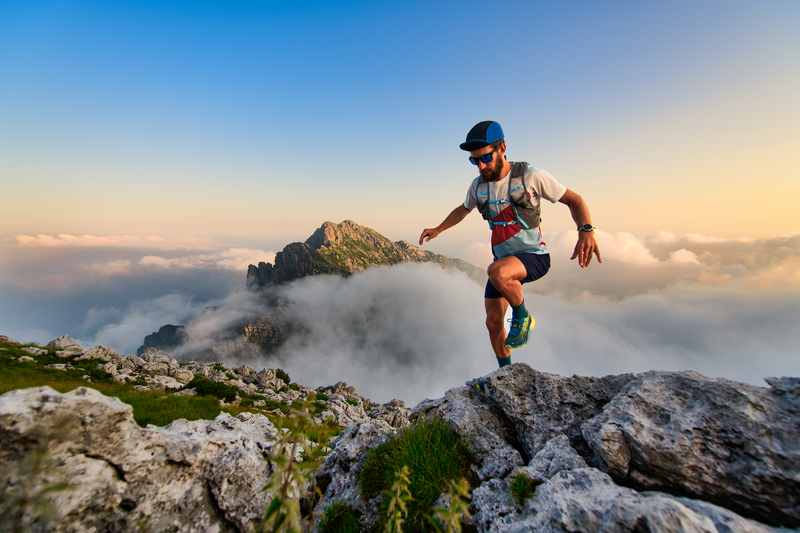
95% of researchers rate our articles as excellent or good
Learn more about the work of our research integrity team to safeguard the quality of each article we publish.
Find out more
REVIEW article
Front. Microbiol. , 20 May 2020
Sec. Antimicrobials, Resistance and Chemotherapy
Volume 11 - 2020 | https://doi.org/10.3389/fmicb.2020.01000
This article is part of the Research Topic Insights into New Strategies to Combat Biofilms View all 29 articles
Most biofilms in nature are formed by multiple microbial species, and such mixed-species biofilms represent the actual lifestyles of microbes, including bacteria, fungi, viruses (phages), and/or protozoa. Microorganisms cooperate and compete in mixed-species biofilms. Mixed-species biofilm formation and environmental resistance are major threats to water supply, food industry, and human health. The methods commonly used for microbial eradication, such as antibiotic or disinfectant treatments, are often ineffective for mixed-species biofilm consortia due to their physical matrix barrier and physiological interactions. For the last decade, an increasing number of investigations have been devoted to the usage of cold atmospheric plasma (CAP), which is produced by dielectric barrier discharges or plasma jets to prevent or eliminate microbial biofilms. Here, we summarized the production of CAP, the inactivation of microorganisms upon CAP treatment, and the microbial factors affecting the efficacy of CAP procedure. The applications of CAP as antibiotic alternative strategies for fighting mixed-species biofilms were also addressed.
A biofilm represents a syntrophic microbial community in which microbes adhere to each other and to a biotic or abiotic surface (Scholtz et al., 2015). Biofilms are usually formed by a variety of microorganisms, and mixed-species biofilms can cause persistent infections in medicine, drinking water pollution in water reservoirs, and food spoilage in the industry (Sun et al., 2018; Fan et al., 2020; Tan et al., 2020). Approximately 60–80% of microbial infections are associated with mixed-species biofilms (Tytgat et al., 2019). In some disease situations, multispecies biofilm infections lead to worse outcomes than monospecies infections (Trifilio et al., 2015; Jorge et al., 2018). For example, patients with cystic fibrosis present a more rapid decline of lung function when co-infected with Pseudomonas aeruginosa and Staphylococcus aureus than those who are infected by only one species (Limoli et al., 2016; Limoli and Hoffman, 2019). Therefore, controlling mixed-species biofilms has been one of the hot research areas in recent years.
Microbial cells within a mixed-species biofilm present increased resistance to environmental conditions, such as antibiotic treatment, oxidative stress, and nutrient starvation, in comparison with planktonic cells (Bridier et al., 2015). For the last decade, most investigations have been aimed at controlling biofilms by using different strategies (Ermolaeva et al., 2015), including bacteriophage therapy, biofilm matrix-active enzyme treatment, bacteriocin management, and using new antibiofilm compounds (such as essential oils, phenolic acids, curcumin, and polyphenolic components) (Simmons et al., 2018; Yuan et al., 2019). However, these techniques are not considered as perfect biofilm eradication strategies because of their potential to eventually contaminate the treated surface or lead to environmental pollution (Gupta and Ayan, 2019). Furthermore, many studies have warned that the complete elimination of mixed-species biofilms may not be achieved by using one treatment method only, and comprehensive measures are often required (Duske et al., 2015).
Plasma is one of the four fundamental states of matter in nature (Šimončicová et al., 2019). The “cocktail” constituents of plasma, including molecules and neutral atoms, charged particles, metastable radicals, and photons, play synergistic functions in microbial inactivation (Guo et al., 2018). In recent years, cold atmospheric plasma (CAP) has been extensively investigated for its potential as an alternative treatment in wound healing, dental cure, oncological therapy, and food decontamination (Scholtz et al., 2015; Jiao et al., 2019; Eggers et al., 2020). Significant progress has been made in controlling mixed-species biofilms with CAP, which is considered a rapid, environmentally friendly, energy saving, and versatile antimicrobial technology (Šimončicová et al., 2019). The present review focuses on the potential of CAP treatment to combat biofilms especially mixed-species biofilms, which are the dominant form of microorganisms existing in nature.
The high resistance and physiological change in microorganisms due to external treatments from hostile environments are nerve-wracking issues in biofilm control (Gupta and Ayan, 2019). Microbial cells in biofilms are embedded in extracellular polymeric substance (EPS), which makes up approximately 90% of the biofilm volume. Microorganisms cooperate and compete with each other to achieve the overall fitness in the consortia. EPS protects microbial cells from inactivation through host immunity and environment factors, such as antimicrobial agents, chemical disinfectants, and UV radiation (Rice et al., 2016).
The physiological events during the cell proliferation and the biofilm maturation of mixed-species communities are complex (Fan et al., 2020). One common interaction among microorganisms in mixed-species biofilms is the competition for nutrient acquisition and space occupation. Pang et al. (2019) showed that indigenous microbial cells in the runoff fluids of fresh salmon compete with Listeria monocytogenes for nutrients in mixed-species biofilms, resulting in a remarkable reduction in the number of L. monocytogenes cells compared with that in monospecies biofilms. Toxic substances, such as bacteriocins, hydrogen peroxide, organic acids, and enzymes, which are secreted by some microbial species provide competitive advantages over other species within mixed-species biofilms (Rendueles and Ghigo, 2012). The biofilm formation of Candida albicans can be inhibited when co-cultured with P. aeruginosa through the secretion of virulence factors (Bandara et al., 2010). Lactobacillus metabolites can kill L. monocytogenes in mixed-species biofilms by using hydrogen peroxide, lactic acid, and bacteriocin (Winkelstroter et al., 2015).
The cooperative interactions are widely existent when all members in the consortia benefit each other during biofilm formation (Ren et al., 2014; Liu et al., 2016). The cooperative microbial interactions in mixed-species biofilms may be based on the enhancement of the adhesion of the secreted matrix produced by partners or through metabolic cross-feeding by the products that promote the growth of other members. The dual-species biofilms formed by Lactococcus lactis and Pseudomonas fluorescens result in increased bacterial adhesion by up to 20,000- and 100-fold, respectively (Yuan et al., 2019). The authors proposed that the poor biofilm former L. lactis may benefit from the enhanced adhesion ability supported by the quick matrix-producing P. fluorescens. By contrast, some metabolites that originated from L. lactis may be utilized as nutrient sources by P. fluorescens. Liu et al. (2016) showed that the mixed-species biofilm formed by Escherichia coli, Salmonella enterica, and L. monocytogenes are facilitated by Ralstonia insidiosa, which provides a microenvironment for microbial accumulation and growth in nutrient-limited environments through its highly efficient nutrient utilization and cell proliferation. Given the complex microbial interactions, a mixed-species biofilm often achieves substantially more biomass than a monospecies biofilm without the need to input more nutrients (Ren et al., 2015).
The resistance of microbial biofilms is reinforced in a synergistic manner after the formation of mixed-species communities (Burmolle et al., 2014). EPS plays a critical role in biofilm resistance enhancement (Rice et al., 2016). The production of EPS, such as exopolysaccharide, is increased in biofilm cells compared with their planktonic counterparts. The constitution of EPS also varies markedly depending on the environmental elements and the bacterial species involved (Flemming and Wingender, 2010). The EPS of S. aureus possesses proteins, eDNA, and polysaccharides (Rice et al., 2016), whereas the biofilm matrix of P. aeruginosa can produce at least three polysaccharides, namely, alginate, Psl, and Pel (Flemming and Wingender, 2010). Studies have proven that EPS can act as cement to enhance initial adhesion and promote bacterial accumulation on a surface (Souza et al., 2020). Components of the biofilm matrix may also act as a reserve source of energy facilitating the nutrient accumulation in the microenvironment (Ermolaeva et al., 2015). Therefore, methods that destroy the EPS are effective for biofilm prevention. For instance, eDNA is widely present in biofilms and DNase treatment is currently considered effective in curing biofilm infections (Jiao et al., 2019). A study reported that a SigB(Q225P) mutation can enhance the S. aureus biofilm by downregulating the expression of the nuc gene, and the biofilm is evidently reduced after DNase I treatment compared with the untreated strain (Liu et al., 2018).
Besides the protection role of EPS, microbial interactions in mixed-species biofilms also may contribute to resistance enhancement. The organization of microorganisms within mixed-species biofilms is finely controlled for the fitness contribution of the whole consortium (Liu et al., 2016). Single-species biofilms lack commensal interactions between species, whereas mixed-species biofilms can form an intermixing structure (Yuan et al., 2019). The bacterial species may be present promiscuously throughout the community or as a layered structure, in which one species is in the bottom layers and the other one grows in the top layers. These spatial structures may be attributed to the survival rate of individual species within the mixed-species biofilms as a result of cooperative or competitive interactions (Nadell et al., 2016). In a dual-species biofilm infection, P. aeruginosa exhibits excellent colonizing capacity and often forms the basic biofilm structure, while K. pneumoniae usually takes shape as a tower-like structure at the biofilm top due to its higher growth rate (Chhibber et al., 2015). The interaction between P. aeruginosa and K. pneumoniae in the biofilms can enhance their resistance to the treatment of antimicrobial agents.
The primary mechanisms of enhanced resistance within mixed-species biofilms are not entirely clear due to the change in the composition of the biofilm matrix and the enhanced microbial interactions in the consortia. Several speculations regarding the enhanced resistance in mixed-species biofilms have been proposed. First, some species may protect others by their aggregation with other strains within the three-dimensional structure spatially arranged by certain species (Lee et al., 2014). Vibrio parahaemolyticus is often located on the top layers of multispecies biofilms because of its competitive advantage, and its minimum inhibitory concentration against antibiotics is remarkably decreased when co-cultured with L. monocytogenes (Chen et al., 2019). The second mechanism involves the matrix of a mixed-species biofilm. The chemical interactions between the EPS produced by distinct species may result in a thick matrix, which varies in accordance with the microbial species involved and the changes in environmental condition (Flemming and Wingender, 2010). The thickness of the matrix is suggested to play functions in mixed-species biofilm resistance by blocking the reach of the risk factors to the lower layers of active microbes (Guillonneau et al., 2018). EPS has also been experimentally proven to bind to antibiotics with positive charges, such as aminoglycosides, blocking their antimicrobial effects on microbes (Ermolaeva et al., 2015). In the mixed-species biofilms formed by Staphylococcus epidermidis and C. albicans, the EPS derived from S. epidermidis can protect C. albicans by arresting the penetration of antifungal drugs, such as fluconazole (Delben et al., 2016).
In addition, the temporary alterations in microbial neighbors contribute to enhanced mixed-species biofilm recalcitrance. A species settling down in a mixed-species biofilm can alter the physiology of neighboring species by interspecies interactions (Herschend et al., 2017), such as antibiotic resistance gene exchange, antibiotic-inactivated enzyme transfer, quorum-sensing signal-induced gene expression changes, and metabolite-mediated electron transport chain inhibition (Hansen et al., 2017; Orazi and O’Toole, 2019). For example, carbapenemase resistance gene-carried plasmid can be transferred from E. coli to either Acinetobacter baumannii or P. aeruginosa in mixed-species biofilms but is not observed in the planktonic state of these organisms (Tanner et al., 2017; Potron et al., 2011). The 2-heptyl-4-hydroxyquinolone N-oxide secreted by P. aeruginosa can alter the susceptibility of S. aureus strains within mixed-species biofilms to several antibacterial agents, such as vancomycin, aminoglycosides, and chloroxylenol (Orazi and O’Toole, 2019). The fungus C. albicans can induce vancomycin resistance of S. aureus during mixed-species biofilm formation (Harriott and Noverr, 2010). These possible mechanisms can result in a 100- to 1000-fold more resistance for mixed-species biofilms to antibiotics than their planktonic cells and therapeutic challenge of persistent biofilm infections in the host (Høiby et al., 2010). Therefore, interfering with the aforementioned mechanisms may be a promising strategy for the control of biofilms in nature and diseases.
Cold atmospheric plasma is an emerging antimicrobial strategy that could improve biofilm eradication (Julák et al., 2018; Gupta and Ayan, 2019). The word “plasma” was first used by Irving Langmuir in 1928 to describe quasineutral ionized or partially ionized gas generated by heating or through the application of a strong electromagnetic field (Šimončicová et al., 2019). Plasma is divided into thermal (at least 15,000 K) and non-thermal (less than 340 K) plasmas in accordance with the temperature of ionized gas. Non-thermal plasma, which is called CAP, is a partially ionized gas with a temperature generally close to room temperature (Gherardi et al., 2018). The systems of CAP can produce numerous species of active ingredients, such as positive and negative ions, neutral atoms (e.g., atomic oxygen and ozone), reactive molecules (e.g., superoxide and oxides of nitrogen), metastable radicals (e.g., OH and NO), and photons (e.g., UV). These components can work synergistically to kill microorganisms and also destroy the matrix of biofilms (Jiao et al., 2019).
The effectiveness of plasma may be dose-dependent, which depends on plasma source allocation, working gas supply, and biological target composition (Šimončicová et al., 2019). Currently, there is no single prototype that can be used for all applications. The most widely used plasma devices for biological applications are dielectric barrier discharges (DBDs) and atmospheric pressure plasma jets (APPJs) (Figure 1). The characteristics of DBD and APPJ are listed in Table 1. The defining features of DBDs and APPJs are the designs of dielectric material between the electrodes. DBD devices generally have electrode gaps ranging from tens of micrometers to a few centimeters and operate at frequencies of 50–500 kHz and voltage of tens of kilovolts. DBD devices, which are more suitable in the treatment of massive surfaces and large quantity of samples of varied shapes and sizes, are used in several fields, such as food industry (Šimončicová et al., 2019). In contrast with DBDs, APPJs are beneficial to use for the direct treatment of target objects (Gupta and Ayan, 2019). As such, the application of APPJ is advantageous in medicine, wherein APPJ may be used in localized decontamination, wound healing, and cancer therapy.
Figure 1. Schematic diagram of the typical configurations of CAP device. (A) The dielectric barrier discharge system. (B) The atmospheric plasma jet system.
Cold atmospheric plasma is a versatile strategy for microbial inactivation. The application of CAP for the prevention and eradication of mixed-species biofilms includes two aspects: the direct and indirect applications of CAP for biofilm destruction (Frías et al., 2020). The indirect way is done by first applying CAP on biotic or abiotic surfaces, which are then used to control biofilm formation, or by first activating water with CAP and treating the biofilm by using plasma-activated water (Patange et al., 2019; Zhou et al., 2019). The first investigation of bacterial inactivation by CAP was performed by imposing a voltage of 300 V to produce plasma by ionization of air (Krueger et al., 1957). In a further study, a high voltage (20 kV/cm) was imposed on four electrodes to treat Saccharomyces cerevisiae or Bacillus subtilis (formerly Bacillus natto), and cell destruction was observed (Mizuno and Hori, 1988). A set of plasma species can coordinate to inactivate microorganisms. Some species, such as molecules and neutral atoms, can break the bonds in the peptidoglycan structure of Gram-positive bacteria (Yusupov et al., 2012, 2013), whereas others may lead to the lipid peroxidation of the Gram-negative bacterial membrane (Joshi et al., 2011). After the disruption of the bacterial cell wall, reactive oxygen (ROS) or reactive nitrogen (RNS) species penetrates the bacteria to damage further the intracellular protein and genomic DNA (Mai-Prochnow et al., 2014).
Several common observations have been achieved in the bacterial inactivation with CAP. First, Gram-positive bacteria are less sensitive to CAP treatment than Gram-negative bacteria, which is probably due to the thick and tough peptidoglycan layers of Gram-positive bacteria (Mai-Prochnow et al., 2016; Nishime et al., 2017). Pompl et al. (2009) reported that the cell disruption of E. coli under low-temperature plasma treatment is more serious than that of S. aureus. The antimicrobial effect of CAP was also stronger in A. baumannii and Salmonella Typhimurium (Gram-negative strains) than in S. aureus (Gram-positive isolate) (Hafner et al., 2018; Huang et al., 2020). However, Gram-positive Enterococcus faecalis presented higher overall susceptibility to CAP treatment compared with P. aeruginosa, and the mechanism underlying E. faecalis’ high sensitivity to CAP processing is interesting and is worth further investigation. Second, vegetative bacterial cells are more susceptible to plasma sterilization than their spore counterparts (O’Connor et al., 2014). Lastly, the complete eradication of bacterial biofilm requires a much longer treatment duration than killing its planktonic counterparts (Mai-Prochnow et al., 2014).
The mechanisms participating in CAP inactivation are considered complicated due to the numerous species in plasma. CAP may have numerous targets in bacterial cells, including cell wall, cell membrane, genomic DNA, and intracellular proteins (Vatansever et al., 2013; Mai-Prochnow et al., 2014). Several plasma–cell interactions have been involved in the bactericidal effect of CAP. One of these interactions is the mechanical etching role performed by the atomic and molecular radicals of CAP, resulting in pore formation and cell erosion on the polymeric surface of microorganisms (Handorf et al., 2018; Huang et al., 2020). Another interaction is the photodesorption implemented by UV radiation, which results in the subsequent chemical bond breakage of bacterial organic macromolecules (Van Der Paal et al., 2016; Brun et al., 2018). The third interaction is the DNA damage triggered by reactive species (Delben et al., 2016; Sakudo et al., 2018), and the last interaction is the oxidative damage of bacterial proteins, polysaccharides, and lipids caused by ROS and RNS (Edengeiser et al., 2015; Ji et al., 2018). As a result, Gram-negative bacteria, such as P. aeruginosa, often exhibit visible morphological changes in cell shape, whereas minor or inconspicuous morphological deformities are observed in Gram-positive bacteria, such as S. aureus and E. faecalis, after the CAP procedure (Nishime et al., 2017; Yang et al., 2020).
The efficacy of CAP treatment against biofilms formed by certain microorganisms varies depending on microbial type, growth phase, produced matrix, biofilm thickness, and process factors (Šimončicová et al., 2019). The bacterial envelope structure plays important roles in microbial defense for CAP processing (Mai-Prochnow et al., 2016). Gram-positive bacteria have a relatively thick cell wall (20–80 nm) composed of peptidoglycan, whereas Gram-negative bacteria have a thin cell wall (3–5 nm) but possess an additional outer membrane embedded by lipopolysaccharide and several pores. These differences in the microbial envelope can confer the different sensitivities of bacterial cells to CAP treatment. Mai-Prochnow et al. (2016) revealed that the bacterial cell wall thickness is correlated with the log10 colony forming unit (CFU) reduction after different durations (1, 3, or 10 min) of CAP treatment. Moreover, P. aeruginosa exhibits more resistance to CAP when co-cultured with S. epidermidis as dual-species biofilms than that as a monospecies biofilm. Modic et al. (2017) reported the biphasic bacterial effect of ROS-dominated CAP, generated ROS-dominated gas-phase regimen with a low power discharge (8 W), and revealed that mixed-species biofilms containing P. aeruginosa, S. aureus, E. faecalis, and K. pneumoniae present a biphasic bacterial killing under ROS exposure. In the four-species biofilms, P. aeruginosa was much less susceptible to ROS-dominated plasma and presented a more gradual reduction in viable count across the 240 s of exposure (less than 3 log10 CFU), whereas the three other species were more susceptible to ROS plasma with more than 3 log reduction in viable cell count, showing a quicker reduction between 60 and 120 s of plasma treatment.
Formation of the viable but non-culturable (VBNC) state of microorganisms also contributes to CAP resistance. Alessandria et al. (2019) showed the presence of inactivated bacterial cells or their VBNC state after CAP treatment through quantitative polymerase chain reaction amplification targeting the 16S rRNA. Liao et al. (2020) reported that a level of 7.4 to 7.6 log10 CFU/ml of S. aureus VBNC cells can be induced after 8.1 to 24.3 kJ CAP treatment. The authors also found that most energy-dependent physiological activities are arrested, while the oxidative stress response-related pathways are obviously upregulated in S. aureus VBNC state. In addition, genome comparison between control cells and survivors in transposon-mutated S. aureus biofilms has shown that the genes involved in the synthesis of the pigment staphyloxanthin are associated with resistance to CAP treatment (Mai-Prochnow et al., 2015). A recent study showed that CAP-processed S. aureus cells present decreased pigment phenotype, and the deletion of the staphyloxanthin biosynthetic genes crtM and crtN confers Newman-ΔcrtM and Newman-ΔcrtN, but not Newman-ΔcrtO, with increased sensitivity to CAP treatment (Yang et al., 2020). These data suggest that the yellow intermediates of S. aureus pigment’s biosynthetic pathway are important factors for the resistance of S. aureus against CAP inactivation. The reason may be the antioxidant property of the golden carotenoid pigment for deactivating ROS and helping bacteria evade ROS killing (Chen et al., 2016). Other antioxidant molecules produced by microorganisms, such as pyocyanin of P. aeruginosa, may also protect bacteria from CAP treatment. The anti-CAP roles of antioxidant molecules need further investigation.
The pioneering work regarding the possible inactivation of mixed-species biofilms through CAP has been conducted by Denes et al. (2001), who showed that the CAP deposition on mixed-species biofilms formed by S. epidermidis, P. fluorescens, and S. Typhimurium results in the decrease of 56.5% bacterial attachment and 72.2% biofilm formation. The authors concluded that the CAP deposition of polyethylene glycol-like structures may be useful for food processing and medical practice to reduce bacterial contamination. Mai-Prochnow et al. (2014) reviewed the advances in the decontamination of biofilm-associated bacteria by using CAP. Scholtz et al. (2015) recently reviewed the use of CAP as a tool for decontamination and disinfection, such as in microorganism inactivation, pollutant removal, surface modification, food preparation, biofilm degradation, wound therapy, nosocomial infection prevention, cancer treatment, prion inactivation, and apoptosis initiation. Gupta and Ayan (2019) presented an overview of plasma instrumentation and summarized the studies of plasma on biofilms by mostly using monospecies biofilms.
Mixed-species biofilms represent the primary environment of most pathogens in the clinical setting. The normal ecological environment of microbial consortia that often coexist with its dwelling host may be easily disrupted by invading pathogens, which can outcompete healthy normal flora during their growth and transform the commensal consortia into pathogenic biofilms (Tytgat et al., 2019). Many pathogens often colonize joint replacements, heart valves, and metal or plastic implants to form mixed-species biofilms, which cause life-threatening diseases (Julák et al., 2018). Gomila et al. (2019) conducted a multicenter retrospective cohort study on patients with complicated urinary tract infections from 20 hospitals in eight countries and found that 42.2% (341/807) of the patients have catheter-associated urinary tract infections. Another study conducted in Singapore reported that about 14.7% (69/470) of the patients who underwent peritoneal dialysis have been subjected to catheter removal due to infection (Kwan et al., 2019). Indwelling medical device-related and catheter-associated infections can be caused by mixed-species biofilms, and many pathogens involved in biofilm formation can be cultured (Figure 2). Mixed-species biofilm infections have raised great concerns and are difficult to cure (Azeredo et al., 2017).
Figure 2. Peritoneal dialysis catheter-associated infections caused by mixed-species biofilm. (A) The peritoneal dialysis catheter was filled with thick pus. (B) Several bacterial species derived from catheter-infection pus were grown on sheep blood agar after being cultured at 37°C for 16 h postinoculation.
As a novel antimicrobial strategy, applications of CAP in fighting mixed-species biofilms have been investigated (Table 2). In an early work, Koban et al. (2011) demonstrated the role of CAP in killing multispecies human saliva biofilms grown on titanium disks in vitro and found a 5.67 reduction in log10 CFU after 10 min of DBD argon plasma treatment. The authors concluded that the treatment of mixed-species dental biofilms on titanium disks by using CAP resulted in a reduction of 1.50 log10 CFU for saliva biofilm and that CAP is more efficient than chlorhexidine digluconate rinse. Koban’s group also evaluated the synergistic effects of CAP and disinfectants against multispecies dental biofilms and reported that the combination of disinfecting agent NaOCl or hydrogen peroxide with CAP achieves a higher level of CFU reduction than each agent alone (Koban et al., 2013). Du et al. (2013) confirmed the synergistic effect of CAP with 2% chlorhexidine digluconate on mixed-species biofilms by incubating polyspecies bacteria from human dental root canal infections on sterile bovine dentin disks. Delben et al. (2016) reported the role of CAP on pathogenic oral biofilms constituted by C. albicans and S. aureus. In their study, the dual-species biofilms presented a considerable log10 CFU/ml reduction (1.52 for C. albicans and 1.23 for S. aureus) after 60 s of CAP treatment. Colony counting, confocal laser scanning microscopy, and scanning electron microscopy revealed reduced viability and the alteration of the morphology of the microorganism/biofilm in plasma group in comparison with those in the negative control. Importantly, low cytotoxicity and high viability in the oral epithelium were observed after treatment with CAP. Further study showed no sign of necrosis in the plasma-treated epithelium, and cell proliferation was well maintained, indicating that CAP is a safe approach to eliminate pathogenic oral biofilms (Delben et al., 2016).
Cold atmospheric plasma has also been proven to be a promising strategy for the control of multispecies biofilms formed by foodborne pathogens (Govaert et al., 2020; Huang et al., 2020). The cultures of S. Typhimurium mixed with cultivable indigenous microorganisms exhibited greater resistance to cold oxygen plasma (COP) compared with monoculture biofilms on fresh iceberg lettuce (Jahid et al., 2015). However, a reduction in log10 CFU/cm2 by approximately 4.6 was achieved in mixed cultures after COP treatment, and a notable shift in the colony type of S. Typhimurium from smooth to rugose was observed for biofilms on stainless-steel coupons even after 10 s of processing procedure with COP. By contrast, 5 min of COP treatment did not achieve any morphological shift in S. Typhimurium mixed biofilms on lettuce coupons. This difference may be ascribed to the penetration of COP to biotic and abiotic surfaces because COP does not penetrate the lettuce. Patange et al. (2019) showed that the bacterial biofilm populations of L. monocytogenes and P. fluorescens on abiotic surfaces treated with autologous conditioned plasma of high voltage (80 kV) has yielded non-detectable levels after 120 s of treatment. The inoculation on lettuce required an extended time to complete the eradication of mixed-species biofilms, and L. monocytogenes and P. fluorescens reduced by 2.2 and 4.2 log10 CFU/ml, respectively, after 120 s of contained treatment. Govaert et al. (2020) reported the air-based CAP treatment of dual-species biofilms formed by L. monocytogenes and S. Typhimurium, and a result of log10 CFU/cm2-reductions ranging between 1.5 and 2.5 was observed. The inactivation efficacy of CAP treatment may depend on the biofilm population type, but not the working gas supplied (Table 2).
Mixed-species biofilms also grow and thrive in drinking water systems and usually result in human health concerns. Sun et al. (2018) treated the simulated polymicrobial drinking water biofilms with a 9 × 9 array of plasma jet, and results showed that all biofilms tested vanished in 20 min of CAP exposure. Confocal laser scanning microscopy revealed that the number of active cells in mixed-species biofilms was reduced by more than 93% after 15 min of CAP treatment. Zhou et al. (2019) emphasized the importance of biochemically reactive species from plasma in the eradication of multispecies water biofilms and created a microplasma-bubble reactor to generate underwater microplasma bubbles that served as transport vehicles for delivering plasma reactive species to the water biofilm sites. The underwater microplasma bubbles generated in an artificial fish tank could enhance the mass transfer of ROS and RNS (mostly nitric oxide) into water for applications, such as initiating biofilm dispersal and treating bacterial biofilms on the skin of infected fish.
The microplasma bubbles are considered to be effective for underwater biofilm eradication. When reaching the close vicinity of a water biofilm, the self-burst of microbubbles may produce pressure waves that can break up the EPS matrix of the biofilms. Such pressure waves can further induce the bursting of other yet-to-burst microbubbles like a chain reaction to keep on disrupting the biofilm matrix until the whole biofilm structure is collapsed (Zhou et al., 2019). While the biofilm is dispersing, the resident microbial cells are exposed to bactericidal plasma-activated water carrying ROS and RNS. However, the fundamental features of plasma-liquid physics and chemistry are largely unknown and are worth further investigation.
Most advantages of CAP process on multispecies biofilms have been accompanied by numerous limitations. The first obvious limitation is the weak penetrating capacity of plasma species into the deeper layers of mixed-species biofilms (Jiao et al., 2019). The increased production of EPS in biofilms may impede CAP penetration and prevent the antimicrobial effect of CAP on microbial cells at the bottom of the biofilms. The resistance of microbial cells and mixed-species biofilms to CAP varies depending on the microbial species involved, the changes in environmental condition, and the composition of active species generated by CAP systems (Jiao et al., 2019). The second limitation involves the nature and quantity of mixed-species biofilms to be sterilized. CAP sterilization in the food industry, for example, the treatment of bulky and irregularly shaped food, limited volume of the investigated material should be considered (Scholtz et al., 2015). The rough biofilm surfaces often protect microbial cells from CAP exposure, and result in CAP treatment failure (Šimončicová et al., 2019). The third limitation involves the formation of VBNC microbial state. CAP treatment has been proven to induce the VBNC state of microbial cells, although the mechanisms underlying VBNC formation are obscure (Alessandria et al., 2019; Liao et al., 2020). The fourth limitation involves the servicing costs. Although the opening expenses and maintenance costs have been considerably decreased in CAP generated in ambient air, a CAP system that operates at low pressure usually requires high investment costs (Šimončicová et al., 2019). Possible solutions should be investigated to counter these limitations before the translation of CAP into real-life applications.
Naturally existing biofilms are composed of varied microbial species. The biological characteristics of microbes were explored as part of mixed-species biofilms rather than in strain isolation. The resident microorganisms in a mixed-species biofilm cooperate and/or compete to access available spaces and nutrients. The current information on microbial interactions among mixed-species biofilms are mainly derived from cultural investigations of polymicrobial biofilms in vitro, and information on actual interactions in mixed-species biofilms formed in vivo are scarce. Most common diseases, such as indwelling medical device-related and catheter-associated infections, are caused by mixed-species biofilms rather than by isolated pathogens.
Mixed-species biofilms display greater resistance to external stressors, including antibiotics and disinfectants. The mechanisms underlying mixed-species biofilm’s elevated resistance to environmental factors are still unclear. The increased EPS production and enhanced microbial interaction in multispecies communities contribute to this resistance. In recent years, much effort has been devoted to mixed-species biofilm control. The most striking strategy used for mixed-species biofilm eradication and prevention is CAP, which can produce many species of active ingredients to implement different microbicidal mechanisms. Accumulated studies have confirmed that CAP can deconstruct the mixed-species biofilm matrix and also kill microbial cells. CAP can be developed as an effective tool against mixed-species biofilms in the water supply system, food industry, and medical practice. However, the safety assessment for CAP applications is extremely limited, important, and worthy of study in the future.
YR, RZ, and XR contributed to the conception and design of the review. YR wrote the first draft of the manuscript. XR designed the figures. WS and YY contributed to various sections of the manuscript, including figures. All authors contributed to manuscript revision, editing, and approved the submitted version.
This work was supported by the National Key Biosafety Technology Research and Development Program of China (2017YFC1200404-4), the Biosafety Research Program of PLA (17SAZ08), and the National Natural Science Foundation of China (81672071).
The authors declare that the research was conducted in the absence of any commercial or financial relationships that could be construed as a potential conflict of interest.
Alessandria, V., Rantsiou, K., Cavallero, M. C., and Cocolin, L. S. (2019). Effect of atmospheric pressure plasma on Listeria monocytogenes attached to abiotic surfaces. J. Food Protec. 82, 233–237. doi: 10.4315/0362-028X.JFP-18-228
Azeredo, J., Azevedo, N. F., Briandet, B., Cerca, N., Coenye, T., Costa, A. R., et al. (2017). Critical review on biofilm methods. Crit. Rev. Microbiol. 43, 313–351. doi: 10.1080/1040841X.2016.1208146
Bandara, H. M., Yau, J. Y., Watt, R. M., Jin, L. J., and Samaranayake, L. P. (2010). Pseudomonas aeruginosa inhibits in-vitro Candida biofilm development. BMC Microbiol. 10:125. doi: 10.1186/1471-2180-10-125
Bridier, A., Sanchez-Vizuete, P., Guilbaud, M., Piard, J., and Naïtali, M. (2015). Biofilm-associated persistence of food-borne pathogens. Food Microbiol. 45, 167–178. doi: 10.1016/j.fm.2014.04.015
Brun, P., Bernabè, G., Marchiori, C., Scarpa, M., Zuin, M., Cavazzana, R., et al. (2018). Antibacterial efficacy and mechanisms of action of low power atmospheric pressure cold plasma: membrane permeability, biofilm penetration and antimicrobial sensitization. J. Appl. Microbiol. 125, 398–408. doi: 10.1111/jam.13780
Burmolle, M., Ren, D., Bjarnsholt, T., and Sørensen, S. J. (2014). Interactions in multispecies biofilms: do they actually matter? Trends Microbiol. 22, 84–91. doi: 10.1016/j.tim.2013.12.004
Chen, F., Di, H., Wang, Y., Cao, Q., Xu, B., Zhang, X., et al. (2016). Small-molecule targeting of a diapophytoene desaturase inhibits S. aureus virulence. Nat. Chem. Biol. 12, 174–179. doi: 10.1038/nchembio.2003
Chen, P., Wang, J. J., Hong, B., Tan, L., Yan, J., Zhang, Z., et al. (2019). Characterization of mixed-species biofilm formed by Vibrio parahaemolyticus and Listeria monocytogenes. Front. Microbiol. 10:2543. doi: 10.3389/fmicb.2019.02543
Chhibber, S., Bansal, S., and Kaur, S. (2015). Disrupting the mixed-species biofilm of Klebsiella pneumoniae B5055 and Pseudomonas aeruginosa PAO using bacteriophages alone or in combination with xylitol. Microbiology 161, 1369–1377. doi: 10.1099/mic.0.000104
Delben, J. A., Zago, C. E., Tyhovych, N., Duarte, S., and Vergani, C. E. (2016). Effect of atmospheric-pressure cold plasma on pathogenic oral bBiofilms and in vitro reconstituted oral epithelium. PLoS One 11:e0155427. doi: 10.1371/journal.pone.0155427
Denes, A., Somers, E., Wong, A., and Denes, F. (2001). 12-Crown-4-ether and tri(ethylene glycol) dimethyl-ether plasma-coated stainless steel surfaces and their ability to reduce bacterial biofilm deposition. J. Appl. Polym. Sci. 81, 3425–3438. doi: 10.1002/app.1799
Du, T., Shi, Q., Shen, Y., Cao, Y., Ma, J., Lu, X., et al. (2013). Effect of modified nonequilibrium plasma with chlorhexidine digluconate against endodontic biofilms in vitro. J. Endod. 39, 1438–1443. doi: 10.1016/j.joen.2013.06.027
Duske, K., Jablonowski, L., Koban, I., Matthes, R., Holtfreter, B., Sckell, A., et al. (2015). Cold atmospheric plasma in combination with mechanical treatment improves osteoblast growth on biofilm covered titanium discs. Biomaterials 52, 327–334. doi: 10.1016/j.biomaterials.2015.02.035
Edengeiser, E., Lackmann, J., Bründermann, E., Schneider, S., Benedikt, J., Bandow, J. E., et al. (2015). Synergistic effects of atmospheric pressure plasma-emitted components on DNA oligomers: a Raman spectroscopic study. J. Biophotonics 8, 918–924. doi: 10.1002/jbio.201400123
Eggers, B., Marciniak, J., Memmert, S., Kramer, F. J., Deschner, J., and Nokhbehsaim, M. (2020). The beneficial effect of cold atmospheric plasma on parameters of molecules and cell function involved in wound healing in human osteoblast-like cells in vitro. Odontology 6:487. doi: 10.1007/s10266-020-00487-y
Ermolaeva, S. A., Sysolyatina, E. V., and Gintsburg, A. L. (2015). Atmospheric pressure nonthermal plasmas for bacterial biofilm prevention and eradication. Biointerphases 10:29404. doi: 10.1116/1.4914382
Fan, Y., Huang, X., Chen, J., and Han, B. (2020). Formation of a mixed-species biofilm is a survival strategy for unculturable lactic acid bacteria and Saccharomyces cerevisiae in Daqu, a Chinese traditional fermentation starter. Front. Microbiol. 11:138. doi: 10.3389/fmicb.2020.00138
Flemming, H. C., and Wingender, J. (2010). The biofilm matrix. Nat. Rev. Microbiol. 8, 623–633. doi: 10.1038/nrmicro2415
Frías, E., Iglesias, Y., Alvarez-Ordóñez, A., Prieto, M., González-Raurich, M., and López, M. (2020). Evaluation of cold atmospheric pressure plasma (CAPP) and plasma-activated water (PAW) as alternative non-thermal decontamination technologies for tofu: impact on microbiological, sensorial and functional quality attributes. Food Res. Int. 129:108859. doi: 10.1016/j.foodres.2019.108859
Fridman, G., Friedman, G., Gutsol, A., Shekhter, A. B., Vasilets, V. N., and Fridman, A. (2008). Applied plasma medicine. Plasma Process Polym. 5, 503–533. doi: 10.1002/ppap.200700154
Gherardi, M., Tonini, R., and Colombo, V. (2018). Plasma in dentistry: brief history and current status. Trends Biotechnol. 36, 583–585. doi: 10.1016/j.tibtech.2017.06.009
Gomila, A., Carratalà, J., Eliakim-Raz, N., Shaw, E., Tebé, C., Wolkewitz, M., et al. (2019). Clinical outcomes of hospitalised patients with catheter-associated urinary tract infection in countries with a high rate of multidrug-resistance: the COMBACTE-MAGNET RESCUING study. Antimicrob. Resist. Infect. Control. 8:198. doi: 10.1186/s13756-019-0656-6
Govaert, M., Smet, C., Graeffe, A., Walsh, J. L., and Van Impe, J. F. M. (2020). Inactivation of L. monocytogenes and S. typhimurium biofilms by means of an air-based cold atmospheric plasma (CAP) system. Foods 9:e157. doi: 10.3390/foods9020157
Govaert, M., Smet, C., Walsh, J. L., and Van Impe, J. F. M. (2019). Dual-species model biofilm consisting of Listeria monocytogenes and Salmonella Typhimurium: development and inactivation with cold atmospheric plasma (CAP). Front. Microbiol. 10:2524. doi: 10.3389/fmicb.2019.02524
Guillonneau, R., Baraquet, C., Bazire, A., and Molmeret, M. (2018). Multispecies biofilm development of marine bacteria implies complex relationships through competition and synergy and modification of matrix components. Front. Microbiol. 9:1960. doi: 10.3389/fmicb.2018.01960
Guo, L., Xu, R., Zhao, Y., Liu, D., Liu, Z., Wang, X., et al. (2018). Gas plasma pre-treatment increases antibiotic sensitivity and persister eradication in methicillin-resistant Staphylococcus aureus. Front. Microbiol. 9:537. doi: 10.3389/fmicb.2018.00537
Gupta, T. T., and Ayan, H. (2019). Application of non-thermal plasma on biofilm: a review. Appl. Sci. 9:3548. doi: 10.3390/app9173548
Hafner, S., Ehrenfeld, M., Neumann, A. C., and Wieser, A. (2018). Comparison of the bactericidal effect of cold atmospheric pressure plasma (CAPP), antimicrobial photodynamic therapy (aPDT), and polihexanide (PHX) in a novel wet surface model to mimic oral cavity application. J. CranioMaxilloFac. Surg. 46, 2197–2202. doi: 10.1016/j.jcms.2018.09.006
Handorf, O., Weihe, T., Bekeschus, S., Graf, A. C., Schnabel, U., Riedel, K., et al. (2018). Nonthermal plasma jet treatment negatively affects viability and structure of Candida albicans SC5314 biofilms. Appl. Environ. Microbiol. 84:e01163-18. doi: 10.1128/AEM.01163-18
Hansen, L. B. S., Ren, D., Burmølle, M., and Sørensen, S. J. (2017). Distinct gene expression profile of Xanthomonas retroflexus engaged in synergistic multispecies biofilm formation. ISME J. 11, 300–303. doi: 10.1038/ismej.2016.107
Harriott, M. M., and Noverr, M. C. (2010). Ability of Candida albicans mutants to induce Staphylococcus aureus vancomycin resistance during polymicrobial biofilm formation. Antimicrob. Agents Chemother. 54, 3746–3755. doi: 10.1128/AAC.00573-10
Herschend, J., Damholt, Z. B. V., Marquard, A. M., Svensson, B., and Sørensen, S. J. (2017). A meta-proteomics approach to study the interspecies interactions affecting microbial biofilm development in a model community. Sci. Rep. 7:16483. doi: 10.1038/s41598-017-16633-6
Høiby, N., Bjarnsholt, T., Givskov, M., Molin, S., and Ciofu, O. (2010). Antibiotic resistance of bacterial biofilms. Int. J. Antimicrob. Agents 35, 322–332. doi: 10.1016/j.ijantimicag.2009.12.011
Huang, M., Zhuang, H., Zhao, J., Wang, J., Yan, W., and Zhang, J. (2020). Differences in cellular damage induced by dielectric barrier discharge plasma between Salmonella Typhimurium and Staphylococcus aureus. Bioelectrochemistry 132:107445. doi: 10.1016/j.bioelechem.2019.107445
Jahid, I. K., Han, N., Zhang, C. Y., and Ha, S. D. (2015). Mixed culture biofilms of Salmonella Typhimurium and cultivable indigenous microorganisms on lettuce show enhanced resistance of their sessile cells to cold oxygen plasma. Food Microbiol. 46, 383–394. doi: 10.1016/j.fm.2014.08.003
Ji, S. H., Ki, S. H., Ahn, J. H., Shin, J. H., Hong, E. J., Kim, Y. J., et al. (2018). Inactivation of Escherichia coli and Staphylococcus aureus on contaminated perilla leaves by dielectric barrier discharge (DBD) plasma treatment. Arch. Biochem. Biophys. 643, 32–41. doi: 10.1016/j.abb.2018.02.010
Jiao, Y., Tay, F. R., Niu, L., and Chen, J. (2019). Advancing antimicrobial strategies for managing oral biofilm infections. Int. J. Oral Sci. 11:28. doi: 10.1038/s41368-019-0062-1
Jorge, L. S., Fucuta, P. S., Oliveira, M. G. L., Nakazone, M. A., de Matos, J. A., Chueire, A. G., et al. (2018). Outcomes and risk factors for polymicrobial posttraumatic osteomyelitis. J. Bone Jt. Infect. 3, 20–26. doi: 10.7150/jbji.22566
Joshi, S. G., Cooper, M., Yost, A., Paff, M., Ercan, U. K., Fridman, G., et al. (2011). Nonthermal dielectric-barrier discharge plasma-induced inactivation involves oxidative DNA damage and membrane lipid peroxidation in Escherichia coli. Antimicrob. Agents Chemother. 55, 1053–1062. doi: 10.1128/AAC.01002-10
Julák, J., Scholtz, V., and Vaňková, E. (2018). Medically important biofilms and non-thermal plasma. World J. Microbiol. Biotechnol. 34:178. doi: 10.1007/s11274-018-2560-2
Koban, I., Geisel, M. H., Holtfreter, B., Jablonowski, L., Hübner, N. O., Matthes, R., et al. (2013). Synergistic effects of nonthermal plasma and disinfecting agents against dental biofilms in vitro. ISRN Dent. 2013:573262. doi: 10.1155/2013/573262
Koban, I., Holtfreter, B., Hubner, N. O., Matthes, R., Sietmann, R., Kindel, E., et al. (2011). Antimicrobial efficacy of non-thermal plasma in comparison to chlorhexidine against dental biofilms on titanium discs in vitro – proof of principle experiment. J. Clin. Periodontol. 38, 956–965. doi: 10.1111/j.1600-051X.2011.01740.x
Krueger, A. P., Smith, R. F., and Go, I. G. (1957). The action of air ions on bacteria. I. Protective and lethal effect on suspensions of staphylococci in droplets. J. Gen. Physiol. 41, 359–381. doi: 10.1085/jgp.41.2.359
Kwan, J. R., Chong, T. T., Low, G. Z., Low, G. W., Htay, H., Foo, M. W., et al. (2019). Outcomes following peritoneal dialysis catheter removal with reinsertion or permanent transfer to haemodialysis. J. Vasc. Access. 20, 60–64. doi: 10.1177/1129729818773984
Laroussi, M., and Akan, T. (2007). Arc-free atmospheric pressure cold plasma jets: a review. Plasma Process Polym. 4, 777–788. doi: 10.1002/ppap.20070006
Lee, K. W. K., Periasamy, S., Mukherjee, M., Xie, C., Kjelleberg, S., and Rice, S. A. (2014). Biofilm development and enhanced stress resistance of a model, mixed-species community biofilm. ISME J. 8, 894–907. doi: 10.1038/ismej.2013.194
Liao, X., Liu, D., and Ding, T. (2020). Nonthermal plasma induces the viable-but-nonculturable state in Staphylococcus aureus via metabolic suppression and the oxidative stress response. Appl. Environ. Microbiol. 86, e2216–e2219. doi: 10.1128/AEM.02216-19
Limoli, D. H., and Hoffman, L. R. (2019). Help, hinder, hide and harm: what can we learn from the interactions between Pseudomonas aeruginosa and Staphylococcus aureus during respiratory infections? Thorax 74, 684–692. doi: 10.1136/thoraxjnl-2018-212616
Limoli, D. H., Yang, J., Khansaheb, M. K., Helfman, B., Peng, L., Stecenko, A. A., et al. (2016). Staphylococcus aureus and Pseudomonas aeruginosa co-infection is associated with cystic fibrosis-related diabetes and poor clinical outcomes. Eur. J. Clin. Microbiol. Infect. Dis. 35, 947–953. doi: 10.1007/s10096-016-2621-0
Liu, H., Shang, W., Hu, Z., Zheng, Y., Yuan, J., Hu, Q., et al. (2018). A novel SigB(Q225P) mutation in Staphylococcus aureus retains virulence but promotes biofilm formation. Emerg. Microbes Infect. 7:72. doi: 10.1038/s41426-018-0078-1
Liu, W., Røder, H. L., Madsen, J. S., Bjarnsholt, T., Sørensen, S. J., and Burmølle, M. (2016). Interspecific bacterial interactions are reflected in multispecies biofilm spatial organization. Front. Microbiol. 7:1366. doi: 10.3389/fmicb.2016.01366
Liu, N. T., Bauchan, G. R., Francoeur, C. B., Shelton, D. R., Lo, Y. M., and Nou, X. (2016). Ralstonia insidiosa serves as bridges in biofilm formation by foodborne pathogens Listeria monocytogenes, Salmonella enterica, and Enterohemorrhagic Escherichia coli. Food Control 65, 14–20. doi: 10.1016/j.foodcont.2016.01.004
Mai-Prochnow, A., Bradbury, M., Ostrikov, K., and Murphy, A. B. (2015). Pseudomonas aeruginosa bioilm response and resistance to cold atmospheric pressure plasma is linked to the redox-active molecule phenazine. PLoS One 10:e0130373. doi: 10.1371/journal.pone.0130373
Mai-Prochnow, A., Clauson, M., Hong, J., and Murphy, A. B. (2016). Gram positive and Gram negative bacteria differ in their sensitivity to cold plasma. Sci. Rep. 6:38610. doi: 10.1038/srep38610
Mai-Prochnow, A., Murphy, A. B., McLean, K. M., Kong, M. G., and Ostrikov, K. (2014). Atmospheric pressure plasmas: infection control and bacterial responses. Int. J. Antimicrob. Agents 43, 508–517. doi: 10.1016/j.ijantimicag.2014.01.025
Mizuno, A., and Hori, Y. (1988). Destruction of living cells by pulsed high voltage application. IEEE Trans. Ind. Appl. 24, 387–394. doi: 10.1109/28.2886
Modic, M., McLeod, N. P., Sutton, J. M., and Walsh, J. L. (2017). Cold atmospheric pressure plasma elimination of clinically important single- and mixed-species biofilms. Int. J. Antimicrob. Agents. 49, 375–378. doi: 10.1016/j.ijantimicag.2016.11.022
Nadell, C. D., Drescher, K., and Foster, K. R. (2016). Spatial structure, cooperation and competition in biofilms. Nat. Rev. Microbiol. 14, 589–600. doi: 10.1038/nrmicro.2016.84
Nishime, T. M. C. C., Borges, A. C., Koga-ito, C. Y., Machida, M., Hein, L. R. O. O., and Kostov, K. G. (2017). Non-thermal atmospheric pressure plasma jet applied to inactivation of different microorganisms. Surf. Coat. Technol. 312, 19–24. doi: 10.1016/j.surfcoat.2016.07.076
O’Connor, N., Cahill, O., Daniels, S., Galvin, S., and Humphreys, H. (2014). Cold atmospheric pressure plasma and decontamination. Can it contribute to preventing hospital-acquired infections? J. Hosp. Infect. 88, 59–65. doi: 10.1016/j.jhin.2014.06.015
Orazi, G., and O’Toole, G. A. (2019). It takes a village: mechanisms underlying antimicrobial recalcitrance of polymicrobial biofilms. J. Bacteriol. 202:e0530-19. doi: 10.1128/JB.00530-19
Pang, X., Wong, C., Chung, H. J., and Yuk, H. G. (2019). Biofilm formation of Listeria monocytogenes and its resistance to quaternary ammonium compounds in a simulated salmon processing environment. Food Control 98, 200–208. doi: 10.1016/j.foodcont.2018.11.029
Patange, A., Boehm, D., Ziuzina, D., Cullen, P. J., Gilmore, B., and Bourke, P. (2019). High voltage atmospheric cold air plasma control of bacterial biofilms on fresh produce. Int. J. Food Microbiol. 293, 137–145. doi: 10.1016/j.ijfoodmicro.2019.01.005
Pompl, P., Jamitzky, F., Shimizu, T., Steffes, B., Bunk, W., Schmidt, H. U., et al. (2009). The effect of lowtemperature plasma on bacteria as observed by repeated AFM imaging. New J. Phys. 11:115023. doi: 10.1088/1367-2630/11/11/115023
Potron, A., Poirel, L., and Nordmann, P. (2011). Plasmid-mediated transfer of the bla(NDM-1) gene in Gram-negative rods. FEMS Microbiol. Lett. 324, 111–116. doi: 10.1111/j.1574-6968.2011.02392.x
Ren, D., Madsen, J. S., de la Cruz-Perera, C. I., Bergmark, L., Sørensen, S. J., and Burmølle, M. (2014). High-throughput screening of multispecies biofilm formation and quantitative PCR-based assessment of individual species proportions, useful for exploring interspecific bacterial interactions. Microb. Ecol. 68, 146–154. doi: 10.1007/s00248-013-0315-z
Ren, D., Madsen, J. S., Sorensen, S. J., and Burmolle, M. (2015). High prevalence of biofilm synergy among bacterial soil isolates in cocultures indicates bacterial interspecific cooperation. ISME J. 9, 81–89. doi: 10.1038/ismej.2014.96
Rendueles, O., and Ghigo, J. M. (2012). Multi-species biofilms: how to avoid unfriendly neighbors. FEMS Microbiol. Rev. 36, 972–989. doi: 10.1111/j.1574-6976.2012.00328.x
Rice, S. A., Wuertz, S., and Kjelleberg, S. (2016). Next-generation studies of microbial biofilm communities. Microb. Biotechnol. 9, 677–680. doi: 10.1111/1751-7915.12390
Sakudo, A., Miyagi, H., Horikawa, T., Yamashiro, R., and Misawa, T. (2018). Treatment of Helicobacter pylori with dielectric barrier discharge plasma causes UVinduced damage to genomic DNA leading to cell death. Chemosphere 200, 366–372. doi: 10.1016/j.chemosphere.2018.02.115
Scholtz, V., Pazlarova, J., Souskova, H., Khun, J., and Julak, J. (2015). Nonthermal plasma — A tool for decontamination and disinfection. Biotechnol. Adv. 33, 1108–1119. doi: 10.1016/j.biotechadv.2015.01.002
Simmons, M., Drescher, K., Nadell, C. D., and Bucci, V. (2018). Phage mobility is a core determinant of phage-bacteria coexistence in biofilms. ISME J. 12, 531–543. doi: 10.1038/ismej.2017.190
Šimončicová, J., Kryštofová, S., Medvecká, V., Ďurišová, K., and Kaliňáková, B. (2019). Technical applications of plasma treatments: current state and perspectives. Appl. Microbiol. Biotechnol. 103, 5117–5129. doi: 10.1007/s00253-019-09877-x
Souza, J. G. S., Bertolini, M., Thompson, A., Mansfield, J. M., Grassmann, A. A., Maas, K., et al. (2020). Role of glucosyltransferase R in biofilm interactions between Streptococcus oralis and Candida albicans. ISME J. 10:608. doi: 10.1038/s41396-020-0608-4
Sun, P. P., Araud, E. M., Huang, C., Shen, Y., Monroy, G. L., Zhong, S., et al. (2018). Disintegration of simulated drinking water biofilms with arrays of microchannel plasma jets. NPJ Biofilms Microb. 4:24. doi: 10.1038/s41522-018-0063-4
Tan, C. A. Z., Antypas, H., and Kline, K. A. (2020). Overcoming the challenge of establishing biofilms in vivo: a roadmap for Enterococci. Curr. Opin. Microbiol. 53, 9–18. doi: 10.1016/j.mib.2020.01.013
Tanner, W. D., Atkinson, R. M., Goel, R. K., Toleman, M. A., Benson, L. S., Porucznik, C. A., et al. (2017). Horizontal transfer of the blaNDM-1 gene to Pseudomonas aeruginosa and Acinetobacter baumannii in biofilms. FEMS Microbiol. Lett. 364:fnx048. doi: 10.1093/femsle/fnx048
Trifilio, S., Zhou, Z., Fong, J. L., Zomas, A., Liu, D., Zhao, C., et al. (2015). Polymicrobial bacterial or fungal infections: incidence, spectrum of infection, risk factors, and clinical outcomes from a large hematopoietic stem cell transplant center. Transpl. Infect. Dis. 17, 267–274. doi: 10.1111/tid.12363
Tytgat, H. L. P., Nobrega, F. L., van der Oost, J., and de Vos, W. M. (2019). Bowel biofilms: tipping points between a healthy and compromised gut? Trends Microbiol. 27, 17–25. doi: 10.1016/j.tim.2018.08.009
Van Der Paal, J., Neyts, E. C., Verlackt, C. C. W., and Bogaerts, A. (2016). Effect of lipid peroxidation on membrane permeability of cancer and normal cells subjected to oxidative stress. Chem. Sci. 7, 489–498. doi: 10.1039/c5sc02311d
Vatansever, F., de Melo, W. C., Avci, P., Vecchio, D., Sadasivam, M., Gupta, A., et al. (2013). Antimicrobial strategies centered around reactive oxygen species - bactericidal antibiotics, photodynamic therapy, and beyond. FEMS Microbiol. Rev. 37, 955–989. doi: 10.1111/1574-6976.12026
Winkelstroter, L. K., Tulini, F. L., and De Martinis, E. C. P. (2015). Identification of the bacteriocin produced by cheese isolate Lactobacillus paraplantarum FT259 and its potential influence on Listeria monocytogenes biofilm formation. LWT Food Sci. Tech. 64, 586–592. doi: 10.1016/j.lwt.2015.06.014
Yang, Y., Wang, H., Zhou, H., Hu, Z., Shang, W., Rao, Y., et al. (2020). Protective effect of the golden staphyloxanthin biosynthesis pathway on Staphylococcus aureus under cold atmospheric plasma treatment. Appl. Environ. Microbiol. 86:e01998-19. doi: 10.1128/AEM.01998-19
Yuan, L., Hansen, M. F., Røder, H. L., Wang, N., Burmølle, M., and He, G. (2019). Mixed-species biofilms in the food industry: current knowledge and novel control strategies. Crit. Rev. Food Sci. Nutr. 2019, 1–7. doi: 10.1080/10408398.2019.1632790
Yusupov, M., Bogaerts, A., Huygh, S., Snoeckx, R., van Duin, A. C. T., and Neyts, E. C. (2013). Plasma-induced destruction of bacterial cell wall components: a reactive molecular dynamics dimulation. J. Phys. Chem. C 117, 5993–5998. doi: 10.1021/jp3128516
Yusupov, M., Neyts, E. C., Khalilov, U., Snoeckx, R., van Duin, A. C. T., and Bogaerts, A. (2012). Atomic-scale simulations of reactive oxygen plasma species interacting with bacterial cell walls. New J. Phys. 14:093043. doi: 10.1088/1367-2630/14/9/093043
Keywords: mixed-species biofilms, cold atmospheric plasma, biofilm resistance, biofilm infection, biofilm eradication
Citation: Rao Y, Shang W, Yang Y, Zhou R and Rao X (2020) Fighting Mixed-Species Microbial Biofilms With Cold Atmospheric Plasma. Front. Microbiol. 11:1000. doi: 10.3389/fmicb.2020.01000
Received: 01 February 2020; Accepted: 24 April 2020;
Published: 20 May 2020.
Edited by:
Luis Cláudio Nascimento da Silva, Universidade Ceuma, BrazilReviewed by:
Lucía Fernández, Institute of Dairy Products of Asturias (IPLA), SpainCopyright © 2020 Rao, Shang, Yang, Zhou and Rao. This is an open-access article distributed under the terms of the Creative Commons Attribution License (CC BY). The use, distribution or reproduction in other forums is permitted, provided the original author(s) and the copyright owner(s) are credited and that the original publication in this journal is cited, in accordance with accepted academic practice. No use, distribution or reproduction is permitted which does not comply with these terms.
*Correspondence: Renjie Zhou, emhvdV9yakBhbGl5dW4uY29t; Xiancai Rao, cmFveGlhbmNhaUAxMjYuY29t
Disclaimer: All claims expressed in this article are solely those of the authors and do not necessarily represent those of their affiliated organizations, or those of the publisher, the editors and the reviewers. Any product that may be evaluated in this article or claim that may be made by its manufacturer is not guaranteed or endorsed by the publisher.
Research integrity at Frontiers
Learn more about the work of our research integrity team to safeguard the quality of each article we publish.