- 1Departments of Ecology and Conservation Biology, and Wildlife and Fisheries Sciences, Texas A&M University, College Station, TX, United States
- 2Laboratorio de Genética y Genómica Comparativa, Unidad Académica Multidisciplinaria Reynosa Aztlan, Universidad Autónoma de Tamaulipas, Ciudad Victoria, Mexico
- 3Instituto de Genética ‘Ewald A. Favret’ – GV IABIMO (INTA-CONICET) Hurlingham, Buenos Aires, Argentina
- 4El Colegio de la Frontera Sur, Tapachula, Mexico
- 5Department of Environmental Engineering, University of Patras, Agrinio, Greece
- 6Laboratory of Entomology and Agricultural Zoology, Department of Agriculture Crop Production and Rural Environment, University of Thessaly, Larissa, Greece
- 7Department of Plant Protection, Institute of Industrial and Forage Crops, Hellenic Agricultural Organization – DEMETER, Patras, Greece
Members of the true fruit flies (family Tephritidae) are among the most serious agricultural pests worldwide, whose control and management demands large and costly international efforts. The need for cost-effective and environmentally friendly integrated pest management (IPM) has led to the development and implementation of autocidal control strategies. These approaches include the widely used sterile insect technique and the incompatible insect technique (IIT). IIT relies on maternally transmitted bacteria (namely Wolbachia) to cause a conditional sterility in crosses between released mass-reared Wolbachia-infected males and wild females, which are either uninfected or infected with a different Wolbachia strain (i.e., cytoplasmic incompatibility; CI). Herein, we review the current state of knowledge on Wolbachia-tephritid interactions including infection prevalence in wild populations, phenotypic consequences, and their impact on life history traits. Numerous pest tephritid species are reported to harbor Wolbachia infections, with a subset exhibiting high prevalence. The phenotypic effects of Wolbachia have been assessed in very few tephritid species, due in part to the difficulty of manipulating Wolbachia infection (removal or transinfection). Based on recent methodological advances (high-throughput DNA sequencing) and breakthroughs concerning the mechanistic basis of CI, we suggest research avenues that could accelerate generation of necessary knowledge for the potential use of Wolbachia-based IIT in area-wide integrated pest management (AW-IPM) strategies for the population control of tephritid pests.
Introduction
The Economic Importance and Management of Tephritid Pest Species
Flies in the family Tephritidae (Diptera) include some of the world’s most important agricultural pests. The family is comprised of ∼4,900 described species within 481 genera, of which six (Anastrepha, Bactrocera, Ceratitis, Dacus, Rhagoletis, and Zeugodacus) contain ∼70 major pest species (White and Elson-Harris, 1992; Norrbom, 2004a, b, 2010; Mengual et al., 2017). Pest tephritids represent an enormous economic cost because they cause direct losses to a diversity of crops (fruits, vegetables, and flowers) (White and Elson-Harris, 1992). Furthermore, they hamper the development of agriculture in numerous countries, due to the strict quarantines imposed by countries importing affected crops, and to the huge costs associated with efforts aimed at prevention, containment, suppression, and eradication.
To prevent or minimize the harmful effects of tephritid pests, growers must comply with health and safety standards required by the market, applying an area-wide management approach involving chemical, biological, cultural, and autocidal control practices (Reyes et al., 2000; Enkerlin, 2005). Autocidal refers to methods that use the insect to control itself, by releasing insects that are sterile or induce sterility upon mating with wild insects in the next or subsequent generations (Black et al., 2011; Leftwich et al., 2014; Handler, 2016). Autocidal strategies include the sterile insect technique (SIT) (Knipling, 1955; Hendrichs and Robinson, 2009); one of the most widespread control methods used against fruit flies (reviewed in Dias et al., 2018). SIT relies on the mass-rearing production, sterilization and recurrent release of insects (preferentially males) of the targeted species. Sterilization is typically attained by radiation (Bakri et al., 2005), in a way that does not impair male mating and insemination capabilities. Wild females that mate with sterilized males lay unfertilized eggs. At the appropriate sterile:wild (S:W) ratio, the reproductive potential of the target population can be reduced (Knipling, 1955; Klassen and Curtis, 2005; Cáceres et al., 2007). Historically, at least 28 countries have used the SIT at a large-scale for the suppression or eradication of pests (Hendrichs et al., 1995, 2005; Suckling et al., 2016). SIT has been applied successfully for several non-tephritid insect pests including the New World screw worm Cochliomyia hominivorax (Coquerel), several species of tsetse fly (Glossina spp.), the codling moth Cydia pomonella (L.) (reviewed in Robinson, 2002b; Dyck et al., 2005; Bourtzis and Robinson, 2006), and mosquitoes (Benedict and Robinson, 2003; Lees et al., 2015). Successful SIT programs as part of Area-wide Integrated Pest Management (AW-IPM) strategies have also been implemented for several tephritids: Ceratitis capitata (Wiedemann); Anastrepha ludens (Loew); Anastrepha obliqua (Macquart); Zeugodacus cucurbitae (Coquillett); Bactrocera dorsalis Hendel; and Bactrocera tryoni (Froggatt) (Enkerlin, 2005; Hendrichs et al., 2005; Klassen and Curtis, 2005; Cáceres et al., 2007). SIT is currently being developed for three additional tephritid species: Anastrepha fraterculus (Wiedemann) (Cladera et al., 2014); Dacus ciliatus Loew (Rempoulakis et al., 2015) and Bactrocera tau (Walker) (Du et al., 2016). The advantages of the SIT over other pest control approaches (e.g., use of pesticides) are that it is species-specific and environmentally friendly (Lees et al., 2015; Bourtzis et al., 2016), and resistance is less likely to evolve (but see Hibino and Iwahashi, 1991; McInnis et al., 1996).
Another autocidal strategy where mating between mass-reared and wild insects can be used to suppress pest populations is the incompatible insect technique (IIT); coined by Boller et al. (1976). The earliest successful pilot application of IIT was in Culex mosquitoes (Laven, 1967), and interest in applying it to mosquitoes has resurged in recent years (reviewed in Ross et al., 2019b). IIT also relies on the principle of reducing female fertility, but utilizes endosymbiotic bacteria instead of radiation, to induce a context-dependent sterility in wild females. It is based on the ability of certain maternally inherited bacteria (mainly from the genus Wolbachia) to induce a form of reproductive incompatibility known as cytoplasmic incompatibility (CI; explained in the section below). Herein we review the current knowledge on taxonomic diversity of Wolbachia-tephritid associations and their phenotypic consequences, and identify gaps in knowledge and approaches in the context of potential application of IIT, alone or in combination with SIT, in AW-IPM programs to control tephritid pests.
The Influence of Wolbachia on Host Ecology
Insects and other arthropods are common hosts of maternally inherited bacteria (reviewed in Duron and Hurst, 2013). These heritable endosymbionts can have a strong influence on host ecology. Typically, such vertically transmitted bacteria are vastly (or fully) dependent on the host for survival and transmission. Certain associations are obligate for both partners, and generally involve a nutritional benefit to the host. Other heritable bacteria are facultative, with such associations ranging from mutualistic to parasitic from the host’s perspective. Among these, Wolbachia is the most common and widespread facultative symbiont of insects and arthropods (Hilgenboecker et al., 2008; Zug and Hammerstein, 2012; de Oliveira et al., 2015; Weinert et al., 2015).
Wolbachia is a diverse and old genus (possibly older than 200 million years; Gerth and Bleidorn, 2016) of intracellular Gram-negative Alphaproteobacteria (within the order Rickettsiales) associated with arthropods and filarial nematodes. Wolbachia cells resemble small spheres 0.2–1.5 μm, occur in all tissue types, but tend to be more prevalent in ovaries and testes of infected hosts, and are closely associated with the female germline (reviewed by Harris et al., 2010; see also Sacchi et al., 2010). Wolbachia is estimated to infect 40–66% of insect species (Hilgenboecker et al., 2008; Zug and Hammerstein, 2012; de Oliveira et al., 2015; Weinert et al., 2015). Within a species or population, the infection prevalence of Wolbachia can be quite variable over space (e.g., Kriesner et al., 2016) and time (e.g., Turelli and Hoffmann, 1991, 1995).
The most commonly documented effects of Wolbachia on arthropod hosts fall under the category of reproductive parasitism, which involves manipulation of host reproduction to enhance symbiont transmission and persistence, in general by increasing the relative frequency of Wolbachia-infected vs. uninfected females. Females are typically the sex that can transmit Wolbachia and other heritable bacteria, although rare exceptions exist (Hoffmann and Turelli, 1988; Moran and Dunbar, 2006; Chafee et al., 2010). Wolbachia employs all four types of reproductive manipulation (reviewed by Werren et al., 2008; Saridaki and Bourtzis, 2010; Schneider et al., 2011). Feminization converts genetic males into functional females, and occurs in the orders Hemiptera, Lepidoptera, and Isopoda. Wolbachia-induced parthenogenesis occurs in haplo-diploid hosts (e.g., Acari, Hymenoptera, and Thysanoptera), where unfertilized eggs, which would otherwise develop into males, develop into females. Male killing causes death of infected males to the presumed advantage of surviving infected female siblings, and occurs in Coleoptera, Diptera, Lepidoptera, and Pseudoscorpionida. Cytoplasmic incompatibility (CI) (Yen and Barr, 1971) prevents infected males from producing viable offspring upon mating with females lacking Wolbachia (or a compatible strain of Wolbachia; see below; Figure 1). CI is the most commonly reported Wolbachia-induced reproductive phenotype, and is found in Acari, Coleoptera, Diptera, Hemiptera, Hymenoptera, Isopoda, Lepidoptera, and Orthoptera.
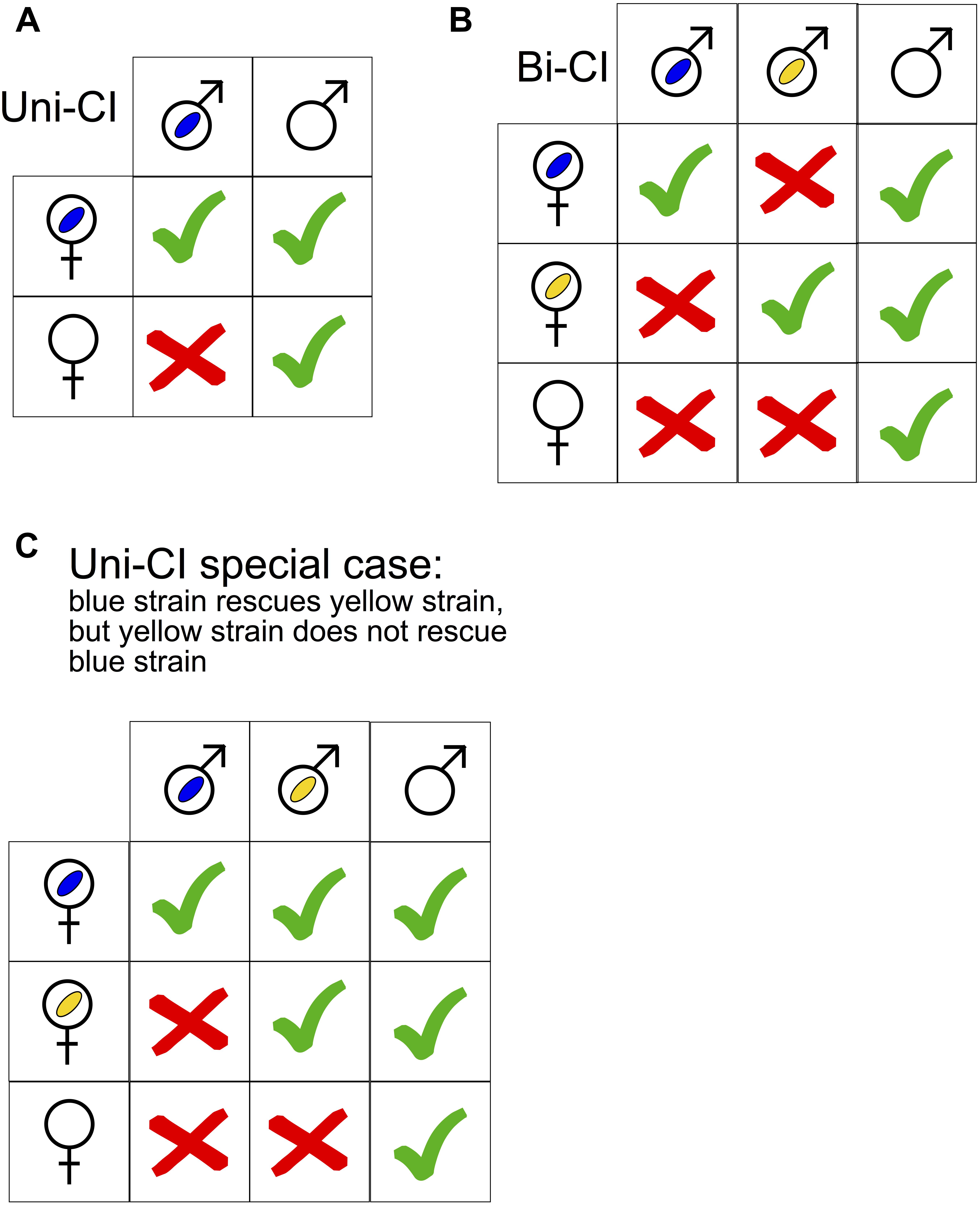
Figure 1. (A,B) Qualitative illustration of uni- and bidirectional cytoplasmic incompatibility (CI) on the basis of the Wolbachia infection status of the parent generation. Empty male and female symbols signify absence of Wolbachia. Blue and yellow ovals represent distinct Wolbachia strains. Green tick marks = Successful offspring production. Red crosses = no offspring production. (C) A special case of unidirectional incompatibility in which one Wolbachia strain (see text) can rescue another strain (i.e., the yellow one), but not vice versa.
Cytoplasmic incompatibility was discovered almost half a century ago (Yen and Barr, 1971), but its mechanism has not been fully elucidated. A useful conceptual model to understand the observed patterns of CI is “mod/resc” (Hurst, 1991; Werren, 1997). It postulates that Wolbachia has two functions: mod (for modification), which acts as a toxin or imprint of the male germline; and resc (for rescue), which acts as an antidote. The mod function acts on the nucleus in the male germline, before Wolbachia are shed from maturing sperm (Presgraves, 2000). When a sperm nucleus affected by mod enters the egg of an uninfected female, this nucleus encounters problems such as delays in DNA replication and cell-cycle progression, leading to embryo death. In contrast, if the appropriate resc (“the antidote”) function is active in the egg, the defect caused by mod in the sperm is rescued, and the embryo proceeds through normal development. In the case of unidirectional CI (uni-CI), all or some of the eggs from uninfected females that are fertilized by sperm from Wolbachia-infected males (the “CI cross”) fail to develop (Figure 1A). Wolbachia-infected females are compatible with uninfected males, and thus have a reproductive advantage over their Wolbachia-uninfected counterparts. Consequently, above a certain threshold of Wolbachia infection frequency in a host population, Wolbachia frequencies can rapidly increase to a stable equilibrium frequency. When CI is strong (e.g., all embryos from the CI cross fail), fitness costs of Wolbachia are low, and maternal (vertical) transmission is high, the threshold Wolbachia frequency to achieve invasion can be close to zero, and the stable equilibrium frequency can be close to 100% (Caspari and Watson, 1959; Turelli and Hoffmann, 1999; Rasgon, 2008). Bi-directional CI (bi-CI) results from crosses involving two different (incompatible) Wolbachia strains (Figure 1B). Crosses between females and males infected with the same or compatible Wolbachia strains are viable. Under bi-CI between two Wolbachia strains with equivalent fitness effects on a host, the infection frequency of an introduced strain must exceed 50% to achieve invasion (Rousset et al., 1991; Dobson et al., 2002). Special cases of uni-CI and bi-CI patterns can occur. For example, a strain may not induce CI, but is able to rescue the defect caused by a different strain (Figure 1C) (Zabalou et al., 2004a).
Several recent breakthrough studies have collectively identified Wolbachia-encoded genes (of viral origin) that contribute to the induction and rescue of CI (Beckmann et al., 2017; LePage et al., 2017; Bonneau et al., 2018, 2019; Lindsey et al., 2018; Shropshire et al., 2018; Beckmann et al., 2019c; Chen et al., 2019; Shropshire and Bordenstein, 2019). Wolbachia-encoded genes that rescue CI are labeled as cifA, cidA, or cindA, depending on whether they rescue a defect caused by deubiquitylase (d), nuclease (n), both (nd); “f” is used by certain authors and/or when the nature of the defect is unknown (see Beckmann et al., 2019a, b; Shropshire et al., 2019). In CI-inducing Wolbachia strains, each of the above genes occurs upstream of a gene (its “cognate”) similarly labeled, but with a “B” replacing the “A” (i.e., cifB, cidB, or cindB, respectively) that seems to function as a toxin. Certain Wolbachia strains have more than one “A–B” pair, and the combination of these is consistent with patterns of incompatibility in Drosophila (LePage et al., 2017) and Culex (Bonneau et al., 2018). Knowledge accrued to date indicates that more than one Wolbachia-encoded mechanism of CI exists, and thus, information on the genes encoded by Wolbachia genomes can help predict expected patterns of incompatibility among strains that have not been experimentally characterized.
In addition to its reproductive phenotypes on arthropods, Wolbachia engages in obligate mutualistic interactions with filarial nematodes (Werren et al., 2008) and with members of five insect orders (reviewed in Zug and Hammerstein, 2015). As a facultative symbiont, Wolbachia can provide direct fitness benefits to its insect hosts by influencing development, nutrition, iron metabolism, lifespan, and fecundity (Dean, 2006; Aleksandrov et al., 2007; Weeks et al., 2007; Brownlie et al., 2009; Ikeya et al., 2009; Kremer et al., 2009; Newton and Rice, 2020), and most notably, by conferring resistance or tolerance to pathogens, particularly single-stranded RNA viruses (Hedges et al., 2008; Teixeira et al., 2008; Moreira et al., 2009). The interference of Wolbachia with the replication and transmission of certain viruses, along with its ability to spread in populations via CI, form the basis of several population replacement programs (reviewed in Ross et al., 2019b; Chrostek et al., 2020). Wolbachia in Drosophila appears to confer an additional fitness benefit in the form of increased recombination (Bryant and Newton, 2019; Singh, 2019).
Certain host-Wolbachia combinations incur fitness costs to the host, beyond reproductive parasitism, including reduced longevity, sperm competitive ability, and fecundity, as well as higher susceptibility to natural enemies (Hoffmann et al., 1990; Min and Benzer, 1997; Snook et al., 2000; Champion de Crespigny and Wedell, 2006; Fytrou et al., 2006; Vasquez et al., 2011; Suh et al., 2017; Sumida et al., 2017). Similarly, certain host-Wolbachia combinations may potentially enhance pathogen-vectoring capacities (Hughes et al., 2012b; Baton et al., 2013; Dodson et al., 2014; Murdock et al., 2014). Wolbachia has been reported to influence positively or negatively numerous aspects of their host’s behavior including sleep, learning and memory capacity, mating, feeding, thermal preference, locomotion, and agression (reviewed by Bi and Wang, 2019; Wedell, 2019).
Methods to Study Wolbachia
Methods to Assess Wolbachia Infection Status
For purposes of this review, we consider a host species or population as “infected” with Wolbachia, even if the infection is transient or found at low titer. Wolbachia, and most cytoplasmically transmitted endosymbionts, are fastidious to culture outside host cells, such that their study typically relies on culture-independent methods. A recommended flow-chart of steps is depicted in Figure 2. The most utilized approach to date for identifying hosts infected with Wolbachia is through PCR screening of Wolbachia genes in DNA extracts of hosts. Different PCR primers have been used to perform such surveys, traditionally targeting a portion of the 16S ribosomal (r)RNA gene or of a ubiquitous protein-coding gene (e.g., wsp or ftsZ). Simoes et al. (2011) evaluated the relative sensitivity and specificity of different primer pairs aimed at Wolbachia detection and identification, revealing that no single PCR protocol is capable of specific detection of all known Wolbachia strains. A related method known as “loop mediated isothermal amplification” (LAMP; not shown in Figure 2), which requires less infrastructure than PCR, has been successfully employed for Wolbachia detection in several insects (da Silva Gonçalves et al., 2014).
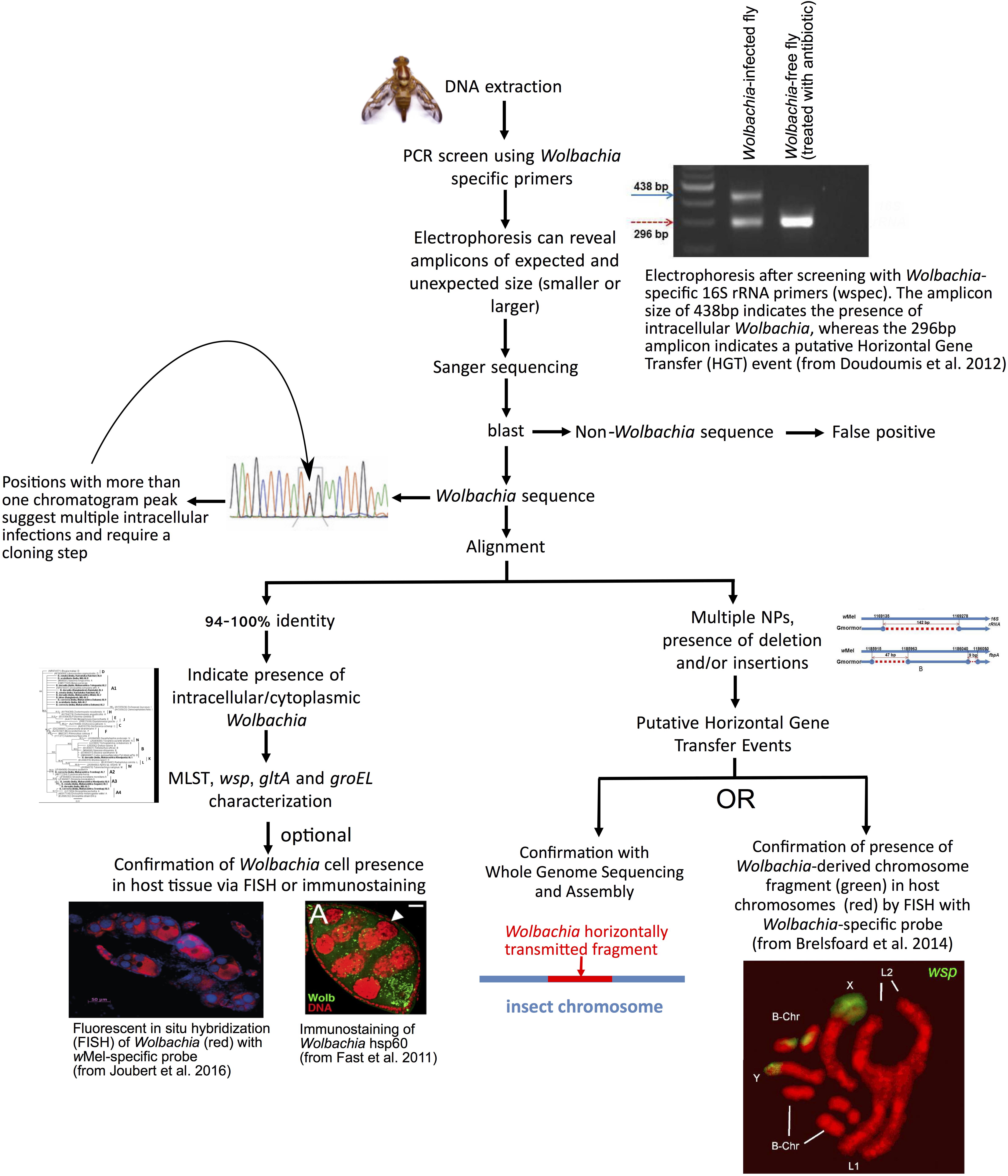
Figure 2. Recommended steps to screen for Wolbachia infections in tephritids and other arthropods. A PCR is performed with Wolbachia-specific primers on DNA isolated from whole, or parts of (e.g., abdomens), insect. Agarose gel electrophoresis of the PCR products is used to determine whether the amplicon is of the expected size. Amplicons of expected size are directly sequenced (e.g., Sanger method). High sequence identity to other Wolbachia suggests Wolbachia infection. Clean chromatograms are consistent with a single Wolbachia strain. Otherwise, a cloning step to identify different Wolbachia alleles is required. Other genes are then amplified and sequenced for further genetic characterization of the strain. As an optional step, localization of Wolbachia cells within host tissues can be achieved by Fluorescent In Situ Hybridization (FISH) with Wolbachia-specific rRNA probe or immunolabeling with antibody specific to Wolbachia protein. An amplicon of an unexpected size might indicate the occurrence of a horizontally transmitted Wolbachia genome fragment to the insect chromosome, rather than a current infection. Similarly, multiple nucleotide polymorphisms (NP) or insertions/deletions, compared to known strains, are suggestive of Wolbachia pseudogenes (e.g., horizontally transferred to host genome). This can be further tested by in situ hybridization of Wolbachia-specific probe to host chromosomes, and/or by Whole Genome Sequencing of host. Photo of fly (Anastrepha obliqua) by Fabiola Roque (ECOSUR-UT). Image from Fast et al. (2011) freely available at https://www.ncbi.nlm.nih.gov/pmc/articles/PMC4030408/bin/NIHMS391830-supplement-Supporting_Online_Material.pdf (accessed April 01, 2019). Original sources of other photographs are available in open access journals (Doudoumis et al., 2012; Brelsfoard et al., 2014; Joubert et al., 2016).
The two major shortcomings of utilizing solely PCR (or LAMP) to detect Wolbachia presence are the occurrence of false negatives and false positives. A false negative occurs when a specimen is infected by Wolbachia, yet the screening approach fails to detect its presence. The efficiency of the PCR can be affected by the presence of inhibitors (Marcon et al., 2011; Beckmann and Fallon, 2012), by low concentration/poor quality of the target DNA molecule, as well as type and concentration of the polymerase and other PCR reagents. At the very least, negative Wolbachia detection PCRs should be validated by evaluating the quality of the DNA extract, through positive amplification of a host-encoded gene (e.g., the mitochondrial Cytochrome Oxidase subunit I or single-copy nuclear genes). Several higher sensitivity approaches have been devised, particularly for low-titer infections, such as: long PCR (Jeyaprakash and Hoy, 2000); nested PCR (Arthofer et al., 2009a); quantitative PCR (Mee et al., 2015); or the design of alternative and/or more specific primers, including the use of Wolbachia multi-copy genes as PCR targets (Schneider et al., 2014). These methods, however, have not been widely implemented, likely due to the higher effort or cost involved.
False positives occur when a specimen not harboring Wolbachia is identified as Wolbachia-infected. Several instances have been reported where insect chromosomes carry Wolbachia-derived fragments, presumably from a horizontal gene transfer event that occurred at some point in the host lineage as the result of an active infection that was subsequently lost. The size of the horizontally transmitted fragment can range from ca. 500 bp to the equivalent of an entire Wolbachia chromosome (Dunning Hotopp et al., 2007). In some cases, entire Wolbachia chromosomes have been transferred more than once onto the same host genome (Brelsfoard et al., 2014; International Glossina Genome Initiative, 2014). The range of hosts carrying Wolbachia-derived genome fragments is broad and includes several dipterans (tephritids, Glossina morsitans Westwood; Drosophila spp., mosquitoes), other insects, as well as nematodes (Fenn et al., 2006; Dunning Hotopp et al., 2007; Nikoh et al., 2008; Brelsfoard et al., 2014; Morrow et al., 2015; Attardo et al., 2019). It is therefore desirable to corroborate PCR-based inferences with approaches that detect Wolbachia cells in host tissues. Such microscopy approaches can be based on nucleic acid hybridization (e.g., Chen et al., 2005) or antibody-based detection of Wolbachia proteins (e.g., wsp; Veneti et al., 2003; and ftsZ; Newton et al., 2015). A major drawback of these methods is that they require substantial investment in time and equipment compared to PCR-based approaches. False positives can also occur if the primers targeted at Wolbachia turn out to amplify a fragment of the genome of the host (not derived from Wolbachia) or of another symbiont of the host. Such false positives are relatively easy to rule out upon sequencing and analysis of the amplified product. Finally, as with any PCR work, false positives can result from contamination of the specimen, the DNA template, or the PCR reagents. Thus, it is important to implement adequate sterile practices and negative controls.
The above approaches require destruction of specimens for DNA isolation or for tissue fixation. As a rapid and non-destructive alternative, Near-Infrared Spectroscopy (NIR) has been developed for identification of specimens infected with Wolbachia, including the distinction of two different Wolbachia strains (Sikulu-Lord et al., 2016). This method, however, requires standardization according to species, sex, age, or any other condition that may affect absorbance, and is not 100% efficient. To our knowledge, this method has not been employed to assess Wolbachia infection in tephritids.
Methods to Taxonomically Characterize Wolbachia Strains
The main evolutionary lineages of Wolbachia are assigned to “supergroups” (Zhou et al., 1998). Sixteen supergroups have been recognized to date (Glowska et al., 2015; Bleidorn and Gerth, 2017). Supergroups A and B are widespread in arthropods and are common reproductive manipulators (Baldo et al., 2006; Werren et al., 2008). Supergroups C and D are obligate mutualists of filarial nematodes, whereas supergroup F is found in both arthropods and nematodes (Ros et al., 2009). Other supergroups have more restricted host distributions (Augustinos et al., 2011). Wolbachia are generally compared and classified on the basis of Multilocus Sequence Typing (MLST) systems (Baldo et al., 2006; Paraskevopoulos et al., 2006). The most commonly used MLST is based on the PCR amplification of fragments of five ubiquitous genes: coxA, fbpA, ftsZ, hcpA, and gatB. However, this MLST system has limitations, in that not all genes are readily amplified in all Wolbachia strains, and it fails to distinguish among very closely related strains (Augustinos et al., 2011; Bleidorn and Gerth, 2017). Several additional genes commonly amplified and reported are the 16S rRNA, groEL, gltA, and the wolbachia surface protein (wsp) (O’Neill et al., 1992; Braig et al., 1998; Zhou et al., 1998; Augustinos et al., 2011). The wsp gene is highly variable and shows evidence of intragenic recombination (Werren and Bartos, 2001; Ros et al., 2012). An MLST database1 is available to compare sequences of alleles for the five MLST loci and the wsp gene. Upon submission to the MLST database, new alleles for the wsp and for each of the MLST loci are assigned a unique number. A Wolbachia sequence type (ST) is defined on the basis of MLST allele combinations, with each allele combination assigned a unique ST number. Further characterization of each MLST-defined strain can be achieved by examination of four hypervariable regions (HVRs) of the wsp gene (Baldo et al., 2005).
Hosts can be infected by one or more distinct strains of Wolbachia. Traditionally, direct Sanger sequencing of PCR products that resulted in sequences with ambiguous base pairs would be subjected to cloning followed by sequencing. The allele intersection analysis method (AIA; Arthofer et al., 2011) can then be used to assign MLST alleles to Wolbachia strains, but it requires a priori knowledge on the number of strains present. AIA identifies pairs of multiply infected individuals that share Wolbachia and differ by only one strain. Alternative approaches to circumvent cloning include the use of strain-specific primers (e.g., for the wsp gene; Zhou et al., 1998; Arthofer et al., 2009b), or of high throughput sequencing approaches (e.g., Illumina HiSeq, MiSeq, and NovaSeq) to sequence MLST or other marker PCR amplicons (e.g., Gibson et al., 2014; Brandon-Mong et al., 2015). Primer bias, however, where the fragment of one Wolbachia strain is preferentially amplified over the other, has been reported (Arthofer et al., 2011), such that presence of certain Wolbachia strains might be missed.
Use of the MLST system alone has two major drawbacks. First, strains of Wolbachia sharing identical MLST or wsp alleles can differ from each other at other loci (Paraskevopoulos et al., 2006; Riegler et al., 2012). Secondly, the MLST, 16S rRNA, and wsp loci contain limited phylogenetic signal for inferring relationships within Wolbachia supergroups (Bleidorn and Gerth, 2017). Therefore, to assess such intra-ST variation and to infer evolutionary relationships among closely related Wolbachia strains, additional (more variable) loci must be evaluated. The multiple locus variable number tandem repeat analysis developed by Riegler et al. (2012) allows distinction of closely related Wolbachia strains based on PCR and gel electrophoresis.
Whole genome sequencing represents a powerful approach to distinguish closely related Wolbachia strains, infer their evolutionary relationships, test for recombination, and identify genes of interest (e.g., Klasson et al., 2009; LePage et al., 2017). Due to its fastidious nature (but see Uribe-Alvarez et al., 2018 for a recent breakthrough) and occurrence of repetitive elements, genome sequencing and assembly of Wolbachia (and other host-associated uncultivable bacteria) has proven difficult. Recent advances, particularly those based on targeted hybrid enrichment (Lemmon and Lemmon, 2013) prior to high-throughput sequencing (Bleidorn, 2016; Goodwin et al., 2016) have been successfully applied to Wolbachia for short-read technologies (i.e., Illumina; Kent et al., 2011; Geniez et al., 2012). The combination of targeted hybrid capture and long-read technologies, such as Pacific Biosciences’ Single Molecule Real-Time (e.g., Wang et al., 2015) or Oxford Nanopore Technologies’ platforms is expected to greatly advance Wolbachia genomics research.
Methods to Functionally Characterize Wolbachia Strains
A major challenge to investigating the effects of Wolbachia on a host is to generate Wolbachia-present and Wolbachia-free treatments while controlling for host genetic background. The challenge stems from the difficulty of adding or removing Wolbachia to/from particular hosts. Addition of Wolbachia to a particular host background can be achieved by transinfection (reviewed in Hughes and Rasgon, 2014). Because the vertical transmission of Wolbachia appears to be dependent on its close association to the host germline, successful artificial transfer of Wolbachia typically relies on injection of cytoplasm from a donor egg (but see Frydman et al., 2006) or early embryo into a recipient embryo via microinjection (reviewed in Hughes and Rasgon, 2014). The success rate of the transinfection procedure is generally very low; in tephritids it is 0–0.09% (calculated as the proportion of injected embryos that emerged as Wolbachia-infected adult females that transmitted Wolbachia to offspring) (Zabalou et al., 2004b, 2009; Apostolaki et al., 2011; Martinez et al., 2016). The low success rate is generally a result of the low survival of injected embryos, the low proportion of Wolbachia-positive survivors, and the low/incomplete transmission of Wolbachia to their offspring.
Intra-species (or between sibling species) transfer of Wolbachia to a particular host nuclear background can also be achieved through introgression, whereby males of the desired background are repeatedly backcrossed with Wolbachia-infected females (e.g., Dobson et al., 1999; Jaenike, 2007). Under this scheme, after eight generations of consistent backcrossing, ∼99.6% of the host nuclear background is expected to have been replaced (Figure 3). The drawback of this approach is that the mitochondrial genome will not be replaced. Therefore, the effects of mitochondrial type and Wolbachia infection cannot be separated.
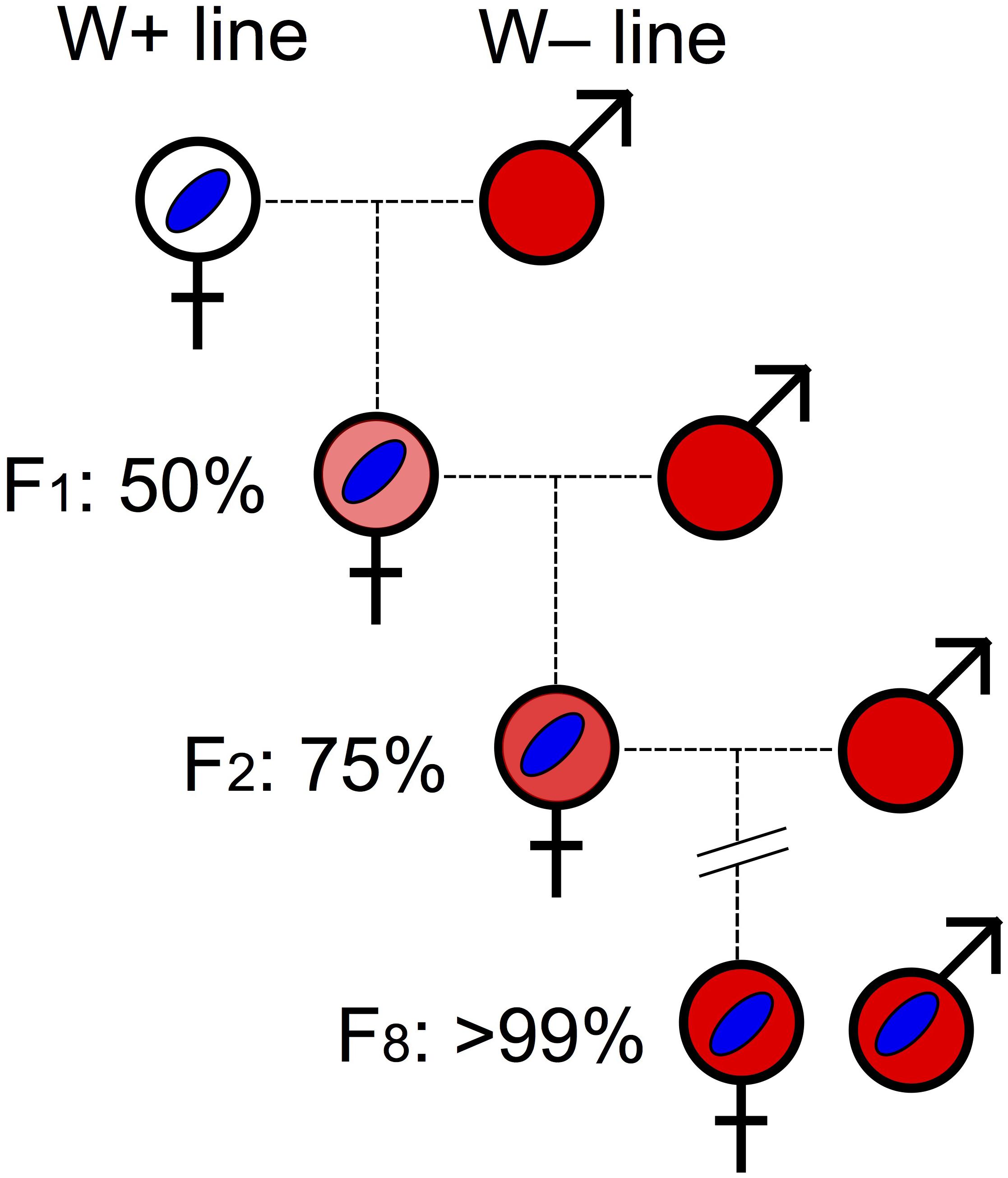
Figure 3. Backcrossing procedure. Wolbachia infection is indicated by blue oval. Host nuclear backgrounds are indicated by colors: white represents the initial nuclear background of Wolbachia-infected host; red (darkest) indicates the host background of the Wolbachia-free line contributing males every generation. Different shades of red represent the increasing replacement of “white” nuclear background over backcrossing generations (F1 to F8) by “red” nuclear background.
Due to less than perfect transmission, passive loss of Wolbachia in certain host individuals may be used to obtain Wolbachia-free and Wolbachia-infected hosts of equivalent genetic background. Wolbachia removal has also been achieved by “extreme” temperature treatment (e.g., 30°C; Ribeiro, 2009). The most common way of removing Wolbachia, however, is achieved via antibiotic treatment, but several potential biases must be addressed (reviewed by Li et al., 2014). Antibiotic treatment is likely to alter the microbiota, other than Wolbachia, associated with the host. In addition, antibiotics may affect the host in a microbe-independent manner. For instance, antibiotic treatment can affect host mitochondria (Ballard and Melvin, 2007), which in turn can reduce host fitness. A common practice to circumvent these problems is to wait several generations after antibiotic treatment, and to promote “restoration” of the host’s pre-antibiotic microbiota, excluding Wolbachia (e.g., exposing the insects to the feces of non-treated individuals). Wolbachia does not appear to be efficiently transmitted via ingestion (e.g., Faria et al., 2016), but see discussion on horizontal transmission routes below. It is essential to monitor Wolbachia infection status of antibiotic-treated host strains, because antibiotics may not always fully remove infection. Instead, they may reduce Wolbachia densities to non-detectable levels in one or few generations (Li et al., 2014); this has been our experience in both Anastrepha (S.B. Lanzavecchia, C. Conte, and D.F. Segura, pers. obs.) and Drosophila (M. Mateos, pers. obs.).
Unidirectional CI is tested by comparing the embryo hatching rates of the CI cross (uninfected female X infected male) to that of one or more control crosses. For testing bidirectional CI, the reciprocal crosses of hosts infected by the different Wolbachia strains are assessed. A significantly lower embryo hatching rate of the CI cross(es) compared to that of the control cross(es) constitutes evidence of CI. CI can be partial or complete (100% embryo failure). As with any fitness assay, care must be taken to prevent potential biases, including crowding and age effects; which have been shown to influence CI (Hoffmann et al., 1990; Turelli and Hoffmann, 1995; Reynolds and Hoffmann, 2002). Adequate assessment of fertilization must be performed to ensure that failed embryos are not confused with unfertilized eggs. This may require testing for insemination of females that produce no larval progeny (e.g., Zabalou et al., 2009; Conte et al., 2019), or exclusion of females that predominantly lay unfertilized eggs, such as old Drosophila melanogaster virgin females (Menon et al., 2014).
Wolbachia in Tephritids
Taxonomic Distribution of Wolbachia-Tephritid Associations
Based mostly on PCR and sequencing approaches, ∼66% of ∼87 tephritid species screened have at least one record of positive Wolbachia infection (excluding pseudogenes) in laboratory and natural populations (see Supplementary File S1; only supergroups A and B have been found in tephritids). For the genus Anastrepha, all but one species (Anastrepha ludens) of 17 screened to date harbor Wolbachia (Werren et al., 1995; and this study; Selivon et al., 2002; Coscrato et al., 2009; Martínez et al., 2012; Mascarenhas et al., 2016; Morán-Aceves, 2016; Prezotto et al., 2017; Conte et al., 2019; Devescovi et al., 2019). Most Anastrepha species harbor Wolbachia strains assigned to supergroup A. Anastrepha striata Schiner and Anastrepha serpentina (Wiedemann), however, harbor supergroup B in southern Mexico (Martínez et al., 2012; and H. Martinez and M. Mateos, pers. obs.; see Supplementary File S1) and supergroup A in Brazil (Coscrato et al., 2009). Up to three Wolbachia sequence types have been detected per locality within morphotypes of the A. fraterculus complex (Prezotto et al., 2017; Conte et al., 2019), but co-infection of a single individual is generally not observed (except for one report in A. fraterculus; Cáceres et al., 2009).
Of the ∼49 species of Bactrocera that have been examined, ∼14 are reported to harbor Wolbachia (supergroup A and/or B) and three (Bactrocera peninsularis Drew & Hancock, Bactrocera perkinsi Drew & Hancock, and Bactrocera nigrofemoralis White & Tsuruta) carry what appear to be Wolbachia-derived pseudogenes, but not active infections (Kittayapong et al., 2000; Jamnongluk et al., 2002; Morrow et al., 2014, 2015). There is also the case of Bactrocera zonata Saunders, B. dorsalis, and Bactrocera correcta Bezzi that have been found to carry both active infections (cytoplasmic) and pseudogenized Wolbachia sequences (Asimakis et al., 2019). Up to five Wolbachia strains have been reported in a single individual of Bactrocera ascita Hardy (Jamnongluk et al., 2002), and double/multi infections have been reported in individuals of the following five Bactrocera species in Australia: Bactrocera bryoniae Tryon; Bactrocera decurtans May; Bactrocera frauenfeldi Schiner; Bactrocera neohumeralis Hardy; and Bactrocera strigifinis Walker (Morrow et al., 2014, 2015). Within the genus Bactrocera, polyphagous species are more likely to harbor Wolbachia compared to stenophagous or monophagous ones (Kittayapong et al., 2000).
For the genus Ceratitis, two species have been screened for Wolbachia. No evidence of Wolbachia was found in Ceratitis fasciventris Bezzi. Also, no evidence of infection was found in several populations of C. capitata, the Mediterranean fruit fly (medfly), in the early ‘1990s’ (Bourtzis et al., 1994). PCR amplification and sequencing of the 16S rRNA gene in several field and lab specimens of C. capitata from Brazil suggested infection with Wolbachia supergroup A (Rocha et al., 2005). However, recent thorough surveys of wild populations and lab colonies indicate that Wolbachia is absent in C. capitata from numerous localities in different continents (Supplementary File S1).
Wolbachia is reported in the four species of Rhagoletis examined to date: Rhagoletis cerasi L.; Rhagoletis pomonella Walsh; Rhagoletis cingulata Loew; and Rhagoletis completa Cresson (Zabalou et al., 2004b; Arthofer et al., 2009b; Drosopoulou et al., 2011; Schuler et al., 2011, 2012, 2013, 2016b; Augustinos et al., 2014; Bakovic et al., 2018). Both A and B supergroups are found in R. cerasi and R. completa, including a putative A–B recombinant strain, and co-infections are common (e.g., R. cerasi and R. pomonella).
In Zeugodacus (formerly Bactrocera), both Z. cucurbitae and Z. diversa are reported to harbor Wolbachia or Wolbachia pseudogenes (Kittayapong et al., 2000; Jamnongluk et al., 2002; Asimakis et al., 2019). Two out of the six species of Dacus examined to date are reported to harbor Wolbachia: Dacus axanus Hering (Morrow et al., 2014); and Dacus destillatoria Bezzi (Jamnongluk et al., 2002). Wolbachia has not been detected in the monotypic genus Dirioxa (Morrow et al., 2015). Wolbachia (supergroup A) has been reported in Carpomya vesuviana (Karimi and Darsouei, 2014) and Neoceratitis asiatica (Wang et al., 2019).
Wolbachia Prevalence in Tephritids (in Time/Space)
Numerous studies report Wolbachia infection frequencies (or data from which this measure can be estimated) in natural populations of tephritids. Few of these studies, however, have adequate sample sizes for such inferences (e.g., many such studies are based on 10 or fewer individuals). Notwithstanding, inferred Wolbachia prevalence in tephritid populations is highly variable. In Anastrepha, ∼10 species harbor at least one population with prevalence ∼100%, whereas populations of three species reported lower frequencies (e.g., 88%, 51–60%, and 8.7%) (Supplementary File S1). In Bactrocera, one population of B. caudata had 100% prevalence, whereas all other species with positive Wolbachia results exhibited low prevalence.
The best studied tephritid system in terms of spatial and temporal variation in Wolbachia prevalence is that of R. cerasi in Europe, which was surveyed over a ∼15-year-period in 59 localities (Schuler et al., 2016b). Collectively, at least six strains wCer1–6 have been identified from Europe and the Middle East. In an early (1998) survey, Riegler and Stauffer (2002) found all European R. cerasi individuals infected by one strain (wCer1), most central and southern European populations harbored an additional strain wCer2 (i.e., wCer1 + wCer2), and at least one Italian population harbored wCer1 + wCer4 (Zabalou et al., 2004b). A rapid spread of wCer2 (a strain associated with cytoplasmic incompatibility) has been detected. Multiple infections, in various combinations of all five known Wolbachia strains from Europe, have been revealed recently. Samples from Poland, Italy, and Austria, are infected with strains wCer1–5 those from Czech Republic (prior to 2009) and Portugal lacked wCer2 only, while the Swiss samples lacked wCer3 (Arthofer et al., 2009b). A more recent study of the Czech Republic (2015) and Hungary (2016) revealed that wCer2 is spreading at a speed of 1.9 and 1 km/yr, respectively (Bakovic et al., 2018). Analysis of 15 Greek, two German and one Russian population confirm fixation for wCer1 in all R. cerasi populations, and the presence of complex patterns of infections with four of the five known wCer European strains (1, 2, 4, and 5) and the possible existence of new Wolbachia strains for the southernmost European R. cerasi population (i.e., Crete; Augustinos et al., 2014) and from Iran (wCer6) (Karimi and Darsouei, 2014). Similarly, strain wCin2 (which is identical to wCer2 based on loci examined to date) is fixed in all populations of R. cingulata; a species native to the United States, but introduced into Europe at the end of the 20th century. Invasive populations in Europe harbor wCin1 (identical to wCer1 based on loci examined to date) at frequencies that vary over space and time (up to 61.5%), as a result of horizontal transfer (multiple events) from R. cerasi (Schuler et al., 2016b). The above studies indicate that the prevalence of Wolbachia types in R. cerasi and R. cingulata is highly dynamic.
Phenotypic Effects of Wolbachia in Tephritids
Despite the numerous reports of Wolbachia in tephritids, the fitness consequences of such associations remain mostly unknown. The studies reporting phenotypic effects of Wolbachia have relied on transinfection and on antibiotic-curing; only two species of tephritids have been successfully transinfected with Wolbachia (Table 1). Evidence of Wolbachia-induced CI has been detected in four species of tephritids. Early studies (Boller and Bush, 1974; Boller et al., 1976) identified reproductive incompatibilities in R. cerasi that were later attributed to the Wolbachia strain wCer2 (100% embryonic mortality in the CI cross; Riegler and Stauffer, 2002). Artificially transferred Wolbachia (strains wCer2 and wCer4) originally from R. cerasi to C. capitata also resulted in strong CI (100% embryonic mortality). wCer2 in two genetic backgrounds of B. oleae resulted in strong CI as well (Apostolaki et al., 2011). In addition, wCer2 and wCer4 are bi-directionally incompatible in C. capitata (Zabalou et al., 2004b, 2009).
In addition to CI, Wolbachia-infected C. capitata females (Benakeio strain) exhibit higher embryonic mortality (17–32% in crosses with Wolbachia-free males and 65–67% in crosses with Wolbachia-infected males) than their Wolbachia-free counterparts crossed with Wolbachia-free males (12% embryonic mortality). Therefore, it appears that wCer2 and wCer4 have additional fertility effects on medfly females, other than CI. It is also possible that these Wolbachia strains can only partially rescue the modification that they induce in sperm (Zabalou et al., 2004b). A similar pattern is reported in the Vienna 8 genetic sexing strain (GSS) infected with wCer2 (Zabalou et al., 2009). The wCer2 strain also causes increased embryo death in non-CI crosses of B. oleae (Apostolaki et al., 2011). In D. simulans, wCer2 causes fecundity costs, moderate levels of CI, and incomplete rescue of its own CI modification (Riegler et al., 2004). Interestingly, a recent study examined the genome of wCer2 and revealed the presence of three pairs of Type I cif genes and one Type IV cifB gene without a cifA complement, which might explain its idiosyncratic expression of CI (Morrow et al., 2020).
Two studies conducted several years apart (Sarakatsanou et al., 2011; Kyritsis, 2016; Kyritsis et al., 2019) examined the effects of a single Wolbachia strain (wCer2) on fitness components of two C. capitata genotypes (i.e., Benakeio and Vienna 8 GSS laboratory lines), as well as the effects of two different Wolbachia strains (wCer2 and wCer4) on a single medfly genotype (Benakeio). The following general patterns emerged (exceptions noted): (a) Wolbachia causes higher egg-to-larva mortality; (b) Wolbachia causes higher egg-to-adult mortality (exception: Vienna 8 GSS + wCer2 in Sarakatsanou et al., 2011); (c) Wolbachia shortens egg-to-adult development time (exception: Benakeio + wCer2 in Kyritsis, 2016; Kyritsis et al., 2019). In addition, Sarakatsanou et al. (2011) found that Wolbachia shortens both male and female adult lifespan (exception: males of Vienna 8 GSS and wCer2), and reduces life time female net fecundity. However, Kyritsis (2016) and Kyritsis et al. (2019) reported no effects of Wolbachia infection on adult lifespan, and a reduced fecundity in the case of wCer4 infection only. Even though wCer2 and wCer4 in general tended to have consistent effects on medfly, the magnitude of their effects differed. Collectively, the results from these studies indicate that the effect of Wolbachia infection on life history traits depends both on the C. capitata genetic background and on the Wolbachia strain. Furthermore, inconsistencies between the two studies might be indicative of evolution of the host and/or Wolbachia strain during that period. Evidence of Wolbachia evolving reduced fitness costs has been reported in D. simulans (Weeks et al., 2007). Adult flight ability and longevity under stress conditions also appear to be determined by the interaction of Wolbachia strain and medfly genotype (Kyritsis, 2016; Kyritsis et al., 2019). A more recent study (Dionysopoulou et al., 2020) demonstrated Wolbachia effects on medfly reared in natural host fruits and at different temperatures. Medlfies infected with wCer4 had low survival rates in both apples and bitter oranges, whereas those infected with wCer2 were less vulnerable in apples than in bitter oranges. In addition, wCer4 infected flies were particularly susceptible to high temperatures.
A recent study by Conte et al. (2019) examined the phenotypic effects induced by two Wolbachia strains native to A. fraterculus (sp1). No evidence of bidirectional cytoplasmic incompatibility was detected in reciprocal crosses among singly infected laboratory strains. However, the same work described the presence of slightly detrimental effects on larval survival and a female-biased sex ratio, suggesting the induction of male-killing phenomena. Moreover, Devescovi et al. (2019) found that Wolbachia reduced the embryo hatching in crosses involving cured females and infected males (uni-directional CI) within two morphotypes of this cryptic species complex; stronger CI was detected within the Peruvian morphotype than the Brazilian-1 morphotype (also referred as to “A. fraterculus sp. 1”). No evidence of bidirectional CI was detected in the crosses between the two morphotypes, leading Devescovi et al. (2019) to conclude that Wolbachia is not directly involved in the speciation process of these morphotypes. Ribeiro (2009) reported evidence consistent with CI caused by Wolbachia in A. obliqua and in “A. fraterculus sp. 1,” which according to wsp sequences, are identical. Nonetheless, confounding effects of the treatment to remove Wolbachia (removed by exposure of pupae to 30°C) or other potential biases cannot be ruled out, as all intraspecific crosses involving at least one cured parent resulted in much lower (<30%) embryo hatching than the intraspecific crosses involving both infected parents (66 and 81% embryo hatching).
Recent work demonstrates that Wolbachia infection can affect male sexual competitiveness of C. capitata. Different Wolbachia strains (wCer2 and wCer4) exerted differential impact on males mating competitiveness, and a single strain (wCer2) had different impact on different medfly genotypes (Benakeio and Vienna 8 GSS laboratory lines) (Kyritsis, 2016; Kyritsis et al., 2019).
Modes of Horizontal Transmission of Wolbachia Between Tephritid Hosts
Considering the dynamics of Wolbachia associated with arthropods in general, at the population level Wolbachia appears to be predominantly maintained by vertical transmission. Above the species level, however, the lack of congruence between the host and symbiont phylogenetic trees implies that Wolbachia horizontal transfers and extinctions are common and underlie its widespread taxonomic and geographic distribution (Bailly-Bechet et al., 2017).
The possible routes by which Wolbachia may be horizontally acquired by a new host can generally be classified as via ingestion or via a vector. In both cases, to become established as a stable cytoplasmically inherited infection, Wolbachia must cross one or more cell types or tissues. For example, if Wolbachia invaded the host hemolymph directly as a result of a vector (e.g., parasitoid wasp or ectoparasitic mite), it would have to invade the egg during oogenesis. Similarly, if Wolbachia were acquired via ingestion (e.g., as a result of scavenging), it would have to cross the gut into the hemolymph, before it invaded the egg. Support for the above routes comes from studies reporting: (a) that Wolbachia can retain viability outside cells and infect mosquito cell lines, as well as ovaries and testes that are maintained ex vivo (Rasgon et al., 2006; Hughes et al., 2012a); (b) that Wolbachia cells injected into Drosophila hemolymph reach the germline after crossing multiple somatic tissues (Frydman et al., 2006); (c) that Wolbachia can move between parasitic wasp larvae (Trichogramma) sharing the same host egg, and achieve vertical transmission (Huigens et al., 2004); and (d) that parasitic wasps of the white fly, Bemisia tabaci (Gennadius), can transfer Wolbachia from an infected to a naïve host, as a result of non-lethal probing (i.e., probing without oviposition), whereby the parasitoid ovipositor or mouthparts function as a “dirty needle” (Ahmed et al., 2015).
No direct evidence of Wolbachia transmission via parasitoids exists in tephritids, but sharing of Wolbachia strains between a parasitoid and several sympatric tephritids (Morrow et al., 2014; Mascarenhas et al., 2016) is consistent with parasitoid-mediated transmission, or transmission from tephritid host to parasitoid (Johannesen, 2017). The potential for horizontal transfer of Wolbachia among tephritids via parasitoids is high, due to the multiple instances where a single parasitoid utilizes several different tephritid host species (Quilici and Rousse, 2012; Murillo et al., 2016; Schuler et al., 2016a), and the high frequency of superparasitism by some fruit fly parasitoids (Tormos et al., 2012; Devescovi et al., 2017).
Wolbachia may invade a new host species via introgressive hybridization between two host species. This mechanism would also transfer mitochondria from the infected to the uninfected species nuclear background, akin to the artificial backcrossing approach described above (Figure 3). Ability of tephritids to hybridize in the lab has been reported in numerous species (Table 2), and hybridization in nature has been documented in B. dorsalis/B. carambolae (Wee and Tan, 2005), members of the Ceratitis FAR complex (Virgilio et al., 2013), and R. cingulata/R. cerasi in Europe (Johannesen et al., 2013). Thus, there is potential for wild tephritid populations to acquire Wolbachia infections via hybridization.
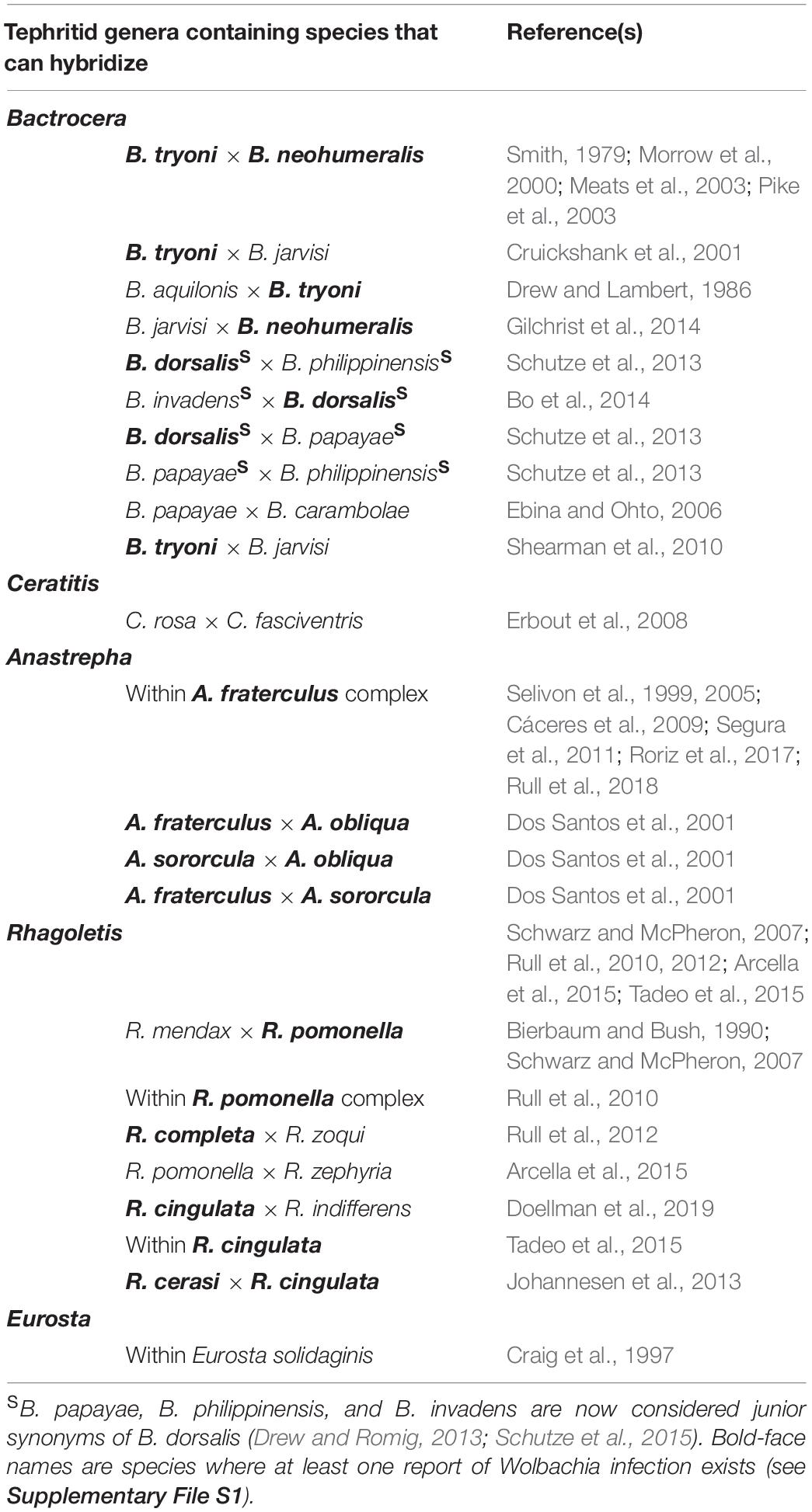
Table 2. Representative tephritid genera where hybridization between one or more species has been reported.
Considerations for Wolbachia-Based Iit in Tephritids
There are two main approaches for implementing IIT, which depend on whether uni- or bi-directional CI will be used. If the target pest population lacks Wolbachia, such as the tephritids C. capitata, B. oleae (Gmelin), and A. ludens [and the mosquito Aedes aegypti (L.)], only unidirectional CI is feasible. In target populations that harbor one (or more) CI-inducing Wolbachia strain(s) (i.e., native strain; yellow in Figure 4), bi-directional CI can be achieved by releasing males that lack the native strain(s) and harbor one (or more) “foreign” Wolbachia strain(s) (blue in Figure 4) that is incompatible with the native strain. In contrast, if the released males are doubly infected with the native and foreign strains, the CI pattern employed for population suppresion is uni-directional (Figure 4).
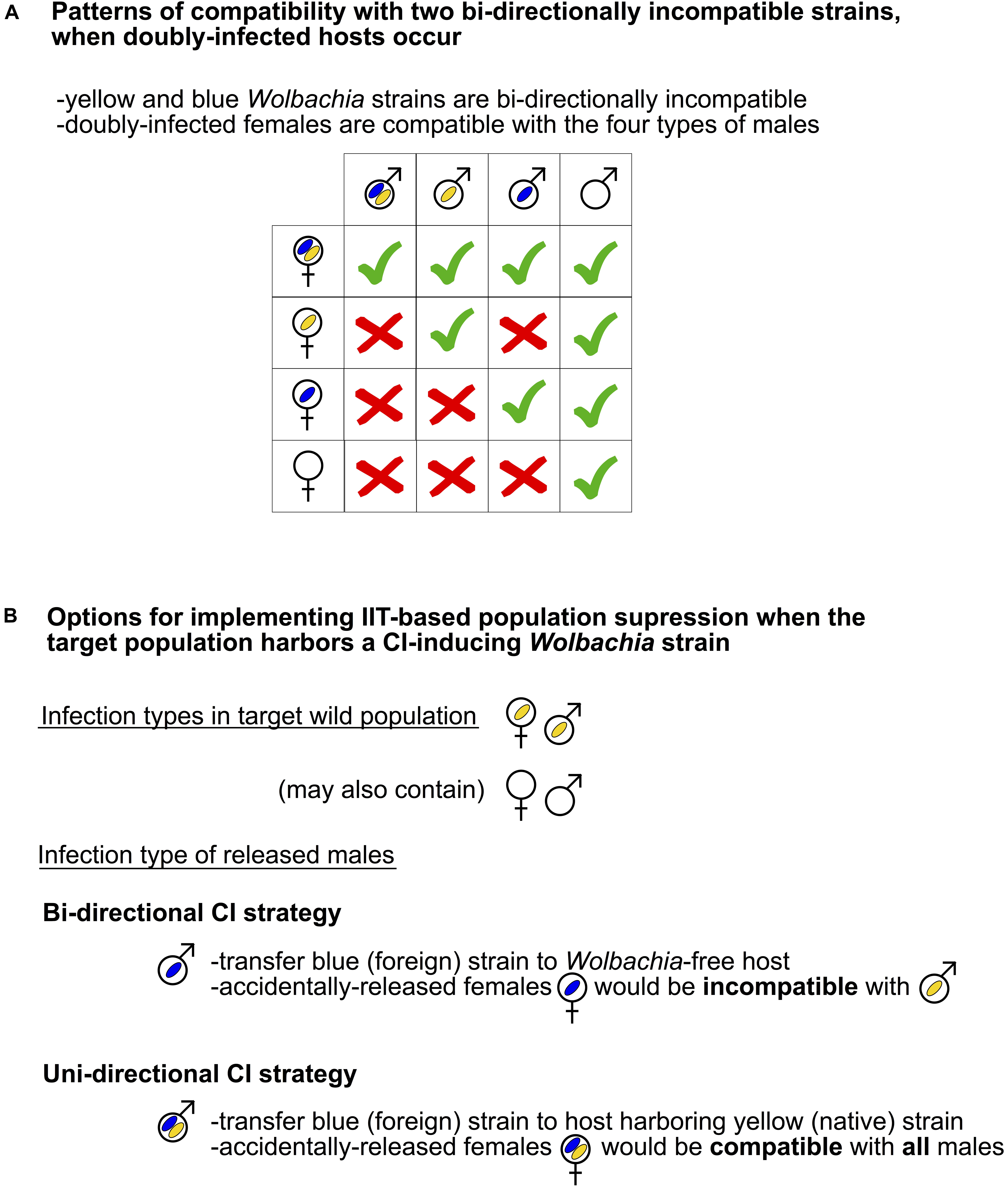
Figure 4. Use of bi-directional CI in IIT-based population suppression programs. (A) Patterns of compatibility with two bi-directionally incompatible strains, when doubly infected hosts occur. Empty male and female symbols signify absence of Wolbachia. Blue and yellow ovals represent distinct (mutually incompatible) Wolbachia strains. Green tick marks = Successful offspring production. Red crosses = no offspring production. (B) Options for implementing IIT-based population suppression when the target wild population harbors a CI inducing strain (yellow = “native”), according to the patterns of compatibility depicted in panel a. Bi-directional CI is achieved when the released males only harbor a strain (blue = “foreign”) that is incompatible with the native strain. Additional options exist, including double infections of both target and released insects with different Wolbachia strains (not shown), such as in Aedes albopictus (Moretti et al., 2018b).
In the case of Uni-CI patterns, the accidental release of Wolbachia-infected females, which would be reproductively compatible with wild and released males, may result in the replacement of the target pest population with a population harboring the Wolbachia infection of the released males, leading to failure of the IIT-based suppression program (Bourtzis, 2008). As described in Section “The Influence of Wolbachia on Host Ecology,” under certain conditions, a Wolbachia infection with frequency close to zero might be able to rapidly spread through a host population. Thus, without efficient sex separation mechanisms (outlined in section below), it is desirable to ensure that accidentally released females are sterile. In several tephritid systems, female sterility is achieved at a lower irradiation dose than male sterility, such as A. ludens, A. obliqua, Anastrepha suspensa, A. serpentina, B. tryoni, and Z. curcubitae (Toledo et al., 2004; Bakri et al., 2005; Rull et al., 2007; Collins et al., 2009). Therefore, an IIT program that used radiation at doses to ensure female sterility without compromising male quality (e.g., male competitiveness) could be effective (e.g., Drosophila suzukii based on results to date; Nikolouli et al., 2020).
In an IIT program based on bi-CI pattern (e.g., the recent field study of Aedes albopictus; Caputo et al., 2019), accidental release of fertile transinfected females, which would only be compatible with the released males, would not necessarily lead to population replacement and program failure. This is due to the generally higher threshold density required to achieve invasion (theoretically above 50% when the two incompatible Wolbachia strains exert equivalent fitness costs/benefits; see Section “The Influence of Wolbachia on Host Ecology”). Nonetheless, the actual outcome is strongly dependent in multiple factors (see Dobson et al., 2002; Moretti et al., 2018a). Therefore, for both uni-CI- and bi-CI-based IIT programs, as pointed out by Bourtzis et al. (2014), the outcome of accidental releases of infected females must be thoroughly evaluated via modeling and/or semi-field assays prior to field applications.
The Advantage of Genetic Sexing Strains (GSS)
In general, SIT and IIT are most effective when only males are produced and released (Kerremans and Franz, 1995; Rendón et al., 2004). The release of only males in a large-scale operation can be accomplished by either killing female zygotes during development or by selectively removing them from the mass-reared population prior to release (Robinson, 2002a; Gilles et al., 2014; Lutrat et al., 2019). Genetic sexing strains (GSS) are those in which individuals can be separated by sex prior to the adult stage on the basis of a sex-linked phenotype (Franz, 2005). The earlier in development the females are removed, the most cost-effective the mass rearing operation will be, as investment in growth of females would be null or minimized. In most tephritids, male sex is determined by the presence of the maleness factor on the Y chromosome (Pane et al., 2002). GSS based on male-linked [e.g., Y chromosome – autosome (Y;A)] translocations have been developed in a few species to produce conditional female lethality (e.g., temperature sensitive lethality during embryonic development) or a visual sex marker (e.g., pupal color). Examples of tephritid species for which GSS are available include C. capitata (Franz, 2005), A. ludens (Zepeda-Cisneros et al., 2014), Z. cucurbitae (McInnis et al., 2004), B. dorsalis (Isasawin et al., 2012), and B. carambolae (Isasawin et al., 2014). Unfortunately, despite substantial efforts, GSS are still lacking for most tephritid pests. The recent development of CRISPR/Cas9-mediated mutagenesis in tephritids, however, might enable a faster development of tephritid GSS (Reid and O’Brochta, 2016; Choo et al., 2017; see reviews by Ogaugwu and Durvasula, 2017).
Choice and Evaluation of Wolbachia Strains
The target population and the donor colony should be thoroughly screened for Wolbachia, ideally with the higher sensitivity methods described in Section “Methods to Assess Wolbachia Infection Status,” to detect low-titer and multi-strain infections. The Wolbachia strain selected should cause strong uni-CI with a Wolbachia-free, or strong bi-CI with Wolbachia-infected, target population. The selected Wolbachia strain should be artificially transferred to one or more lab colonies, representative of the genetic background of the target pest population. Most cases of successful establishment of stable transinfected insect lines have relied on embryonic microinjection (Hughes and Rasgon, 2014). Introgressive backcrossing might be feasible in scenarios where geographically isolated populations of the same target species harbor distinct Wolbachia strains (e.g., A. striata in Mexico vs. A. striata in Brazil; Supplementary File S1).
A thorough biological characterization of the artificial host-Wolbachia association should be conducted, as both host background and Wolbachia strain are important determinants of CI expression and other relevant fitness parameters (Bourtzis and Robinson, 2006; reviewed in Bourtzis, 2008; see also Kyritsis et al., 2019). The main desired characteristics of the association are: strong induction of CI; no rescue by Wolbachia strain(s) present in the target population; small or no fitness cost for parameters relevant to the program. These fitness parameters can be classified into those related to a cost-effective mass production (e.g., female fecundity including embryo hatching success) and those related to the success of released males (e.g., mating and sperm competitive ability, as well as dispersal/flight ability). Some host-Wolbachia combinations result in higher female fecundity, such as D. simulans after many generations (Weeks et al., 2007) and Drosophila mauritiana Tsacas and David (Fast et al., 2011). In contrast, other host-Wolbachia combinations result in lower fertility (e.g., low embryo success in C. capitata and B. oleae; Zabalou et al., 2004b, 2009; Apostolaki et al., 2011). Wolbachia could affect male mating success by influencing assortative mating; a phenomenon detected in some studies of Drosophila (e.g., Koukou et al., 2006; Miller et al., 2010), but not others (e.g., Champion de Crespigny and Wedell, 2007; Arbuthnott et al., 2016; Cooper et al., 2017). Such influence of Wolbachia on mating preferences was questioned (Sharon et al., 2010) on the basis of evidence that gut microbiota influence assortative mating in Drosophila (Sharon et al., 2010; Ringo et al., 2011; Arbuthnott et al., 2016), a finding that itself has been questioned recently (Leftwich et al., 2017, 2018). In addition, at least one case has been reported where sperm from Wolbachia-infected males was less competitive (Champion de Crespigny and Wedell, 2006). Similarly, Wolbachia-infected D. simulans produce fewer sperm (Snook et al., 2000). All of the above parameters should be evaluated under relevant conditions known to interact with Wolbachia, such as temperature and nutrition (reviewed in Bourtzis and Robinson, 2006; e.g., Serbus et al., 2015; Corbin et al., 2017; Ross et al., 2019a), interaction with other microorganisms (e.g., Hughes et al., 2014; Ye et al., 2017), as well as male age, paternal grandmother age, and mating status (e.g., Karr et al., 1998; Awrahman et al., 2014; Layton et al., 2019).
Other Considerations
Species Recalcitrant to Wolbachia?
Certain species or clades appear to be “resistant” to Wolbachia infection, based on their lack of infection in nature and the failure to achieve stable transfections. The reasons are unknown, but could involve host and/or bacterial factors. For example, none of the members of the diverse repleta species group of Drosophila, comprised mostly of cactophilic flies (Markow and O’Grady, 2005), has ever been found to harbor Wolbachia (Mateos et al., 2006). Similarly, due to numerous failed transinfection attempts, and the lack of natural infection in wild Anopheles mosquitoes, this genus was regarded impervious to Wolbachia (reviewed in Hughes and Rasgon, 2014). This view has been challenged by the successful establishment of Wolbachia-transfected Anopheles stephensi Liston (Bian et al., 2013), and the recent discovery of a natural stable Wolbachia infection in Anopheles coluzzii Coetzee & Wilkerson (Shaw et al., 2016). Nonetheless, reports of Wolbachia in other species of Anopheles have been called into question (Sicard et al., 2019). The lack of natural infections and transinfection failure in A. ludens may reflect a general refractoriness to Wolbachia. Nonetheless, initial attempts to transinfect C. capitata also failed and transfection with Wolbachia was attained subsequently with different Wolbachia strains (Zabalou et al., 2004b). Hence, transinfection attempts with additional Wolbachia strains may result in successful and stable infection in A. ludens as well.
Potential for Target Populations to Become Resistant to Sterile Males
There are two ways in which a target population may become resistant to the effects of released Wolbachia-infected males. The first is endosymbiont-based, whereby the target population may acquire (e.g., via horizontal transmission) a Wolbachia strain that can rescue the modification (sterility) induced by the strain present in the released males. Generally, such acquisition of a Wolbachia strain during the relatively short lifespan of a release program seems unlikely. Nonetheless, knowledge on the Wolbachia infection status and strain identity of interacting species, such as other fruit flies sharing the same host plant and parasitoids, might aid in the selection of Wolbachia strains that are unlikely to be compatible with strains that can potentially be horizontally acquired by the target population. Permanent screening of wild flies from the target population could provide valuable information in order to foresee potential lack of effectiveness of the method. Laboratory experiments in which the conditions for horizontal transmission are favored (or even forced) might also help to determine the probability of such phenomena to occur in nature.
The second mechanism is host-based, whereby pre- or post-mating selection on wild females to avoid or reduce fertilization by incompatible sperm (reviewed by Wedell, 2013), acts on standing (or de novo) genetic variation. Evidence consistent with the influence of Wolbachia on premating mechanisms comes from the observation that females and males of Drosophila paulistorum Dobzhansky and Pavan exhibit assortative mating according to the Wolbachia strain they harbor (Miller et al., 2010; Schneider et al., 2019). In addition, treatment with antibiotic (which removed Wolbachia) decreases mate discrimination in D. melanogaster (Koukou et al., 2006). The evolution of resistance to mating with mass-reared males by wild females can be potentially minimized by frequently refreshing the genetic background of the mass-reared strain, with or without artificial selection (McInnis et al., 2002; Gilchrist et al., 2012; Zygouridis et al., 2014; Quintero-Fong et al., 2016; Sánchez-Rosario et al., 2017), which is a routine process in mass-rearing programs aimed at countering inbreeding and adaptation to mass rearing that is detrimental the success of released males (Robinson and Hendrichs, 2005). Nonetheless, if the basis for mate discrimination were solely determined by Wolbachia infection state (e.g., if females could distinguish Wolbachia-infected vs. Wolbachia-uninfected males solely on the basis of a Wolbachia-encoded factor), refreshing the fly genetic background of mass-reared strain is unlikely to slow down the evolution of resistance to released males in the target population.
Several lines of evidence are consistent with the influence of Wolbachia infection on post-mating mechanisms. The existence of genetic incompatibility is predicted to favor polyandry (multiple mating by females) as a female strategy to minimize the probability of her eggs being fertilized by sperm from incompatible males (Zeh and Zeh, 1996). Consistent with this prediction, uninfected D. simulans females remate sooner than Wolbachia-infected females (Champion de Crespigny et al., 2008). Furthermore, Wolbachia modifies the length of the spermathecal duct of females of the cricket Allonemobius socius Scudder (Marshall, 2007), which in turn may afford the female greater control on the outcome of sperm competition (e.g., D. melanogaster; Miller and Pitnick, 2002). Finally, the fact that host background can influence the CI phenotype (reviewed by Bourtzis and Robinson, 2006), suggests that target populations may have genetic variants that are more resistant to CI, which could increase in frequency as a result of the strong selection exerted by the massive release of Wolbachia-infected males.
Potential Alternative Ways of Implementing Wolbachia-Based Approaches
The recent identification of Wolbachia “CI genes” offers potential alternative ways of harnessing reproductive incompatibility in control of pest tephritids. First, to identify strains with the desired characteristics, at least ability to induce CI, a productive endeavor might be to search for CI loci in the genomes of candidate strains being considered for IIT, prior to artificial transfer efforts. A candidate Wolbachia strain that lacks CI loci homologs, or that contains CI loci homologs that are highly similar to (and thus potentially compatible with) strains present in target population, should be avoided. Secondly, it may be possible in the future to genetically engineer Wolbachia strains with the desired characteristics (e.g., one or more specific CI operons) for IIT programs, or to replace strains used previously in a control program, as a means of addressing resistance phenomena (Sullivan and O’Neill, 2017). Finally, a thorough understanding of the CI mechanism might enable the development of IIT based on Wolbachia transgenes, rather than Wolbachia infection. This might be particularly helpful in the control of species that are resistant to Wolbachia infection. Nonetheless, the release of such genetically modified insects might not be feasible due to regulatory hurdles and lack of public acceptance.
It has recently been shown that some Wolbachia strains can provide protection against major pathogens and parasites of insects, including RNA viruses and bacteria (Hedges et al., 2008; Teixeira et al., 2008; Ye et al., 2013; Martinez et al., 2014). It is very common for pathogens to appear in rearing facilities. Thus, if a Wolbachia strain could simultaneously cause strong CI and protect against one or more pathogens (e.g., RNA virus), this would have multiple benefits in an operational Wolbachia-based population suppression program. Furthermore, a Wolbachia strain that does not induce (strong) CI, but protects against pathogens might be desirable in a program that does not rely on CI (e.g., SIT) for population suppression. Wolbachia-mediated pathogen protection would enable high production and quality levels, thereby contributing to a cost-effective and sustainable insect pest management program.
Potential Influence of Other Symbionts
Multiple studies have revealed that although Wolbachia appears to be the dominant facultative heritable symbiont of arthropods, numerous other diverse bacteria (e.g., Spiroplasma, Arsenophonus, Rickettsia, and Cardinium) form such associations with insects, causing a diversity of reproductive and non-reproductive phenotypes (reviewed in Zchori-Fein and Bourtzis, 2011; Hurst and Frost, 2015; McLean et al., 2018). Despite the long-standing recognition that “Wolbachia do not walk alone” (Duron et al., 2008), many studies of Wolbachia fail to rule out the association of their study organism with other facultative heritable symbionts. Even intensely studied groups in terms of heritable symbionts, such as tsetse flies (genus Glossina), can yield surprises of bacterial associates (e.g., the recent discovery of Spiroplasma in two species of Glossina; Doudoumis et al., 2017). With few exceptions (Martínez et al., 2012; Augustinos et al., 2015; Asimakis et al., 2019; Conte et al., 2019; Devescovi et al., 2019), research on tephritid facultative heritable bacteria has not examined the possibility of players other than Wolbachia. Therefore, we urge that such research include screens for other symbionts, including viruses, protozoans, and fungi.
Tephritids are hosts to non-heritable bacteria, generally harbored in their gut (for recent reviews see Noman et al., 2019; Raza et al., 2020). Whether Wolbachia influences tephritid interactions with other microbes, has not been evaluated, but evidence for such interactions exists for other systems (reviewed in Brinker et al., 2019). For example, in Drosophila neotestacea Grimaldi, James, and Jaenike, the presence of Wolbachia promotes the abundance of Spiroplasma, and is positively correlated with abundance of Bacteroidales and Lactobacillales (Fromont et al., 2019). Similarly, Wolbachia influences the microbiome of D. melanogaster (Simhadri et al., 2017) and Armadillidium vulgare (Latreille) (Dittmer and Bouchon, 2018). It is therefore important to evaluate interactions between Wolbachia and the microbiome that influence negatively or positively aspects of mass-reared tephritids used in IIT or SIT.
Conclusion
Given the widespread occurrence of Wolbachia in tephritids and its known fitness consequences in this group of dipterans and in other host taxa, Wolbachia is likely an influential component of tephritid ecology and evolution. Further exploration of Wolbachia-tephritid associations is expected to reveal a diversity of effects, including interactions with other microbial partners, as seen in more extensively studied systems such as Drosophila and mosquitoes. The recent exciting progress in understanding the basis of CI, and many other aspects of Wolbachia biology, should accelerate progress in the development of Wolbachia-based IIT for tephritid species, particularly with the aid of comparative Wolbachia genomics to identify potential CI patterns on the basis of CI gene composition. We consider that one of the major obstacles to effectively implementing IIT will be to avoid population replacement due to accidental release of Wolbachia-infected females. The threshold number of accidentally released females, which is generally much higher in systems that employ bidirectional-CI compared to unidirectional-CI, must be thoroughly investigated prior to any field implementation. Where an unacceptable risk of population replacement exists, we recommend that SIT be explored as a complementary strategy to support IIT.
Author’s Note
An earlier version of manuscript has been released as a Pre-Print at https://www.biorxiv.org/content/10.1101/358333v1.
Author Contributions
MM led the drafting. HM, PL, JT, BM-A, KG, SL, DS, CC, AA, GT, EA, VD, NP, and GK edited multiple drafts.
Funding
Funding was provided by the Joint FAO/IAEA Coordinated Research Project “Use of Symbiotic Bacteria to Reduce Mass-Rearing Costs and Increase Mating Success in Selected Fruit Pests in Support of SIT Application” and TAMU-CONACYT (050 Proposal # 10586).
Conflict of Interest
The authors declare that the research was conducted in the absence of any commercial or financial relationships that could be construed as a potential conflict of interest.
Acknowledgments
K. Bourtzis provided valuable suggestions on an earlier version of this manuscript. We thank two reviewers for their thorough and helpful feedback.
Supplementary Material
The Supplementary Material for this article can be found online at: https://www.frontiersin.org/articles/10.3389/fmicb.2020.01080/full#supplementary-material
FILE S1 | Compilation of published reports of screenings of Wolbachia (and other heritable bacteria) in pest Tephritidae. In our counts of species, A. fraterculus morphotypes (Hernández-Ortiz et al., 2015) are regarded as separate species. Additional references not cited in main text but cited in this table (Drosopoulou et al., 2010; Karimi and Darsouei, 2014; Yong et al., 2017; Gichuhi et al., 2019; Schebeck et al., 2019; Wang et al., 2019).
Footnotes
References
Ahmed, M. Z., Li, S. J., Xue, X., Yin, X. J., Ren, S. X., Jiggins, F. M., et al. (2015). The intracellular bacterium Wolbachia uses parasitoid wasps as phoretic vectors for efficient horizontal transmission. PLoS Pathog. 10:e1004672. doi: 10.1371/journal.ppat.1004672
Aleksandrov, I., Aleksandrova, M., Goriacheva, I., Roshchina, N., Shaikevich, E., and Zakharov, I. (2007). Removing endosymbiotic Wolbachia specifically decreases lifespan of females and competitiveness in a laboratory strain of Drosophila melanogaster. Genetika 43, 1372–1378.
Apostolaki, A., Livadaras, I., Saridaki, A., Chrysargyris, A., Savakis, C., and Bourtzis, K. (2011). Transinfection of the olive fruit fly Bactrocera oleae with Wolbachia: towards a symbiont-based population control strategy. J. Appl. Entomol. 135, 546–553.
Arbuthnott, D., Levin, T. C., and Promislow, D. E. (2016). The impacts of Wolbachia and the microbiome on mate choice in Drosophila melanogaster. J. Evol. Biol. 29, 461–468. doi: 10.1111/jeb.12788
Arcella, T., Hood, G. R., Powell, T. H., Sim, S. B., Yee, W. L., Schwarz, D., et al. (2015). Hybridization and the spread of the apple maggot fly, Rhagoletis pomonella (Diptera: Tephritidae), in the northwestern United States. Evol. Appl. 8, 834–846. doi: 10.1111/eva.12298
Arthofer, W., Riegler, M., Avtzis, D. N., and Stauffer, C. (2009a). Evidence for low-titre infections in insect symbiosis: Wolbachia in the bark beetle Pityogenes chalcographus (Coleoptera, Scolytinae). Environ. Microbiol. 11, 1923–1933. doi: 10.1111/j.1462-2920.2009.01914.x
Arthofer, W., Riegler, M., Schneider, D., Krammer, M., Miller, W. J., and Stauffer, C. (2009b). Hidden Wolbachia diversity in field populations of the European cherry fruit fly, Rhagoletis cerasi (Diptera, Tephritidae). Mol. Ecol. 18, 3816–3830. doi: 10.1111/j.1365-294X.2009.04321.x
Arthofer, W., Riegler, M., Schuler, H., Schneider, D., Moder, K., Miller, W. J., et al. (2011). Allele intersection analysis: a novel tool for multi locus sequence assignment in multiply infected hosts. PLoS One 6:e22198. doi: 10.1371/journal.pone.0022198
Asimakis, E. D., Doudoumis, V., Hadapad, A. B., Hire, R. S., Batargias, C., Niu, C., et al. (2019). Detection and characterization of bacterial endosymbionts in Southeast Asian tephritid fruit fly populations. BMC Microbiol. 19:290. doi: 10.1186/s12866-019-1653-x
Attardo, G. M., Abd-Alla, A. M. M., Acosta-Serrano, A., Allen, J. E., Bateta, R., and Benoit, J. B. (2019). Comparative genomic analysis of six Glossina genomes, vectors of African trypanosomes. Genome Biol. 20:187. doi: 10.1186/s13059-019-1768-2
Augustinos, A. A., Asimakopoulou, A. K., Moraiti, C. A., Mavragani-Tsipidou, P., Papadopoulos, N. T., and Bourtzis, K. (2014). Microsatellite and Wolbachia analysis in Rhagoletis cerasi natural populations: population structuring and multiple infections. Ecol. Evol. 4, 1943–1962. doi: 10.1002/ece3.553
Augustinos, A. A., Drosopoulou, E., Gariou-Papalexiou, A., Asimakis, E. D., Cáceres, C., Tsiamis, G., et al. (2015). Cytogenetic and symbiont analysis of five members of the B. dorsalis complex (Diptera, Tephritidae): no evidence of chromosomal or symbiont-based speciation events. Zookeys 2, 273–298. doi: 10.3897/zookeys.540.9857
Augustinos, A. A., Santos-Garcia, D., Dionyssopoulou, E., Moreira, M., Papapanagiotou, A., Scarvelakis, M., et al. (2011). Detection and characterization of Wolbachia infections in natural populations of aphids: is the hidden diversity fully unraveled? PLoS One 6:e28695. doi: 10.1371/journal.pone.0028695
Awrahman, Z. A., Champion, de Crespigny, F., and Wedell, N. (2014). The impact of Wolbachia, male age and mating history on cytoplasmic incompatibility and sperm transfer in Drosophila simulans. J. Evol. Biol. 27, 1–10. doi: 10.1111/jeb.12270
Bailly-Bechet, M., Martins-Simões, P., Szöllõsi, G. J., Mialdea, G., Sagot, M.-F., and Charlat, S. (2017). How long does Wolbachia remain on board? Mol. Biol. Evol. 34, 1183–1193. doi: 10.1093/molbev/msx073
Bakovic, V., Schebeck, M., Telschow, A., Stauffer, C., and Schuler, H. (2018). Spatial spread of Wolbachia in Rhagoletis cerasi populations. Biol. Lett. 14:161. doi: 10.1098/rsbl.2018.0161
Bakri, A., Mehta, K., and Lance, R. (2005). “Sterilizing insects with ionizing radiation,” in Sterile Insect Technique, Principles and Practices in Area-Wide Integrated Pest Management, eds V. A. Dyck, J. Hendrichs, and A. S. Robinson, (Cham: Springer), 233–268.
Baldo, L., Dunning Hotopp, J. C., Jolley, K. A., Bordenstein, S. R., Biber, S. A., Choudhury, R. R., et al. (2006). Multilocus sequence typing system for the endosymbiont Wolbachia pipientis. Appl. Environ. Microbiol. 72, 7098–7110. doi: 10.1128/AEM.00731-06
Baldo, L., Lo, N., and Werren, J. H. (2005). Mosaic nature of the Wolbachia surface protein. J. Bacteriol. 187, 5406–5418.
Ballard, J. W., and Melvin, R. G. (2007). Tetracycline treatment influences mitochondrial metabolism and mtDNA density two generations after treatment in Drosophila. Insect Mol. Biol. 16, 799–802. doi: 10.1111/j.1365-2583.2007.00760.x
Baton, L. A., Pacidonio, E. C., Goncalves, D. S., and Moreira, L. A. (2013). wFlu: characterization and evaluation of a native Wolbachia from the mosquito Aedes fluviatilis as a potential vector control agent. PLoS One 8:e59619. doi: 10.1371/journal.pone.0059619
Beckmann, J. F., Bonneau, M., Chen, H., Hochstrasser, M., Poinsot, D., Merçot, H., et al. (2019a). Caution does not preclude predictive and testable models of cytoplasmic incompatibility: a reply to Shropshire et al. Trends Genet. 35:399. doi: 10.1016/j.tig.2019.03.002
Beckmann, J. F., Bonneau, M., Chen, H., Hochstrasser, M., Poinsot, D., Merçot, H., et al. (2019b). The toxin–antidote model of cytoplasmic incompatibility: genetics and evolutionary implications. Trends Genet. 35, 175–185. doi: 10.1016/j.tig.2018.12.004
Beckmann, J. F., and Fallon, A. M. (2012). Decapitation improves detection of Wolbachia pipientis (Rickettsiales: Anaplasmataceae) in Culex pipiens (Diptera: Culicidae) mosquitoes by the polymerase chain reaction. J. Med. Entomol. 49, 1103–1108. doi: 10.1603/me12049
Beckmann, J. F., Ronau, J. A., and Hochstrasser, M. (2017). A Wolbachia deubiquitylating enzyme induces cytoplasmic incompatibility. Nat. Microbiol. 2:17007. doi: 10.1038/nmicrobiol.2017.7
Beckmann, J. F., Sharma, G. D., Mendez, L., Chen, H., and Hochstrasser, M. (2019c). The Wolbachia cytoplasmic incompatibility enzyme CidB targets nuclear import and protamine-histone exchange factors. eLife 8:26. doi: 10.7554/eLife.50026
Benedict, M. Q., and Robinson, A. S. (2003). The first releases of transgenic mosquitoes: an argument for the sterile insect technique. Trends Parasitol. 19, 349–355. doi: 10.1016/s1471-4922(03)00144-2
Bi, J., and Wang, Y.-F. (2019). The effect of the endosymbiont Wolbachia on the behavior of insect hosts. Insect Sci. 1–13. doi: 10.1111/1744-7917.12731
Bian, G., Joshi, D., Dong, Y., Lu, P., Zhou, G., Pan, X., et al. (2013). Wolbachia invades Anopheles stephensi populations and induces refractoriness to Plasmodium infection. Science 340, 748–751. doi: 10.1126/science.1236192
Bierbaum, T. J., and Bush, G. L. (1990). Genetic differentiation in the viability of sibling species of Rhagoletis fruit flies on host plants, and the influence of reduced hybrid viability on reproductive isolation. Entomol. Exp. Appl. 55, 105–118.
Black, W. C., Alphey, L., and James, A. A. (2011). Why RIDL is not SIT. Trends Parasitol. 27, 362–370. doi: 10.1016/j.pt.2011.04.004
Bleidorn, C. (2016). Third generation sequencing: technology and its potential impact on evolutionary biodiversity research. Syst. Biodivers. 14, 1–8.
Bleidorn, C., and Gerth, M. (2017). A critical re-evaluation of multilocus sequence typing (MLST) efforts in Wolbachia. FEMS Microbiol. Ecol. 94:fix163. doi: 10.1093/femsec/fix163
Bo, W., Ahmad, S., Dammalage, T., Tomas, U. S., Wornoayporn, V., Haq, I. U., et al. (2014). Mating compatibility between Bactrocera invadens and Bactrocera dorsalis (Diptera: Tephritidae). J. Econ. Entomol. 107, 623–629. doi: 10.3897/zookeys.540.6568
Boller, E., and Bush, G. (1974). Evidence for genetic variation in populations of the European cherry fruit fly, Rhagoletis cerasi (Diptera: Tephritidae) based on physiological parameters and hybridization experiments. Entomol. Exp. Appl. 17, 279–293.
Boller, E., Russ, K., Vallo, V., and Bush, G. (1976). Incompatible races of European cherry fruit fly, Rhagoletis cerasi (Diptera: Tephritidae), their origin and potential use in biological control. Entomol. Exp. Appl. 20, 237–247.
Bonneau, M., Atyame, C., Beji, M., Justy, F., Cohen-Gonsaud, M., Sicard, M., et al. (2018). Culex pipiens crossing type diversity is governed by an amplified and polymorphic operon of Wolbachia. Nat. Commun. 9:319. doi: 10.1038/s41467-017-02749-w
Bonneau, M., Caputo, B., Ligier, A., Caparros, R., Unal, S., Perriat-Sanguinet, M., et al. (2019). Variation in Wolbachia cidB gene, but not cidA, is associated with cytoplasmic incompatibility mod phenotype diversity in Culex pipiens. Mol. Ecol. 28, 4725–4736. doi: 10.1111/mec.15252
Bourtzis, K. (2008). Wolbachia-based technologies for insect pest population control. Transgen. Manag. Vect. Borne Dis. 627, 104–113. doi: 10.1007/978-0-387-78225-6_9
Bourtzis, K., Dobson, S. L., Xi, Z., Rasgon, J. L., Calvitti, M., Moreira, L. A., et al. (2014). Harnessing mosquito-Wolbachia symbiosis for vector and disease control. Acta Trop. 132(Suppl.), S150–S163. doi: 10.1016/j.actatropica.2013.11.004
Bourtzis, K., Lees, R. S., Hendrichs, J., and Vreysen, M. J. (2016). More than one rabbit out of the hat: radiation, transgenic and symbiont-based approaches for sustainable management of mosquito and tsetse fly populations. Acta Trop. 157, 115–130. doi: 10.1016/j.actatropica.2016.01.009
Bourtzis, K., Nirgianaki, A., Onyango, P., and Savakis, C. (1994). A prokaryotic dnaA sequence in Drosophila melanogaster: Wolbachia infection and cytoplasmic incompatibility among laboratory strains. Insect Mol. Biol. 3, 131–142. doi: 10.1111/j.1365-2583.1994.tb00160.x
Bourtzis, K., and Robinson, A. (2006). “Insect pest control using Wolbachia and/or radiation,” in Insect Symbiosis, eds K. Bourtzis and T. A. Miller, (Boca Raton, FL: CRC Press), 225–246.
Braig, H. R., Zhou, W., Dobson, S. L., and O’Neill, S. L. (1998). Cloning and characterization of a gene encoding the major surface protein of the bacterial endosymbiont Wolbachia pipientis. J. Bacteriol. 180, 2373–2378.
Brandon-Mong, G. J., Gan, H. M., Sing, K. W., Lee, P. S., Lim, P. E., and Wilson, J. J. (2015). DNA metabarcoding of insects and allies: an evaluation of primers and pipelines. Bull. Entomol. Res. 105, 717–727. doi: 10.1017/S0007485315000681
Brelsfoard, C., Tsiamis, G., Falchetto, M., Gomulski, L. M., Telleria, E., Alam, U., et al. (2014). Presence of extensive Wolbachia symbiont insertions discovered in the genome of its host Glossina morsitans morsitans. PLoS Negl. Trop. Dis. 8:e2728. doi: 10.1371/journal.pntd.0002728
Brinker, P., Fontaine, M. C., Beukeboom, L. W., and Falcao Salles, J. (2019). Host, symbionts, and the microbiome: the missing tripartite interaction. Trends Microbiol. 27, 480–488. doi: 10.1016/j.tim.2019.02.002
Brownlie, J. C., Cass, B. N., Riegler, M., Witsenburg, J. J., Iturbe-Ormaetxe, I., McGraw, E. A., et al. (2009). Evidence for metabolic provisioning by a common invertebrate endosymbiont, Wolbachia pipientis, during periods of nutritional stress. PLoS Pathog. 5:e1000368. doi: 10.1371/journal.ppat.1000368
Bryant, K. N., and Newton, I. L. G. (2019). The intracellular symbiont Wolbachia enhances recombination in a dose-dependent manner. bioRxiv [Preprint], doi: 10.1101/686444
Cáceres, C., McInnis, D., Shelly, T., Jang, E., Robinson, A., and Hendrichs, J. (2007). Quality management systems for fruit fly (Diptera: Tephritidae) sterile insect technique. Fla. Entomol. 90, 1–9.
Cáceres, C., Segura, D. F., Vera, M. T., Wornoayporn, V., Cladera, J. L., Teal, P., et al. (2009). Incipient speciation revealed in Anastrepha fraterculus (Diptera; Tephritidae) by studies on mating compatibility, sex pheromones, hybridization, and cytology. Biol. J. Linn. Soc. 97, 152–165.
Caputo, B., Moretti, R., Manica, M., Serini, P., Lampazzi, E., Bonanni, M., et al. (2019). A bacterium against the tiger: preliminary evidence of fertility reduction after release of Aedes albopictus males with manipulated Wolbachia infection in an Italian urban area. Pest Manag. Sci. 76:5643. doi: 10.1002/ps.5643
Caspari, E., and Watson, G. (1959). On the evolutionary importance of cytoplasmic sterility in mosquitoes. Evolution 13, 568–570.
Chafee, M. E., Funk, D. J., Harrison, R. G., and Bordenstein, S. R. (2010). Lateral phage transfer in obligate intracellular bacteria (Wolbachia): verification from natural populations. Mol. Biol. Evol. 27, 501–505. doi: 10.1093/molbev/msp275
Champion de Crespigny, F. E., Hurst, L. D., and Wedell, N. (2008). Do Wolbachia-associated incompatibilities promote polyandry? Evolution 62, 107–122. doi: 10.1111/j.1558-5646.2007.00274.x
Champion de Crespigny, F. E., and Wedell, N. (2006). Wolbachia infection reduces sperm competitive ability in an insect. Proc. R. Soc. Lond. B Biol. Sci. 273, 1455–1458. doi: 10.1098/rspb.2006.3478
Champion de Crespigny, F. E., and Wedell, N. (2007). Mate preferences in Drosophila infected with Wolbachia? Behav. Ecol. Sociobiol. 61:1229.
Chen, H., Ronau, J. A., Beckmann, J. F., and Hochstrasser, M. (2019). A Wolbachia nuclease and its binding partner provide a distinct mechanism for cytoplasmic incompatibility. Proc. Natl. Acad. Sci. U.S.A. 2019, 14571. doi: 10.1073/pnas.1914571116
Chen, W.-J., Tsai, K.-H., Cheng, S.-L., Huang, C.-G., and Wu, W.-J. (2005). Using in situ hybridization to detect endosymbiont Wolbachia in dissected tissues of mosquito host. J. Med. Entomol. 42, 120–124. doi: 10.1093/jmedent/42.2.120
Choo, A., Crisp, P., Saint, R., O’Keefe, L., and Baxter, S. (2017). CRISPR/Cas9-mediated mutagenesis of the white gene in the tephritid pest Bactrocera tryoni. J. Appl. Entomol. 142:12411. doi: 10.1111/jen.12411
Chrostek, E., Hurst, G. D., and McGraw, E. A. (2020). Infectious diseases: antiviral Wolbachia limits dengue in malaysia. Curr. Biol. 30, R30–R32. doi: 10.1016/j.cub.2019.11.046
Cladera, J. L., Vilardi, J. C., Juri, M., Paulin, L. E., Giardini, M. C., Cendra, P. V. G., et al. (2014). Genetics and biology of Anastrepha fraterculus: research supporting the use of the sterile insect technique (SIT) to control this pest in Argentina. BMC Genet. 15:S12. doi: 10.1186/1471-2156-15-S2-S12
Collins, S. R., Weldon, C. W., Banos, C., and Taylor, P. W. (2009). Optimizing irradiation dose for sterility induction and quality of Bactrocera tryoni. J. Econ. Entomol. 102, 1791–1800. doi: 10.1603/029.102.0509
Conte, C., Segura, D., Milla, F., Augustinos, A., Cladera, J., Bourtzis, K., et al. (2019). Wolbachia infection in argentinean populations of Anastrepha fraterculus sp1: preliminary evidence of sex ratio distortion by one of two strains. BMC Microbiol. 19:289. doi: 10.1186/s12866-019-1652-y
Cooper, B. S., Ginsberg, P. S., Turelli, M., and Matute, D. R. (2017). Wolbachia in the Drosophila yakuba complex: pervasive frequency variation and weak cytoplasmic incompatibility, but no apparent efect on reproductive isolation. Genetics 205, 333–351. doi: 10.1534/genetics.116.196238
Corbin, C., Heyworth, E. R., Ferrari, J., and Hurst, G. D. (2017). Heritable symbionts in a world of varying temperature. Heredity 118, 10–20. doi: 10.1038/hdy.2016.71
Coscrato, V. E., Braz, A. S., Perondini, A. L. P., Selivon, D., and Marino, C. L. (2009). Wolbachia in Anastrepha fruit flies (Diptera: Tephritidae). Curr. Microbiol. 59, 295–301. doi: 10.1007/s00284-009-9433-9438
Craig, T. P., Horner, J. D., and Itami, J. K. (1997). Hybridization studies on the host races of Eurosta solidaginis: implications for sympatric speciation. Evolution 51, 1552–1560. doi: 10.1111/j.1558-5646.1997.tb01478.x
Cruickshank, L., Jessup, A. J., and Cruickshank, D. J. (2001). Interspecific crosses of Bactrocera tryoni (Froggatt) and Bactrocera jarvisi (Tryon)(Diptera: Tephritidae) in the laboratory. Aust. Entomol. 40, 278–280.
da Silva Gonçalves, D., Cassimiro, A. P. A., de Oliveira, C. D., Rodrigues, N. B., and Moreira, L. A. (2014). Wolbachia detection in insects through LAMP: loop mediated isothermal amplification. Parasit. Vect. 7:228. doi: 10.1186/1756-3305-7-228
de Oliveira, C. D., Goncalves, D. S., Baton, L. A., Shimabukuro, P. H., Carvalho, F. D., and Moreira, L. A. (2015). Broader prevalence of Wolbachia in insects including potential human disease vectors. Bull. Entomol. Res. 105, 305–315. doi: 10.1017/S0007485315000085
Dean, M. D. (2006). A Wolbachia-associated fitness benefit depends on genetic background in Drosophila simulans. Proc. R. Soc. Lond. B Biol. Sci. 273, 1415–1420. doi: 10.1098/rspb.2005.3453
Devescovi, F., Bachmann, G. E., Nussenbaum, A. L., Viscarret, M. M., Cladera, J. L., and Segura, D. F. (2017). Effects of superparasitism on immature and adult stages of Diachasmimorpha longicaudata Ashmead (Hymenoptera: Braconidae) reared on Ceratitis capitata Wiedemann (Diptera: Tephritidae). Bull. Entomol. Res. 107, 756–767. doi: 10.1017/S000748531700027X
Devescovi, F., Conte, C. A., Augustinos, A., Cancio Martinez, E. I., Segura, D. F., Caceres, C., et al. (2019). Symbionts do not affect the mating incompatibility between the Brazilian-1 and Peruvian morphotypes of the Anastrepha fraterculus cryptic species complex. Sci. Rep. 9:18319. doi: 10.1038/s41598-019-54704-y
Dias, N. P., Zotti, M. J., Montoya, P., Carvalho, I. R., and Nava, D. E. (2018). Fruit fly management research: a systematic review of monitoring and control tactics in the world. Crop Prot. 112, 187–200. doi: 10.1016/j.cropro.2018.05.019
Dionysopoulou, N. K., Papanastasiou, S. A., Kyritsis, G. A., and Papadopoulos, N. T. (2020). Effect of host fruit, temperature and Wolbachia infection on survival and development of Ceratitis capitata immature stages. PLoS One 15:e0229727. doi: 10.1371/journal.pone.0229727
Dittmer, J., and Bouchon, D. (2018). Feminizing Wolbachia influence microbiota composition in the terrestrial isopod Armadillidium vulgare. Sci. Rep. 8:6998. doi: 10.1038/s41598-018-25450-4
Dobson, S. L., Bourtzis, K., Braig, H. R., Jones, B. F., Zhou, W., Rousset, F., et al. (1999). Wolbachia infections are distributed throughout insect somatic and germ line tissues. Insect Biochem. Mol. Biol. 29, 153–160. doi: 10.1016/s0965-1748(98)00119-2
Dobson, S. L., Fox, C. W., and Jiggins, F. M. (2002). The effect of Wolbachia-induced cytoplasmic incompatibility on host population size in natural and manipulated systems. Proc. Natl. Acad. Sci. U.S.A. 269, 437–445. doi: 10.1098/rspb.2001.1876
Dodson, B. L., Hughes, G. L., Paul, O., Matacchiero, A. C., Kramer, L. D., and Rasgon, J. L. (2014). Wolbachia enhances West Nile virus (WNV) infection in the mosquito Culex tarsalis. PLoS Negl. Trop. Dis. 8:e2965. doi: 10.1371/journal.pntd.0002965
Doellman, M. M., Schuler, H., Jean Saint, G., Hood, G. R., Egan, S. P., Powell, T. H., et al. (2019). Geographic and ecological dimensions of host plant-associated genetic differentiation and speciation in the Rhagoletis cingulata (Diptera: Tephritidae) sibling species group. Insects 10:275. doi: 10.3390/insects10090275
Dos Santos, P., Uramoto, K., and Matioli, S. (2001). Experimental hybridization among Anastrepha species (Diptera: Tephritidae): production and morphological characterization of F1 hybrids. Ann. Entomol. Soc. Am. 94, 717–725.
Doudoumis, V., Blow, F., Saridaki, A., Augustinos, A., Dyer, N. A., Goodhead, I., et al. (2017). Challenging the Wigglesworthia, Sodalis, Wolbachia symbiosis dogma in tsetse flies: Spiroplasma is present in both laboratory and natural populations. Sci. Rep. 7:4699. doi: 10.1038/s41598-017-04740-3
Doudoumis, V., Tsiamis, G., Wamwiri, F., Brelsfoard, C., Alam, U., Aksoy, E., et al. (2012). Detection and characterization of Wolbachia infections in laboratory and natural populations of different species of tsetse flies (genus Glossina). BMC Microbiol. 12(Suppl. 1):S3. doi: 10.1186/1471-2180-12-S1-S3
Drew, R., and Lambert, D. (1986). On the specific status of Dacus (Bactrocera aquilonis and D.(Bactrocera tryoni (Diptera: Tephritidae). Ann. Entomol. Soc. Am. 79, 870–878.
Drosopoulou, E., Augustinos, A., Nakou, I., Koeppler, K., Kounatidis, I., Vogt, H., et al. (2011). Genetic and cytogenetic analysis of the American cherry fruit fly, Rhagoletis cingulata (Diptera: Tephritidae). Genetica 139, 1449–1464. doi: 10.1007/s10709-012-9644-y
Drosopoulou, E., Koeppler, K., Kounatidis, I., Nakou, I., Papadopoulos, N., Bourtzis, K., et al. (2010). Genetic and cytogenetic analysis of the walnut-husk fly (Diptera: Tephritidae). Ann. Entomol. Soc. Am. 103, 1003–1011.
Du, Y., Ji, Q., Pan, J., and Lai, Z. (2016). Suitability of the pumpkin fruit fly, Bactrocera tau (Walker)(Diptera: Tephritidae) for a sterile insect technique program. Egypt. J. Biol. Pest Control 26, 665–669.
Dunning Hotopp, J. C., Clark, M. E., Oliveira, D., Foster, J. M., Fischer, P., Torres, M. C., et al. (2007). Widespread lateral gene transfer from intracellular bacteria to multicellular eukaryotes. Science 317, 1753–1756. doi: 10.1126/science.1142490
Duron, O., Bouchon, D., Boutin, S., Bellamy, L., Zhou, L., Engelstadter, J., et al. (2008). The diversity of reproductive parasites among arthropods: Wolbachia do not walk alone. BMC Biol. 6:27. doi: 10.1186/1741-7007-6-27
Duron, O., and Hurst, G. D. (2013). Arthropods and inherited bacteria: from counting the symbionts to understanding how symbionts count. BMC Biol. 11:45. doi: 10.1186/1741-7007-11-45
Dyck, V. A., Hendrichs, J., and Robinson, A. S. (eds) (2005). Sterile Insect Technique: Principles and Practice in Area-Wide Integrated Pest Management. Berlin: Springer.
Ebina, T., and Ohto, K. (2006). Morphological characters and PCR-RFLP markers in the interspecific hybrids between Bactrocera carambolae and B. papayae of the B. dorsalis species complex (Diptera: Tephritidae). Res. Bull. Prot. Jpn. 42, 23–34.
Enkerlin, W. R. (2005). “Impact of fruit fly control programmes using the Sterile Insect Technique,” in Sterile Insect Technique: Principles and Practice In Area-Wide Integrated Pest Management, eds V. A. Dyck, J. Hendrichs, and A. S. Robinson, (Berlin: Springer), 651–676.
Erbout, N., De Meyer, M., and Lens, L. (2008). Hybridization between two polyphagous fruit-fly species (Diptera: Tephritidae) causes sex-biased reduction in developmental stability. Biol. J. Linn. Soc. 93, 579–588.
Faria, V. G., Paulo, T. F., and Sucena, E. (2016). Testing cannibalism as a mechanism for horizontal transmission of Wolbachia in Drosophila. Symbiosis 1, 79–85.
Fast, E. M., Toomey, M. E., Panaram, K., Desjardins, D., Kolaczyk, E. D., and Frydman, H. M. (2011). Wolbachia enhance Drosophila stem cell proliferation and target the germline stem cell niche. Science 334, 990–992. doi: 10.1126/science.1209609
Fenn, K., Conlon, C., Jones, M., Quail, M. A., Holroyd, N. E., Parkhill, J., et al. (2006). Phylogenetic relationships of the Wolbachia of nematodes and arthropods. PLoS Pathog. 2:e94. doi: 10.1371/journal.ppat.0020094
Franz, G. (2005). “Genetic sexing strains in Mediterranean fruit fly, an example for other species amenable to large-scale rearing for the sterile insect technique,” in Sterile Insect Technique, eds V. A. Dyck, J. Hendrichs, and A. Robinson, (Dordrecht: Springer), 427–451.
Fromont, C., Adair, K. L., and Douglas, A. E. (2019). Correlation and causation between the microbiome, Wolbachia and host functional traits in natural populations of Drosophilid flies. Mol. Ecol. 28, 1826–1841. doi: 10.1111/mec.15041
Frydman, H. M., Li, J. M., Robson, D. N., and Wieschaus, E. (2006). Somatic stem cell niche tropism in Wolbachia. Nature 441, 509–512. doi: 10.1038/nature04756
Fytrou, A., Schofield, P. G., Kraaijeveld, A. R., and Hubbard, S. F. (2006). Wolbachia infection suppresses both host defence and parasitoid counter-defence. Proc. R. Soc. Lond. B Biol. Sci. 273, 791–796. doi: 10.1098/rspb.2005.3383
Geniez, S., Foster, J. M., Kumar, S., Moumen, B., LeProust, E., Hardy, O., et al. (2012). Targeted genome enrichment for efficient purification of endosymbiont DNA from host DNA. Symbiosis 58, 201–207. doi: 10.1007/s13199-012-0215-x
Gerth, M., and Bleidorn, C. (2016). Comparative genomics provides a timeframe for Wolbachia evolution and exposes a recent biotin synthesis operon transfer. Nat. Microbiol. 2:16241. doi: 10.1038/nmicrobiol.2016.241
Gibson, J., Shokralla, S., Porter, T. M., King, I., van Konynenburg, S., Janzen, D. H., et al. (2014). Simultaneous assessment of the macrobiome and microbiome in a bulk sample of tropical arthropods through DNA metasystematics. Proc. Natl. Acad. Sci. U.S.A. 111, 8007–8012. doi: 10.1073/pnas.1406468111
Gichuhi, J., Khamis, F. M., Van den Berg, J., Ekesi, S., and Herren, J. K. (2019). Unexpected diversity of Wolbachia associated with Bactrocera dorsalis (Diptera: Tephritidae) in Africa. Insects 10:155. doi: 10.3390/insects10060155
Gilchrist, A., Cameron, E., Sved, J., and Meats, A. (2012). Genetic consequences of domestication and mass rearing of pest fruit fly Bactrocera tryoni (Diptera: Tephritidae). J. Econ. Entomol. 105, 1051–1056. doi: 10.1603/ec11421
Gilchrist, A. S., Shearman, D. C., Frommer, M., Raphael, K. A., Deshpande, N. P., Wilkins, M. R., et al. (2014). The draft genome of the pest tephritid fruit fly Bactrocera tryoni: resources for the genomic analysis of hybridising species. BMC Genomics 15:1153. doi: 10.1186/1471-2164-15-1153
Gilles, J. R., Schetelig, M. F., Scolari, F., Marec, F., Capurro, M. L., Franz, G., et al. (2014). Towards mosquito sterile insect technique programmes: exploring genetic, molecular, mechanical and behavioural methods of sex separation in mosquitoes. Acta Trop. 132(Suppl.), S178–S187. doi: 10.1016/j.actatropica.2013.08.015
Glowska, E., Dragun-Damian, A., Dabert, M., and Gerth, M. (2015). New Wolbachia supergroups detected in quill mites (Acari: Syringophilidae). Infect. Genet. Evol. 30, 140–146. doi: 10.1016/j.meegid.2014.12.019
Goodwin, S., McPherson, J. D., and McCombie, W. R. (2016). Coming of age: ten years of next-generation sequencing technologies. Nat. Rev. Genet. 17, 333–351. doi: 10.1038/nrg.2016.49
Handler, A. M. (2016). Enhancing the stability and ecological safety of mass-reared transgenic strains for field release by redundant conditional lethality systems. Insect Sci. 23, 225–234. doi: 10.1111/1744-7917.12245
Harris, H. L., Brennan, L. J., Keddie, B. A., and Braig, H. R. (2010). Bacterial symbionts in insects: balancing life and death. Symbiosis 51, 37–53.
Hedges, L. M., Brownlie, J. C., O’Neill, S. L., and Johnson, K. N. (2008). Wolbachia and virus protection in insects. Science 322:702. doi: 10.1126/science.1162418
Hendrichs, J., Franz, G., and Rendon, P. (1995). Increased effectiveness and applicability of the sterile insect technique through male-only releases for control of Mediterranean fruit flies during fruiting seasons. J. Appl. Entomol. 119, 371–377.
Hendrichs, J., and Robinson, A. (2009). “Chapter 243 - Sterile insect technique,” in Encyclopedia of Insects (Second Edition), eds V. H. Resh, and R. T. Cardé, (San Diego: Academic Press), 953–957.
Hendrichs, J., Vreysen, M., Enkerlin, W., and Cayol, J. (2005). “Strategic options in using sterile insects for area-wide integrated pest management,” in Sterile Insect Technique, eds V. A. Dyck, J. Hendrichs, A. Robinson, (Dordrecht: Springer), 563–600.
Hernández-Ortiz, V., Canal, N. A., Salas, J. O. T., Ruíz-Hurtado, F. M., and Dzul-Cauich, J. F. (2015). Taxonomy and phenotypic relationships of the Anastrepha fraterculus complex in the mesoamerican and Pacific neotropical dominions (Diptera, Tephritidae). Zookeys 2015:95. doi: 10.3897/zookeys.540.6027
Hibino, Y., and Iwahashi, O. (1991). Appearance of wild females unreceptive to sterilized males on Okinawa Is. in the eradication program of the melon fly, Dacus cucurbitae Coquillett (Diptera: Tephritidae). Appl. Entomol. Zool. 26, 265–270.
Hilgenboecker, K., Hammerstein, P., Schlattmann, P., Telschow, A., and Werren, J. H. (2008). How many species are infected with Wolbachia?–A statistical analysis of current data. FEMS Microbiol. Lett. 281, 215–220. doi: 10.1111/j.1574-6968.2008.01110.x
Hoffmann, A. A., and Turelli, M. (1988). Unidirectional incompatibility in Drosophila simulans: inheritance, geographic variation and fitness effects. Genetics 119, 435–444.
Hoffmann, A. A., Turelli, M., and Harshman, L. G. (1990). Factors affecting the distribution of cytoplasmic incompatibility in Drosophila simulans. Genetics 126, 933–948.
Hughes, G. L., Dodson, B. L., Johnson, R. M., Murdock, C. C., Tsujimoto, H., Suzuki, Y., et al. (2014). Native microbiome impedes vertical transmission of Wolbachia in Anopheles mosquitoes. Proc. Natl. Acad. Sci. U.S.A. 111, 12498–12503. doi: 10.1073/pnas.1408888111
Hughes, G. L., Pike, A. D., Xue, P., and Rasgon, J. L. (2012a). Invasion of Wolbachia into Anopheles and other insect germlines in an ex vivo organ culture system. PLoS One 7:e36277. doi: 10.1371/journal.pone.0036277
Hughes, G. L., and Rasgon, J. L. (2014). Transinfection: a method to investigate Wolbachia-host interactions and control arthropod-borne disease. Insect Mol. Biol. 23, 141–151. doi: 10.1111/imb.12066
Hughes, G. L., Vega-Rodriguez, J., Xue, P., and Rasgon, J. L. (2012b). Wolbachia strain wAlbB enhances infection by the rodent malaria parasite Plasmodium berghei in Anopheles gambiae mosquitoes. Appl. Environ. Microbiol. 78, 1491–1495. doi: 10.1128/AEM.06751-11
Huigens, M. E., de Almeida, R. P., Boons, P. A., Luck, R. F., and Stouthamer, R. (2004). Natural interspecific and intraspecific horizontal transfer of parthenogenesis-inducing Wolbachia in Trichogramma wasps. Proc. R. Soc. Lond. B Biol. Sci. 271, 509–515. doi: 10.1098/rspb.2003.2640
Hurst, G. D., and Frost, C. L. (2015). Reproductive parasitism: maternally inherited symbionts in a biparental world. Cold Spring Harb. Perspect. Biol. 7:a017699. doi: 10.1101/cshperspect.a017699
Hurst, L. D. (1991). The evolution of cytoplasmic incompatibility or when spite can be successful. J. Theor. Biol. 148, 269–277. doi: 10.1016/s0022-5193(05)80344-3
Ikeya, T., Broughton, S., Alic, N., Grandison, R., and Partridge, L. (2009). The endosymbiont Wolbachia increases insulin/IGF-like signalling in Drosophila. Proc. R. Soc. Lond. B Biol. Sci. 276, 3799–3807. doi: 10.1098/rspb.2009.0778
International Glossina Genome Initiative, I. (2014). Genome sequence of the tsetse fly (Glossina morsitans): vector of African Trypanosomiasis. Science 344, 380–386. doi: 10.1126/science.1249656
Isasawin, S., Aketarawong, N., Lertsiri, S., and Thanaphum, S. (2014). Development of a genetic sexing strain in Bactrocera carambolae (Diptera: Tephritidae) by introgression of sex sorting components from B. dorsalis Salaya1 strain. BMC Genet. 15:S2. doi: 10.1186/1471-2156-15-S2-S2
Isasawin, S., Aketarawong, N., and Thanaphum, S. (2012). Characterization and evaluation of microsatellite markers in a strain of the oriental fruit fly, Bactrocera dorsalis (Diptera: Tephritidae), with a genetic sexing character used in sterile insect population control. Eur. J. Entomol. 109, 331–338.
Jamnongluk, W., Kittayapong, P., Baimai, V., and O’Neill, S. L. (2002). Wolbachia infections of tephritid fruit flies: molecular evidence for five distinct strains in a single host species. Curr. Microbiol. 45, 255–260. doi: 10.1007/s00284-002-3746-1
Jeyaprakash, A., and Hoy, M. A. (2000). Long PCR improves Wolbachia DNA amplification: wsp sequences found in 76% of sixty-three arthropod species. Insect Mol. Biol. 9, 393–405. doi: 10.1046/j.1365-2583.2000.00203.x
Johannesen, J. (2017). Tracing the history and ecological context of Wolbachia double infection in a specialist host (Urophora cardui)—parasitoid (Eurytoma serratulae) system. Ecol. Evol. 7, 986–996. doi: 10.1002/ece3.2713
Johannesen, J., Keyghobadi, N., Schuler, H., Stauffer, C., and Vogt, H. (2013). Invasion genetics of American cherry fruit fly in Europe and signals of hybridization with the European cherry fruit fly. Entomol. Exp. Appl. 147, 61–72.
Joubert, D. A., Walker, T., Carrington, L. B., De Bruyne, J. T., Kien, D. H., Hoang Nle, T., et al. (2016). Establishment of a Wolbachia superinfection in Aedes aegypti mosquitoes as a potential approach for future resistance management. PLoS Pathog. 12:e1005434. doi: 10.1371/journal.ppat.1005434
Karimi, J., and Darsouei, R. (2014). Presence of the endosymbiont Wolbachia among some fruit flies (Diptera: Tephritidae) from Iran: a multilocus sequence typing approach. J. Asia Pac. Entomol. 17, 105–112.
Karr, T. L., Yang, W., and Feder, M. E. (1998). Overcoming cytoplasmic incompatibility in Drosophila. Proc. R. Soc. Lond. B Biol. Sci. 265, 391–395. doi: 10.1098/rspb.1998.0307
Kent, B. N., Salichos, L., Gibbons, J. G., Rokas, A., Newton, I. L., Clark, M. E., et al. (2011). Complete bacteriophage transfer in a bacterial endosymbiont (Wolbachia) determined by targeted genome capture. Genome Biol. Evol. 3, 209–218. doi: 10.1093/gbe/evr007
Kerremans, P., and Franz, G. (1995). Use of a temperature-sensitive lethal mutation strain of medfly (Ceratitis capitata) for the suppression of pest populations. Theor. Appl. Genet. 90, 511–518. doi: 10.1007/BF00221997
Kittayapong, P., Milne, J. R., Tigvattananont, S., and Baimai, V. (2000). Distribution of the reproduction-modifying bacteria, Wolbachia, in natural populations of tephritid fruit flies in Thailand. Sci. Asia 26, 93–103.
Klassen, W., and Curtis, C. F. (2005). “History of the sterile insect technique,” in Sterile Insect Technique: Principles And Practice In Area-Wide Integrated Pest Management, eds V. A. Dyck, J. Hendrichs, and A. S. Robinson, (Berlin: Springer), 3–36.
Klasson, L., Westberg, J., Sapountzis, P., Nasiund, K., Lutnaes, Y., Darby, A. C., et al. (2009). The mosaic genome structure of the Wolbachia wRi strain infecting Drosophila simulans. Proc. Natl. Acad. Sci. U.S.A. 106, 5725–5730. doi: 10.1073/pnas.0810753106
Knipling, E. (1955). Possibilities of insect control or eradication through the use of sexually sterile males. J. Econ. Entomol. 48, 459–462.
Koukou, K., Pavlikaki, H., Kilias, G., Werren, J. H., Bourtzis, K., and Alahiotis, S. N. (2006). Influence of antibiotic treatment and Wolbachia curing on sexual isolation among Drosophila melanogaster cage populations. Evolution 60, 87–96.
Kremer, N., Voronin, D., Charif, D., Mavingui, P., Mollereau, B., and Vavre, F. (2009). Wolbachia interferes with ferritin expression and iron metabolism in insects. PLoS Pathog. 5:e1000630. doi: 10.1371/journal.ppat.1000630
Kriesner, P., Conner, W. R., Weeks, A. R., Turelli, M., and Hoffmann, A. A. (2016). Persistence of a Wolbachia infection frequency cline in Drosophila melanogaster and the possible role of reproductive dormancy. Evolution 70, 979–997. doi: 10.1111/evo.12923
Kyritsis, G. A. (2016). Effect of Symbiotic Bacteria On The Biological And Behavioral Traits Of The Mediterranean Fruit Flies (Ceratitis capitata: Diptera, Tephritidae). Ph. D. Dissertation, University of Thessaly, Volos.
Kyritsis, G. A., Augustinos, A. A., Livadaras, I., Cáceres, C., Bourtzis, K., and Papadopoulos, N. T. (2019). Medfly-Wolbachia symbiosis: genotype x genotype interactions determine host’s life history traits under mass rearing conditions. BMC Microbiol. 19:96. doi: 10.1186/s12896-019-0586-7
Laven, H. (1967). Eradication of Culex pipiens fatigans through cytoplasmic incompatibility. Nature 216, 383–384. doi: 10.1038/216383a0
Layton, E. M., On, J., Perlmutter, J. I., Bordenstein, S. R., and Shropshire, J. D. (2019). Paternal grandmother age affects the strength of Wolbachia-Induced cytoplasmic incompatibility in Drosophila melanogaster. MBio 10:1879. doi: 10.1128/mBio.01879-19
Lees, R. S., Gilles, J. R., Hendrichs, J., Vreysen, M. J., and Bourtzis, K. (2015). Back to the future: the sterile insect technique against mosquito disease vectors. Curr. Opin. Insect Sci. 10, 156–162. doi: 10.1016/j.cois.2015.05.011
Leftwich, P. T., Clarke, N. V. E., Hutchings, M. I., and Chapman, T. (2017). Gut microbiomes and reproductive isolation in Drosophila. Proc. Natl. Acad. Sci. U.S.A. 114, 12767–12772. doi: 10.1073/pnas.1708345114
Leftwich, P. T., Hutchings, M. I., and Chapman, T. (2018). Diet, gut microbes and host mate choice: understanding the significance of microbiome effects on host mate choice requires a case by case evaluation. Bioessays 40:e1800053. doi: 10.1002/bies.201800053
Leftwich, P. T., Koukidou, M., Rempoulakis, P., Gong, H. F., Zacharopoulou, A., Fu, G., et al. (2014). Genetic elimination of field-cage populations of Mediterranean fruit flies. Proc. R. Soc. Lond. B Biol. Sci. 281, 1372. doi: 10.1098/rspb.2014.1372
Lemmon, E. M., and Lemmon, A. R. (2013). High-throughput genomic data in systematics and phylogenetics. Annu. Rev. Ecol. Evol. Syst. 44, 99–121.
LePage, D. P., Metcalf, J. A., Bordenstein, S. R., On, J., Perlmutter, J. I., Shropshire, J. D., et al. (2017). Prophage WO genes recapitulate and enhance Wolbachia-induced cytoplasmic incompatibility. Nature 543, 243–247. doi: 10.1038/nature21391
Li, Y.-Y., Floate, K., Fields, P., and Pang, B.-P. (2014). Review of treatment methods to remove Wolbachia bacteria from arthropods. Symbiosis 62, 1–15.
Lindsey, A. R. I., Rice, D. W., Bordenstein, S. R., Brooks, A. W., Bordenstein, S. R., and Newton, I. L. G. (2018). Evolutionary genetics of cytoplasmic incompatibility genes cifA and cifB in prophage WO of Wolbachia. Genome Biol. Evol. 10, 434–451. doi: 10.1093/gbe/evy012
Lutrat, C., Giesbrecht, D., Marois, E., Whyard, S., Baldet, T., and Bouyer, J. (2019). Sex sorting for pest control: it’s raining men! Trends Parasitol. 35, 649–662. doi: 10.1016/j.pt.2019.06.001
Marcon, H. S., Coscrato, V. E., Selivon, D., Perondini, A. L. P., and Marino, C. L. (2011). Variations in the sensitivity of different primers for detecting Wolbachia in Anastrepha (Diptera: Tephritidae). Braz. J. Microbiol. 42, 778–785. doi: 10.1590/S1517-838220110002000046
Markow, T. A., and O’Grady, P. M. (2005). Drosophila: A Guide To Species Identification And Use. London: Academic Press.
Marshall, J. L. (2007). Rapid evolution of spermathecal duct length in the Allonemobius socius complex of crickets: species, population and Wolbachia effects. PLoS One 2:e720. doi: 10.1371/journal.pone.0000720
Martinez, H., Morán-Aceves, B. M., Toledo, J., Liedo, P., Guillén, K., Palomeque, M. A., et al. (2016). Attempts to Transfer Wolbachia from Anastrepha striata to Anastrepha ludens, in IAEA-D41024-CR-3 Report of the Third Research Coordination Meeting of an FAO/IAEA Coordinated Research Project “Use of Symbiotic Bacteria to Reduce Mass-Rearing Costs and Increase Mating Success in Selected Fruit Pests in Support of SIT Application”, Antigua, Guatemala, 26–30 October 2015. (Austria, Vienna: IAEA), 144. Available: http://www-naweb.iaea.org/nafa/ipc/crp/RCM3-Use-Symbiotic-Bacteria-Report.pdf (accessed March 13, 2020).
Martínez, H., Toledo, J., Liedo, P., and Mateos, M. (2012). Survey of heritable endosymbionts in southern Mexico populations of the fruit fly species Anastrepha striata and A. ludens. Curr. Microbiol. 65, 711–718. doi: 10.1007/s00284-012-0223-3
Martinez, J., Longdon, B., Bauer, S., Chan, Y. S., Miller, W. J., Bourtzis, K., et al. (2014). Symbionts commonly provide broad spectrum resistance to viruses in insects: a comparative analysis of Wolbachia strains. PLoS Pathog. 10:e1004369. doi: 10.1371/journal.ppat.1004369
Mascarenhas, R. O., Prezotto, L. F., Perondini, A. L. P., Marino, C. L., and Selivon, D. (2016). Wolbachia in guilds of Anastrepha fruit flies (Tephritidae) and parasitoid wasps (Braconidae). Genet. Mol. Biol. 39, 600–610. doi: 10.1590/1678-4685-GMB-2016-0075
Mateos, M., Castrezana, S., Nankivell, B., Estes, A., Markow, T. A., and Moran, N. A. (2006). Heritable endosymbionts of Drosophila. Genetics 174, 363–376.
McInnis, D., Lance, D., and Jackson, C. (1996). Behavioral resistance to the sterile insect technique by Mediterranean fruit fly (Diptera: Tephritidae) in Hawaii. Ann. Entomol. Soc. Am. 89, 739–744.
McInnis, D., Tam, S., Lim, R., Komatsu, J., Kurashima, R., and Albrecht, C. (2004). Development of a pupal color-based genetic sexing strain of the melon fly, Bactrocera cucurbitae (Coquillett)(Diptera: Tephritidae). Ann. Entomol. Soc. Am. 97, 1026–1033.
McInnis, D. O., Shelly, T. E., and Komatsu, J. (2002). Improving male mating competitiveness and survival in the field for medfly, Ceratitis capitata (Diptera: Tephritidae) SIT programs. Genetica 116, 117–124. doi: 10.1023/a:1020919927542
McLean, A. H. C., Parker, B. J., Hrcek, J., Kavanagh, J. C., Wellham, P. A. D., and Godfray, H. C. J. (2018). Consequences of symbiont co-infections for insect host phenotypes. J. Anim. Ecol. 87, 478–488. doi: 10.1111/1365-2656.12705
Meats, A., Pike, N., An, X., Raphael, K., and Wang, W. (2003). The effects of selection for early (day) and late (dusk) mating lines of hybrids of Bactrocera tryoni and Bactrocera neohumeralis. Genetica 119, 283–293. doi: 10.1023/b:gene.0000003683.42395.51
Mee, P. T., Weeks, A. R., Walker, P. J., Hoffmann, A. A., and Duchemin, J. B. (2015). Detection of low-level Cardinium and Wolbachia infections in Culicoides. Appl. Environ. Microbiol. 81, 6177–6188. doi: 10.1128/AEM.01239-15
Mengual, X., Kerr, P., Norrbom, A. L., Barr, N. B., Lewis, M. L., Stapelfeldt, A. M., et al. (2017). Phylogenetic relationships of the tribe Toxotrypanini (Diptera: Tephritidae) based on molecular characters. Mol. Phylogenet. Evol. 113, 84–112. doi: 10.1016/j.ympev.2017.05.011
Menon, A., Varma, V., and Sharma, V. K. (2014). Rhythmic egg-laying behaviour in virgin females of fruit flies Drosophila melanogaster. Chronobiol. Int. 31, 433–441. doi: 10.3109/07420528.2013.866131
Miller, G. T., and Pitnick, S. (2002). Sperm female coevolution in Drosophila. Science 298, 1230–1233.
Miller, W. J., Ehrman, L., and Schneider, D. (2010). Infectious speciation revisited: impact of symbiont-depletion on female fitness and mating behavior of Drosophila paulistorum. PLoS Pathog. 6:e1001214. doi: 10.1371/journal.ppat.1001214
Min, K.-T., and Benzer, S. (1997). Wolbachia, normally a symbiont of Drosophila, can be virulent, causing degeneration and early death. Proc. Natl. Acad. Sci. U.S.A. 94, 10792–10796. doi: 10.1073/pnas.94.20.10792
Moran, N. A., and Dunbar, H. E. (2006). Sexual acquisition of beneficial symbionts in aphids. Proc. Natl. Acad. Sci. U.S.A. 103, 12803–12806. doi: 10.1073/pnas.0605772103
Morán-Aceves, B. M. (2016). Prevalencia de Wolbachia en Anastrepha serpentina (Diptera: Tephritidae) De México. BsC thesis, Universidad Autónoma de Chiapas, Tapachula.
Moreira, L. A., Saig, E., Turley, A. P., Ribeiro, J. M., O’Neill, S. L., and McGraw, E. A. (2009). Human probing behavior of Aedes aegypti when infected with a life-shortening strain of Wolbachia. PLoS Negl. Trop. Dis. 3:e568. doi: 10.1371/journal.pntd.0000568
Moretti, R., Marzo, G. A., Lampazzi, E., and Calvitti, M. (2018a). Cytoplasmic incompatibility management to support Incompatible Insect Technique against Aedes albopictus. Parasit. Vectors 11:649. doi: 10.1186/s13071-018-3208-7
Moretti, R., Yen, P.-S., Houé, V., Lampazzi, E., Desiderio, A., Failloux, A.-B., et al. (2018b). Combining Wolbachia-induced sterility and virus protection to fight Aedes albopictus-borne viruses. PLoS Negl. Trop. Dis. 12:e0006626. doi: 10.1371/journal.pntd.0006626
Morrow, J., Scott, L., Congdon, B., Yeates, D., Frommer, M., and Sved, J. (2000). Close genetic similarity between two sympatric species of tephritid fruit fly reproductively isolated by mating time. Evolution 54, 899–910. doi: 10.1111/j.0014-3820.2000.tb00090.x
Morrow, J. L., Frommer, M., Royer, J. E., Shearman, D. C., and Riegler, M. (2015). Wolbachia pseudogenes and low prevalence infections in tropical but not temperate Australian tephritid fruit flies: manifestations of lateral gene transfer and endosymbiont spillover? BMC Evol. Biol. 15:202. doi: 10.1186/s12862-015-0474-2
Morrow, J. L., Frommer, M., Shearman, D., and Riegler, M. (2014). Tropical tephritid fruit fly community with high incidence of shared Wolbachia strains as platform for horizontal transmission of endosymbionts. Environ. Microbiol. 16, 3622–3637. doi: 10.1111/1462-2920.12382
Morrow, J. L., Schneider, D. I., Klasson, L., Janitz, C., Miller, W. J., and Riegler, M. (2020). Parallel sequencing of Wolbachia wCer2 from donor and novel hosts reveals multiple incompatibility factors and genome stability after host transfers. Genome Biol. Evol. 2020:evaa050. doi: 10.1093/gbe/evaa050
Murdock, C. C., Blanford, S., Hughes, G. L., Rasgon, J. L., and Thomas, M. B. (2014). Temperature alters Plasmodium blocking by Wolbachia. Sci. Rep. 4:3932. doi: 10.1038/srep03932
Murillo, F. D., Liedo, P., Nieto-Lopez, M. G., Cabrera-Mireles, H., Barrera, J. F., and Montoya, P. (2016). First instar larvae morphology of Opiinae (Hymenoptera: Braconidae) parasitoids of Anastrepha (Diptera: Tephritidae) fruit flies. Implications for interspecific competition. Arthropod. Struct. Dev. 45, 294–300. doi: 10.1016/j.asd.2016.01.003
Newton, I. L., Savytskyy, O., and Sheehan, K. B. (2015). Wolbachia utilize host actin for efficient maternal transmission in Drosophila melanogaster. PLoS Pathog. 11:e1004798. doi: 10.1371/journal.ppat.1004798
Newton, I. L. G., and Rice, D. W. (2020). The Jekyll and Hyde symbiont: could Wolbachia be a nutritional mutualist? J. Bacteriol. 202:e00589-19. doi: 10.1128/JB.00589-19
Nikoh, N., Tanaka, K., Shibata, F., Kondo, N., Hizume, M., Shimada, M., et al. (2008). Wolbachia genome integrated in an insect chromosome: evolution and fate of laterally transferred endosymbiont genes. Genome Res. 18, 272–280. doi: 10.1101/gr.7144908
Nikolouli, K., Sassù, F., Mouton, L., Stauffer, C., and Bourtzis, K. (2020). Combining sterile and incompatible insect techniques for the population suppression of Drosophila suzukii. J. Pest Sci. 93, 647–661. doi: 10.1007/s10340-020-01199-6
Noman, M. S., Liu, L., Bai, Z., and Li, Z. (2019). Tephritidae bacterial symbionts: potentials for pest management. Bull. Entomol. Res. 110, 1–14. doi: 10.1017/S0007485319000403
Norrbom, A. L. (2004a). Fruit Fly (Diptera: Tephritidae) Phylogeny. Maryland: Agricultural Research Service.
Norrbom, A. L. (2004b). Updates to Biosystematic Database of World Diptera for Tephritidae through 1999”. Diptera Data Dissemination Disk (CD-ROM) 2). Washington, DC: USDA.
Norrbom, A. L. (2010). Tephritidae (fruit flies, moscas de frutas). Man. Central Am. Diptera 2, 909–954.
Ogaugwu, C. E., and Durvasula, R. V. (2017). “Developing the arsenal against pest and vector dipterans: inputs of transgenic and paratransgenic biotechnologies,” in Biological Control of Pest and Vector Insects, ed. V. D. C. Shields, (Rijeka: InTech), 325–347.
O’Neill, S. L., Giordano, R., Colbert, A. M., Karr, T. L., and Robertson, H. M. (1992). 16S rRNA phylogenetic analysis of the bacterial endosymbionts associated with cytoplasmic incompatibility in insects. Proc. Natl. Acad. Sci. U.S.A. 89, 2699–2702. doi: 10.1073/pnas.89.7.2699
Pane, A., Salvemini, M., Delli Bovi, P., Polito, C., and Saccone, G. (2002). The transformer gene in Ceratitis capitata provides a genetic basis for selecting and remembering the sexual fate. Development 129, 3715–3725.
Paraskevopoulos, C., Bordenstein, S. R., Wernegreen, J. J., Werren, J. H., and Bourtzis, K. (2006). Toward a Wolbachia multilocus sequence typing system: discrimination of Wolbachia strains present in Drosophila species. Curr. Microbiol. 53, 388–395. doi: 10.1007/s00284-006-0054-1
Pike, N., Wang, W., and Meats, A. (2003). The likely fate of hybrids of Bactrocera tryoni and Bactrocera neohumeralis. Heredity 90, 365–370. doi: 10.1038/sj.hdy.6800253
Presgraves, D. C. (2000). A genetic test of the mechanism of Wolbachia-induced cytoplasmic incompatibility in Drosophila. Genetics 154, 771–776.
Prezotto, L. F., Perondini, A. L. P., Hernandez-Ortiz, V., Marino, C. L., and Selivon, D. (2017). Wolbachia strains in cryptic species of the Anastrepha fraterculus complex (Diptera, Tephritidae) along the neotropical region. Syst. Appl. Microbiol. 40, 59–67. doi: 10.1016/j.syapm.2016.11.002
Quilici, S., and Rousse, P. (2012). Location of host and host habitat by fruit fly parasitoids. Insects 3, 1220–1235. doi: 10.3390/insects3041220
Quintero-Fong, L., Toledo, J., Ruiz, L., Rendón, P., Orozco-Dávila, D., Cruz, L., et al. (2016). Selection by mating competitiveness improves the performance of Anastrepha ludens males of the genetic sexing strain Tapachula-7. Bull. Entomol. Res. 106, 624–632. doi: 10.1017/S0007485316000316
Rasgon, J. L. (2008). Using predictive models to optimize Wolbachia-based strategies for vector-borne disease control. Adv. Exp. Med. Biol. 627, 114–125. doi: 10.1007/978-0-387-78225-6_10
Rasgon, J. L., Gamston, C. E., and Ren, X. (2006). Survival of Wolbachia pipientis in cell-free medium. Appl. Environ. Microbiol. 72, 6934–6937. doi: 10.1128/AEM.01673-06
Raza, M. F., Yao, Z., Bai, S., Cai, Z., and Zhang, H. (2020). Tephritidae fruit fly gut microbiome diversity, function and potential for applications. Bull. Entomol. Res. 5, 1–15. doi: 10.1017/S0007485319000853
Reid, W., and O’Brochta, D. A. (2016). Applications of genome editing in insects. Curr. Opin. Insect Sci. 13, 43–54. doi: 10.1016/j.cois.2015.11.001
Rempoulakis, P., Castro, R., Nemny-Lavy, E., and Nestel, D. (2015). Effects of radiation on the fertility of the Ethiopian fruit fly, Dacus ciliatus. Entomol. Exp. Appl. 155, 117–122.
Rendón, P., McInnis, D., Lance, D., and Stewart, J. (2004). Medfly (Diptera: Tephritidae) genetic sexing: large-scale field comparison of males-only and bisexual sterile fly releases in Guatemala. J. Econ. Entomol. 97, 1547–1553. doi: 10.1603/0022-0493-97.5.1547
Reyes, J., Santiago, G., and Hernández, P. (2000). “The Mexican fruit fly eradication programme,” in Area-Wide Control of Fruit Flies and Other Insect Pests, ed. K. H. Tan, (Penang, MA: Penerbit Universiti Sains Malaysia), 377–380.
Reynolds, K. T., and Hoffmann, A. A. (2002). Male age, host effects and the weak expression or non-expression of cytoplasmic incompatibility in Drosophila strains infected by maternally transmitted Wolbachia. Genet. Res. 80, 79–87. doi: 10.1017/s0016672302005827
Ribeiro, R. M. (2009). Wolbachia e Incompatibilidade Citoplasmática em Anastrepha sp. 1 aff. fraterculus e bliqua (Diptera: Tephritidae). Ph. D. thesis, Universidade de São Paulo, Brazil.
Riegler, M., Charlat, S., Stauffer, C., and Mercot, H. (2004). Wolbachia transfer from Rhagoletis cerasi to Drosophila simulans: investigating the outcomes of host-symbiont coevolution. Appl. Environ. Microbiol. 70, 273–279. doi: 10.1128/aem.70.1.273-279.2004
Riegler, M., Iturbe-Ormaetxe, I., Woolfit, M., Miller, W. J., and O’Neill, S. L. (2012). Tandem repeat markers as novel diagnostic tools for high resolution fingerprinting of Wolbachia. BMC Microbiol. 12(Suppl. 1):S12. doi: 10.1186/1471-2180-12-S1-S12
Riegler, M., and Stauffer, C. (2002). Wolbachia infections and superinfections in cytoplasmically incompatible populations of the European cherry fruit fly Rhagoletis cerasi (Diptera:Tephritidae). Mol. Ecol. 11, 2425–2434. doi: 10.1046/j.1365-294x.2002.01614.x
Ringo, J., Sharon, G., and Segal, D. (2011). Bacteria-induced sexual isolation in Drosophila. Fly (Austin) 5, 310–315. doi: 10.4161/fly.5.4.15835
Robinson, A., and Hendrichs, J. (2005). “Prospects for the future development and application of the sterile insect technique,” in Sterile Insect Technique: Principles and Practice in Area-Wide Integrated Pest Management, eds V. A. Dyck, J. Hendrichs, and A. S. Robinson, (Berlin: Springer), 727–760. doi: 10.1017/s1431927603030344
Robinson, A. S. (2002a). Genetic sexing strains in medfly, Ceratitis capitata, sterile insect technique programmes. Genetica 116, 5–13. doi: 10.1023/a:1020951407069
Robinson, A. S. (2002b). Mutations and their use in insect control. Mutation Res. 511, 113–132. doi: 0.1016/S1383-5742(02)00006-6
Rocha, L. S., Mascarenhas, R. O., Perondini, A. L. P., and Selivon, D. (2005). Occurrence of Wolbachia in Brazilian samples of Ceratitis capitata (Wiedemann) (Diptera: Tephritidae). Neotrop. Entomol. 34, 1013–1015.
Roriz, A. K. P., Japyassú, H. F., and Joachim-Bravo, I. S. (2017). Incipient speciation in the Anastrepha fraterculus cryptic species complex: reproductive compatibility between A. sp. 1 aff. fraterculus and A. sp. 3 aff. fraterculus. Entomol. Exp. Appl. 162, 346–357.
Ros, V. I., Fleming, V. M., Feil, E. J., and Breeuwer, J. A. (2009). How diverse is the genus Wolbachia? Multiple-gene sequencing reveals a putatively new Wolbachia supergroup recovered from spider mites (Acari: Tetranychidae). Appl. Environ. Microbiol. 75, 1036–1043. doi: 10.1128/AEM.01109-08
Ros, V. I., Fleming, V. M., Feil, E. J., and Breeuwer, J. A. (2012). Diversity and recombination in Wolbachia and Cardinium from Bryobia spider mites. BMC Microbiol. 12(Suppl. 1):S13. doi: 10.1186/1471-2180-12-S1-S13
Ross, P. A., Ritchie, S. A., Axford, J. K., and Hoffmann, A. A. (2019a). Loss of cytoplasmic incompatibility in Wolbachia-infected Aedes aegypti under field conditions. PLoS Negl. Trop. Dis. 13:e0007357. doi: 10.1371/journal.pntd.0007357
Ross, P. A., Turelli, M., and Hoffmann, A. A. (2019b). Evolutionary ecology of Wolbachia releases for disease control. Annu. Rev. Genet. 53:609. doi: 10.1146/annurev-genet-112618-043609
Rousset, F., Raymond, M., and Kjellberg, F. (1991). Cytoplasmic incompatibilities in the mosquito Culex pipiens: how to explain a cytotype polymorphism? J. Evol. Biol. 4, 69–81.
Rull, J., Aluja, M., and Feder, J. L. (2010). Evolution of intrinsic reproductive isolation among four North American populations of Rhagoletis pomonella (Diptera: Tephritidae). Biol. J. Linn. Soc. 100, 213–223.
Rull, J., Diaz-Fleischer, F., and Arredondo, J. (2007). Irradiation of Anastrepha ludens (Diptera: Tephritidae) revisited: optimizing sterility induction. J. Econ. Entomol. 100, 1153–1159. doi: 10.1603/0022-0493(2007)100[1153:ioaldt]2.0.co;2
Rull, J., Tadeo, E., Aluja, M., Guillen, L., Egan, S. P., and Feder, J. L. (2012). Hybridization and sequential components of reproductive isolation between parapatric walnut-infesting sister species Rhagoletis completa and Rhagoletis zoqui. Biol. J. Linn. Soc. 107, 886–898.
Rull, J., Tadeo, E., Lasa, R., Rodríguez, C. L., Altuzar-Molina, A., and Aluja, M. (2018). Experimental hybridization and reproductive isolation between two sympatric species of tephritid fruit flies in the Anastrepha fraterculus species group. Insect Sci. 6, 1045–1055. doi: 10.1111/1744-7917.12489
Sacchi, L., Genchi, M., Clementi, E., Negri, I., Alma, A., Ohler, S., et al. (2010). Bacteriocyte-like cells harbour Wolbachia in the ovary of Drosophila melanogaster (Insecta, Diptera) and Zyginidia pullula (Insecta, Hemiptera). Tissue Cell 42, 328–333. doi: 10.1016/j.tice.2010.07.009
Sánchez-Rosario, M., Pérez-Staples, D., Toledo, J., Valle-Mora, J., and Liedo, P. (2017). Artificial selection on mating competitiveness of Anastrepha ludens for sterile insect technique application. Entomol. Exp. Appl. 162, 133–147.
Sarakatsanou, A., Diamantidis, A. D., Papanastasiou, S. A., Bourtzis, K., and Papadopoulos, N. T. (2011). Effects of Wolbachia on fitness of the Mediterranean fruit fly (Diptera: Tephritidae). J. Appl. Entomol. 135, 554–563.
Saridaki, A., and Bourtzis, K. (2010). Wolbachia: more than just a bug in insects genitals. Curr. Opin. Microbiol. 13, 67–72. doi: 10.1016/j.mib.2009.11.005
Schebeck, M., Feldkirchner, L., Stauffer, C., and Schuler, H. (2019). Dynamics of an ongoing Wolbachia spread in the European cherry fruit fly, Rhagoletis cerasi (Diptera: Tephritidae). Insects 10:172. doi: 10.3390/insects10060172
Schneider, D., Miller, W. J., and Riegler, M. (2011). “Arthropods shopping for Wolbachia,” in Manipulative Tenants Bacteria Associated with Arthropods, eds E. Zchori-Fein and K. Bourtzis, (Boca Raton, Fl: CRC Press), 149–173.
Schneider, D. I., Ehrman, L., Engl, T., Kaltenpoth, M., Hua-Van, A., Le Rouzic, A., et al. (2019). Symbiont-driven male mating success in the neotropical Drosophila paulistorum superspecies. Behav. Genet. 49, 83–98. doi: 10.1007/s10519-018-9937-8
Schneider, D. I., Klasson, L., Lind, A. E., and Miller, W. J. (2014). More than fishing in the dark: PCR of a dispersed sequence produces simple but ultrasensitive Wolbachia detection. BMC Microbiol. 14:121. doi: 10.1186/1471-2180-14-121
Schuler, H., Arthofer, W., Krumböck, S., Bertheau, C., and Stauffer, C. (2012). Wolbachia infection in the Walnut-husk fly Rhagoletis completa Cresson 1929 (Diptera: Tephritidae). Mitt. Dtsch. Ges. Allg. Angew. Entomol. 18, 243–245.
Schuler, H., Arthofer, W., Riegler, M., Bertheau, C., Krumbock, S., Koppler, K., et al. (2011). Multiple Wolbachia infections in Rhagoletis pomonella. Entomol. Exp. Appl. 139, 138–144. doi: 10.1111/j.1570-7458.2011.01115.x
Schuler, H., Bertheau, C., Egan, S. P., Feder, J. L., Riegler, M., Schlick-Steiner, B. C., et al. (2013). Evidence for a recent horizontal transmission and spatial spread of Wolbachia from endemic Rhagoletis cerasi (Diptera: Tephritidae) to invasive Rhagoletis cingulata in Europe. Mol. Ecol. 22, 4101–4111. doi: 10.1111/mec.12362
Schuler, H., Kern, P., Arthofer, W., Vogt, H., Fischer, M., Stauffer, C., et al. (2016a). Wolbachia in parasitoids attacking native European and introduced eastern cherry fruit flies in Europe. Environ. Entomol. 45, 1424–1431. doi: 10.1093/ee/nvw137
Schuler, H., Koppler, K., Daxbock-Horvath, S., Rasool, B., Krumbock, S., Schwarz, D., et al. (2016b). The hitchhiker’s guide to Europe: the infection dynamics of an ongoing Wolbachia invasion and mitochondrial selective sweep in Rhagoletis cerasi. Mol. Ecol. 25, 1595–1609. doi: 10.1111/mec.13571
Schutze, M., Jessup, A., Ul-Haq, I., Vreysen, M., Wornoayporn, V., Vera, M. T., et al. (2013). Mating compatibility among four pest members of the Bactrocera dorsalis fruit fly species complex (Diptera: Tephritidae). J. Econ. Entomol. 106, 695–707. doi: 10.1603/ec12409
Schutze, M. K., Aketarawong, N., Amornsak, W., Armstrong, K. F., Augustinos, A. A., Barr, N., et al. (2015). Synonymization of key pest species within the Bactrocera dorsalis species complex (Diptera: Tephritidae): taxonomic changes based on a review of 20 years of integrative morphological, molecular, cytogenetic, behavioural and chemoecological data. Syst. Entomol. 40, 456–471.
Schwarz, D., and McPheron, B. A. (2007). When ecological isolation breaks down: sexual isolation is an incomplete barrier to hybridization between Rhagoletis species. Evol. Ecol. Res. 9, 829–841.
Segura, D. F., Vera, M. T., Rull, J., Wornoayporn, V., Islam, A., and Robinson, A. S. (2011). Assortative mating among Anastrepha fraterculus (Diptera: Tephritidae) hybrids as a possible route to radiation of the fraterculus cryptic species complex. Biol. J. Linn. Soc. 102, 346–354.
Selivon, D., Perondini, A., and Morgante, J. (2005). A genetic–morphological characterization of two cryptic species of the Anastrepha fraterculus complex (Diptera: Tephritidae). Ann. Entomol. Soc. Am. 98, 367–381.
Selivon, D., Perondini, A. L., and Morgante, J. S. (1999). Haldane’s rule and other aspects of reproductive isolation observed in the Anastrepha fraterculus complex (Diptera: Tephritidae). Genet. Mol. Biol. 22, 507–510.
Selivon, D., Perondini, A. L. P., Ribeiro, A. F., Marino, C. L., Lima, M. M. A., and Coscrato, V. E. (2002). Wolbachia endosymbiont in a species of the Anastrepha fraterculus complex (Diptera : Tephritidae). Invertebr. Reprod. Dev. 42, 121–127.
Serbus, L. R., White, P. M., Silva, J. P., Rabe, A., Teixeira, L., Albertson, R., et al. (2015). The impact of host diet on Wolbachia titer in Drosophila. PLoS Pathog. 11:e1004777. doi: 10.1371/journal.ppat.1004777
Sharon, G., Segal, D., Ringo, J. M., Hefetz, A., Zilber-Rosenberg, I., and Rosenberg, E. (2010). Commensal bacteria play a role in mating preference of Drosophila melanogaster. Proc. Natl. Acad. Sci. U.S.A. 107, 20051–20056. doi: 10.1073/pnas.1009906107
Shaw, W. R., Marcenac, P., Childs, L. M., Buckee, C. O., Baldini, F., Sawadogo, S. P., et al. (2016). Wolbachia infections in natural Anopheles populations affect egg laying and negatively correlate with Plasmodium development. Nat. Commun. 7:11772. doi: 10.1038/ncomms11772
Shearman, D., Frommer, M., Morrow, J., Raphael, K., and Gilchrist, A. (2010). Interspecific hybridization as a source of novel genetic markers for the sterile insect technique in Bactrocera tryoni (Diptera: Tephritidae). J. Econ. Entomol. 103, 1071–1079. doi: 10.1603/ec09241
Shropshire, J. D., and Bordenstein, S. R. (2019). Two-By-One model of cytoplasmic incompatibility: Synthetic recapitulation by transgenic expression of cifA and cifB in Drosophila. PLoS Genet. 15:e1008221. doi: 10.1371/journal.pgen.1008221
Shropshire, J. D., Leigh, B., Bordenstein, S. R., Duplouy, A., Riegler, M., Brownlie, J. C., et al. (2019). Models and nomenclature for cytoplasmic incompatibility: caution over premature conclusions - a response to Beckmann et al. Trends Genet. 35, 397–399. doi: 10.1016/j.tig.2019.03.004
Shropshire, J. D., On, J., Layton, E. M., Zhou, H., and Bordenstein, S. R. (2018). One prophage WO gene rescues cytoplasmic incompatibility in Drosophila melanogaster. Proc. Natl. Acad. Sci. U.S.A. 115, 4987–4991. doi: 10.1073/pnas.1800650115
Sicard, M., Bonneau, M., and Weill, M. (2019). Wolbachia prevalence, diversity, and ability to induce cytoplasmic incompatibility in mosquitoes. Curr. Opin. Insect Sci. 34, 12–20. doi: 10.1016/j.cois.2019.02.005
Sikulu-Lord, M. T., Maia, M. F., Milali, M. P., Henry, M., Mkandawile, G., Kho, E. A., et al. (2016). Rapid and non-destructive detection and identification of two strains of Wolbachia in Aedes aegypti by near-infrared spectroscopy. PLoS Negl. Trop. Dis. 10:e0004759. doi: 10.1371/journal.pntd.0004759
Simhadri, R. K., Fast, E. M., Guo, R., Schultz, M. J., Vaisman, N., Ortiz, L., et al. (2017). The gut commensal microbiome of Drosophila melanogaster is modified by the endosymbiont Wolbachia. mSphere 2:e00287. doi: 10.1128/mSphere.00287-17
Simoes, P. M., Mialdea, G., Reiss, D., Sagot, M. F., and Charlat, S. (2011). Wolbachia detection: an assessment of standard PCR protocols. Mol. Ecol. Resour. 11, 567–572. doi: 10.1111/j.1755-0998.2010.02955.x
Singh, N. D. (2019). Wolbachia infection associated with increased recombination in Drosophila. G3 9, 229–237. doi: 10.1534/g3.118.200827
Smith, P. H. (1979). Genetic manipulation of the circadian clock’s timing of sexual behaviour in the Queensland fruit flies, Dacus tryoni and Dacus neohumeralis. Physiol. Entomol. 4, 71–78.
Snook, R. R., Cleland, S. Y., Wolfner, M. F., and Karr, T. L. (2000). Offsetting effects of Wolbachia infection and heat shock on sperm production in Drosophila simulans: analyses of fecundity, fertility and accessory gland proteins. Genetics 155, 167–178.
Suckling, D. M., Kean, J. M., Stringer, L. D., Caceres-Barrios, C., Hendrichs, J., Reyes-Flores, J., et al. (2016). Eradication of tephritid fruit fly pest populations: outcomes and prospects. Pest Manag. Sci. 72, 456–465. doi: 10.1002/ps.3905
Suh, E., Mercer, D. R., and Dobson, S. L. (2017). Life-shortening Wolbachia infection reduces population growth of Aedes aegypti. Acta Trop. 172, 232–239. doi: 10.1016/j.actatropica.2017.05.015
Sullivan, W., and O’Neill, S. L. (2017). Microbiology: manipulation of the manipulators. Nature 543, 182–183. doi: 10.1038/nature21509
Sumida, Y., Katsuki, M., Okada, K., Okayama, K., and Lewis, Z. (2017). Wolbachia induces costs to life-history and reproductive traits in the moth, Ephestia kuehniella. J. Stored Prod. Res. 71, 93–98.
Tadeo, E., Feder, J. L., Egan, S. P., Schuler, H., Aluja, M., and Rull, J. (2015). Divergence and evolution of reproductive barriers among three allopatric populations of Rhagoletis cingulata across eastern North America and Mexico. Entomol. Exp. Appl. 156, 301–311.
Teixeira, L., Ferreira, A., and Ashburner, M. (2008). The bacterial symbiont Wolbachia induces resistance to RNA viral infections in Drosophila melanogaster. PLoS Biol. 6:2753–2763. doi: 10.1371/journal.pbio.1000002
Toledo, J., Rull, J., Oropeza, A., Hernandez, E., and Liedo, P. (2004). Irradiation of Anastrepha obliqua (Diptera: Tephritidae) revisited: optimizing sterility induction. J. Econ. Entomol. 97, 383–389. doi: 10.1093/jee/97.2.383
Tormos, J., Asis, J., Sabater-Muñoz, B., Baños, L., Gayubo, S., and Beitia, F. (2012). Superparasitism in laboratory rearing of Spalangia cameroni (Hymenoptera: Pteromalidae), a parasitoid of medfly (Diptera: Tephritidae). Bull. Entomol. Res. 102, 51–61. doi: 10.1017/S0007485311000393
Turelli, M., and Hoffmann, A. (1999). Microbe-induced cytoplasmic incompatibility as a mechanism for introducing transgenes into arthropod populations. Insect Mol. Biol. 8, 243–255. doi: 10.1046/j.1365-2583.1999.820243.x
Turelli, M., and Hoffmann, A. A. (1991). Rapid spread of an inherited incompatibility factor in California Drosophila. Nature 353, 440–442. doi: 10.1038/353440a0
Turelli, M., and Hoffmann, A. A. (1995). Cytoplasmic incompatibility in Drosophila simulans: dynamics and parameter estimates from natural populations. Genetics 140, 1319–1338.
Uribe-Alvarez, C., Chiquete-Felix, N., Morales-Garcia, L., Bohorquez-Hernandez, A., Delgado-Buenrostro, N. L., Vaca, L., et al. (2018). Wolbachia pipientis grows in Saccharomyces cerevisiae evoking early death of the host and deregulation of mitochondrial metabolism. Microbiol. Open 7:e00675. doi: 10.1002/mbo3.675
Vasquez, C. J., Stouthamer, R., Jeong, G., and Morse, J. G. (2011). Discovery of a CI-inducing Wolbachia and its associated fitness costs in the biological control agent Aphytis melinus DeBach (Hymenoptera: Aphelinidae). Biol. Control 58, 192–198.
Veneti, Z., Clark, M. E., Zabalou, S., Karr, T. L., Savakis, C., and Bourtzis, K. (2003). Cytoplasmic incompatibility and sperm cyst Infection in different Drosophila-Wolbachia associations. Genetics 164, 545–552.
Virgilio, M., Delatte, H., Quilici, S., Backeljau, T., and De Meyer, M. (2013). Cryptic diversity and gene flow among three African agricultural pests: Ceratitis rosa, Ceratitis fasciventris and Ceratitis anonae (Diptera, Tephritidae). Mol. Ecol. 22, 2526–2539. doi: 10.1111/mec.12278
Wang, M., Beck, C. R., English, A. C., Meng, Q., Buhay, C., Han, Y., et al. (2015). PacBio-LITS: a large-insert targeted sequencing method for characterization of human disease-associated chromosomal structural variations. BMC Genomics 16:214. doi: 10.1186/s12864-015-1370-2
Wang, X., Li, Z., Zhang, R., He, J., Zhao, Z., Wei, S., et al. (2019). Wolbachia infection of Neoceratitis asiatica (Diptera: Tephritidae). Fla. Entomol. 102, 125–129.
Wedell, N. (2013). The dynamic relationship between polyandry and selfish genetic elements. Philos. Trans. R. Soc. Lond. B Biol. Sci. 368:20120049. doi: 10.1098/rstb.2012.0049
Wedell, N. (2019). “The effect of non-self genes on the behaviour of hosts,” in Genes and Behaviour: Beyond Nature-Nurture, eds D. J. Hosken, J. Hunt, and N. Wedell (Chichester: John Wiley & Sons). 157–180.
Wee, S.-L., and Tan, K.-H. (2005). Evidence of natural hybridization between two sympatric sibling species of Bactrocera dorsalis complex based on pheromone analysis. J. Chem. Ecol. 31, 845–858. doi: 10.1007/s10886-005-3548-6
Weeks, A. R., Turelli, M., Harcombe, W. R., Reynolds, K. T., and Hoffmann, A. A. (2007). From parasite to mutualist: rapid evolution of Wolbachia in natural populations of Drosophila. PLoS Biol. 5:e114. doi: 10.1371/journal.pbio.0050114
Weinert, L. A., Araujo-Jnr, E. V., Ahmed, M. Z., and Welch, J. J. (2015). The incidence of bacterial endosymbionts in terrestrial arthropods. Proc. R. Soc. Lond. B Biol. Sci. 282, 20150249. doi: 10.1098/rspb.2015.0249
Werren, J. H. (1997). Biology of Wolbachia. Annu. Rev. Entomol. 42, 587–609. doi: 10.1146/annurev.ento.42.1.587
Werren, J. H., Baldo, L., and Clark, M. E. (2008). Wolbachia: master manipulators of invertebrate biology. Nat. Rev. Microbiol. 6, 741–751. doi: 10.1038/nrmicro1969
Werren, J. H., Zhang, W., and Guo, L. R. (1995). Evolution and phylogeny of Wolbachia: reproductive parasites of arthropods. Proc. R. Soc. Lond. B Biol. Sci. 261, 55–63. doi: 10.1098/rspb.1995.0117
White, I. M., and Elson-Harris, M. M. (1992). Fruit Flies of Economic Significance: Their Identification and Bionomics. Wallingford: CAB International.
Ye, Y. H., Seleznev, A., Flores, H. A., Woolfit, M., and McGraw, E. A. (2017). Gut microbiota in Drosophila melanogaster interacts with Wolbachia but does not contribute to Wolbachia-mediated antiviral protection. J. Invertebr. Pathol. 143, 18–25. doi: 10.1016/j.jip.2016.11.011
Ye, Y. H., Woolfit, M., Rances, E., O’Neill, S. L., and McGraw, E. A. (2013). Wolbachia-associated bacterial protection in the mosquito Aedes aegypti. PLoS Negl. Trop. Dis. 7:e2362. doi: 10.1371/journal.pntd.0002362
Yen, J. H., and Barr, A. R. (1971). New hypothesis of the cause of cytoplasmic incompatibility in Culex pipiens L. Nature 232, 657–658. doi: 10.1038/232657a0
Yong, H. S., Song, S. L., Chua, K. O., and Lim, P. E. (2017). Predominance of Wolbachia endosymbiont in the microbiota across life stages of Bactrocera latifrons (Insecta: Tephritidae). Meta Gene 14, 6–17.
Zabalou, S., Apostolaki, A., Livadaras, I., Franz, G., Robinson, A. S., Savakis, C., et al. (2009). Incompatible insect technique: incompatible males from a Ceratitis capitata genetic sexing strain. Entomol. Exp. Appl. 132, 232–240.
Zabalou, S., Charlat, S., Nirgianaki, A., Lachaise, D., Mercot, H., and Bourtzis, K. (2004a). Natural Wolbachia infections in the Drosophila yakuba species complex do not induce cytoplasmic incompatibility but fully rescue the wRi modification. Cell 167, 827–834. doi: 10.1534/genetics.103.015990
Zabalou, S., Riegler, M., Theodorakopoulou, M., Stauffer, C., Savakis, C., and Bourtzis, K. (2004b). Wolbachia-induced cytoplasmic incompatibility as a means for insect pest population control. Proc. Natl. Acad. Sci. U.S.A. 101, 15042–15045. doi: 10.1073/pnas.0403853101
Zchori-Fein, E., and Bourtzis, K. (eds) (2011). Manipulative Tenants: Bacteria Associated with Arthropods (A Volume in the Frontiers in Microbiology Series). Florida: CRC Press.
Zeh, J. A., and Zeh, D. W. (1996). The evolution of polyandry I: intragenomic conflict and genetic incompatibility. Proc. R. Soc. Lond. B Biol. Sci. 263, 1711–1717.
Zepeda-Cisneros, C. S., Meza Hernandez, J. S., Garcia-Martinez, V., Ibanez-Palacios, J., Zacharopoulou, A., and Franz, G. (2014). Development, genetic and cytogenetic analyses of genetic sexing strains of the Mexican fruit fly. Anastrepha ludens Loew (Diptera: Tephritidae). BMC Genet. 15(Suppl. 2):S1. doi: 10.1186/1471-2156-15-S2-S1
Zhou, W. G., Rousset, F., and O’Neill, S. (1998). Phylogeny and PCR-based classification of Wolbachia strains using wsp gene sequences. Proc. R. Soc. Lond. B Biol. Sci. 265, 509–515. doi: 10.1098/rspb.1998.0324
Zug, R., and Hammerstein, P. (2012). Still a host of hosts for Wolbachia: analysis of recent data suggests that 40% of terrestrial arthropod species are infected. PLoS One 7:e38544. doi: 10.1371/journal.pone.0038544
Zug, R., and Hammerstein, P. (2015). Bad guys turned nice? A critical assessment of Wolbachia mutualisms in arthropod hosts. Biol. Rev. Camb. Philos. Soc. 90, 89–111. doi: 10.1111/brv.12098
Keywords: insect control, symbiosis, endosymbiont, incompatible insect technique, cytoplasmic incompatibility
Citation: Mateos M, Martinez Montoya H, Lanzavecchia SB, Conte C, Guillén K, Morán-Aceves BM, Toledo J, Liedo P, Asimakis ED, Doudoumis V, Kyritsis GA, Papadopoulos NT, Augustinos AA, Segura DF and Tsiamis G (2020) Wolbachia pipientis Associated With Tephritid Fruit Fly Pests: From Basic Research to Applications. Front. Microbiol. 11:1080. doi: 10.3389/fmicb.2020.01080
Received: 18 December 2019; Accepted: 30 April 2020;
Published: 03 June 2020.
Edited by:
Dimitris G. Hatzinikolaou, National and Kapodistrian University of Athens, GreeceReviewed by:
Perran Ross, The University of Melbourne, AustraliaRiccardo Moretti, Italian National Agency for New Technologies, Energy and Sustainable Economic Development (ENEA), Italy
Copyright © 2020 Mateos, Martinez Montoya, Lanzavecchia, Conte, Guillén, Morán-Aceves, Toledo, Liedo, Asimakis, Doudoumis, Kyritsis, Papadopoulos, Augustinos, Segura and Tsiamis. This is an open-access article distributed under the terms of the Creative Commons Attribution License (CC BY). The use, distribution or reproduction in other forums is permitted, provided the original author(s) and the copyright owner(s) are credited and that the original publication in this journal is cited, in accordance with accepted academic practice. No use, distribution or reproduction is permitted which does not comply with these terms.
*Correspondence: Mariana Mateos, bW1hdGVvc0B0YW11LmVkdQ==
†ORCID: Jorge Toledo, orcid.org/0000-0001-9569-6079