for the measurement of solute potential (Ball and Oosterhuis, 2005).
Turgor potential was calculated as the difference between water potential and osmotic potential by following the equation as cited by Nobel (1999).
The soluble proteins of the samples were determined by the Bradford method (Bradford, 1976). To extract protein, 0.25 g fresh leaves were grinded using a tissue grinder in 5 ml of 50 mM cooled phosphate buffer (pH 7.8) placed in an ice bath. The homogenate was centrifuged at 15,000 rpm for 15 min at 4°C. The supernatant was used for protein determination. Each sample, 100 μl, was taken in an Eppendorf tube and mixed with 1.0 ml of Bradford reagent. These sample solutions were incubated at 37°C for 10–15 min along with the blank and absorbance was noted at 595 nm using a spectrophotometer (IRMECO U2020).
Enzymatic antioxidants of wheat plants were extracted by grinding 0.25 g of fresh leaf material in 5 ml of 50 mM cooled potassium phosphate buffer (pH 7.8). This homogenized material was centrifuged at 10000 × g for 22 min at 4°C. The pellet was discarded and the supernatant was used for the estimation of activities of different antioxidants enzymes.
The activity of superoxide dismutase (SOD) was determined by monitoring its potential to cause inhibition in the photo reduction of nitro blue tetrazolium chloride (NBT) by following the procedure given by Giannoplitis and Ries (1977). The reaction mixture (3 ml of 50 mM potassium phosphate buffer (pH 7.8), 75 mM MEDTA, 13 mM methionine, 50 μM NBT, riboflavin, 1.3 μM) and 50 μl enzyme extract for the detection of enzyme activity. The tubes with reaction mixture lacking enzyme extract were used as control. Then these tubes were placed under fluorescent lamp (30 W) for 10 min, lamp was turned off and absorbance of mixture was recorded at 560 nm using a spectrophotometer (IRMECO U2020). One unit of enzyme was taken as the amount of enzyme used to cause 50% inhibition in the photochemical reduction of NBT.
The activities of catalase (CAT) and peroxidase (POD) were evaluated according to the procedure given by Chance and Maehly (1955) with some minor modifications. The reaction mixture (3 ml) for CAT contains 5.9 mM H2O2, 50 mM potassium phosphate buffer (pH 7.8). To initiate the reaction 0.1 ml of the enzyme extract was added the above prepared mixture. The decrease in absorbance was read at 240 nm at every 20 s interval. One unit of CAT was taken as absorbance change of 0.01 units per min. The POD reaction mixture (3 ml) contained 40 mM H2O2, 20 mM guaiacol, 50 mM potassium phosphate buffer (pH 7.8) and 100 μl of enzyme extract. Then the change in absorbance at 470 nm was monitored after every 20 s. One unit of POD activity was defined as the change of 0.01 absorbance unit per min per mg of protein.
Free proline content was determined by using the protocol described by Bates et al. (1973). The third leaf from the top (0.25 g) was homogenized in 5 ml of 3% aqueous sulfosalicylic acid and homogenate was filtered through Whatman No. 2 filter paper. One ml of filtrate was taken and mixed with 1 ml of acid ninhydrin (1.25 g ninhydrin in 30 ml glacial acetic acid) and 1 ml of glacial acetic acid in a test tube. The mixture was vortexed shortly and heated at 100°C in a water bath for 1 h and then the reaction was terminated in the ice bath. 2 ml of toluene was added to the solution and vortexed for 15–20 s while it cooled. The chromophore containing proline was extracted from the aqueous phase in a test tube and warmed to laboratory temperature. The absorbance was taken at 520 nm using spectrophotometer (U2020 IRMECO).
Grieve and Grattan (1983) method was followed for determination of glycine betaine content in leaf tissues. Briefly, 0.25 g dry material was homogenized with 5 ml of 0.5% toluene solution. Extract was centrifuged. 1 ml extract and 1 ml of 2N H2SO4 was mixed and 0.5 ml of this extract was taken in a separate test tube. 200 μL potassium tri-iodide was added in this extract and the test tube left in ice for 90 min. 2.8 ml distilled water was added then followed by addition of 6 ml 1,2-Dichloroethane. Upper layer was discarded and red lower layer was taken for reading at 365 nm.
To carry out plant mineral analysis, (which includes plant material digestion and mineral content determination) a method described by Allen et al. (1985) was followed, i.e., the dried (0.1 g) plant material was grinded well and placed in the 50ml flasks containingH2SO4. The mixture was boiled on a hot plate under a fume hood until digestion was completed which was indicated by the presence of white fumes in the flasks. Upon cooling, 50 ml distilled water was added and mixture was filtered by using Whatman paper # 42. Filtrate was further used for the determination of mineral nutrients.
Root and shoot sodium (Na+) potassium (K+) and calcium (Ca2+) were determined by using a flame photometer (Jenway, PFP-7, United Kingdom).
At maturity, crop was harvested and data regarding spikes length per plant, number of spikelets per spike and 100 grains weight was recorded.
The data was analyzed using Statistix version. 8.1. An ANOVA (two-way) was performed to analyze the effect of treatments and errors associated with the experiment. Further, LSD (p = 0.05) test was used to identify significant difference among treatments means.
Effects of different treatments (single strains and consortium) on root length were recorded on both wheat varieties. However, the salt tolerant variety; Aas-11, showed substantial increase compared to the salt sensitive variety; Galaxy-13. Seeds of variety Aas-11, bio-primed with P. fluorescens exhibited significant increase (28.59%) in root length followed by B. pumilus (26.22%) as compared to un-inoculated control plants. Plants inoculated with E. aurantiacum (T4) and consortium of PGPR (T5) expressed a non-significant increase in root length compared to untreated control plants. On the other hand, in variety Galaxy-13, all treatments exhibited significant increase except T2. The highest increase was recorded in T5 (71.72%) followed by T3 (67.35%) and T4 (39.33%), respectively (Figure 1A).
Figure 1. Effect of salt tolerant PGPR on growth attributes of two contrasting wheat varieties under saline condition. (A) Root length. (B) Shoot length. (C) Root fresh weight. (D) Shoot fresh weight. (E) Root dry weight. (F) Shoot dry weight.
Shoot length was significantly increased upon inoculation with PGPR in variety Aas-11; consortium of PGPR (T5) represented maximum (14.36%) impact followed by T3 = 13.94%, T2 = 11.96% and T4 = 10.44%. In contrast to it, variety Galaxy-13, experienced variable effects on length of plant shoot like T4 (5.72%) followed by T2 (2.90%) exhibited maximum increase but T5 (consortium of PGPR) and T3 (B. pumilus) showed reduced shoot length than control pants (Figure 1B).
Root fresh and dry weights were also significantly increased among all treatments in the salt tolerant variety where T3 (0.704 and 0.386 g) showed highest increase followed by T5 (0.584 and 0.342 g), T4 (0.54 and 0.334 g) and T2 (0.244 and 0.068 g), respectively, as compared to un-inoculated control plants (T1 = 0.092 and 0.04 g). However, in Galaxy-13, only the combined application of PGPR manifested a significant increase in root fresh (30.72%) and dry (66.66%) weight than T1 (control). All other treatments showed decreased values ranging between (6 to 15%) except T3 (B. pumilus) where a slight increase was observed in root fresh weight. On the other hand, root dry weight was decreased in following manner T2 > T4 > T3, in contrast with control plants (Figures 1C,E).
Just like root fresh weight, PGPR inoculation imposed a significant positive impact on shoot fresh weight in Aas-11. T5 (72.73%) expressed highest values for shoot fresh weight followed by T3 (B. pumilus = 61.84%) > T4 (E. aurantiacum = 52.96%) > T2. (P. fluorescens = 49.39%) In the case of Galaxy-13, none of the treatments showed a significant increase but T5 (consortium of PGPR = 10.36%) showed maximum increase followed by T2 (2.83%) and T4 (1.80%) as compared to untreated control plants while T3 (19.95% decrease) showed the least value (Figure 1D).
Shoot dry weight was significantly increased among all PGPR treated plants such as T4 (E. aurantiacum = 70.39%) followed by T5 (PGPR consortium = 69.83%), T3 (B. pumilus = 65.92%) and T4 = 54.74% in salt tolerant variety (Aas-11) but in case of salt sensitive variety, this improvement was observed in T5 (6.86%) followed by T2 (2.61%) then control. However, T4 (E. aurantiacum) treated plants exhibited reduced value for shoot dry weight. B. pumilus (T3) inoculated plants showed significant reduction in value (Figure 1F). The variations in dry and fresh weight of different treatments depend on many physiological and environmental conditions.
Water and osmotic potential were significantly improved in the salt tolerant variety (Aas-11). Maximum improvement was observed in plants inoculated with E. aurantiacum (T4 = 29.43% decrease). Other treatments represented the improved trend (19.52 to 10.36%) as follows; T3 > T5 and T2 in contrast with un-treated control plants. However, turgor potential was significantly lower among plants which received P. fluorescens (T2) as inoculation with gradual increase in T5, T3, and T4 as compared with un-inoculated plants. In the case of the salt sensitive variety (Galaxy-13), water and osmotic potential of PGPR treated plants were significantly improved except T5 (6.84%) which showed non-significant improvement in osmotic potential. T2 (P. fluorescens) treated plants exhibited the highest (22.09%) improved water potential followed by T4 (20.82%), T3 (18.925%), and T5 (13.85%) compared to control. whereas osmotic potential was highly improved in T2 followed by T3 (B. pumilus) and T4 (E. aurantiacum), respectively. Turgor potential was maximum in T5 (231.01%) followed by T4 while T3 (141 to 43%) results were at par with the control value. However, T2 showed a slight decrease compared to control (Table 2).
Table 2. Mean values of physiological attributes of two contrasting wheat genotypes inoculated with salt tolerant PGPR under saline condition.
Information about the effects of PGPR application on different biochemical attributes is presented in Figure 2. PGPR application caused a significant impact on the accumulation of free proline, glycine betaine and total soluble contents in the salt tolerant variety, i.e., Aas-11. However, effect of E. aurantiacum (T4) on glycine betaine accumulation remained non-significant. Free proline content was observed to be maximum among B. pumilus (T3 = 73.78 μmol g–1) treated plants followed by E. aurantiacum (T4 = 62.29 μmol g–1), P. fluorescens (T2 = 40.25 μmol g–1) and PGPR consortium (T5 = 32.85 μmol g–1), respectively (Figure 2A). The highest increase in glycine betaine content was recorded in T5 (55.21 μmol g–1) plants followed by T3 (52.06 μmol g–1) and T2 (49.48 μmol g–1) compared to the non-treated control (44.66 μmol g–1) plants (Figure 2B). Moreover, the total soluble proteins were increased in T3 (3.39 unit/mg protein) followed by T4 (3.37 unit/mg protein), T5 (3.27 unit/mg protein) and T2 (2.89 unit/mg protein) as compared to un-inoculated control (1.32 unit/mg protein) plants (Figure 2C). Examining the salt sensitive variety, i.e., Galaxy-13, free proline content was significantly enhanced in all treatments such as T5, T3, and T4 (63.81–48.89 μmol g–1) but T2 treated plants represented a decrease in its level. However, it differed non-significantly to the control plants. While the accumulation of glycine betaine was significantly high in T5 (63.81 μmol g–1) followed by T3, T2, and T4 which ranged between 52.06 and 45.9 μmol g–1, respectively (Figure 2B). However, only B. pumilus (T3 = 3.68 unit/mg protein) manipulated a significant increase in total soluble proteins content in contrast with un-treated control plants while the rest of the treatments showed a non-significant increase (10.69 to 0.78%) in their content which is as follow, T5 > T4 and T2 (Figure 2C).
Figure 2. Effect of salt tolerant PGPR on accumulation of osmotically active metabolites of two contrasting wheat varieties under saline conditions. (A) Free proline content. (B) Glycine betaine content. (C) Total soluble proteins content.
All treatments imposed their substantial impact on activities of antioxidant enzymes in the salt tolerant variety (Figure 3). Significant reduction in activity of superoxide dismutase was observed among all treatments (Figure 3A). The least activity was recorded in T3 (B. pumilus = 3.744 unit/mg protein) with gradual increase in T4 (E. aurantiacum = 3.834 unit/mg protein) < T5 (Consortium = 4.958 unit/mg protein) < T2 (P. fluorescens = 5.070 unit/mg protein). A variable effect on the activity of peroxidase was observed among all PGPR treated plants. (Figure 3B) i.e., least activity was observed in T4 (0.834 unit/mg protein) with gradual increase among T3 < T2 (1.314 and 1.826 unit/mg protein) which is lower than control (T1 = 2.113 unit/mg protein) plants. However, peroxidase activity in T5 (PGPR consortium = 2.574 unit/mg protein) inoculated plants, significantly higher than un-inoculated control. Catalase activity was non-significantly increased in T3 > T5 > T2. However, plants treated with E. aurantiacum (T4 = 3.799 unit/mg protein) expressed reduced activity in comparison with un-inoculated control plants (Figure 3C).
Figure 3. Effect of salt tolerant PGPR on the activity of enzymatic antioxidants of two contrasting wheat varieties under saline conditions. (A) Superoxide dismutase activity. (B) Peroxidase activity. (C) Catalase activity.
On the other hand, in the case of the salt sensitive variety (Galaxy-13), a significant decrease in superoxide dismutase activity was recorded in B. pumilus (T3 = 2.695 unit/mg protein) with a gradual increase in P. fluorescens (T2 = 2.79 unit/mg protein) while no change was observed in E. aurantiacum (T4 = 4.42 unit/mg protein) treated plants (Figure 3A). Enhanced activity was found in only T5 (PGPR consortium = 6.040 unit/mg protein) treated plants. Peroxidase activity was significantly lower in T4 (1.242 unit/mg protein) treated with gradual increase in T5 (1.609 unit/mg protein) plant leaves which differed significantly with other treatments. Non-significant reduction in peroxidase enzyme activity was observed among T2 > T3 as compared with control plants (Figure 3B). Catalase activity was significantly enhanced among E. aurantiacum inoculated plants T4 = 7.262 unit/mg protein followed by T2 = 6.24 unit/mg protein treated plants while T3 treated plants exhibited non-significant increase in its value. However, T5 plants, whose seeds were bioprimed with PGPR consortium, showed 6.86% reduced activity compared to control plants but the difference was not significant (Figure 3C).
Variable results on nutrient acquisition were recorded upon PGPR application among both varieties (Table 3). Sodium content in plant roots remained non-significant in all treatments of the salt tolerant variety (Aas-11). All treatments showed a slightly reduced sodium uptake compared to the control except the T5 (PGPR consortium) treated plant, which showed no change in its value. On the other hand, in the salt sensitive variety (Galaxy-13), a slight increase (11 and 29%) in its acquisition was observed in T2 (P. fluorescens) and T4 (E. aurantiacum) inoculated plants while the effect of rest of the treatments remained un-changed as compared to control plants.
Table 3. Mean values of mineral nutritional attributes of two contrasting wheat genotypes inoculated with salt tolerant PGPR under saline condition.
Shoot sodium acquisition was significantly increased among both varieties upon PGPR inoculation. Where T2 showed maximum sodium uptake followed by T5, T3, and T4. Similarly, significant results were recorded in Galaxy-13, where the maximum value was represented in T2 followed by T4 and T5 plants. However, T3 (B. pumilus) showed slight low (8.16%) sodium uptake compared to control.
In variety, Aas-11, maximum potassium content in root tissues was recorded in T4 (286.36%) followed by T3 > T5 > T2 (195.45–98.28%) treated plants. On the other hand, highest amount of potassium in plant shoots was found in T5 (29.72%) followed by T4 (21.62%) and T2 (16.21%) as compared with un-treated control plants. Collectively, root and shoot potassium uptake was significantly increased in the salt tolerant variety upon PGPR inoculation except T3 which exhibited only a slight decrease in shoot potassium content compared to the control plants. In the case of salt susceptible variety, T5 (PGPR consortium = 113.3%) followed by T2 (P. fluorescens) treated plants exhibit significant increase in root potassium content while this increase remained non-significant in T4 and T3 treated plants. Variable impact on potassium uptake by plant shoots was observed among all treatments where T2 (23.07%) followed by T5 (15.38%) showed a non-significant increase in value. While its content among E. aurantiacum inoculated plants remained similar to control, however, T3 exhibited slight reduced potassium value.
PGPR imposed non-significant impact on root calcium content in both varieties. PGPR consortium showed similar values to control but rest of treatments exhibited slight (12.5%) decrease in root calcium pool in salt tolerant variety. However, in the salt sensitive variety, maximum root calcium was recorded in T2 (50%) followed by T4 (33%) treated plants than control but the rest of the two treatments showed no significant change in its value as compared with un-treated control plants.
Calcium content in plant shoots was substantially increased among all treatments in Aas-11 where values for T5 and T4 were equal (400% increase) and followed by T3 and T2 (316 and 288%). On the other hand, in Galaxy-13, only T5 and T3 (37.78% increase) treated plants showed a significant increase in calcium content while T4 was at par with control plants. However, T2 showed a slight decrease in its value compared to the control.
Yield contributing components including spike length, number of spikelets per spike and 100 grains weight were substantially increased upon PGPR seed inoculation either as single strain or consortium (Table 4). Maximum values were recorded in plants treated with B. pumilus followed by PGPR consortium (T5) and E. aurantiacum (T4) in salt tolerant variety. However, impact of P. fluorescens inoculation was only evident in increasing 100 grains weight. Whereas in salt susceptible variety; Galaxy-13, P. fluorescens inoculation exhibited maximum values among all yield contributing components, followed by T5 and T4. While the effect of B. pumilus inoculation was at par with un-inoculated control plants.
Table 4. Mean values of yield attributes of two contrasting wheat genotypes inoculated with halophilic PGPR under saline condition.
Soil salinity is a prevalent environmental restraint to agriculture productivity and food security. Salt stress is responsible for 20–50% yield losses of important agricultural commodities including wheat, rice and maize around the world (Subiramani et al., 2020). So, there is a pressing need to adapt new sustainable approaches in addition to the use of organic or inorganic soil amendments along with salt resistant crop varieties to improve the productivity of such problematic soils (Egamberdieva et al., 2019). Exploitation of salt tolerant PGPR has recently emerged as an effective strategy to handle aforesaid situation (Grover et al., 2011). These halophilic and halotolerant microorganisms are capable of being sustained in hyper-saline habitats (Talaat, 2018). Main mechanisms responsible for their survival under stressful environment include de novo synthesis or uptake of osmoprotectants and specialized ion transport systems like Na+/H+ antiporters, respectively (Egamberdieva et al., 2019). Bacterial species belonging to genera Pseudomonas, Bacillus, Enterobacter, Agrobacterium, Streptomyces, Klebsiella, and Ochromobacter have been extensively reported as efficient bio-inoculants in saline agriculture (Sharma et al., 2016; Singh and Jha, 2016; Sarkar et al., 2018).
The main focus of this study was to investigate the potential impact of salt tolerant PGPR on wheat growth and yield enhancement through modulation of morpho-physiological and biochemical mechanisms. For this purpose, three PGPR strains were used. A strain of P. fluorescens was isolated from roots of maize plant grown in non-saline habitat. The other two microbes, B. pumilus and E. aurantiacum were isolated from roots of wheat, grown in Khewra salt range (Ullah, 2019). The salt tolerant potential of the latter two PGPR strains was higher than the first (Ullah, 2019).
Previous studies (Ansari et al., 2019; Xie et al., 2019) have demonstrated the significant contribution of several bacterial sp. belonging to Pseudomonas, Bacillus, and Exiguobacterium (Kasana and Pandey, 2018) genera in plant growth promotion under growth limiting conditions.
Safari et al. (2018) reported the enhanced salt tolerance index and substantial increase in germination percentage and seedling vigor in wheat upon inoculation with P. fluorescens under NaCl induced salinity. A similar response on barley growth and yield (spike length, fertility index and grains weight) parameters was described by Azadikhah et al. (2019) when treated with ACC deaminase producing P. fluorescens under salt stress. Nadeem et al. (2016) claimed that plant growth promotion via treatment with ACCD, IAA, and siderophore producing P. fluorescens is directly related to its better colonizing ability in plant rhizosphere. A number of studies on the potential of Bacillus sp. have also been documented in literature. Din et al. (2019) reported that bacterial strains belonging to Bacillus genus showed substantial role in salt stress alleviation in wheat due to their capability to produce EPS, ACCD and IAA production in vitro. Xie et al. (2019) and Ansari et al. (2019) documented that the supportive role in salt stress alleviation and wheat growth improvement revealed by B. pumilus inoculation was related to escalated levels of photosynthesis, transpiration and proline accumulation as well as reduced antioxidant levels. Bacillus strains resistant to salt stress contributed to improve K+ and Ca2+ acquisition and enhancement of protein and nitrogen content in rice seedlings grown under salt stress (Khattak et al., 2019). However, only limited data is available regarding the PGP activities showed by E. aurantiacum (Ullah and Bano, 2019). Strahsburger et al. (2018) reported the draft genome of E. aurantiacum strain PN47, data obtained from this study confirmed its adaptive features in hyper-osmotic and alkaline environment.
Our findings are concomitant with the previously reported literature that the application of salt tolerant PGPR strains P. fluorescens, B. pumilus, and E. aurantiacum substantially increased the growth and yield of wheat crop grown under saline conditions. It may be due to the fact that these PGPR were able to metabolize ACC deaminase, solubilize insoluble phosphate minerals and produce a significant quantity of IAA (Ullah and Bano, 2019). However, in our study, B. pumilus (T3) and E. aurantiacum (T4) showed promising results in the case of the salt tolerant variety; Aas-11 while the salt susceptible variety; Galaxy-13 performed more effectively upon inoculation with P. fluorescens (T2) and synergetic behavior of inoculated PGPR strains was quiet eminent too (T5). This variant response of inocula can be regarded as the PGPR colonization potential in rhizosphere varies with plant genotype, species, and developmental stage etc. (Delaplace et al., 2015; Poli et al., 2016; Wintermans et al., 2016) as well as physio-chemical characteristics of surrounding soil and nutrition competition among microbial communities. Basically, phytomicrobiome development is recruited by the plant itself which excretes various type of root exudates (Chaparro et al., 2012; Trabelsi and Mhamdi, 2013) and this interaction is regulated at biochemical and genetic level through signal transduction (Nelson and Sadowsky, 2015; Massalha et al., 2017; Smith et al., 2017).
In the current experiment, B. pumilus (characterized for in vitro PGP potential) inoculated plants (variety; Aas-11) showed maximum root fresh and dry weight, accumulation of free proline and total soluble proteins contents in leaf tissues along with reduced activity of enzymatic antioxidants activity. However, reduced activity of antioxidant is an evident feature of PGPR induced modulation of plant physiology that resulted in reduced ROS contents (Singh et al., 2016). E. aurantiacum showed the highest shoot dry weight and improved water and osmotic potential. Maximum Ion (K+ and Ca2+) acquisition by root tissues and decreased level of shoot sodium content suggest the efficacy of this PGPR strain in regulation of plant ion transporters to inhibit sodium uptake and promotion of potassium and calcium uptake by plant (Strahsburger et al., 2018). Combined application of PGPR (T5) exhibited an increase in shoot length, fresh weight, K+ and Ca2+ amount and glycine betaine content. Here, the synergetic role manipulated by PGPR was quite evident in the mitigation of ionic toxicity upon exposure to salt stress and resulted in increased growth and yield of wheat plants. A significant difference in shoot length and turgor potential was noted in P. fluorescens treated plants as compared to un-inoculated control plants.
On the other hand, if we look at salt sensitive variety; Galaxy-13, the effect of P. fluorescens on improved water relations, osmolyte (glycine betaine) accumulation, reduced activity of superoxide dismutase and elevated levels of shoot potassium and root calcium contents were recorded as compared to control plants. The implication of PGPR consortium (T5) showed improved root/shoot growth parameter, increased root potassium content and maximum amount of proline content accumulation. While shoot length and calcium content were maximum in E. aurantiacum treated plants in comparison with un-treated control plants. Whereas total soluble protein content was highest in B. pumilus inoculated plant tissues.
The above-mentioned outcomes revealed the supportive role of inoculated PGPR strains in growth and yield enhancement of wheat crop under salt stressed conditions. It also suggests that IAA producing bacteria accelerated the modulation of the plant’s morpho(growth parameters)-physiological (water relations) characteristics (Emami et al., 2019) and biochemical (osmolytes accumulation and reduced activity of enzymatic antioxidants) attributes. Moreover, inoculation assisted the plant to maintain nutrition balance via increased K+ and Ca2+ uptake and reduced sodium ion acquisition. Hence, PGPR improved the yield of wheat crop planted under stressful conditions.
These results are consistent with the previous reports which showed increased plant growth and yield upon inoculation with salt tolerant PGPR under salt stress (Singh and Jha, 2016). Thus, PGPR strains, P. fluorescens, B. pumilus and E. aurantiacum can be regarded as promising microorganisms to formulate biofertilizer specific for saline soils to minimize wheat crop yield penalties caused by soil salinization. Many PGPR strains belonging to genera Pseudomonas, Ochrobactrum, Bacillus, Azospirillum, Azotobacter, Rhizobium, Stenotrophomonas, Serratia, and Enterobacteria (Maleki et al., 2011) have been extensively reported and exploited as effective candidates to be formulated as biofertilizers – green biotechnology, as an alternative sustainable approach in saline agriculture (Egamberdieva et al., 2019).
Outcomes of the present study inferred that PGPR employed beneficial impact on physio-chemical attributes of inoculated plants consequently leading toward alleviation of salinity induced damages through improved water relations, enhanced compatible solutes accumulation, stimulated potassium and calcium acquisition and reduced antioxidant enzymes activity. These alterations in cellular metabolism ultimately led to the improved growth and yield among both salt tolerant and susceptible varieties under salt stress. However, the salt tolerant variety showed far better growth and yield than the sensitive variety. B. pumilus and E. Aurantiacum single strains and consortium manifested a more evident impact on the salt resistant genotype while in the case of the salt sensitive genotype, P. fluorescens single strain and consortium played a pivotal role in growth and yield improvement. Further experimentation at multiple field locations and detailed investigation of molecular mechanisms in near future can lead toward application of these microbes as biofertilizer in salt affected soil for enhanced wheat crop production.
All datasets presented in this study are included in the article/Supplementary Material.
AN conducted the experiments and sampling. AN and MS designed the project. AN, MS, and A carried out data analysis and manuscript preparation. MS, A, FM, AI, MI, and MM reviewed and edited the manuscript. All authors contributed to the article and approved the submitted version.
Article processing charges will be paid by corresponding and contributing authors of this paper.
The authors declare that the research was conducted in the absence of any commercial or financial relationships that could be construed as a potential conflict of interest.
The reviewer MN declared a shared affiliation with the authors to the handling Editor at the time of review.
We acknowledge A from Quaid-i-Azam University, Islamabad, for providing bacterial strains, further Dr. Abdur Rasool Awan from NIAB for arrangement of saline soil from Biosaline Research Station, Pakka Anna, Faisalabad, Pakistan.
Allen, S., Dobrenz, A., Schonhorst, S., and Stoner, J. (1985). Heritability of NaCl tolerance in germinating alfalfa seeds 1. Agron. J. 77, 99–101. doi: 10.2134/agronj1985.00021962007700010023x
Ansari, F. A., Ahmad, I., and Pichtel, J. (2019). Growth stimulation and alleviation of salinity stress to wheat by the biofilm forming Bacillus pumilus strain FAB10. Appl. Soil Ecol. 143, 45–54. doi: 10.1016/j.apsoil.2019.05.023
Azadikhah, M., Jamali, F., Nooryazdan, H.-R., and Bayat, F. (2019). Growth promotion and yield enhancement of barley cultivars using ACC deaminase producing Pseudomonas fluorescens strains under salt stress. Span. J. Agric. Res. 17:e0801. doi: 10.5424/sjar/2019171-13828
Ball, R., and Oosterhuis, D. (2005). Measurement of root and leaf osmotic potential using the vapor-pressure osmometer. Environ. Exp. Bot. 53, 77–84. doi: 10.1016/j.envexpbot.2004.03.003
Barnawal, D., Bharti, N., Maji, D., Chanotiya, C. S., and Kalra, A. (2012). 1-Aminocyclopropane-1-carboxylic acid (ACC) deaminase-containing rhizobacteria protect Ocimum sanctum plants during waterlogging stress via reduced ethylene generation. Plant Physiol. Biochem. 58, 227–235. doi: 10.1016/j.plaphy.2012.07.008
Bates, L. S., Waldren, R. P., and Teare, I. (1973). Rapid determination of free proline for water-stress studies. Plant Soil 39, 205–207. doi: 10.1007/bf00018060
Bharti, N., Barnawal, D., Awasthi, A., Yadav, A., and Kalra, A. (2014). Plant growth promoting rhizobacteria alleviate salinity induced negative effects on growth, oil content and physiological status in Mentha arvensis. Acta Physiol. Plant 36, 45–60. doi: 10.1007/s11738-013-1385-8
Bharti, N., Pandey, S. S., Barnawal, D., Patel, V. K., and Kalra, A. (2016). Plant growth promoting rhizobacteria Dietzia natronolimnaea modulates the expression of stress responsive genes providing protection of wheat from salinity stress. Sci. Rep. 6, 347–368.
Bradford, M. M. (1976). A rapid and sensitive method for the quantitation of microgram quantities of protein utilizing the principle of protein-dye binding. Ann. Biochem. 72, 248–254. doi: 10.1016/0003-2697(76)90527-3
Chaparro, J. M., Sheflin, A. M., Manter, D. K., and Vivanco, J. M. (2012). Manipulating the soil microbiome to increase soil health and plant fertility. Biol. Fertil. Soils 48, 489–499. doi: 10.1007/s00374-012-0691-4
Cheng, M., Fry, J. E., Pang, S., Zhou, S., Hironaka, C. M., Duncan, D. R., et al. (1997). Genetic transformation of wheat mediated by Agrobacterium tumefaciens. Plant Physiol. 115, 971–980.
Delaplace, P., Delory, B. M., Baudson, C., Mendaluk-Saunier De Cazenave, M., Spaepen, S., Varin, S., et al. (2015). Influence of rhizobacterial volatiles on the root system architecture and the production and allocation of biomass in the model grass Brachypodium distachyon (L.) P. Beauv. BMC Plant Biol. 15:195. doi: 10.1186/s12870-015-0585-3
Din, B. U., Sarfraz, S., Xia, Y., Kamran, M. A., Javed, M. T., Sultan, T., et al. (2019). Mechanistic elucidation of germination potential and growth of wheat inoculated with exopolysaccharide and ACC-deaminase producing Bacillus strains under induced salinity stress. Ecotoxicol. Environ. Saf. 183:109466. doi: 10.1016/j.ecoenv.2019.109466
Egamberdieva, D., Wirth, S., Bellingrath-Kimura, S. D., Mishra, J., and Arora, N. K. (2019). Salt-tolerant plant growth promoting rhizobacteria for enhancing crop productivity of saline soils. Front. Microbiol. 10:2791. doi: 10.3389/fmicb.2019.02791
Emami, T., Mirzaeiheydari, M., Maleki, A., and Bazgir, M. (2019). Effect of native growth promoting bacteria and commercial biofertilizers on growth and yield of wheat (Triticum aestivum) and barley (Hordeum vulgare) under salinity stress conditions. Cell. Mol. Biol. 65, 22–27. doi: 10.14715/cmb/2019.65.6.5
Giannoplitis, C. N., and Ries, S. K. (1977). Superoxide dismutase : 1. Occurrence in higher plants. Plant Physiol. 59, 309–314. doi: 10.1104/pp.59.2.309
Grieve, C., and Grattan, S. (1983). Rapid assay for determination of water soluble quaternary ammonium compounds. Plant Soil 70, 303–307. doi: 10.1007/bf02374789
Grover, M., Ali, S. Z., Sandhya, V., Rasul, A., and Venkateswarlu, B. (2011). Role of microorganisms in adaptation of agriculture crops to abiotic stresses. World J. Microb Biot. 27, 1231–1240. doi: 10.1007/s11274-010-0572-7
Hossain, S. (2019). Present scenario of global salt affected soils, its management and importance of salinity research. Int. J. Biol. Sci. 1, 1–13. doi: 10.1201/9780203739242-1
Hussain, S., Shaukat, M., Ashraf, M., Zhu, C., Jin, Q., and Zhang, J. (2019). Salinity Stress in Arid and Semi-Arid Climates: Effects and Management in Field Crops in Climate Change and Agriculture. London: Intech Open.
Kasana, R. C., and Pandey, C. (2018). Exiguobacterium: an overview of a versatile genus with potential in industry and agriculture. Critical 38, 141–156. doi: 10.1080/07388551.2017.1312273
Khare, E., Mishra, J., and Arora, N. K. (2018). Multifaceted interactions between endophytes and plant: developments and prospects. Front. Microbiol. 9:2732. doi: 10.3389/fmicb.2018.02732
Khattak, M. R., Jan, S. U., Malook, I., Khattak, S. R., Akhtar, N., Khan, S., et al. (2019). “Effects of halophilic bacteria on biochemical characteristics of rice ultivars under salinity stress conditions,” in Saline Soil-based Agriculture by Halotolerant Microorganisms, eds M. Kumar, H. Etesami, and V. Kumar (Cham: Springer), 161–174. doi: 10.1007/978-981-13-8335-9_7
Kim, K., Jang, Y. J., Lee, S. M., Oh, B. T., Chae, J. C., and Lee, K. J. (2014). Alleviation of salt stress by Enterobacter sp. EJ01 in tomato and Arabidopsis is accompanied by up-regulation of conserved salinity responsive factors in plants. Molecular 37:109. doi: 10.14348/molcells.2014.2239
Maleki, M., Mokhtarnejad, L., and Mostafaee, S. (2011). Screening of rhizobacteria for biological control of cucumber root and crown rot caused by Phytophthora drechsleri. Plant Pathol. J. 27, 78–84. doi: 10.5423/PPJ.2011.27.1.078
Massalha, H., Korenblum, E., Tholl, D., and Aharoni, A. (2017). Small molecules below-ground: the role of specialized metabolites in the rhizosphere. Plant J. 90, 788–807. doi: 10.1111/tpj.13543
Meloni, D. A., Oliva, M. A., Martinez, C. A., and Cambraia, J. (2003). Photosynthesis and activity of superoxide dismutase, peroxidase and glutathione reductase in cotton under salt stress. Environ. Exp. Bot. 49, 69–76. doi: 10.1016/s0098-8472(02)00058-8
Munns, R., and Tester, M. (2008). Mechanisms of salinity tolerance. Annu. Rev. Plant Biol. 59, 651–681.
Munns, R, James, R. A., and Läuchli, A. (2006). Approaches to increasing the salt tolerance of wheat and other cereals. J. Exp. Bot. 57, 1025–1043. doi: 10.1093/jxb/erj100
Nadeem, S. M., Ahmad, M., Naveed, M., Imran, M., Zahir, Z. A., and Crowley, D. E. (2016). Relationship between in vitro characterization and comparative efficacy of plant growth-promoting rhizobacteria for improving cucumber salt tolerance. Arch. Microbiol. 198, 379–387. doi: 10.1007/s00203-016-1197-5
Nelson, M. S., and Sadowsky, M. J. (2015). Secretion systems and signal exchange between nitrogen-fixing rhizobia and legumes. Front. Plant Sci. 6:491. doi: 10.3389/fpls.2015.00491
Nobel, P. S. (1999). Physiochemical & Environmental Plant Physiology. Cambridge, MA: Academic press.
Poli, A., Lazzari, A., Prigione, V., Voyron, S., Spadaro, D., and Varese, G. C. (2016). Influence of plant genotype on the cultivable fungi associated to tomato rhizosphere and roots in different soils. Fungal Biol. 120, 862–872. doi: 10.1016/j.funbio.2016.03.008
Qadir, M., Oster, J., Schubert, S., Noble, A., and Sahrawat, K. (2017). Phytoremediation of sodic and saline-sodic soils. Adv. Agron. 96, 197–247. doi: 10.1016/s0065-2113(07)96006-x
Qayyum, F., Saifullah, M. S., and Akhtar, J. (2016). “Salt-affected soils: sources genesis and management,” in Soil Science Concepts and Applications, eds M. Sabir, J. Akhtar, and K. R. Hakeem (Faisalabad: University of Agriculture Faisalabad).
Rajendran, K., Tester, M., and Roy, S. J. (2009). Quantifying the three main components of salinity tolerance in cereals. Plant Cell Environ. 32, 237–249. doi: 10.1111/j.1365-3040.2008.01916.x
Safari, D., Jamali, F., Nooryazdan, H. R., and Bayat, F. (2018). Evaluation of ACC deaminase producing’ Pseudomonas fluorescens strains for their effects on seed germination and early growth of wheat under salt stress. Aust. J. Crop Sci. 12, 413–421. doi: 10.21475/ajcs.18.12.03.pne801
Sarkar, A., Ghosh, P. K., Pramanik, K., Mitra, S., Soren, T., Pandey, S., et al. (2018). A halotolerant Enterobacter sp. displaying ACC deaminase activity promotes rice seedling growth under salt stress. Microbiol. Res. 169, 20–32. doi: 10.1016/j.resmic.2017.08.005
Scholander, P. F., Hammel, H., Hemmingsen, E., and Bradstreet, E. (1964). Hydrostatic pressure and osmotic potential in leaves of mangroves and some other plants. Proc. Natl. Acad. Sci. U.S.A. 52, 119–125. doi: 10.1073/pnas.52.1.119
Selvakumar, G., Kim, K., Hu, S., and Sa, T. (2014). “Effect of salinity on plants and the role of arbuscular mycorrhizal fungi and plant growth-promoting rhizobacteria in alleviation of salt stress,” in Physiological Mechanisms and Adaptation Strategies in Plants Under Changing Environment, eds P. Ahmad and M. R. Wani (Cham: Springer), 115–144. doi: 10.1007/978-1-4614-8591-9_6
Semenov, A., Sgibnev, A., Cherkasov, S., and Bukharin, O. (2007). Bacterial regulation of antagonistic activity of bacteria. Biol. Exp. Biol. Med. 144, 702–705. doi: 10.1007/s10517-007-0410-6
Shabani, L., and Sabzalian, M. R. (2016). Arbuscular mycorrhiza affects nickel translocation and expression of ABC transporter and metallothionein genes in Festuca arundinacea. Mycorrhiza 26, 67–76. doi: 10.1007/s00572-015-0647-2
Sharma, S., Kulkarni, J., and Jha, B. (2016). Halotolerant rhizobacteria promote growth and enhance salinity tolerance in peanut. Front. Microbiol. 7:1600. doi: 10.3389/fmicb.2016.0160
Singh, J. S., Koushal, S., Kumar, A., Vimal, S. R., and Gupta, V. K. (2016). Book review: microbial inoculants in sustainable agricultural productivity- Vol. II: functional application. Front. Microbiol. 7:2105. doi: 10.3389/fmicb.2016.02105
Singh, R. P., and Jha, P. N. (2016). The multifarious PGPR Serratia marcescens CDP-13 augments induced systemic resistance and enhanced salinity tolerance of wheat (Triticum aestivum L.). PLoS One 11:e0155026. doi: 10.1371/journal.pone.0155026
Smith, D. L., Gravel, V., and Yergeau, E. (2017). Editorial: signaling in the phytomicrobiome. Front. Plant Sci. 8:611. doi: 10.3389/fpls.2017.00611
Strahsburger, E., Zapata, F., Pedroso, I., Fuentes, D., Tapia, P., Ponce, R., et al. (2018). Draft genome sequence of Exiguobacterium aurantiacum strain PN47 isolate from saline ponds, known as “Salar del Huasco”, located in the Altiplano in the North of Chile. Braz. J. Microbiol. 49, 7–9. doi: 10.1016/j.bjm.2017.03.011
Subiramani, S., Ramalingam, S., Muthu, T., Nile, S. H., and Venkidasamy, B. (2020). “Development of abiotic stress tolerance in crops by plant growth-promoting rhizobacteria (PGPR),” in Phyto-Microbiome in Stress Regulation, eds M. Kumar, H. Etesami, and V. Kumar (Cham: Springer), 125–145. doi: 10.1007/978-981-15-2576-6_8
Subramanian, S., Souleimanov, A., and Smith, D. L. (2016). Proteomic studies on the effects of lipo-chitooligosaccharide and thuricin 17 under unstressed and salt stressed conditions in Arabidopsis thaliana. Front. Plant Sci. 7:1314. doi: 10.3389/fpls.2016.0131
Talaat, N. B. (2015). Effective microorganisms improve growth performance and modulate the ROS-scavenging system in common bean (Phaseolus vulgaris L.) plants exposed to salinity stress. J. Plant Growth Regul. 34, 35–46. doi: 10.1007/s00344-014-9440-2
Talaat, N. B. (2018). Exploring halotolerant rhizomicrobes as a pool of potent genes for engineering salt stress tolerance in crops. Sali. Responses Tolerance Plants 2, 49–76. doi: 10.1007/978-3-319-90318-7_3
Talaat, N. B., and Shawky, B. T. (2013). Modulation of nutrient acquisition and polyamine pool in salt-stressed wheat (Triticum aestivum L.) plants inoculated with arbuscular mycorrhizal fungi. Acta Physiol. Plant. 35, 2601–2610. doi: 10.1007/s11738-013-1295-9
Trabelsi, D., and Mhamdi, R. (2013). Microbial inoculants and their impact on soil microbial communities: a review. Biomed. Res. Int. 2013:863240.
Ullah, A. (2019). Role of PGPR in the Reclamation and Revegetation of Saline Land. Unpublished doctoral dissertation, Quaid-i-Azam University Islamabad, Pakistan.
Ullah, A., and Bano, A. (2019). Role of PGPR in the reclamation and revegetation of saline land. Pak. J. Bot. 51, 27–35.
Wintermans, P. C., Bakker, P. A., and Pieterse, C. M. (2016). Natural genetic variation in Arabidopsis for responsiveness to plant growth-promoting rhizobacteria. Plant Mol. Biol. 90, 623–634. doi: 10.1007/s11103-016-0442-2
Keywords: plant growth promoting rhizobacteria, salt tolerance, osmotically active compounds, salt stress, climate change
Citation: Nawaz A, Shahbaz M, Asadullah, Imran A, Marghoob MU, Imtiaz M and Mubeen F (2020) Potential of Salt Tolerant PGPR in Growth and Yield Augmentation of Wheat (Triticum aestivum L.) Under Saline Conditions. Front. Microbiol. 11:2019. doi: 10.3389/fmicb.2020.02019
Received: 31 March 2020; Accepted: 30 July 2020;
Published: 02 October 2020.
Edited by:
Dilfuza Egamberdieva, Leibniz Center for Agricultural Landscape Research (ZALF), GermanyReviewed by:
Muhammad Naveed, University of Agriculture, Faisalabad, PakistanCopyright © 2020 Nawaz, Shahbaz, Asadullah, Imran, Marghoob, Imtiaz and Mubeen. This is an open-access article distributed under the terms of the Creative Commons Attribution License (CC BY). The use, distribution or reproduction in other forums is permitted, provided the original author(s) and the copyright owner(s) are credited and that the original publication in this journal is cited, in accordance with accepted academic practice. No use, distribution or reproduction is permitted which does not comply with these terms.
*Correspondence: Aniqa Nawaz, c3VwZXJiX2FuaXFhQHlhaG9vLmNvbQ==; Fathia Mubeen, bXVmYXRoaWFAeWFob28uY29t
Disclaimer: All claims expressed in this article are solely those of the authors and do not necessarily represent those of their affiliated organizations, or those of the publisher, the editors and the reviewers. Any product that may be evaluated in this article or claim that may be made by its manufacturer is not guaranteed or endorsed by the publisher.
Research integrity at Frontiers
Learn more about the work of our research integrity team to safeguard the quality of each article we publish.