- 1Facultad de Ciencias Químicas, Universidad Autónoma de Nuevo León, UANL, San Nicolás de los Garza, Mexico
- 2Centro de Investigación en Biotecnología y Nanotecnología, Facultad de Ciencias Químicas, Parque de Investigación e Innovación Tecnológica, Universidad Autónoma de Nuevo León, Apodaca, Mexico
The inappropriate use of antibiotics and an inadequate control of infections have led to the emergence of resistant strains which represent a major threat to public health and the global economy. Therefore, research and development of a new generation of antimicrobials to mitigate the spread of antibiotic resistance has become imperative. Current research and technology developments have promoted the improvement of antimicrobial agents that can selectively interact with a target site (e.g., a gene or a cellular process) or a specific pathogen. Antimicrobial peptides and metal nanoparticles exemplify a novel approach to treat infectious diseases. Nonetheless, combinatorial treatments have been recently considered as an excellent platform to design and develop the next generation of antibacterial agents. The combination of different drugs offers many advantages over their use as individual chemical moieties; these include a reduction in dosage of the individual drugs, fewer side effects compared to the monotherapy, reduced risk for the development of drug resistance, a better combined response compared to the effect of the individual drugs (synergistic effects), wide-spectrum antibacterial action, and the ability to attack simultaneously multiple target sites, in many occasions leading to an increased antibacterial effect. The selection of the appropriate combinatorial treatment is critical for the successful treatment of infections. Therefore, the design of combinatorial treatments provides a pathway to develop antimicrobial therapeutics with broad-spectrum antibacterial action, bactericidal instead of bacteriostatic mechanisms of action, and better efficacy against multidrug-resistant bacteria.
Introduction
Development of antibacterial resistance is considered one of the leading public health problems, since it has a significant impact on the economy worldwide. Since therapeutic options to treat infections are increasingly being limited due to antibacterial resistance, this escalates the morbidity and mortality associated with infectious diseases caused by bacteria [World Health Organization (WHO), 2020]. ESKAPE pathogens are responsible for the majority of life-threatening nosocomial infections and are capable of “escaping” the biocidal action of antimicrobial agents (Pendleton et al., 2013). The term “ESKAPE” is an acronym for six bacterial pathogens associated with multidrug resistance: Enterococcus faecium (E. faecium), Staphylococcus aureus (S. aureus), Klebsiella pneumoniae (K. pneumoniae), Acinetobacter baumannii (A. baumannii), Pseudomonas aeruginosa (P. aeruginosa), and Enterobacter spp. (Mulani et al., 2019). Multidrug-resistant (MDR) bacteria are resistant to more than one antimicrobial drug, and extensively drug-resistant (XDR) bacteria are types of drug-resistant organisms that are resistant to all, or almost all, approved antimicrobial agents (Magiorakos et al., 2012). For these reasons, it is essential to design and engineer new promising classes of antibiotics (Gajdács, 2019).
The New Therapeutic Alternatives: Input From Recent Studies
As we described above, the development of antimicrobial resistance represents a major threat to public health, and this has been echoed by different health organizations around the globe. Antimicrobial peptides (AMPs) and nanoparticles (NPs) and the design of novel combinatorial therapies are among the new promising alternatives to fight infections caused by MDR- and XDR-resistant bacteria.
Antimicrobial Peptides
Antimicrobial peptides are a highly diverse family of small proteins with a varying number of amino acids; they have also been referred to as cationic host defense peptides (Boparai and Sharma, 2019). A variety of synthetic AMPs have been synthesized in the laboratories, but there are also a wide diversity of AMPs produced by bacteria and yeast, in addition to those found naturally in animals and plants (Wang, 2013). AMPs have demonstrated to participate in a variety of biological activities, including as antimicrobial antiviral, antifungal, and anti-mitogenic agents, in addition to their antitumor and anti-inflammatory properties and their ability to act as immune modulators. Therefore, AMPs represent a potential alternative to replace a wide variety of commonly used drugs. Moreover, most of the available studies demonstrate that AMPs exhibit therapeutic activity in in vitro and in vivo models (Divyashree et al., 2019).
The use of AMPs alone or in combination with conventional drugs has proven effective in combating different infectious agents, mainly MDR bacteria (Zharkova et al., 2019). AMPs are promising potential candidates to counteract multiresistant pathogens since they possess many advantages: they display potent microbicidal activity in the micromolar range (Aoki and Ueda, 2013), they have demonstrated a rapid bacterial death action (Lei et al., 2019), and they have low resistance selection (Mahlapuu et al., 2016). Their mechanism of antibacterial action is multifunctional because it alters the cell membrane (Li et al., 2017) and also attacks specific targets that take part in the development of different intracellular processes (Le et al., 2017), such as inhibition of transcription, translation, protein synthesis, and bacterial cell wall formation (Mwangi et al., 2019). These general mechanisms of action of AMPs are displayed in Figure 1A.
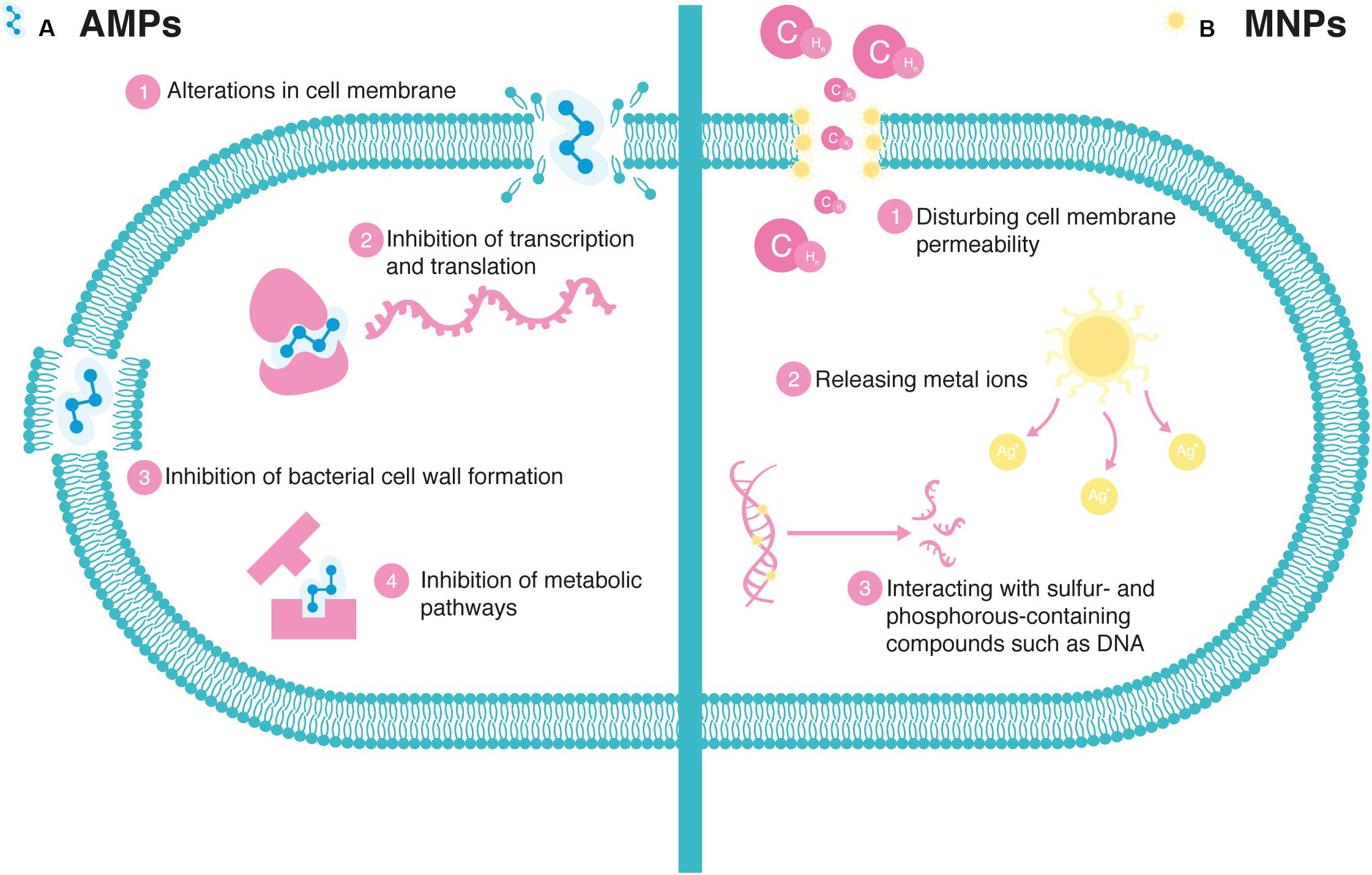
Figure 1. Mechanism of antimicrobial action in bacteria: (A) General mechanisms of action of antimicrobial peptides (AMPs). AMPs act through different mechanisms (Le et al., 2017) such as 1. Alteration in membrane integrity via electrostatic interaction with negatively charged cell membranes which kill cells, 2. Inhibition of DNA synthesis by (i) cross-linking with single- or double-stranded DNA, (ii) prevention of the DNA relaxation by inactivation of DNA topoisomerase I, and (iii) blocking of DNA replication by trapping the gyrase-DNA complex; and protein synthesis by (i) inhibition of protein translation by targeting the ribosomes, (ii) interrupting the protein-folding pathway, and (iii) rapid proteolytic activity causing the degradation of some DNA replication-associated proteins, leading to secondary inhibition of DNA synthesis, 3. Inhibition of bacterial cell wall formation by alteration of the alternating amino sugars in linear form that cross-link via peptide bridges to form the peptidoglycan layer, and 4. Inhibition of metabolic pathways by alteration of nucleic acid metabolism, including nucleotide transport and metabolism, nucleobase, nucleoside, and nucleotide interconversion. (B) Mechanisms for antimicrobial action of metal nanoparticles (MNPs). MNPs act via the following (Shaikh et al., 2019). 1. MNPs disturb cell membrane permeability by interfering with metabolic pathways and inducing changes in membrane shape and function. 2. When MNPs are in solution, metal ions are released in the environment surrounding. Metal ions generate reactive oxygen species (e.g., oxygen ions and hydroxyl radicals) and induce oxidative stress in bacteria. Oxidative stress is a key contributor in altering the bacterial membrane permeability and thus can damage cell membranes. Also, metal ions may cause cell structural changes and aberrant enzyme activities, which perturb normal physiological processes. 3. Interaction with sulfur- and phosphorous-containing compounds such as DNA, which prevent DNA from unwinding and transcription.
One AMP of particular interest is human cathelicidin peptide (LL-37), which has been reported to have wound-healing effects on the host in addition to exhibiting antimicrobial and anti-biofilm activity against a variety of Gram-positive and Gram-negative human pathogens (Duplantier and van Hoek, 2013). LL-37 and its derivatives are considered excellent candidates as antimicrobial therapeutic agents and have been the subject of many studies (Dürr et al., 2006; Kościuczuk et al., 2012; De Breij et al., 2018). Especially, in 2018, De Breij et al. synthesized an LL-37 derivative (SAAP-148), with potent antimicrobial activities, by replacing an amino acid from the terminal carbon of the LL-37 chain. This LL-37 derivative exhibited a minimum inhibitory concentration [MIC] between 0.4 and 12.8 μM against various ESKAPE pathogenic bacteria (e.g., E. faecium, S. aureus, K. pneumoniae, A. baumannii, P. aeruginosa, and Enterobacter species) without selection of resistance. Furthermore, this AMP derivative showed anti-biofilm activity against S. aureus, A. baumannii, and P. aeruginosa (De Breij et al., 2018).
Colistin is another important peptide antibiotic (produced Bacillus polymyxa var. colistinus) used as a last-resort drug to treat MDR infections (Oka and Ito, 2000). It has emerged as an important agent in the treatment of Gram-negative bacterial infections, especially those caused by MDR pathogens in hospitalized patients (Das et al., 2017). Notably, two new colistin-derived AMPs (AA139 and SET-M33), with a mechanism similar to colistin, are in development and have shown excellent therapeutic potential both in vitro against MDR bacteria and in in vivo infection models (van der Weide et al., 2019).
The main limiting factor for the systemic use of AMPs is their sensitivity to proteolytic digestion in different body fluids (e.g., intestinal mucosa, gastrointestinal tract, and blood plasma), which directly affect both their in vivo stability and their pharmacokinetic profile (Moncla et al., 2011; Starr and Wimley, 2017). Therefore, the search for new AMPs continues, particularly in a new class of peptides with high specificity and potency, known as “selectively targeted AMPs” (STAMPs), which show increased sensitivity to specific pathogens, demonstrating a significant increase in their bactericidal capacity without direct effects on the microbiota (Chung and Khanum, 2017). The STAMP technology requires two functionally independent peptide domains integrated through a small linker. One peptide domain serves as the killing AMP moiety and the other peptide domain consisting of a high-affinity binding peptide which functions as a targeting moiety (Aoki and Ueda, 2013). These properties increase the binding to the surface of a targeted pathogen by enhancing the local concentration of the AMP and thus lead to improve bactericidal efficiency (Sarma et al., 2018). In recent years, several new and promising STEMs have been developed against Streptococcus mutans (Huo et al., 2017), Pseudomonas aeruginosa, and Streptococcus mutants together (He et al., 2009), methicillin-resistant Staphylococcus aureus (Mao et al., 2013), Enterococcus faecalis (Xu et al., 2020), and clinical isolates (Pseudomonas aeruginosa; Eckert et al., 2006). Nonetheless, more preclinical and clinical research is needed in the development of targeted antimicrobial therapy.
Metal Nanoparticles
An additional alternative to fighting infections caused by antibiotic-resistant bacteria is the development of NPs since it has been amply reported that metal nanoparticles (MNPs) have antibacterial activity against ESKAPE pathogens (Wang et al., 2017; Lee et al., 2019). Some of the mechanisms of the antimicrobial mode of action of MNPs are summarized in Figure 1B. In the search for new antimicrobials to treat the ESKAPE pathogens, silver has been highlighted as a potential candidate to treat infectious diseases (Borthagaray et al., 2018). Silver nanoparticles (AgNPs) possess antimicrobial activity, and they act by disturbing cell membrane permeability, interacting with sulfur- and phosphorous-containing compounds including DNA, in addition to their ability to release silver ions, contributing to the antibacterial effect (Morones et al., 2005; Morones-Ramirez et al., 2013). Gold (Au) nanoparticles have also been reported as effective antibacterial agents for antibiotic-resistant bacterial strains such as S. aureus, E. faecium, Enterococcus faecalis (E. faecium), Escherichia coli (E. coli), Vibrio cholerae (V. cholerae), Salmonella typhimurium (S. typhimurium), and Salmonella dysenteriae (S. dysenteriae; Kumar et al., 2016).
Among metal oxide nanoparticles, zinc oxide (ZnO) nanoparticles have shown antimicrobial activity against both Gram-negative and Gram-positive bacteria, including Bacillus subtilis (B. subtilis), S. aureus, E. coli, P. aeruginosa, and A. baumannii (Guo et al., 2015; Tiwari et al., 2018). On the other hand, among photocatalytic nanoparticles, titanium dioxide (TiO2) NPs have been extensively studied due to their antimicrobial activity (Gelover et al., 2006). Several studies have reported the antimicrobial activity of TiO2 NPs against methicillin-resistant S. aureus and MDR E. coli (Jesline et al., 2015; Mantravadi, 2017; de Dicastillo et al., 2019).
Despite the advantages that nanoparticles offer, such as a broad therapeutic index, controlled drug release, less prone to bacterial resistance, and fewer side effects than chemical antimicrobials (Lee et al., 2019), to treat infections caused by the ESKAPE pathogens, there are still challenges remaining to be tackled such as improvement of physicochemical properties, better pharmacokinetic profiles, and comprehensive studies on long-term exposure to humans. In terms of design and application, there is a particular interest in the generation of nanohybrids combining different metals with different antimicrobial and sensitizer agents (Zhang et al., 2014; Wolfram et al., 2015). Metal nanoparticle-based compounds (alone or in combination with other antimicrobial agents) provide promising alternatives to combat the development of antibacterial resistance (Shaikh et al., 2019). Therefore, it is imperative to develop a comprehensive understanding of the mechanisms of action responsible for the bactericidal properties as well as the identification of the most promising antimicrobial agents for future clinical translation.
Combinatorial Treatments
The strategies to reduce antibiotic resistance include the limited use of antibiotics and the application of more effective antibacterial therapies. Because the time of exposure to antibiotics correlates with the development of resistance (Andersson et al., 2020), it is necessary to use drugs with a broad spectrum of action and pharmacokinetic properties that facilitate their rapid access to the target site (Krause et al., 2016). However, most of the available treatments do not have all these characteristics, so an alternative option is the use of combination therapies, which can lead to a synergistic and more effective response (Lehár et al., 2009). It has been shown that the combination of drugs leads to a considerably more potent effect, compared to the individual drug (Tamma et al., 2012; Marks et al., 2013). Figure 2 displays the disadvantages of using single drugs (Figure 2A) and the advantages of using combinatorial treatments (Figure 2B).
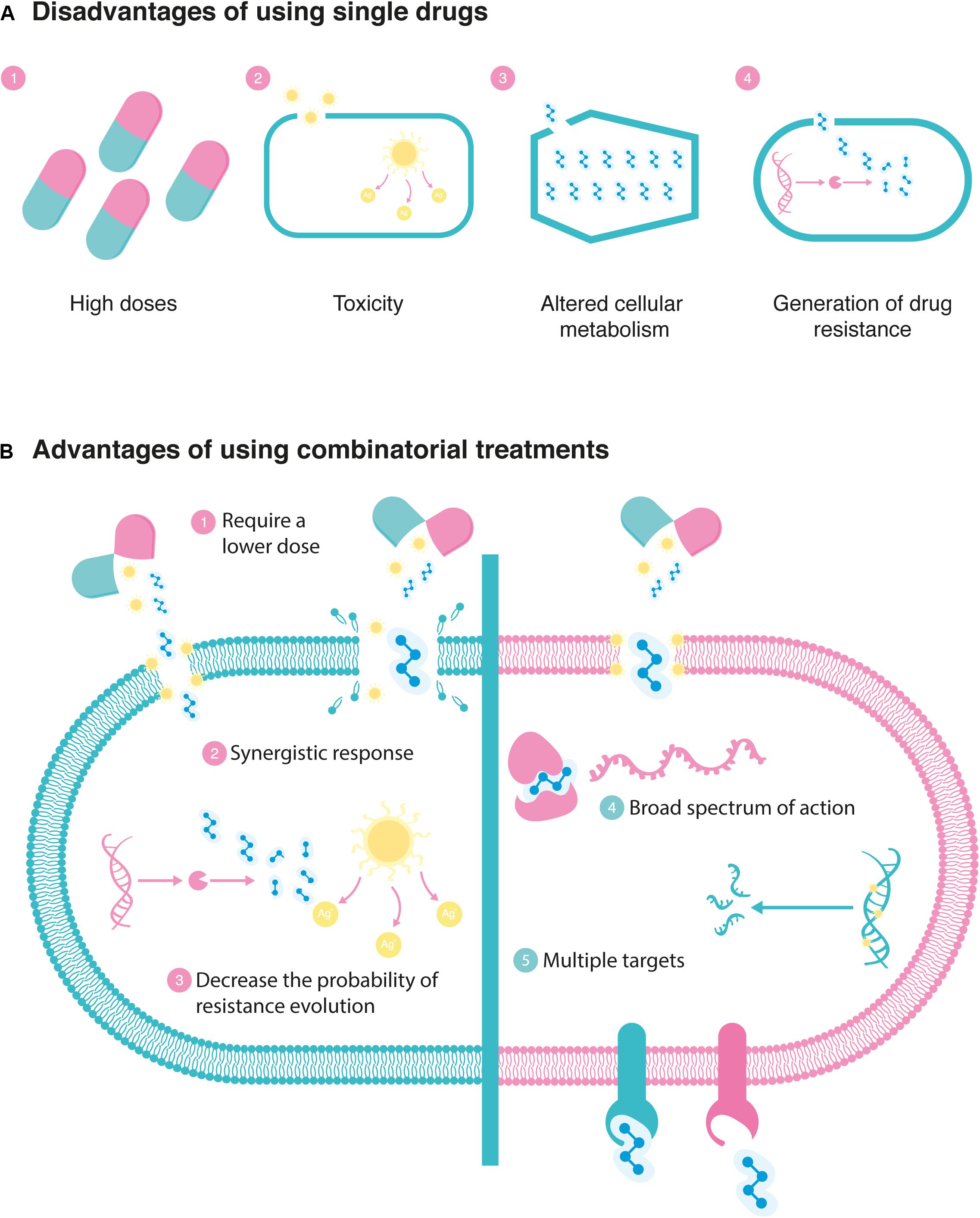
Figure 2. Antimicrobial treatment strategies: (A) disadvantages of using single drugs and (B) advantages of using combinatorial treatments. The advantage of using combinatorial treatments of synergistic drug pairs provides the opportunity to lower the dosage of the individual agents, thereby reducing toxicity while maintaining the wanted effect on bacteria. Moreover, a synergistic response can occur because of complementary drug action (multiple targets sites on the same protein or pathway are hit; Pemovska et al., 2018). By combining two drugs that achieve the same effect through different mechanisms of action, the development of resistance to a single drug in the combination may be less likely to occur, and when it does occur, it may have a lower impact on the therapeutic outcome (Pirrone et al., 2011). Finally, the use of more than one agent broadens the antibacterial spectrum of the empirical therapy and thus ensures that at least one agent will cover the infecting organism (Gurjar et al., 2014).
Antimicrobial Peptide-Based Combinatorial Treatments
Combinations of AMPs with antibiotics have been reported to show synergistic effects in the treatment of bacterial infections. The mechanism of antibacterial action in these combinations involves the disruption of the outer membrane (Cassone and Otvos, 2010). Moreover, the use of AMPs in combinatorial treatments has certain advantages over their use as a single treatment since it has been observed that in combinatorial treatments, AMPs work as enhancers of the antimicrobial effects. This characteristic allows the reduction of their dose, and it also unlocks the bactericidal application of molecules with low molecular weight, which typically do not exhibit antimicrobial properties (Si et al., 2020).
Recent studies have demonstrated the synergistic activity of antibiotics combined with AMPs. Akbari et al. (2019) reported the synergism and other drug interactions between melittin, a cationic amphipathic peptide, and antibiotics such as doripenem, doxycycline, colistin, and ceftazidime, against MDR isolates of A. baumannii and P. aeruginosa. Likewise, combinatorial treatments of conventional antibiotics with new synthetic peptides inspired by human cationic peptides LL-37 and thrombocidin-1 (TC-1) have shown synergistic activity against S. aureus (antibacterial and anti-biofilm activity; Koppen et al., 2019). In addition, the synergistic activity of 30 short AMPs combined with several conventional antibiotics such as beta-lactam antibiotics, cephalosporins, aminoglycosides, and quinolones was tested against an MDR P. aeruginosa isolate (PA910; Ruden et al., 2019). Several combinations between peptides, polymyxin B, erythromycin, and tetracycline, as well as novel variants of indolicidin were found to be synergistic. Furthermore, the results showed that a single amino acid substitution within the peptides can have a powerful effect on the ability to synergize, which represents an opportunity to design treatment strategies based on synergistic interactions (Ruden et al., 2019).
Metal Nanoparticle-Based Combinatorial Treatments
Metal nanoparticles should be considered as an attractive alternative to potentiate the antimicrobial effect of old and current antibiotics, since they have a high tendency to act synergistically when combined with a wide variety of antibiotics (Bankier et al., 2019). This, in addition to the increased biocompatibility achieved by synthesizing them through green chemistry, allows considering the use of MNPs as adjuvant agents for the treatment of infectious diseases (Rout et al., 2018).
In the past years, there has been a marked increase in the use of biopolymers (e.g., proteins, nucleic acids, and polysaccharide) as capping agents to functionalize and stabilize MNPs (Sharma et al., 2019). Exopolysaccharides are biocompatible and eco-friendly biomolecules; therefore, they can be used in the synthesis of MNPs (Escárcega-González et al., 2018). Recently, a silver-based nanobiocomposite was synthesized using an exopolysaccharide produced by Rhodotorula mucilaginosa UANL-001L (EPS). The results showed an increased antibacterial and anti-biofilm activity of this nanobiocomposite against pathogens of clinical relevance (Vazquez-Rodriguez et al., 2020). Moreover, nanocomposites have been synthesized through green chemistry, such as zinc (Zn) and nickel (Ni) MNPs capped with EPS as capping agents, and they have displayed interesting antimicrobial properties as well. Ni-EPS nanoparticles exhibited both antimicrobial and anti-biofilm activity against resistant MDR strains of S. aureus and P. aeruginosa. Furthermore, Zn-EPS nanoparticles showed antimicrobial activity for treatments against MDR S. aureus and P. aeruginosa (Garza-Cervantes et al., 2019).
Among the most studied nanomaterials are silver nanoparticles due to their antimicrobial activity against Gram-positive and Gram-negative bacteria. They can be used in combinatorial treatments with currently used antibiotics for enhanced antimicrobial activity (Shahverdi et al., 2007; Kora and Rastogi, 2013; Naqvi et al., 2013; Singh et al., 2013; Panácek et al., 2016; Lopez-Carrizales et al., 2018; Perveen et al., 2018; Vazquez-Muñoz et al., 2019). Nonetheless, some other MNPs such as gold (El-Sheekh and El Kassas, 2014; Kalita et al., 2016; Al-Mawlawi and Obaid, 2019; Arya et al., 2019; Lee and Lee, 2019; Nishanthi et al., 2019; Yang et al., 2019), copper (Khurana et al., 2016; Woźniak-Budych et al., 2017; Murugan, 2018; Selvaraj et al., 2019), and zinc (Banoee et al., 2010; Bhande et al., 2013) have been used in combination with a variety of antibiotic families to enhance bactericidal efficacy.
Some other interesting studies of silver-based nanomaterials have been reported. A novel silver-microfibrillated cellulose biocomposite has been synthesized, and its antimicrobial activity was determined against relevant clinical strains. The results showed that this biocomposite has antimicrobial activity against Gram-negative and Gram-positive bacteria so that it could be applied in the development of biocompatible biomedical devices (Garza-Cervantes et al., 2020a). Another promising approach toward the development of new antimicrobial combinatorial treatments is the use of transition metals, since they exhibit rapid and significant toxicity, at low concentrations, in different bacterial strains. Garza-Cervantes et al. evaluated the synergistic antimicrobial effects of silver/transition-metal combinatorial treatments. Their results showed combinatorial treatments that exhibited synergism (Ag–Zn, Ag–Co, Ag–Cd, Ag–Ni, and Ag–Cu) since their antimicrobial effects are increased up to 8-fold, compared to the effects observed for the treatments with the individual metals (Garza-Cervantes et al., 2017). Furthermore, Montelongo-Peralta et al. reported synergism between transition metals and antibiotics used to treat first-line drug-resistant strains of Mycobacterium tuberculosis (M. tuberculosis). Combinatorial treatments composed of isoniazid/silver exhibited a synergistic bactericidal effect in an isoniazid-resistant clinical strain of M. tuberculosis (Montelongo-Peralta et al., 2019).
Moreover, a previous study showed the ability of silver to potentiate the activity of a broad range of antibiotics against Gram-negative bacteria, as well as to restore antibiotic susceptibility (re-sensitizing) to a resistant bacterial strain (Morones-Ramirez et al., 2013). Recently, a group of researchers achieved to re-sensitize antibiotic-resistant E. coli using transition-metal micronutrients (Cu2+, Zn2+, Co2+, Cd2+, and Ni2+) combined with antibiotics (ampicillin and kanamycin). These combinatorial treatments showed a therapeutic activity and no toxicological effects in a murine topical infection model caused by antibiotic-resistant strains (Garza-Cervantes et al., 2020b). The above data therefore strongly suggest that combination therapies are a potential strategy in the development of new treatments against infectious diseases.
The search for a new generation of antimicrobials to mitigate the spread of antibiotic resistance is urgent (de la Fuente-Nunez et al., 2017). Current research and technology developments have promoted the improvement of antimicrobial agents that selectively target a target site (e.g., a gene, a cellular process, or a specific pathogen; de la Fuente-Nunez et al., 2017; Jackson et al., 2018). AMPs and MNPs exemplify a novel approach for treating infectious diseases. Nonetheless, the combinatorial treatments are considered as an excellent option for designing and developing next-generation antibacterial agents. As summary, Table 1 describes the mechanism of action, tested bacterial strains, advantages and disadvantages of AMPs, MNPs, and combinatorial treatments.
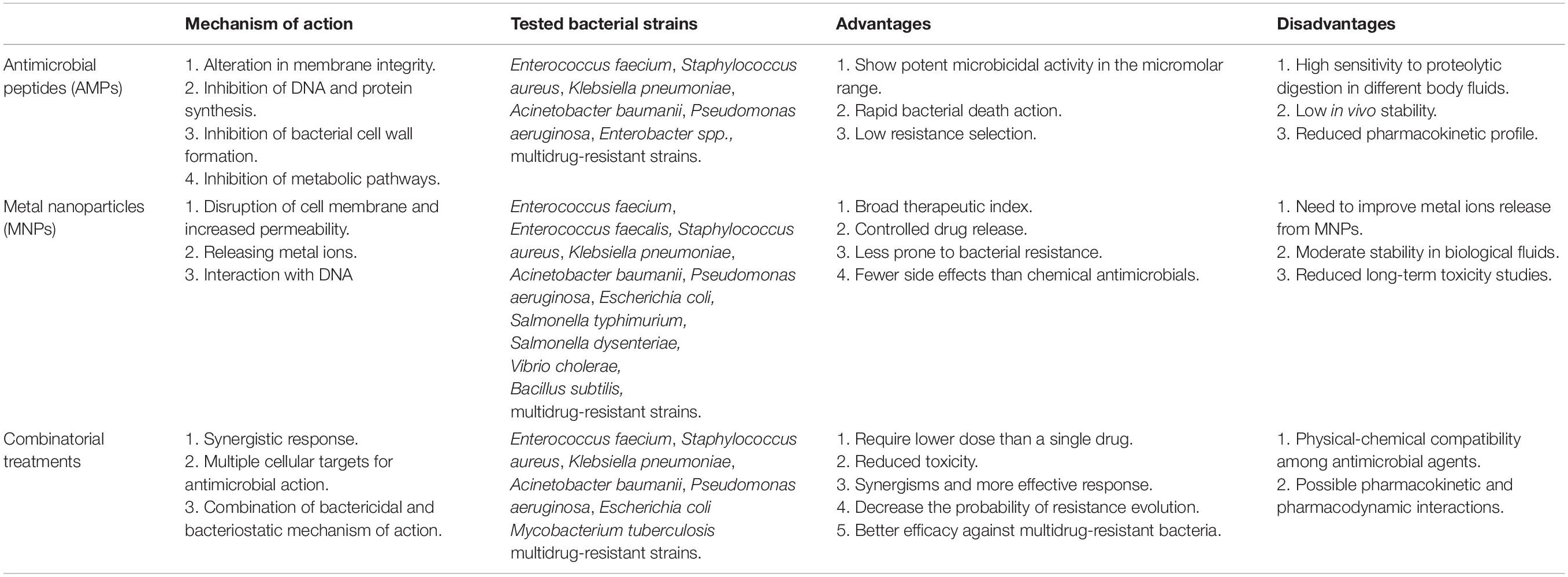
Table 1. Antimicrobial peptides, metal nanoparticles, and combinatorial treatments: mechanism of action, tested bacterial strains, advantages, and disadvantages.
The selection of appropriate combinatorial treatment is critical for the successful prevention of infections (Bayramov and Neff, 2017). The most important challenges include (i) selection of agents with ideal physical–chemical properties (hydrosolubility and chemical stability in biological fluids; Ebejer et al., 2016), (ii) selection of antimicrobials that display appropriate pharmacokinetics and pharmacodynamics properties (Preston, 2004), (iii) selection of biocompatible capping agents or biopolymer-based materials that enable drug release (Campoccia et al., 2013), and (iv) development of a process that ensures the stability and does not compromise the performance of the combination therapy formulation as a whole (Wu and Grainger, 2006). Therefore, the design of combinatorial treatment provides a pathway to develop antimicrobial therapeutics with broad-spectrum antimicrobial activity, bactericidal instead of bacteriostatic mechanism of action, and better efficacy against MDR bacteria.
Author Contributions
Conceptualization, AL-B and JM-R. Writing-original draft preparation AL-B, CG-C, JG-C, JL-E, and JM-R. Graphic design, JL-E. Writing-review and editing, AL-B, CG-C, JG-C, and JM-R. Supervision, AL-B and JM-R. All authors contributed to the article and approved the submitted version.
Funding
We would like to acknowledge Paicyt 2019–2020 and 2020–2021 Science Grant from the Universidad Autónoma de Nuevo León; CONACyT Grants for Basic Science grant 221332; Fronteras de la Ciencia grant 1502; and Infraestructura Grant 279957. JG-C and JL-E were supported by Beca Nacional de Posgrado from CONACyT.
Conflict of Interest
The authors declare that the research was conducted in the absence of any commercial or financial relationships that could be construed as a potential conflict of interest.
Acknowledgments
Dr. Angel Leon Buitimea would like to thank the support from Beca de Posdoctorado Nacional 2018–2020. CG-C received support from a Summer Scholarship (PROVERICyT) from the UANL.
References
Akbari, R., Hakemi-Vala, M., Pashaie, F., Bevalian, P., Hashemi, A., and Bagheri, K. P. (2019). Highly synergistic effects of melittin with conventional antibiotics against multidrug-resistant isolates of Acinetobacter baumannii and Pseudomonas aeruginosa. Microb. Drug Resist. 25, 193–202. doi: 10.1089/mdr.2018.0016
Al-Mawlawi, Z. S., and Obaid, H. H. (2019). Antibacterial activity of synergistic effect of colicin and gold nanoparticles against klebsiella pneumonia. Indian J. Public Heal. Res. Dev. 10:1041. doi: 10.5958/0976-5506.2019.00198.0
Andersson, D. I., Balaban, N. Q., Baquero, F., Courvalin, P., Glaser, P., Gophna, U., et al. (2020). Antibiotic resistance: turning evolutionary principles into clinical reality. FEMS Microbiol. Rev. 43, 341–361. doi: 10.1093/femsre/fuaa001
Aoki, W., and Ueda, M. (2013). Characterization of antimicrobial peptides toward the development of novel antibiotics. Pharmaceuticals 6, 1055–1081. doi: 10.3390/ph6081055
Arya, S. S., Sharma, M. M., Das, R. K., Rookes, J., Cahill, D., and Lenka, S. K. (2019). Vanillin mediated green synthesis and application of gold nanoparticles for reversal of antimicrobial resistance in Pseudomonas aeruginosa clinical isolates. Heliyon 5:e02021. doi: 10.1016/j.heliyon.2019.e02021
Bankier, C., Matharu, R. K., Cheong, Y. K., Ren, G. G., Cloutman-Green, E., and Ciric, L. (2019). Synergistic antibacterial effects of metallic nanoparticle combinations. Sci. Rep. 9:16074. doi: 10.1038/s41598-019-52473-2
Banoee, M., Seif, S., Nazari, Z. E., Jafari-Fesharaki, P., Shahverdi, H. R., Moballegh, A., et al. (2010). ZnO nanoparticles enhanced antibacterial activity of ciprofloxacin against Staphylococcus aureus and Escherichia coli. J. Biomed. Mater. Res. B Appl. Biomater. 93, 557–561. doi: 10.1002/jbm.b.31615
Bayramov, D. F., and Neff, J. A. (2017). Beyond conventional antibiotics — New directions for combination products to combat biofilm. Adv. Drug Deliv. Rev. 112, 48–60. doi: 10.1016/j.addr.2016.07.010
Bhande, R. M., Khobragade, C. N., Mane, R. S., and Bhande, S. (2013). Enhanced synergism of antibiotics with zinc oxide nanoparticles against extended spectrum β-lactamase producers implicated in urinary tract infections. J. Nanoparticle Res. 15:1413. doi: 10.1007/s11051-012-1413-4
Boparai, J. K., and Sharma, P. K. (2019). Mini review on antimicrobial peptides, sources, mechanism and recent applications. Protein Pept. Lett. 26, 4–16. doi: 10.2174/0929866526666190822165812
Borthagaray, G., Mondelli, M., Facchin, G., and Torre, M. H. (2018). “Silver-containing nanoparticles in the research of new antimicrobial agents against ESKAPE pathogens,” in Inorganic Frameworks as Smart Nanomedicines, ed. A. M. Grumezescu (Norwich, NY: William Andrew), 317–386. doi: 10.1016/b978-0-12-813661-4.00008-0
Campoccia, D., Montanaro, L., and Arciola, C. R. (2013). A review of the biomaterials technologies for infection-resistant surfaces. Biomaterials 34, 8533–8554. doi: 10.1016/j.biomaterials.2013.07.089
Cassone, M., and Otvos, L. (2010). Synergy among antibacterial peptides and between peptides and small-molecule antibiotics. Expert Rev. Anti. Infect. Ther. 8, 703–716. doi: 10.1586/eri.10.38
Chung, P. Y., and Khanum, R. (2017). Antimicrobial peptides as potential anti-biofilm agents against multidrug-resistant bacteria. J. Microbiol. Immunol. Infect. 50, 405–410. doi: 10.1016/j.jmii.2016.12.005
Das, P., Sengupta, K., Goel, G., and Bhattacharya, S. (2017). Colistin: pharmacology, drug resistance and clinical applications. J. Acad. Clin. Microbiol. 19, 77–85. doi: 10.4103/jacm.jacm_31_17
De Breij, A., Riool, M., Cordfunke, R. A., Malanovic, N., De Boer, L., Koning, R. I., et al. (2018). The antimicrobial peptide SAAP-148 combats drug-resistant bacteria and biofilms. Sci. Transl. Med. 10:eaan4044. doi: 10.1126/scitranslmed.aan4044
de Dicastillo, C. L., Patiño, C., Galotto, M. J., Vásquez-Martínez, Y., Torrent, C., Alburquenque, D., et al. (2019). Novel hollow titanium dioxide nanospheres with antimicrobial activity against resistant bacteria. Beilstein J. Nanotechnol. 10, 1716–1725. doi: 10.3762/bjnano.10.167
de la Fuente-Nunez, C., Torres, M. D., Mojica, F. J., and Lu, T. K. (2017). Next-generation precision antimicrobials: towards personalized treatment of infectious diseases. Curr. Opin. Microbiol. 37, 95–102. doi: 10.1016/j.mib.2017.05.014
Divyashree, M., Mani, M. K., Reddy, D., Kumavath, R., Ghosh, P., Azevedo, V., et al. (2019). Clinical Applications of Antimicrobial Peptides (AMPs): where do we stand now? Protein Pept. Lett. 27, 120–134. doi: 10.2174/0929866526666190925152957
Duplantier, A. J., and van Hoek, M. L. (2013). The human cathelicidin antimicrobial peptide LL-37 as a potential treatment for polymicrobial infected wounds. Front. Immunol. 4:143. doi: 10.3389/fimmu.2013.00143
Dürr, U. H. N., Sudheendra, U. S., and Ramamoorthy, A. (2006). LL-37, the only human member of the cathelicidin family of antimicrobial peptides. Biochim. Biophys. Acta Biomembr. 1758, 1408–1425. doi: 10.1016/j.bbamem.2006.03.030
Ebejer, J.-P., Charlton, M. H., and Finn, P. W. (2016). Are the physicochemical properties of antibacterial compounds really different from other drugs? J. Cheminform. 8:30. doi: 10.1186/s13321-016-0143-5
Eckert, R., Brady, K. M., Greenberg, E. P., Qi, F., Yarbrough, D. K., He, J., et al. (2006). Enhancement of antimicrobial activity against Pseudomonas aeruginosa by coadministration of G10KHc and tobramycin. Antimicrob. Agents Chemother. 50, 3833–3838. doi: 10.1128/AAC.00509-06
El-Sheekh, M. M., and El Kassas, H. Y. (2014). Biosynthesis, characterization and synergistic effect of phytogenic gold nanoparticles by marine picoeukaryote Picochlorum sp. in combination with antimicrobials. Rend. Lincei. 25, 513–521. doi: 10.1007/s12210-014-0341-x
Escárcega-González, C. E., Garza-Cervantes, J. A., Vázquez-Rodríguez, A., and Morones-Ramírez, J. R. (2018). Bacterial exopolysaccharides as reducing and/or stabilizing agents during synthesis of metal nanoparticles with biomedical applications. Int. J. Polym. Sci. 2018, 1–15. doi: 10.1155/2018/7045852
Gajdács, M. (2019). The concept of an ideal antibiotic: implications for drug design. Molecules 24:892. doi: 10.3390/molecules24050892
Garza-Cervantes, J. A., Chávez-Reyes, A., Castillo, E. C., García-Rivas, G., Ortega-Rivera, O. A., Salinas, E., et al. (2017). Synergistic antimicrobial effects of silver/transition-metal combinatorial treatments. Sci. Rep. 7, 1–16. doi: 10.1038/s41598-017-01017-7
Garza-Cervantes, J. A., Escárcega-González, C. E., Barriga Castro, E. D., Mendiola-Garza, G., Marichal-Cancino, B. A., López-Vázquez, M. A., et al. (2019). Antimicrobial and antibiofilm activity of biopolymer-Ni, Zn nanoparticle biocomposites synthesized using R. mucilaginosa UANL-001L exopolysaccharide as a capping agent. Int. J. Nanomed. 14, 2557–2571. doi: 10.2147/IJN.S196470
Garza-Cervantes, J. A., Mendiola-Garza, G., de Melo, E. M., Dugmore, T. I. J., Matharu, A. S., and Morones-Ramirez, J. R. (2020a). Antimicrobial activity of a silver-microfibrillated cellulose biocomposite against susceptible and resistant bacteria. Sci. Rep. 10:7281. doi: 10.1038/s41598-020-64127-9
Garza-Cervantes, J. A., Meza-Bustillos, J. F., Resendiz-Hernandez, H., Suarez-Cantú, I. A., Ortega-Rivera, O. A., Salinas, E., et al. (2020b). Re-sensitizing ampicillin and kanamycin-resistant E. coli and S. aureus using synergistic metal micronutrients-antibiotic combinations. Front. Bioeng. Biotechnol. 8:612. doi: 10.3389/FBIOE.2020.00612
Gelover, S., Gómez, L. A., Reyes, K., and Teresa Leal, M. (2006). A practical demonstration of water disinfection using TiO2 films and sunlight. Water Res. 40, 3274–3280. doi: 10.1016/j.watres.2006.07.006
Guo, B. L., Han, P., Guo, L. C., Cao, Y. Q., Li, A. D., Kong, J. Z., et al. (2015). The antibacterial activity of Ta-doped ZnO nanoparticles. Nanoscale Res. Lett. 10:336. doi: 10.1186/s11671-015-1047-4
Gurjar, M., Azim, A., Baronia, A., and Ahmed, A. (2014). Current concepts in combination antibiotic therapy for critically ill patients. Indian J. Crit. Care Med. 18, 310–314. doi: 10.4103/0972-5229.132495
He, J., Anderson, M. H., Shi, W., and Eckert, R. (2009). Design and activity of a ‘dual-targeted’ antimicrobial peptide. Int. J. Antimicrob. Agents 33, 532–537. doi: 10.1016/j.ijantimicag.2008.11.013
Huo, L., Huang, X., Ling, J., Liu, H., and Liu, J. (2017). Selective activities of STAMPs against Streptococcus mutans. Exp. Ther. Med. 15, 1886–1893. doi: 10.3892/etm.2017.5631
Jackson, N., Czaplewski, L., and Piddock, L. J. V. (2018). Discovery and development of new antibacterial drugs: learning from experience? J. Antimicrob. Chemother. 73, 1452–1459. doi: 10.1093/jac/dky019
Jesline, A., John, N. P., Narayanan, P. M., Vani, C., and Murugan, S. (2015). Antimicrobial activity of zinc and titanium dioxide nanoparticles against biofilm-producing methicillin-resistant Staphylococcus aureus. Appl. Nanosci. 5, 157–162. doi: 10.1007/s13204-014-0301-x
Kalita, S., Kandimalla, R., Sharma, K. K., Kataki, A. C., Deka, M., and Kotoky, J. (2016). Amoxicillin functionalized gold nanoparticles reverts MRSA resistance. Mater. Sci. Eng. C 61, 720–727. doi: 10.1016/j.msec.2015.12.078
Khurana, C., Sharma, P., Pandey, O. P., and Chudasama, B. (2016). Synergistic effect of metal nanoparticles on the antimicrobial activities of antibiotics against biorecycling microbes. J. Mater. Sci. Technol. 32, 524–532. doi: 10.1016/j.jmst.2016.02.004
Koppen, B. C., Mulder, P. P. G., de Boer, L., Riool, M., Drijfhout, J. W., and Zaat, S. A. J. (2019). Synergistic microbicidal effect of cationic antimicrobial peptides and teicoplanin against planktonic and biofilm-encased Staphylococcus aureus. Int. J. Antimicrob. Agents 53, 143–151. doi: 10.1016/j.ijantimicag.2018.10.002
Kora, A. J., and Rastogi, L. (2013). Enhancement of antibacterial activity of capped silver nanoparticles in combination with antibiotics, on model gram-negative and gram-positive bacteria. Bioinorg. Chem. Appl. 2013:871097. doi: 10.1155/2013/871097
Kościuczuk, E. M., Lisowski, P., Jarczak, J., Strzałkowska, N., Jóźwik, A., Horbañczuk, J., et al. (2012). Cathelicidins: family of antimicrobial peptides. A review. Mol. Biol. Rep. 39, 10957–10970. doi: 10.1007/s11033-012-1997-x
Krause, K. M., Serio, A. W., Kane, T. R., and Connolly, L. E. (2016). Aminoglycosides: an Overview. Cold Spring Harb. Perspect. Med. 6:a027029. doi: 10.1101/cshperspect.a027029
Kumar, R., Shukla, S. K., Pandey, M., Pandey, A., Pathak, A., and Dikshit, A. (2016). Synthesis and antimicrobial effects of colloidal gold nanoparticles against prevalent waterborne bacterial pathogens. Cogent Chem. 2:1192522. doi: 10.1080/23312009.2016.1192522
Le, C. F., Fang, C. M., and Sekaran, S. D. (2017). Intracellular targeting mechanisms by antimicrobial peptides. Antimicrob. Agents Chemother. 61:e02340-16. doi: 10.1128/AAC.02340-16
Lee, B., and Lee, D. G. (2019). Synergistic antibacterial activity of gold nanoparticles caused by apoptosis-like death. J. Appl. Microbiol. 127, 701–712. doi: 10.1111/jam.14357
Lee, N.-Y., Ko, W.-C., and Hsueh, P.-R. (2019). Nanoparticles in the treatment of infections caused by multidrug-resistant organisms. Front. Pharmacol. 10:1153. doi: 10.3389/fphar.2019.01153
Lehár, J., Krueger, A. S., Avery, W., Heilbut, A. M., Johansen, L. M., Price, E. R., et al. (2009). Synergistic drug combinations tend to improve therapeutically relevant selectivity. Nat. Biotechnol. 27, 659–666. doi: 10.1038/nbt.1549
Lei, J., Sun, L., Huang, S., Zhu, C., Li, P., He, J., et al. (2019). The antimicrobial peptides and their potential clinical applications. Am. J. Transl. Res. 11, 3919–3931.
Li, J., Koh, J. J., Liu, S., Lakshminarayanan, R., Verma, C. S., and Beuerman, R. W. (2017). Membrane active antimicrobial peptides: translating mechanistic insights to design. Front. Neurosci. 11:73. doi: 10.3389/fnins.2017.00073
Lopez-Carrizales, M., Velasco, K. I., Castillo, C., Flores, A., Magaña, M., Martinez-Castanon, G. A., et al. (2018). In vitro synergism of silver nanoparticles with antibiotics as an alternative treatment in multiresistant uropathogens. Antibiotics 7:50. doi: 10.3390/antibiotics7020050
Magiorakos, A. P., Srinivasan, A., Carey, R. B., Carmeli, Y., Falagas, M. E., Giske, C. G., et al. (2012). Multidrug-resistant, extensively drug-resistant and pandrug-resistant bacteria: an international expert proposal for interim standard definitions for acquired resistance. Clin. Microbiol. Infect. 18, 268–281. doi: 10.1111/j.1469-0691.2011.03570.x
Mahlapuu, M., Håkansson, J., Ringstad, L., and Björn, C. (2016). Antimicrobial peptides: an emerging category of therapeutic agents. Front. Cell. Infect. Microbiol. 6:194. doi: 10.3389/fcimb.2016.00194
Mantravadi, H. B. (2017). Effectivity of titanium oxide based nano particles on E. coli from clinical samples. J. Clin. Diagnostic Res. 11, DC37–DC40. doi: 10.7860/JCDR/2017/25334.10278
Mao, R., Teng, D., Wang, X., Xi, D., Zhang, Y., Hu, X., et al. (2013). Design, expression, and characterization of a novel targeted plectasin against methicillin-resistant Staphylococcus aureus. Appl. Microbiol. Biotechnol. 97, 3991–4002. doi: 10.1007/s00253-012-4508-z
Marks, L. R., Clementi, E. A., and Hakansson, A. P. (2013). Sensitization of Staphylococcus aureus to methicillin and other antibiotics in vitro and in vivo in the presence of HAMLET. PLoS One 8:e63158. doi: 10.1371/journal.pone.0063158
Moncla, B. J., Pryke, K., Rohan, L. C., and Graebing, P. W. (2011). Degradation of naturally occurring and engineered antimicrobial peptides by proteases. Adv. Biosci. Biotechnol. 02, 404–408. doi: 10.4236/abb.2011.26059
Montelongo-Peralta, L. Z., León-Buitimea, A., Palma-Nicolás, J. P., Gonzalez-Christen, J., and Morones-Ramírez, J. R. (2019). Antibacterial Activity of combinatorial treatments composed of transition-metal/antibiotics against Mycobacterium tuberculosis. Sci. Rep. 9:5471. doi: 10.1038/s41598-019-42049-5
Morones, J. R., Luis Elechiguerra, J., Camacho, A., Holt, K., Kouri, J. B., Tapia Ramírez, J., et al. (2005). The bactericidal effect of silver nanoparticles. Inst. Phys. Publ. Nanotechnol. Nanotechnol. 16, 2346–2353. doi: 10.1088/0957-4484/16/10/059
Morones-Ramirez, J. R., Winkler, J. A., Spina, C. S., and Collins, J. J. (2013). Silver enhances antibiotic activity against gram-negative bacteria. Sci. Transl. Med. 5:190ra81. doi: 10.1126/scitranslmed.3006276
Mulani, M. S., Kamble, E. E., Kumkar, S. N., Tawre, M. S., and Pardesi, K. R. (2019). Emerging strategies to combat ESKAPE pathogens in the era of antimicrobial resistance: a review. Front. Microbiol. 10:539. doi: 10.3389/fmicb.2019.00539
Murugan, S. (2018). Investigation of the synergistic antibacterial action of copper nanoparticles on certain antibiotics against human pathogens. Int. J. Pharm. Pharm. Sci. 10, 83–86. doi: 10.22159/ijpps.2018v10i10.28069
Mwangi, J., Hao, X., Lai, R., and Zhang, Z. (2019). Antimicrobial peptides: new hope in the war against multidrug resistance. Zool. Res. 40, 488–505. doi: 10.24272/j.issn.2095-8137.2019.062
Naqvi, S. Z. H., Kiran, U., Ali, M. I., Jamal, A., Hameed, A., Ahmed, S., et al. (2013). Combined efficacy of biologically synthesized silver nanoparticles and different antibiotics against multidrug-resistant bacteria. Int. J. Nanomed. 8, 3187–3195. doi: 10.2147/IJN.S49284
Nishanthi, R., Malathi, S., John, P. S., and Palani, P. (2019). Green synthesis and characterization of bioinspired silver, gold and platinum nanoparticles and evaluation of their synergistic antibacterial activity after combining with different classes of antibiotics. Mater. Sci. Eng. C 96, 693–707. doi: 10.1016/j.msec.2018.11.050
Oka, H., and Ito, Y. (2000). “ANTIBIOTICS | High-Speed Countercurrent Chromatography,” in Encyclopedia of Separation Science, eds E. R. Adlard I D. Wilson, C. F. Poole, and M. Cooke (Amsterdam: Elsevier), 2058–2067. doi: 10.1016/b0-12-226770-2/03311-1
Panácek, A., Smékalová, M., Kilianová, M., Prucek, R., Bogdanová, K., Věcěrová, R., et al. (2016). Strong and nonspecific synergistic antibacterial efficiency of antibiotics combined with silver nanoparticles at very low concentrations showing no cytotoxic effect. Molecules 21:26. doi: 10.3390/molecules21010026
Pemovska, T., Bigenzahn, J. W., and Superti-Furga, G. (2018). Recent advances in combinatorial drug screening and synergy scoring. Curr. Opin. Pharmacol. 42, 102–110. doi: 10.1016/j.coph.2018.07.008
Pendleton, J. N., Gorman, S. P., and Gilmore, B. F. (2013). Clinical relevance of the ESKAPE pathogens. Expert Rev. Anti. Infect. Ther. 11, 297–308. doi: 10.1586/eri.13.12
Perveen, S., Safdar, N., Chaudhry, G. E., and Yasmin, A. (2018). Antibacterial evaluation of silver nanoparticles synthesized from lychee peel: individual versus antibiotic conjugated effects. World J. Microbiol. Biotechnol. 34:118. doi: 10.1007/s11274-018-2500-1
Pirrone, V., Thakkar, N., Jacobson, J. M., Wigdahl, B., and Krebs, F. C. (2011). Combinatorial approaches to the prevention and treatment of HIV-1 infection. Antimicrob. Agents Chemother. 55, 1831–1842. doi: 10.1128/AAC.00976-10
Preston, S. L. (2004). The importance of appropriate antimicrobial dosing: pharmacokinetic and pharmacodynamic considerations. Ann. Pharmacother. 38(9 Suppl.), S14–S18. doi: 10.1345/aph.1E218
Rout, G. K., Shin, H.-S., Gouda, S., Sahoo, S., Das, G., Fraceto, L. F., et al. (2018). Current advances in nanocarriers for biomedical research and their applications. Artif. Cells Nanomed. Biotechnol. 46, 1053–1062. doi: 10.1080/21691401.2018.1478843
Ruden, S., Rieder, A., Chis Ster, I., Schwartz, T., Mikut, R., and Hilpert, K. (2019). Synergy pattern of short cationic antimicrobial peptides against multidrug-resistant Pseudomonas aeruginosa. Front. Microbiol. 10:2740. doi: 10.3389/fmicb.2019.02740
Sarma, P., Mahendiratta, S., Prakash, A., and Medhi, B. (2018). Specifically targeted antimicrobial peptides: a new and promising avenue in selective antimicrobial therapy. Indian J. Pharmacol. 50:1. doi: 10.4103/ijp.IJP_218_18
Selvaraj, R. C. A., Rajendran, M., and Nagaiah, H. P. (2019). Re-potentiation of β-lactam antibiotic by synergistic combination with biogenic copper oxide nanocubes against biofilm forming multidrug-resistant bacteria. Molecules 24:3055. doi: 10.3390/molecules24173055
Shahverdi, A. R., Fakhimi, A., Shahverdi, H. R., and Minaian, S. (2007). Synthesis and effect of silver nanoparticles on the antibacterial activity of different antibiotics against Staphylococcus aureus and Escherichia coli. Nanomed. Nanotechnol. Biol. Med. 3, 168–171. doi: 10.1016/j.nano.2007.02.001
Shaikh, S., Nazam, N., Rizvi, S. M. D., Ahmad, K., Baig, M. H., Lee, E. J., et al. (2019). Mechanistic insights into the antimicrobial actions of metallic nanoparticles and their implications for multidrug resistance. Int. J. Mol. Sci. 20:2468. doi: 10.3390/ijms20102468
Sharma, D., Kanchi, S., and Bisetty, K. (2019). Biogenic synthesis of nanoparticles: a review. Arab. J. Chem. 12, 3576–3600. doi: 10.1016/j.arabjc.2015.11.002
Si, Z., Lim, H. W., Tay, M. Y. F., Du, Y., Ruan, L., Qiu, H., et al. (2020). A glycosylated cationic block poly(beta-peptide) reverses intrinsic antibiotic resistance in all ESKAPE Gram-negative bacteria. Angew. Chemie Int. Ed. 59, 6819–6826. doi: 10.1002/anie.201914304
Singh, R., Wagh, P., Wadhwani, S., Gaidhani, S., Kumbhar, A., Bellare, J., et al. (2013). Synthesis, optimization, and characterization of silver nanoparticles from Acinetobacter calcoaceticus and their enhanced antibacterial activity when combined with antibiotics. Int. J. Nanomed. 8, 4277–4290. doi: 10.2147/IJN.S48913
Starr, C. G., and Wimley, W. C. (2017). Antimicrobial peptides are degraded by the cytosolic proteases of human erythrocytes. Biochim. Biophys. Acta Biomembr. 1859, 2319–2326. doi: 10.1016/j.bbamem.2017.09.008
Tamma, P. D., Cosgrove, S. E., and Maragakis, L. L. (2012). Combination therapy for treatment of infections with gram-negative bacteria. Clin. Microbiol. Rev. 25, 450–470. doi: 10.1128/CMR.05041-11
Tiwari, V., Mishra, N., Gadani, K., Solanki, P. S., Shah, N. A., and Tiwari, M. (2018). Mechanism of anti-bacterial activity of zinc oxide nanoparticle against carbapenem-resistant Acinetobacter baumannii. Front. Microbiol. 9:1218. doi: 10.3389/fmicb.2018.01218
van der Weide, H., Vermeulen-de Jongh, D. M. C., van der Meijden, A., Boers, S. A., Kreft, D., ten Kate, M. T., et al. (2019). Antimicrobial activity of two novel antimicrobial peptides AA139 and SET-M33 against clinically and genotypically diverse Klebsiella pneumoniae isolates with differing antibiotic resistance profiles. Int. J. Antimicrob. Agents 54, 159–166. doi: 10.1016/j.ijantimicag.2019.05.019
Vazquez-Muñoz, R., Meza-Villezcas, A., Fournier, P. G. J., Soria-Castro, E., Juarez-Moreno, K., Gallego-Hernández, A. L., et al. (2019). Enhancement of antibiotics antimicrobial activity due to the silver nanoparticles impact on the cell membrane. PLoS One 14:e0224904. doi: 10.1371/journal.pone.0224904
Vazquez-Rodriguez, A., Vasto-Anzaldo, X., Leon-Buitimea, A., Zarate, X., and Morones-Ramirez, J. R. (2020). Antibacterial and antibiofilm activity of biosynthesized silver nanoparticles coated with exopolysaccharides obtained from Rhodotorula mucilaginosa. IEEE Trans. Nanobioscience 19, 498–503. doi: 10.1109/TNB.2020.2985101
Wang, G. (2013). Database-guided discovery of potent peptides to combat HIV-1 or superbugs. Pharmaceuticals 6, 728–758. doi: 10.3390/ph6060728
Wang, L., Hu, C., and Shao, L. (2017). The antimicrobial activity of nanoparticles: present situation and prospects for the future. Int. J. Nanomedicine 12, 1227–1249. doi: 10.2147/IJN.S121956
World Health Organization (WHO) (2020). Antibiotic Resistance. Available online at: https://www.who.int/news-room/fact-sheets/detail/antibiotic-resistance (accessed January 13, 2020).
Wolfram, J., Zhu, M., Yang, Y., Shen, J., Gentile, E., Paolino, D., et al. (2015). Safety of nanoparticles in medicine. Curr. Drug Targets 16, 1671–1681. doi: 10.2174/1389450115666140804124808
Woźniak-Budych, M. J., Przysiecka, Ł, Langer, K., Pepliñska, B., Jarek, M., Wiesner, M., et al. (2017). Green synthesis of rifampicin-loaded copper nanoparticles with enhanced antimicrobial activity. J. Mater. Sci. Mater. Med. 28:42. doi: 10.1007/s10856-017-5857-z
Wu, P., and Grainger, D. W. (2006). Drug/device combinations for local drug therapies and infection prophylaxis. Biomaterials 27, 2450–2467. doi: 10.1016/j.biomaterials.2005.11.031
Xu, L., Shao, C., Li, G., Shan, A., Chou, S., Wang, J., et al. (2020). Conversion of broad-spectrum antimicrobial peptides into species-specific antimicrobials capable of precisely targeting pathogenic bacteria. Sci. Rep. 10:944. doi: 10.1038/s41598-020-58014-6
Yang, P., Pageni, P., Rahman, M. A., Bam, M., Zhu, T., Chen, Y. P., et al. (2019). Gold nanoparticles with antibiotic-metallopolymers toward broad-spectrum antibacterial effects. Adv. Healthc. Mater. 8:e1800854. doi: 10.1002/adhm.201800854
Zhang, Y., Bai, Y., Jia, J., Gao, N., Li, Y., Zhang, R., et al. (2014). Perturbation of physiological systems by nanoparticles. Chem. Soc. Rev. 43, 3762–3809. doi: 10.1039/c3cs60338e
Zharkova, M. S., Orlov, D. S., Golubeva, O. Y., Chakchir, O. B., Eliseev, I. E., Grinchuk, T. M., et al. (2019). Application of antimicrobial peptides of the innate immune system in combination with conventional antibiotics-a novel way to combat antibiotic resistance? Front. Cell. Infect. Microbiol. 9:128. doi: 10.3389/fcimb.2019.00128
Keywords: ESKAPE, MDR, XDR, antimicrobial peptides, metal nanoparticles, combinatorial treatments
Citation: León-Buitimea A, Garza-Cárdenas CR, Garza-Cervantes JA, Lerma-Escalera JA and Morones-Ramírez JR (2020) The Demand for New Antibiotics: Antimicrobial Peptides, Nanoparticles, and Combinatorial Therapies as Future Strategies in Antibacterial Agent Design. Front. Microbiol. 11:1669. doi: 10.3389/fmicb.2020.01669
Received: 11 April 2020; Accepted: 25 June 2020;
Published: 24 July 2020.
Edited by:
Hemda Garelick, Middlesex University, United KingdomReviewed by:
Lilit Tonoyan, National University of Ireland Galway, IrelandMaria José Saavedra, Universidade de Trás-os-Montes e Alto Douro, Portugal
Copyright © 2020 León-Buitimea, Garza-Cárdenas, Garza-Cervantes, Lerma-Escalera and Morones-Ramírez. This is an open-access article distributed under the terms of the Creative Commons Attribution License (CC BY). The use, distribution or reproduction in other forums is permitted, provided the original author(s) and the copyright owner(s) are credited and that the original publication in this journal is cited, in accordance with accepted academic practice. No use, distribution or reproduction is permitted which does not comply with these terms.
*Correspondence: Jose R. Morones-Ramírez, am9zZS5tb3JvbmVzcm1yQHVhbmwuZWR1Lm14
†These authors have contributed equally to this work