- 1School of Biological Sciences, University of Reading, Reading, United Kingdom
- 2STLO, INRAE, Institut Agro, Rennes, France
- 3Inserm 1107, Neuro-Dol, Université Clermont Auvergne, Clermont-Ferrand, France
- 4UFR Sciences de la Vie et de l’Environnement, Université de Rennes I, Rennes, France
- 5Food and Nutritional Sciences, University of Reading, Reading, United Kingdom
Salmonella Enteritidis is the most prevalent food-borne pathogen associated with egg-related outbreaks in the European Union. During egg colonization, S. Enteritidis must resist the powerful anti-bacterial activities of egg white (EW) and overcome ovotransferrin-imposed iron-restriction (the most important anti-bacterial mechanism of EW). Many pathogens respond to iron restriction by secreting iron-chelating chemicals called siderophores but EW contains a siderophore-sequestering “lipocalin” protein (Ex-FABP) that is predicted to limit the usefulness of siderophores in EW. S. Enteritidis produces two siderophores: enterobactin, which is strongly bound by Ex-FABP; and the di-glucosylated enterobactin-derivative, salmochelin (a so-called “stealth” siderophore), which is not recognized by Ex-FABP. Thus, production of salmochelin may allow S. Enteritidis to escape Ex-FABP-mediated growth inhibition under iron restriction although it is unclear whether its EW concentration is sufficient to inhibit pathogens. Further, two other lipocalins (Cal-γ and α-1-ovoglycoprotein) are found in EW but their siderophore sequestration potential remains unexplored. In addition, the effect of EW lipocalins on the major EW pathogen, S. Enteritidis, has yet to be reported. We overexpressed and purified the three lipocalins of EW and investigated their ability to interact with the siderophores of S. Enteritidis, as well as their EW concentrations. The results show that Ex-FABP is present in EW at concentrations (5.1 μM) sufficient to inhibit growth of a salmochelin-deficient S. Enteritidis mutant under iron restriction but has little impact on the salmochelin-producing wildtype. Neither Cal-γ nor α-1-ovoglycoprotein bind salmochelin or enterobactin, nor do they inhibit iron-restricted growth of S. Enteritidis. However, both are present in EW at significant concentrations (5.6 and 233 μM, respectively) indicating that α-1-ovoglycoprotein is the 4th most abundant protein in EW, with Cal-γ and Ex-FABP at 11th and 12th most abundant. Further, we confirm the preference (16-fold) of Ex-FABP for the ferrated form (Kd of 5.3 nM) of enterobactin over the iron-free form (Kd of 86.2 nM), and its lack of affinity for salmochelin. In conclusion, our findings show that salmochelin production by S. Enteritidis enables this key egg-associated pathogen to overcome the enterobactin-sequestration activity of Ex-FABP when this lipocalin is provided at levels found in EW.
Introduction
From 2010 to 2013, the number of confirmed human salmonellosis cases in the European Union (EU) decreased by ∼15% (Efsa Panel on Biological Hazards, 2019) but increased again after 2014 (Efsa Panel on Biological Hazards, 2019; ECDC and EFSA, 2017) with more than 750 strongly-evidenced food-borne Salmonella outbreaks reported between 2014 and 2016. Of these cases, eggs and egg products were identified as the main vehicle of infection (276 cases). In comparison, broiler meat (Gallus gallus) and derived meat products were responsible for only 46 such outbreaks (Efsa Panel on Biological Hazards, 2019). Together, Salmonella enterica serovars Enteritidis (SE) and Typhimurium were the most frequently detected in food-borne outbreaks linked with egg and egg products in the EU (92 and 5.9% of outbreaks, respectively), with SE being by far the most prevalent serovar (Efsa Panel on Biological Hazards, 2019). This indicates that, despite the current controls, SE infection of eggs remains a serious threat to health in the EU (Efsa Panel on Biological Hazards, 2014; ECDC and EFSA, 2017; Efsa Panel on Biological Hazards, 2019).
The two possible routes of egg contamination are horizontal and vertical transmission. The former refers to penetration through the eggshell while the latter results from the infection of reproductive organs (Gantois et al., 2009). During egg formation, SE contamination of both the yolk and the egg white (EW) have been reported (Gantois et al., 2009 for a review). Although there is no clear consensus in the literature, it has been suggested that the albumen is the more likely site of initial infection than the yolk (Gast and Beard, 1990; Humphrey et al., 1991; Keller et al., 1995). During horizontal transmission, colonization and survival in EW also seem crucial as SE penetrates through the shell and encounters this medium prior to the vitelline membrane and the yolk.
Egg white carries a powerful set of antimicrobial activities that SE must resist in order to mediate egg-linked infections (Clavijo et al., 2006; Vylder et al., 2013; Baron et al., 2016). The antibacterial properties of EW include its high pH and viscosity, and a diverse range of antibacterial proteins/peptide (Baron et al., 2016). However, the major factor limiting bacterial growth in EW is iron restriction (Schade and Caroline, 1944; Garibaldi, 1970; Lock and Board, 1992; Baron et al., 1997). Iron restriction arises from the high levels (∼170 μM; second most abundant EW protein) of ovotransferrin (oTf; also known as conalbumin; Alderton et al., 1946), a powerful Fe3+-chelating protein (binding constant of 1032 M−1; Chart and Rowe, 1993). OTf is closely related to serum Tf which delivers iron between tissues in man and other vertebrates (Williams, 1968). The iron-binding capacity of oTf outstrips the iron content of EW (0.1 mg iron per 100 g; Nys and Sauveur, 2004; USDA, 2010) by ∼20-fold which suggests that there is virtually no “free” iron in EW (Julien et al., 2019). This high oTF:Fe ratio of EW resembles the conditions of serum where the partial iron saturation of circulating Tf (∼30%; Kelly et al., 2017) causes a powerful bacteriostatic iron-restriction effect (Bullen et al., 1978).
An important mechanism used by bacteria to overcome iron restriction involves the secretion of high-affinity ferric-iron-chelating molecules called siderophores (Andrews et al., 2003). SE produces two related siderophores, enterobactin (Ent) and salmochelin (Sal), both of which are catecholates (Pollack et al., 1970). Ent is a serine macrotrilactone and Sal is its glucosylated derivative. Hence the term Sal can refer to both mono-glucosylated Ent (MGE) and di-glucosylated Ent (DGE). Ent is reported as the strongest siderophore known, binding to ferric ion with an affinity constant of 1052 M−1 (Chart and Rowe, 1993). Because Ent has a higher affinity for iron than oTf/Tf, Ent-producing bacteria can acquire iron from both oTf and Tf (Ford et al., 1988; Carrano and Raymond, 1979; Chart and Rowe, 1993). The genetic locus responsible for this glucosylation of Ent in Salmonella is the iroBCDEN gene cluster (Bäumler et al., 1998). The di-glucosylation of Ent to generate Sal is catalyzed by the glucosyltransferase, IroB (Hantke et al., 2003; Bister et al., 2004). The resulting Sal is then exported across the cytosolic membrane by IroC (Crouch et al., 2008). Uptake and utilization are mediated by IroD, IroE, and IroN (Müller et al., 2009 for a review). Although the affinity of Sal for Fe3+ is not reported (Valdebenito et al., 2007; Watts et al., 2012), there is no indication that glucosylation significantly impacts Fe3+ ligation or affinity (Luo et al., 2006).
The host immune system can counter the action of bacterial siderophores through release of siderophore-binding proteins that remove ferri-siderophores from circulation (Clifton et al., 2009). These proteins belong to the lipocalin superfamily which includes members with a diverse range of functions beyond siderophore sequestration (Johnstone and Nolan, 2015). Human lipocalin 2 (LCN2) is the best-known example of a siderophore-binding lipocalin; it is able to sequester a variety of bacterial siderophores, including Ent (Goetz et al., 2002; Holmes et al., 2005). However, many bacteria can overcome the siderophore-sequestration activities of LCN2 by producing “stealth” siderophores, such Sal, which are not recognized by LCN2 (Fischbach et al., 2006; Valdebenito et al., 2007).
In EW, three lipocalin proteins have been identified: the extra fatty acid binding protein (Ex-FABP, or Ch21); the chondrogenesis-associated lipocalin (Cal-γ or prostaglandin D synthase); and α-1-ovoglycoprotein (Guérin-Dubiard et al., 2006; Mann, 2007; D’Ambrosio et al., 2008; Mann and Mann, 2011). Of these three EW proteins, siderophore-binding function has only been explored for Ex-FABP. Ex-FABP was found to have similar function to LCN2 in sequestering ferric-Ent with high affinity (equilibrium dissociation constant, Kd, of 0.22 nM; Correnti et al., 2011). Ex-FABP also inhibits iron-restricted growth of a strain of Escherichia coli producing Ent as sole siderophore (Correnti et al., 2011), but not strains producing Sal (Garénaux et al., 2013). However, it is unclear whether Ex-FABP is present in EW at concentrations sufficient to inhibit growth of Ent-producing bacteria. Moreover, it is unclear whether the two other lipocalins of EW (Cal-γ and α-1-glycoprotein) might also sequester siderophores. Further, the capacity of Ex-FABP to inhibit the key EW-mediated pathogen, SE, under iron restriction has yet to be explored.
The aim of the research reported here was to explore whether the three lipocalins of EW can sequester the two siderophores produced by SE and to determine whether the EW lipocalins can inhibit SE growth under the iron-restricted conditions. The results show that only Ex-FABP inhibits iron restricted growth of SE and binds Ent with high affinity, and that it is present in EW at levels sufficient to inhibit growth when Ent is the sole siderophore produced. The results reported thus suggest that Ex-FABP is an effective component of the antibacterial repertoire of EW.
Materials and Methods
Growth Media, Strains, and Plasmids
Lysogeny broth (LB) was used as the growth medium for E. coli, whereas either LB or Tryptone Soy Broth (TSB, Sigma-Aldrich) were used for the growth of S. enterica. M9 salts (Sigma-Aldrich) minimal medium (with 1 mM MgSO4.7H2O, 0.2% w/v glucose, 20 μM thiamine, 0.1 mM CaCl2, 0.3% w/v casamino acids) and acid washed glassware were used to achieve iron-restricted growth. Ferric citrate (10 μM) was added to achieve iron sufficiency. Growth in liquid medium was at 37°C with shaking generally at 250 rpm. E. coli TOP10 was used for cloning work and E. coli K-12 BW25113 ΔentB::kan (λDE3) was used as overexpression host. S. enterica serovar Enteritidis PT4-P125109 was used for growth tests and gene knockout. The bacterial strains and plasmids used and generated are listed in Tables 1, 2. Siderophore detection assay was performed using CAS (chrome azurol S) plates according to Louden et al. (2011).
Inactivation of Genes Required for Enterobactin and Salmochelin Production/Utilization
The entB (enterobactin biosynthesis), iroBC (salmochelin uptake and synthesis), and iroDEN (salmochelin uptake and utilization) genes of SE were inactivated using the λRed gene disruption system encoded by plasmid pKD46 (Datsenko and Wanner, 2000; Supplementary Figure S1 and Supplementary Table S1). The chloramphenicol resistance cassette of pKD3 inserted in the genome was subsequently deleted using FLP recombinase encoded by the temperature-sensitive plasmid, pCP20 (Cherepanov and Wackernagel, 1995) to generate clean deletions. Mutants were confirmed by whole-genome sequencing (Illumina; MicrobesNG, Birmingham); corresponding genome sequences were deposited at the ENA database under the following project accession number: PRJEB36543. General molecular genetic methods were as previously described (Sambrook and Russell, 2001).
Cloning of Lipocalin Genes for Over-Production in E. coli
Nucleotide sequences for the human LCN2 and chicken Ex-FABP, α-1-ovoglycoprotein and Cal-γ genes were obtained from the NCBI GeneBank database using the ID numbers 3934, 396393, 395220, and 374110, respectively. The sequences were codon optimized for expression in E. coli (GeneArt optimisation portal;1) (see Supplementary Figure S2 for corresponding sequences) and then synthesized by GeneArt (Invitrogen, Germany) with flanking restriction sites to assist subsequent cloning from the pMK carrier plasmid together with an N-terminal signal sequence (except for LCN2, which does not have an N-terminal signal sequence) to allow secretion of the encoded hexa-His tagged proteins into the periplasm. The lipocalin-coding regions were released from the pMK plasmid using NdeI and XhoI, and then ligated into the corresponding sites of plasmid pET21a using the Rapid DNA Ligation kit (Thermo Fisher Scientific). The resulting constructs were confirmed by restriction digestion and nucleotide sequencing (Eurofins). E. coli K-12 BW25113 ΔentB::kan (JW0587) was converted to λDE3-lysogen status using a Lysogenization kit (Novagen) and the resulting strain then acted as host for over-expression of the lipocalin genes from pET21a. This enterobactin-deficient host strain was used to avoid association of overexpressed lipocalins with host-specified enterobactin.
Lipocalin Purification
Escherichia coli JW0587(λDE3) transformants, carrying a corresponding lipocalin-expressing pET21a plasmid, were grown in LB (supplemented with 10 μM Fe-citrate) and expression was induced with 0.5 mM IPTG. Cells pellet were harvested by centrifugation (3000 × g for 20 min at 4°C), resuspended in 1 mL of osmotic shock solution (20% sucrose, 30 mM Tris pH 8.0, 10 mM EDTA) per 50 mg of cells (∼5 g in total). After 10 min at room temperature, the supernatant was harvested as above and dialysed thoroughly against binding buffer 1 (0.3 M NaCl, 50 mM Na3HPO4, 15 mM imidazole, pH 8.0) at 4°C. The C-terminally hexa-His-tagged lipocalin proteins were isolated from the retentate by Ni2+-affinity chromatography using a 5 mL Mini Nuvia IMAC Ni-charged cartridge (Bio-Rad) according to manufacturer’s instructions. Eluted Ex-FABP and α-1-ovoglycoprotein were further purified using a 5 mL HiScreen Capto Diethylaminoethyl column (DEAE-sepharose, GE Healthcare) equilibrated in binding buffer 2 (20 mM Tris–HCl, pH 8) with elution achieved with a linear gradient of 1 M NaCl in the same buffer. LCN2 and Cal-γ were further purified using a 5 mL HiScreen Capto Multimodel Cation exchanger ImpRes column (MMC, GE Healthcare) equilibrated with binding buffer 3 (50 mM sodium acetate, pH 6); elution was achieved with a linear gradient of 1 M NaCl in the same buffer or in Tris–HCl at pH 8. The purity of the resulting proteins was determined by SDS-PAGE (Sambrook and Russell, 2001; Figure 1A) and identities were confirmed by electrospray-ionisation mass-spectrometry (ESI-MS). Bradford protein assay (Bio-Rad) and absorbance at 280 nm were used to estimate protein concentrations. Proteins were stored in phosphate-buffered saline (PBS, pH 7.4) at −80°C.
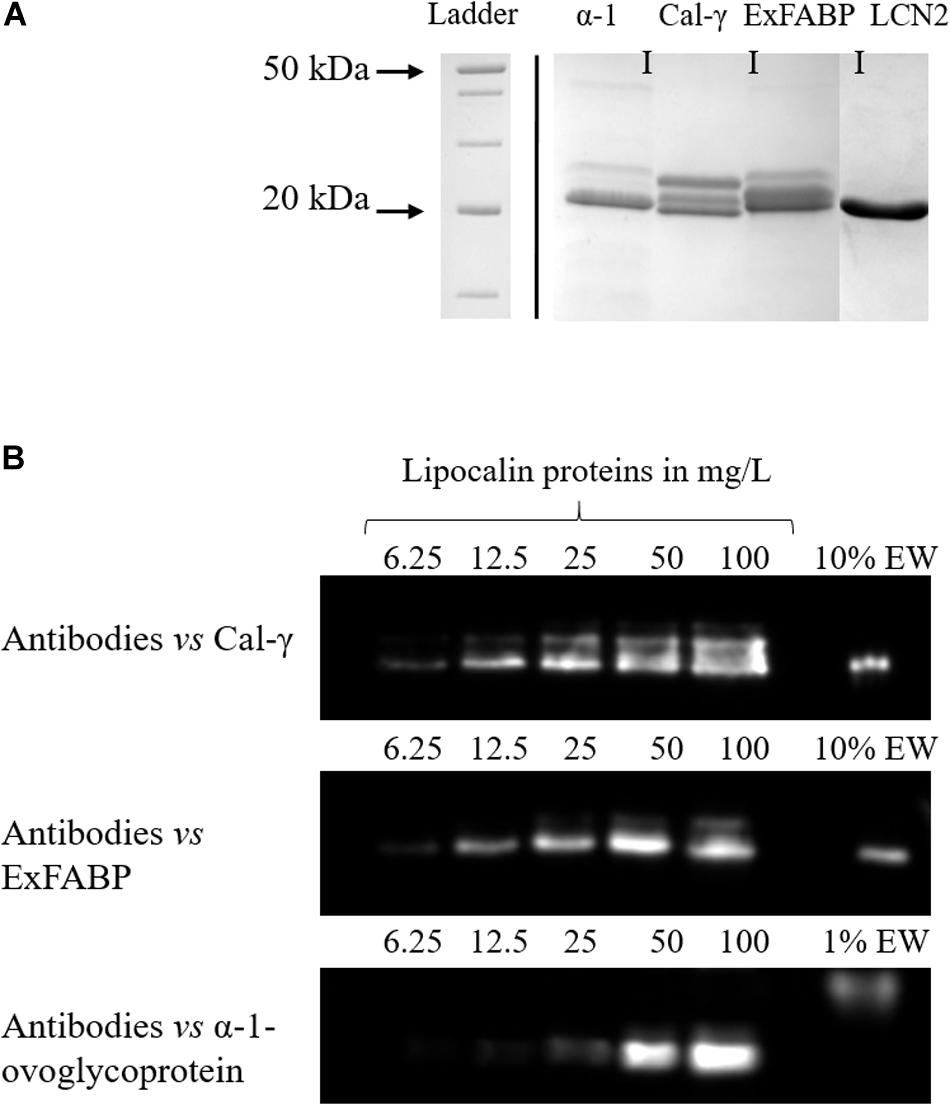
Figure 1. Purification of lipocalins (A) and quantification of lipocalin levels in EW (B). (A) SDS-PAGE analysis of overproduced, purified EW lipocalins. (B) Western-blot analysis of a range of EW lipocalin levels (as indicated) as well as a sample of EW (diluted 1:10 or 1:100 in dH2O). The calibration curves of purified EW lipocalins used to calculate the amounts found naturally in EW are provided in the Supplementary Figure S3.
Electrospray Ionization – Mass Spectrometry
Isolated lipocalin proteins were dialysed against ultrapure water prior to analysis by MicroTOF-Q mass spectrometry (column Ace C8 50 × 2.1, mass spectrometer Thermo Scientific LTQ Orbitrap XL with an ESI source via a Thermo Scientific Accela HPLC). Mass spectrum peaks were averaged and deconvolution to uncharged neutral mass was performed using Xtract within the Xcalibur software (the BioCentre, University of Reading).
Western-Blot Analysis of Lipocalin Levels in EW
Polyclonal antibodies were raised in two New Zealand White rabbits by DC Biosciences (Dundee, United Kingdom) using 2–2.5 mg of each of the three purified chicken-EW lipocalin proteins. A 90-day protocol using Freud’s adjuvant as a stimulating agent was followed. The levels of each lipocalin in EW were then determined by western blotting (Mahmood and Yang, 2012) using corresponding lipocalin standards (6.25; 12.5; 50; 100 mg/L) along with EW dilutions (1:10 or 1:100 in qH2O). Immunodetection was achieved using the primary antibodies raised in rabbit, and secondary anti-rabbit IgG antibody raised in goat and HRP-conjugated (Sigma-Aldrich batch A-6154). Westar C 2.0 chemiluminescent substrate (Cyanagen) was used for signal development. The membrane was imaged using a Syngene G:BOX. Images were analyzed with ImageJ software in order to band determine signal intensities. The lipocalin quantification was repeated three times with eggs of three different egg brands (free range eggs, the Cooperative; free range eggs, Clarence Court; and free-range eggs, Happy Egg). All eggs were certified Class A and produced in the United Kingdom. From the three concentration values gathered, an average and standard error were calculated.
Isothermal Titration Calorimetry
Experiments were carried out with a GE Healthcare Microcal VP-ITC microcalorimeter according to the manufacturer’s guidelines (Operating Instructions 28-9639-79 AA). The device consisted of a motorized injection syringe, in which the ligand was loaded, with two adiabatic cells set at 30 °C. The left cell was filled with the reference (TBS with 1.3% DMSO), whereas the right cell was filled with 5 μM lipocalin protein dissolved in the same buffer. Sequential 10 μL injections (29 in total) of ligand were applied consisting of either 50 μM enterobactin (Sigma-Aldrich) or 50 μM salmochelin (EMC siderophore) dissolved in TBS (pH 7.4) with 1.3% DMSO. Samples were degassed and pre-equilibrated at the desired temperature using a GE MicroCal ThermoVac degassing station before loading into the syringe, sample cell and reference cell.
Growth Tests in Liquid Medium
Growth comparison in iron-free M9 medium (with/without 10 μM ferric citrate) was performed in 100-deepwell Honeycomb plates incubated in a Bioscreen C (Lab System) apparatus (at 37°C with constant shaking at 200 rpm) for ∼20 h. Growth comparison in LB medium (with/without 200 μM 2,2 dipyridyl; DIP; an iron chelator) was achieved using 96-well microplates incubated in a Spectrophotometer SpectraMax 340 PC (Molecular devices) at 37°C with 60 s shaking at 200 rpm every 15 min (for ∼20 h).
Results and Discussion
Isolation of EW Lipocalins
The genes encoding the three EW lipocalins (Ex-FABP, Cal-γ, and α1-glycoprotein) and human lipocalin 2 (LCN2) were codon-optimized (for E. coli) and overexpressed in E. coli to give C-terminal hexa-His-tagged recombinant proteins, as described in Experimental Procedures. Purification was then achieved using Ni2+-affinity and ion-exchange chromatography. SDS-PAGE analysis indicated a purity of ≥90% and polypeptides of the anticipated mobility (Figure 1A). ESI-MS of α-1-ovoglycoprotein and Ex-FABP showed that, in each case, the major polypeptide was a close match to the theoretical mass of the mature forms (Table 3), indicating cleavage of the PelBss had been achieved during secretion. However, lower levels of the immature form of α-1-ovoglycoprotein were recovered (Figure 1A and Table 1). For Cal-γ, ESI-MS indicated a mass that is 763 Da greater than that predicted for both the major mature and minor immature forms. ESI-MS analysis carried out under native and denaturing conditions showed that the additional mass was likely covalently association, and MALDI-ISD showed that the N- and C-terminal amino acid sequences (residues 1–36 and 161–173) of the mature form were as expected. Thus, the nature of the additional 763 Da component remains unclear.
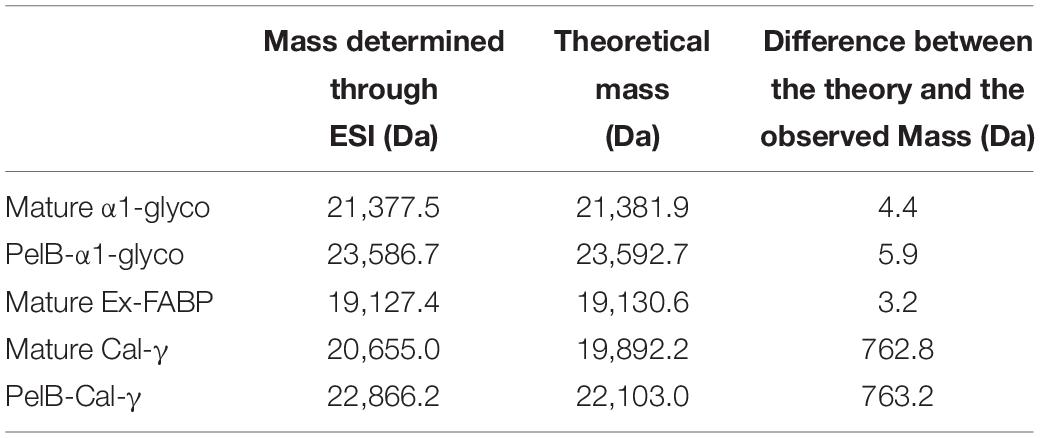
Table 3. Comparison of the purification products detected through ESI-MS and the theoretical mass of the lipocalin-like proteins.
EW Contains Micro-Molar Levels of All Three Lipocalins
The purified EW lipocalins were used to raise antibodies in rabbit to enable quantification of the lipocalins in EW by western blotting (Figure 1B). Western-blot analysis showed that all three lipocalins are indeed present in EW at detectable levels, with mobilities matching those of the purified proteins for Ex-FABP and Cal-γ (Figure 1B). However, α-1-ovoglycoprotein in EW showed a higher apparent mass (37.4 kDa) than that of the recombinant protein (22.7 kDa). Haginaka et al. (1995) also reported a higher mass for α-1-ovoglycoprotein in EW by MALDI-TOF mass spectroscopy (30 kDa). These discrepancies are presumed to arise from the N-type glycosylation of α-1-ovoglycoprotein within EW (30.3% total glycosylation; Ketterer, 1965) resulting in aberrant mobility of glycosylated proteins during SDS-PAGE (Guérin-Dubiard et al., 2006). The semi-quantitative western blots indicated EW concentrations of 232.9, 5.6, and 5.1 μM for α-1-ovoglycoprotein, Cal-γ, and Ex-FABP, respectively (Table 4). They are therefore the 4th, 11th, and 12th most abundant proteins in EW by mass (Kovacs-Nolan et al., 2005). The levels observed were relatively consistent between egg producers which suggests that the abundances reported here are likely to be generally reflective of those found in chicken eggs. Although the 2D-PAGE and MS analyses of previous workers (Guérin-Dubiard et al., 2006) indicated that Ex-FABP and Cal-γ are considerably less abundant in EW than α-1-ovoglycoprotein, no concentration values had been assigned to Ex-FABP and Cal-γ in EW, until the results reported herein.
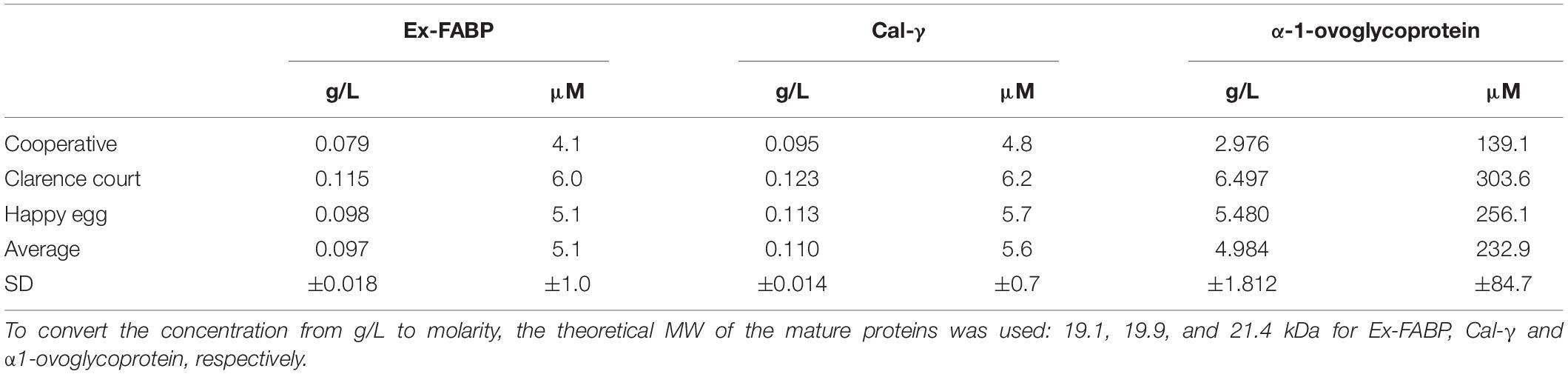
Table 4. Concentration of lipocalin-like proteins found in EW (three trademarks coming from different providers in the United Kingdom) according to western-blot analysis.
The level of α-1-ovoglycoprotein in EW was previously estimated as ∼1 g L−1 (Ketterer, 1965), whereas here a considerably higher concentration of 232.9 μM (or 5 g L−1) is reported (Table 4). This difference may reflect the use of alcohol precipitation before quantification in the previous report (Ketterer, 1965), which could lead to incomplete protein recovery. Since in the work reported here quantification was achieved directly on EW, it seems reasonable to suggest that the estimation obtained is closer to the true value but might still represent an underestimate as the glycosylation of the native EW protein might reduce its immunoreactivity against the antibodies raised to the non-glycosylated form. Given the previous classification of EW proteins based on abundance (Kovacs-Nolan et al., 2005), α-1-ovoglycoprotein belongs to the high concentration group of “major EW protein” set along with ovalbumin (54 g L−1), ovotransferrin (12.0 g L−1), ovomucoid (11 g L−1), globulin (4 g L−1), ovomucin (3.5 g L−1) lysozyme (3.4 g L−1), and ovoinhibitor (1.5 g L−1). However, Cal-γ and Ex-FABP, present at 5.6 and 5.1 μM (or 0.11 and 0.10 g L−1), would belong to the “minor EW protein” set, which include ovoflavoprotein (0.8 g L−1), ovostatin (0.5 g L−1), cystatin (0.05 g L−1), and avidin (0.05 g L−1).
Ex-FABP Binds Enterobactin With High Affinity and Strong Preference for the Ferrated Form
The ability of the three EW lipocalins to specifically bind the iron-free forms of Ent (apoEnt) and Sal (diglucosylated form, apoDGE) was compared with that of LCN2, using isothermal titration calorimetry (ITC). LCN2 bound apoEnt in an exothermic reaction with a high affinity, giving a dissociation constant (Kd) of 58 ± 12 nM (at pH 7.4; Table 5 and Supplementary Figure S4), which is 16-fold higher than the value previously reported using tryptophan quenching fluorescence (TQF; 3.57 nM; Abergel et al., 2006). No binding was observed with apoDGE (Supplementary Figure S4), which matches previous findings showing that LCN2 does not bind DGE (Fischbach et al., 2006). Ex-FABP also bound apoEnt at high affinity, giving an exothermic reaction with a dissociation constant (Kd) of 86 ± 5 nM at pH 7.4 (Table 5 and Figure 2A) and likewise failed to interact with apoDGE, as observed previously (Correnti et al., 2011). It should be noted that the Ex-FABP binding affinity for apoEnt found here is 172-fold weaker than that determined previously (0.5 ± 0.15 nM) using TQF under similar (TSB buffer, pH 7.4) conditions (Correnti et al., 2011). This discrepancy could result from the difference in the techniques used (ITC vs TQF), differences in reaction conditions (e.g. Ex-FABP and DMSO concentrations) or differences in protein preparation. For both LCN2 and Ex-FABP, the apoEnt molar-binding stoichiometry was close to 1:1 at 0.872 and 0.617, respectively (Table 5), which again is consistent with previous reports (Goetz et al., 2002; Correnti et al., 2011).
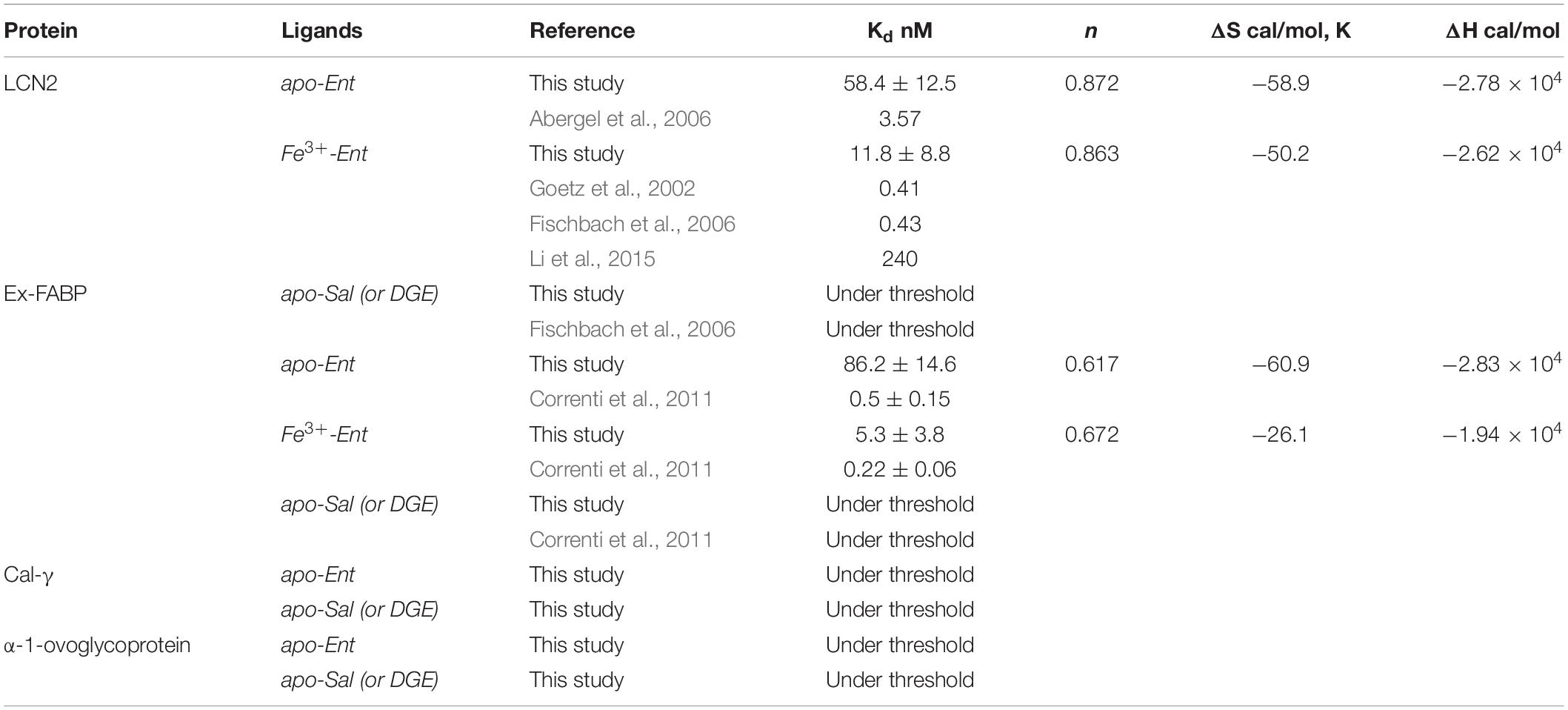
Table 5. Lipocalins-binding affinities towards apo- and ferric-ligands obtained using isothermal titration calorimetry (in TBS pH 7.4 at 30°C) in this study and compared values from the literature. Kd is the equilibrium dissociation constant, n the binding stoichiometry, ΔS the entropy, and ΔH the enthalpy.
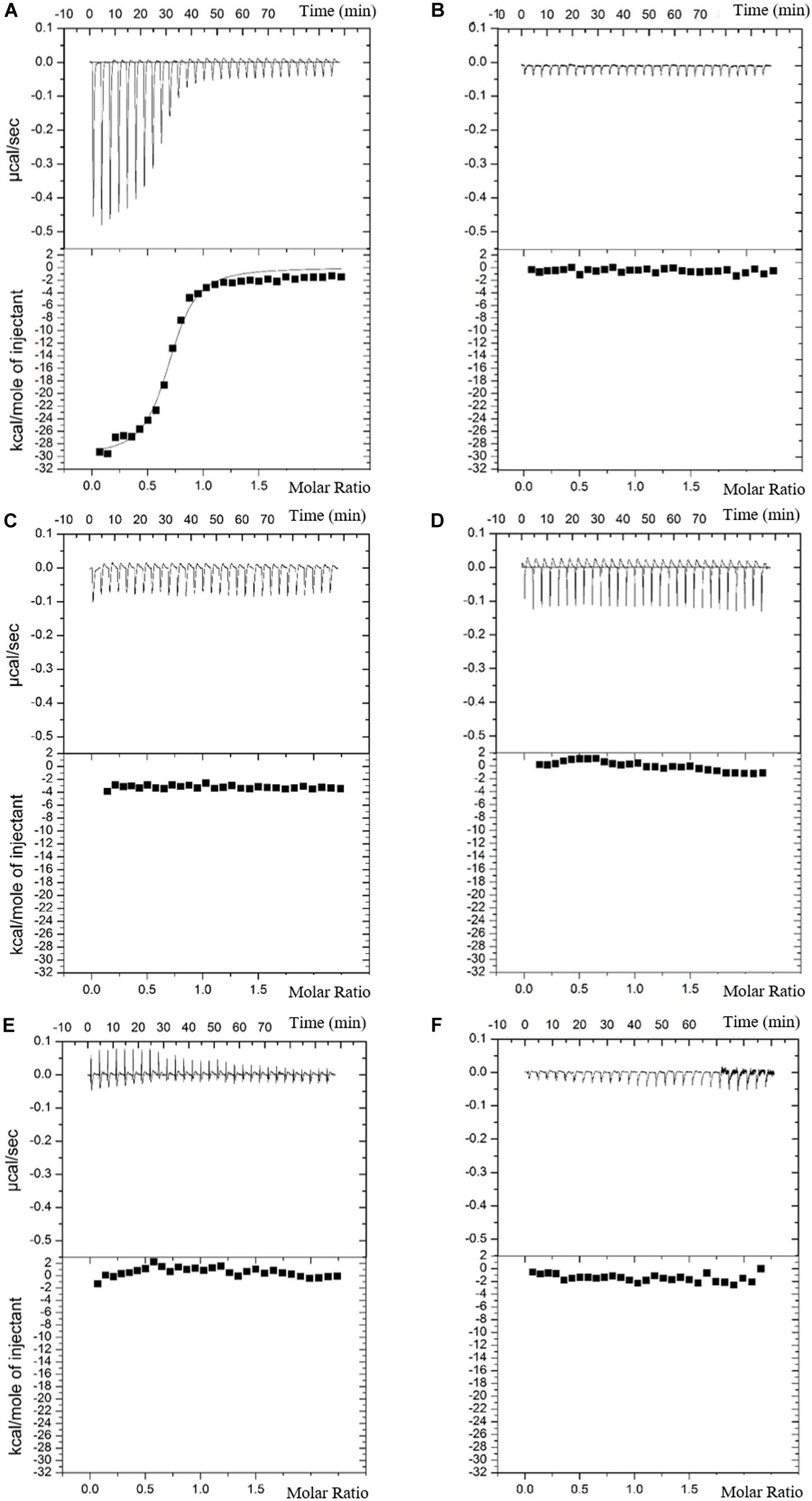
Figure 2. Interactions between lipocalins and SE siderophores. Isothermal titration calorimetry was achieved with 29 injections (10 μL) of 50 μM ligands (Ent and Sal) in an adiabatic well containing 5 μM lipocalins. Proteins and ligands were both dissolved in TBS (20 mM Tris, 500 mM NaCl, 1.3% DMSO, pH 7.4) and experiments were achieved at 30°C. No interactions could be measured between Ex-FABP and apo-Sal (B), whereas a Kd of 86 ± 5 nM was measured between Ex-FABP and apo-Ent (A). No interactions could be measured between Cal-γ and enterobactin (C), Cal-γ and salmochelin (D), α-1-glycoprotein and enterobactin (E) and α-1-glycoprotein and salmochelin (F). Data for LCN2, Fe-Ent are shown in the Supplementary Figure S2.
Since a previous TQF study indicated that Ex-FABP binds Fe3+-Ent with a higher (2.3-fold) affinity than apoEnt (Correnti et al., 2011), and similar findings have been made with LCN2 (8.7-fold; Abergel et al., 2006), the interaction between Fe3+-Ent and Ex-FABP was tested here by ITC. The results show a 16-fold higher affinity (Kd 5.3 ± 3.8 nM) for Fe3+-Ent than apoEnt (Table 5 and Supplementary Figure S4) which indicates a higher preference for the ferric- over the apo-form than was previously suggested (Correnti et al., 2011). The ITC data also indicates that LCN2 possesses a 4.9-fold higher affinity (Kd 11.8 ± 8.8 nM) constant for Fe3+-Ent than for apoEnt (Table 5 and Supplementary Figure S2); a similar higher affinity (8.7-fold) was reported by Abergel et al. (2006), and Bao et al. (2010) also showed that Fe3+ dramatically enhances the affinity of LCN2 for catechols (by at least 95-fold). It should be noted that the ITC-determined binding affinities of LCN2 for Fe3+-Ent reported here (11.8 nM) and previously (240 nM; Li et al., 2015) are both considerably weaker than those determined by TFQ (0.41 and 0.43 nM; Goetz et al., 2002; Fischbach et al., 2006) indicating that differences in published affinity values for LCN2 (and Ex-FABP) are to a large degree related to the methods employed. ITC indicated that neither Cal-γ nor α-1-ovoglycoprotein bind either apoEnt or apoDGE (Table 5 and Figure 2) which suggests that these lipocalins do not act as “siderocalins,” although it remains possible that they could have affinity for siderophores not considered here. It is also important to note that α-1-ovoglycoprotein is glycosylated in EW and such post-translational modifications were not achieved during over-production in E. coli. This lack of glycosylation might have influenced the observed bioactivity of the recombinant protein.
Ex-FABP Inhibits Growth of Salmochelin-Deficient SE Mutants in Iron-Restricted Medium When Provided at Concentrations Found in EW
In order to determine whether EW lipocalins can cause growth inhibition of an SE strain producing Ent, but not Sal, a mutant (ΔiroBC) lacking the ability to produce and export Sal was generated. The iroB gene encodes the glucosyltransferase that converts Ent into Sal, and iroC encodes the transporter required for export of Sal across the cytosolic membrane (Hantke et al., 2003; Bister et al., 2004; Crouch et al., 2008); thus, the iroBC mutant can neither produce nor release Sal, but should retain the capacity to produce and utilize Ent. Furthermore, two other mutants were generated as controls: ΔiroDEN and ΔentB. The former should be able to produce both Ent and Sal but was not expected to utilise Sal due to absence of the IroN OM receptor and IroD/IroE esterases (Müller et al., 2009). The latter (ΔentB) is unable to convert isochorismate to 2,3-dihydro-2,3-dihydroxybenzoate (an Ent precursor; Gehring et al., 1998) and therefore can produce neither Ent nor its derivative, Sal. The genotype of the mutants was confirmed by genome sequencing and propagation on CAS plates (Louden et al., 2011) showed that, as expected, the ΔentB (Ent– Sal–) mutant does not produce siderophore whereas both the ΔiroBC (Ent+ Sal–) and ΔiroDEN (Ent+ Sal+) mutants are siderophore producing (Figure 3).
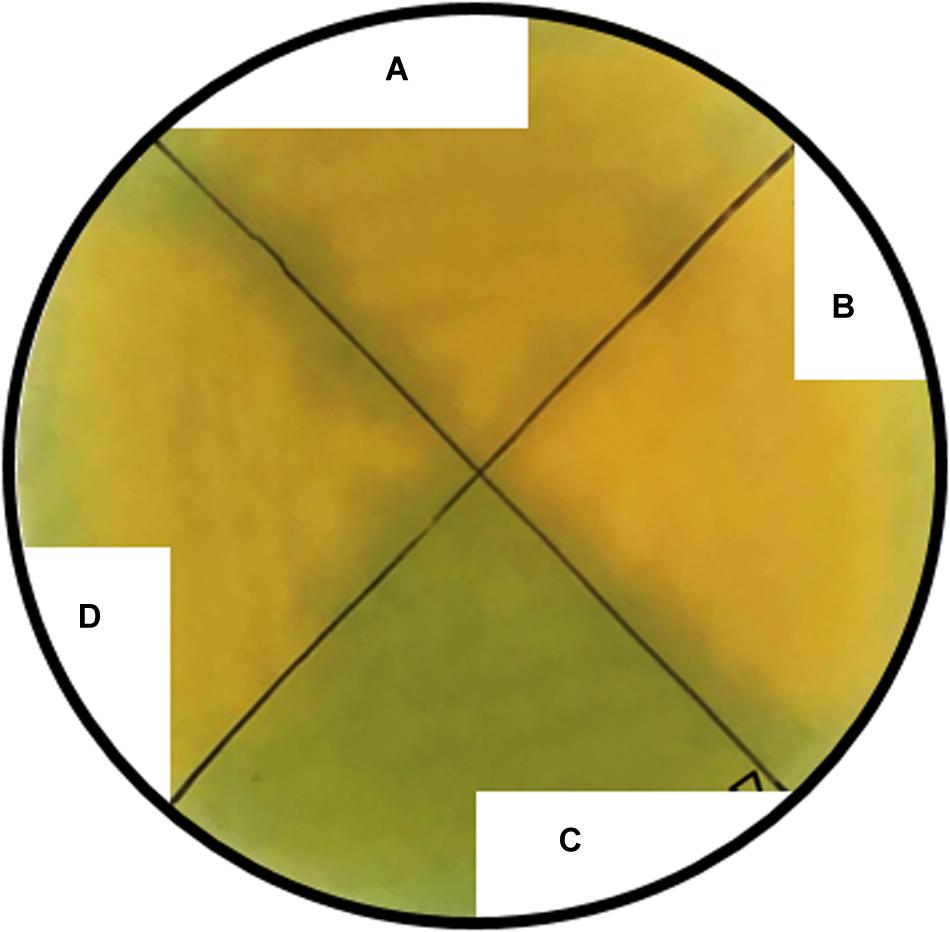
Figure 3. Siderophore production by the wildtype (A), and ΔiroDEN (B), ΔentB (C), and ΔiroBC (D) mutants. All strains were grown on CAS plates to determine siderophore secretion capacity. After 12 h of incubation at 37°C, siderophore-producing strains generated a yellow-golden color while for the non-siderophore producing strain the CAS plate remained green.
To assess whether exposure to EW lipocalins negatively impacts SE growth through Ent sequestration, growth of the siderophore-defective SE mutants and wildtype was monitored in the presence and absence of each of the EW lipocalins and LCN2 (at 5 μM), under low- and sufficient-iron conditions (Figure 4). Of the lipocalins tested, only Ex-FABP and LCN2 had any impact on iron-deficient growth (Figure 4), and none had any impact under iron sufficiency. The ΔiroBC mutant (Ent+ Sal–) was particularly affected with a 2.5-fold growth reduction (at 6 h; Supplementary Figure S5) under low-iron with Ex-FABP or LCN2 (Figures 4E,G). This reduced growth is fully consistent with the Ent sequestering activities of Ex-FABP and LCN2, and the dependence of the ΔiroBC mutant on Ent as the sole siderophore supporting its low-iron growth. The wildtype also displayed a reduced growth with Ex-FABP or LCN2 under low iron, but this was far more modest (at just 1.25-fold; at 6 h; Supplementary Figure S5) than that seen for the ΔiroBC mutant. This mild reduction in iron-restricted growth is consistent with the co-dependence of the wildtype on Ent and Sal under iron deficiency. Thus, production of Sal by the wildtype appears to largely overcome the impact of Fe-Ent sequestration by Ex-FABP or LCN2, as reported previously for other bacteria (Fischbach et al., 2006; Correnti et al., 2011).
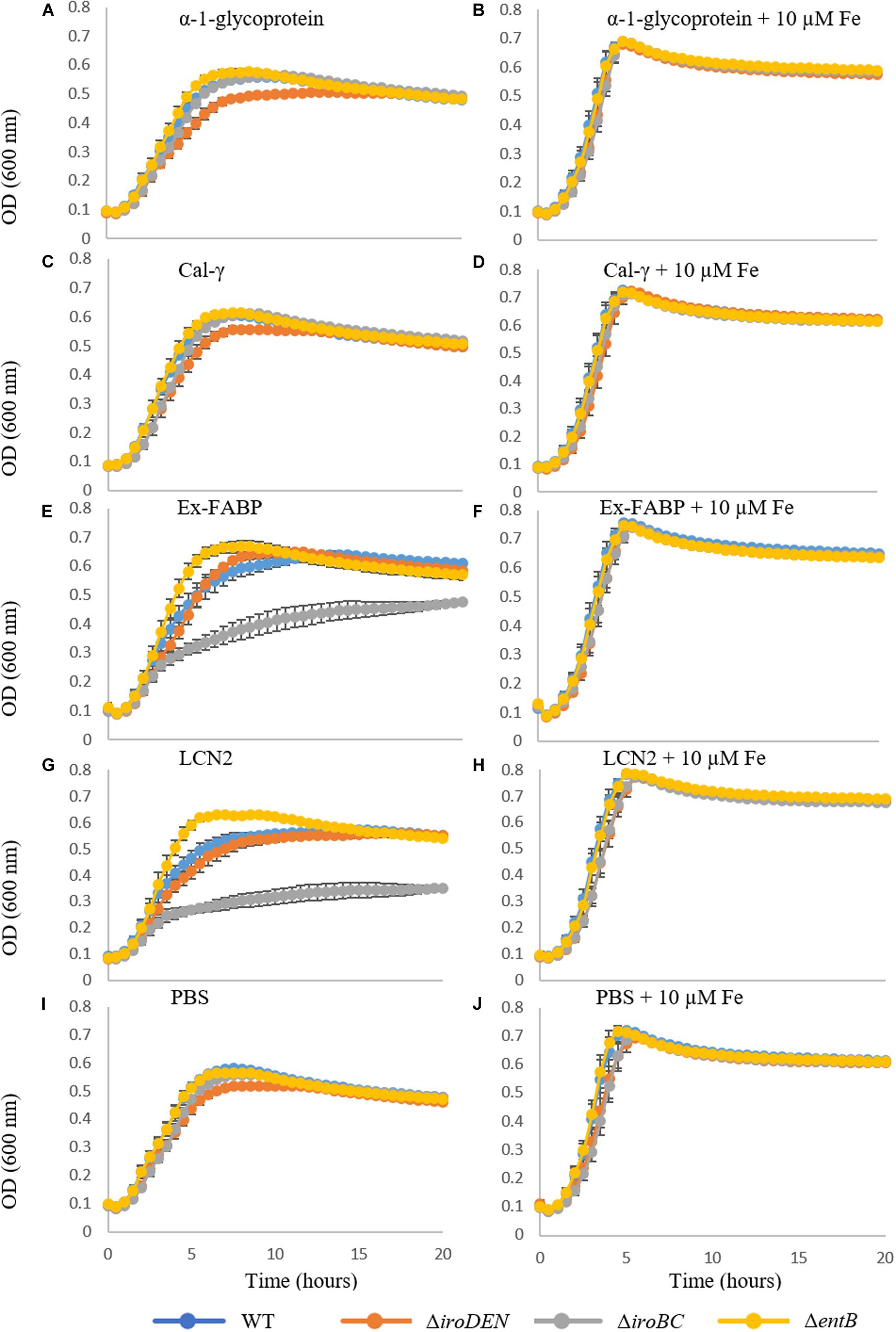
Figure 4. Effect of EW lipocalins on growth of Salmonella Enteritidis and mutants defective in siderophore production and/or utilization under iron restriction. Growth of the WT and the ΔiroBC, ΔiroDEN, and ΔentB mutants was compared in M9 medium with (right) or without (left) 10 μM ferric citrate at 37°C and 200 rpm, for 20 h. The medium included 5 μM of either α-1-glycoprotein (A,B), Cal-γ (C,D), Ex-FABP (E,F), LCN2 (G,H) or ∼100 μL of PBS (I,J). Error bars indicate standard error from three biological replicates with two technical replicates.
In contrast to the ΔiroBC mutant, the ΔentB mutant (Ent– Sal–) exhibited better low-iron growth (1.2-fold at 6 h; Supplementary Figure S5) than the wildtype in the presence of Ex-FABP or LCN2 (Figures 4E,G), with no apparent reduction in growth exhibited in the presence of these lipocalins. The failure of Ex-FABP or LCN2 to inhibit the ΔentB mutant is consistent with the ability of this mutant to escape competition for iron by LCN2 or Ex-FABP due its lack of Ent production. Thus, the results indicate that production of Ent as sole siderophore is deleterious to growth under low-iron conditions in the presence of Ex-FABP or LCN2. The ΔiroDEN mutant showed reduced growth with respect to the other three strains (∼1.1-fold at 6 h) under low-iron conditions in the absence of Ex-FABP or LCN2 (Figure 4I). This indicates that the capacity to secrete Sal, but not utilize it, results in deficient growth under iron restriction, which presumably arises due to sequestration of extracellular iron by secreted Sal. Cal-γ and α-1-ovoglycoprotein had no apparent effect on the growth of SE or its mutants under low- or sufficient-iron conditions (Figure 4). This is consistent with the ITC data which indicate that these lipocalins do not bind Ent or Sal.
In summary, Ex-FABP at 5 μM caused a major inhibition of iron-restricted growth for an SE strain relying on Ent as sole siderophore but had only a modest impact on the wildtype deploying Sal alongside Ent. Since the western blotting data indicate that Ex-FABP is present in EW at 5.1 μM, these results suggest that Ex-FABP concentrations in EW are sufficient to cause inhibition of iron-restricted growth for an SE strain, and potentially other bacterial species, relying on Ent as sole siderophore.
Although no iron-restricted growth defect was observed for the ΔentB mutant in minimal (M9) medium, when growth tests were performed in rich (LB) medium with the iron chelator DIP, a major reduction in growth (∼4-fold), with respect to the wildtype, was observed (Figure 5). No growth defect was seen in the absence of DIP indicating that the phenotype obtained is related to iron restriction imposed by DIP chelation. This observation matches previous work (Liu et al., 2015) and likely arises from the inability of the ΔentB mutant to effectively compete with DIP for iron due to its lack of Ent (and Sal) production. In contrast to the ΔentB mutant, the ΔiroDEN and ΔiroBC strains showed no growth defect in LB with DIP (Figure 5), presumably because they retain the ability to produce and utilize Ent, and can thus compete with DIP for iron.
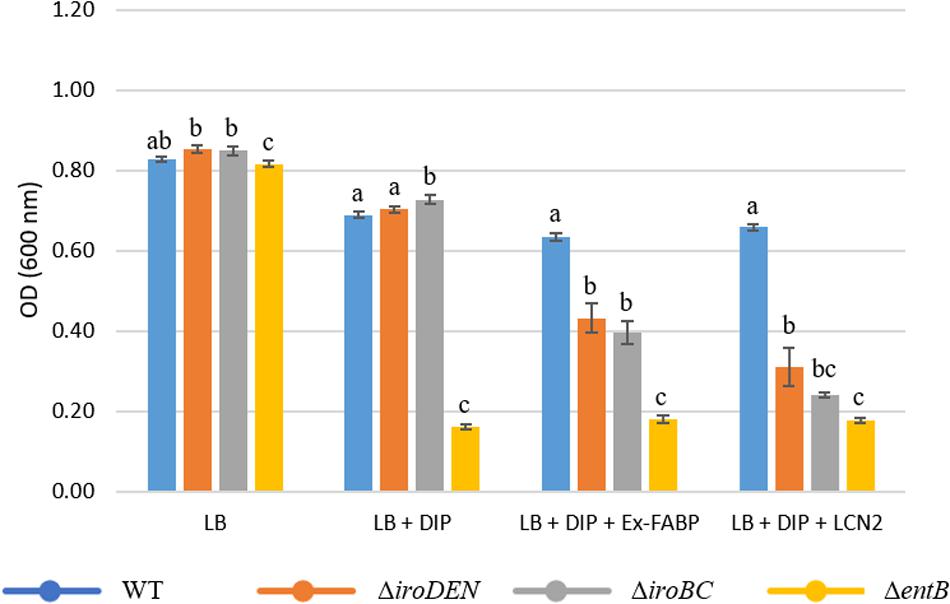
Figure 5. Effect of Ex-FABP on growth of Salmonella Enteritidis and mutants defective in siderophore production and/or utilization under iron restriction in rich medium (LB broth). Growth was at 37°C with shaking and the OD values presented are those achieved at 20 h of growth. The chelator 2,2′-dipyridyl (DIP) was at 200 μM where indicated, and Ex-FABP or LCN2 were present at 5 μM (where indicated). The error bars are the standard error calculated from three biological replicates with three technical replicates. For each growth medium, one-way ANOVA followed by a multiple comparison of means (Tukey Contrasts) was achieved using R software (version 3.5.3). This allowed to identify groups significantly different (identified as “a”, “b”, and “c”).
As expected, the provision of either Ex-FABP or LCN2 had little impact on the weak growth of the ΔentB mutant in LB with DIP (Figure 5). However, the ΔiroDEN and ΔiroBC mutants showed a significant growth defect (∼1.5–2-fold reduction) compared to the wildtype in LB with DIP when 5 μM Ex-FABP or LCN2 were provided (Figure 5). This is consistent with the ability of Ex-FABP and LCN2 to sequester Ent, but not Sal. LCN2 caused a greater growth reduction than Ex-FABP (2-fold cf. 1.5-fold, respectively) for the ΔiroDEN and ΔiroBC mutants, and the growth of ΔiroBC mutant was slightly more reduced than that of the ΔiroDEN mutant (Figure 5).
The findings above are fully consistent with the Ent-sequestering roles of LCN2 and Ex-FABP, as shown here and previously (Goetz et al., 2002; Fischbach et al., 2006; Correnti et al., 2011). The results above are also in accordance with previous work showing that mutations in iroBC (Sal production and export) and iroN (Sal uptake) do not impair growth of S. Typhimurium in rich (LB) medium (Raffatellu et al., 2009) but do significantly reduce growth in (low iron) tissue culture medium (DMEM) containing LCN2. The present study also shows that Ex-FABP is present at sufficient concentration in EW to cause inhibition of iron-restricted growth for an SE strain relying on Ent as sole siderophore, but not for SE deploying Sal alongside Ent. These results reflect previous findings showing that 2.5 and 10 μM Ex-FABP cause iron-restricted growth inhibition of Ent-producing E. coli (Correnti et al., 2011; Garénaux et al., 2013) and that complementation with iroBCDEN reverses inhibition by Ex-FABP (Garénaux et al., 2013).
Conclusion
The results presented here show for the first time that SE, the major pathogen associated with egg-linked food-borne outbreaks, is resistant to the enhanced iron-restriction imposed by the EW-lipocalin, Ex-FABP. This resistance is due to the ability of SE to produce and utilize the siderophore, Sal. This Sal-resistance property would be expected to contribute to survival of SE in the iron-restricted conditions of EW and would thus be likely to support the capacity of SE to infect eggs (Clavijo et al., 2006; Gantois et al., 2009; Vylder et al., 2013; Baron et al., 2016). Furthermore, the results provide the first immuno-quantification of the three EW lipocalins in eggs and reveal that Ex-FABP is present at concentrations (5.1 μM) sufficient to inhibit iron-restricted, Ent-dependent growth. In contrast, neither Cal-γ nor α-1-ovoglycoprotein influenced the growth of SE under the conditions tested here, indicating that these two EW lipocalins do not bind the siderophores produced by SE and do not contribute directly to iron restriction; the ITC data fully support the lack of Ent or Sal binding activity by these lipocalins. ITC also showed that both Ex-FABP and LCN2 bind Ent (but not Sal) with high affinity and with a preference for Fe-Ent (Kd of 5.4 and 11.8 nM, respectively) over apo-Ent (Kd of 86.2 and 58.4 nM, respectively). These binding patterns are consistent with previous work (Goetz et al., 2002; Abergel et al., 2006; Fischbach et al., 2006; Bao et al., 2010; Correnti et al., 2011; Li et al., 2015) although the affinity value ranges reported here and elsewhere show wide variation, which largely correlate with the methodology (ITC or TFQ) employed. A key finding of the results provided here is that the natural concentration of Ex-FABP in EW appears sufficient to play a biological role in limiting bacterial growth through sequestration of the siderophore, Ent. However, this effect is overcome by SE through its ability to deploy a Sal as second, “stealth” siderophore.
Data Availability Statement
All datasets generated for this study are included in the article/Supplementary Material.
Author Contributions
LJ, FB, SB, SJ, and SA designed the experiments. LJ and CF performed the experiments and analyzed the corresponding results. LJ wrote the manuscript with FB, SB, CG-D, FN, MG, KK, SJ, and SA.
Funding
This work was supported by the Strategic Fund of the University of Reading (United Kingdom) and the Regional Council of Brittany (Allocation de Recherche Doctorale, ARED; France).
Conflict of Interest
The authors declare that the research was conducted in the absence of any commercial or financial relationships that could be construed as a potential conflict of interest.
Acknowledgments
We would like to thank Nicholas Michael working at the University of Reading BioCentre for achieving ESI-MS; the staff of the University of Reading (Jordan Kirby); and the STLO for their guidance regarding the ITC (Pascaline Hamon, Thomas Croguennec, Said Bouhallab). We also would like to thank Hirotada Mori and Barry Wanner for strain and plasmid provision.
Supplementary Material
The Supplementary Material for this article can be found online at: https://www.frontiersin.org/articles/10.3389/fmicb.2020.00913/full#supplementary-material
Footnotes
References
Abergel, R. J., Moore, E. G., Strong, R. K., and Raymond, K. N. (2006). Microbial evasion of the immune system: structural modifications of enterobactin impair siderocalin recognition. J. Am. Chem. Soc. 128, 10998–10999. doi: 10.1021/ja062476+
Alderton, G., Ward, W. H., and Fevold, H. L. (1946). Identification of the bacteria-inhibiting iron-binding protein of egg-white as conalbumin. Arch. Biochem. Biophys. 11, 9–13.
Andrews, S. C., Robinson, A. K., and Rodríguez-Quiñones, F. (2003). Bacterial iron homeostasis. FEMS Microbiol. Rev. 27, 215–237.
Bao, G., Clifton, M., Hoette, T. M., Mori, K., Deng, S. X., Qiu, A., et al. (2010). Iron traffics in circulation bound to a siderocalin (Ngal)-catechol complex. Nat. Chem. Biol. 6, 602–609. doi: 10.1038/nchembio.402
Baron, F., Gautier, M., and Brulé, G. (1997). Factors involved in the inhibition of growth of Salmonella enteritidis in liquid egg white. J. Food Prot. 60, 1318–1323. doi: 10.4315/0362-028X-60.11.1318
Baron, F., Nau, F., Guérin-Dubiard, C., Bonnassie, S., Gautier, M., Andrews, S. C., et al. (2016). Egg white versus Salmonella Enteritidis! A harsh medium meets a resilient pathogen. Food Microbiol. 53, 82–93. doi: 10.1016/j.fm.2015.09.009
Bäumler, A. J., Norris, T. L., Lasco, T., Voigt, W., Reissbrodt, R., Rabsch, W., et al. (1998). IroN, a novel outer membrane siderophore receptor characteristic of Salmonella enterica. J. Bacteriol. 180, 1446–1453.
Bister, B., Bischoff, D., Nicholson, G. J., Valdebenito, M., Schneider, K., Winkelmann, G., et al. (2004). The structure of salmochelins: C-glucosylated enterobactins of Salmonella enterica. Biometals 17, 471–481. doi: 10.1023/b:biom.0000029432.69418.6a
Bullen, J. J., Rogers, H. J., and Griffiths, E. (1978). Role of iron in bacterial infection. Curr. Top. Microbiol. Immunol. 80, 1–35.
Carrano, C. J., and Raymond, K. N. (1979). Ferric ion sequestering agents 2. Kinetics and mechanism of iron removal from transferrin by enterobactin and synthetic tricatechols. J. Am. Chem. Soc. 101, 5401–5404.
Chart, H., and Rowe, B. (1993). Iron restriction and the growth of Salmonella enteritidis. Epidemiol. Infect. 110, 41–47.
Cherepanov, P. P., and Wackernagel, W. (1995). Gene disruption in Escherichia coli: TcR and KmR cassettes with the option of Flp-catalyzed excision of the antibiotic-resistance determinant. Gene 158, 9–14. doi: 10.1016/0378-1119(95)00193-a
Clavijo, R. I., Loui, C., Andersen, G. L., Riley, L. W., and Lu, S. (2006). Identification of genes associated with survival of Salmonella enterica serovar Enteritidis in chicken egg albumen. Appl. Environ. Microbiol. 72, 1055–1064. doi: 10.1128/AEM.72.2.1055-1064.2006
Clifton, M. C., Corrent, C., and Strong, R. K. (2009). Siderocalins: siderophore-binding proteins of the innate immune system. Biometals 22, 557–564. doi: 10.1007/s10534-009-9207-6
Correnti, C., Clifton, M. C., Abergel, R. J., Allred, B., Hoette, T. M., Ruiz, M., et al. (2011). Galline Ex-FABP is an antibacterial siderocalin and a lysophosphatidic acid sensor functioning through dual ligand specificities. Structure 19, 1796–1806. doi: 10.1016/j.str.2011.09.019
Crouch, M. V., Castor, M., Karlinsey, J. E., Kalhorn, T., and Fang, F. C. (2008). Biosynthesis and IroC-dependent export of the siderophore salmochelin are essential for virulence of Salmonella enterica serovar Typhimurium. Mol. Microbiol. 67, 971–998. doi: 10.1111/j.1365-2958.2007.06089.x
D’Ambrosio, C., Arena, S., Scaloni, A., Guerrier, L., Boschetti, E., Mendieta, M. E., et al. (2008). Exploring the chicken egg white proteome with combinatorial peptide ligand libraries. J. Proteome Res. 7, 3461–3474. doi: 10.1021/pr800193y
Datsenko, K. A., and Wanner, B. L. (2000). One-step inactivation of chromosomal genes in Escherichia coli K-12 using PCR products. Proc. Natl. Acad. Sci. U.S.A. 97, 6640–6645. doi: 10.1073/pnas.120163297
ECDC and EFSA (2017). Multicountry Outbreak of Salmonella Enteritidis Phage Type 8, MLVA type 2-9-7-3-2 and 2-9-6-3-2 Infections. Stockholm and Parma: ECDC and EFSA.
Efsa Panel on Biological Hazards. (2014). Scientific Opinion on the public health risks of table eggs due to deterioration and development of pathogens. EFSA J. 12, 1–147.
Efsa Panel on Biological Hazards. (2019). Scientific opinion on the Salmonella control in poultry flocks and its public health impact. EFSA J. 17, 1–155.
Fischbach, M. A., Lin, H., Zhou, L., Yu, Y., Abergel, R. J., Liu, D. R., et al. (2006). The pathogen-associated iroA gene cluster mediates bacterial evasion of lipocalin 2. Proc. Natl. Acad. Sci. U.S.A. 103, 16502–16507. doi: 10.1073/pnas.0604636103
Ford, S., Cooper, R. A., Evans, R. W., Hider, R. C., and Williams, P. H. (1988). Domain preference in iron removal from human transferrin by the bacterial siderophores aerobactin and enterochelin. Eur. J. Biochem. 178, 477–481. doi: 10.1111/j.1432-1033.1988.tb14473.x
Gantois, I., Ducatelle, R., Pasmans, F., Haesebrouck, F., Gast, R., Humphrey, T. J., et al. (2009). Mechanisms of egg contamination by Salmonella Enteritidis: review article. FEMS Microbiol. Rev. 33, 718–738.
Garénaux, A., Houle, S., Folch, B., Dallaire, G., Truesdell, M., Lépine, F., et al. (2013). Avian lipocalin expression in chickens following Escherichia coli infection and inhibition of avian pathogenic Escherichia coli growth by Ex-FABP. Vet. Immunol. Immunopathol. 152, 156–167. doi: 10.1016/j.vetimm.2012.09.018
Garibaldi, J. A. (1970). Role of microbial iron transport compounds in bacterial spoilage of eggs. Appl. Microbiol. 20, 558–560.
Gast, R. K., and Beard, C. W. (1990). Production of Salmonella enteritis-contaminated eggs by experimentally infected hens. Avian. Dis. 34, 438–446.
Gehring, A. M., Mori, I., and Walsh, C. T. (1998). Reconstitution and characterization of the Escherichia coli enterobactin synthetase from EntB, EntE, and EntF. Biochemistry 37, 2648–2659. doi: 10.1021/bi9726584
Goetz, D. H., Holmes, M. A., Borregaard, N., Bluhm, M. E., Raymond, K. N., and Strong, R. K. (2002). The neutrophil lipocalin NGAL is a bacteriostatic agent that interferes with siderophore-mediated iron acquisition. Mol. Cell. 10, 1033–1043. doi: 10.1016/s1097-2765(02)00708-6
Guérin-Dubiard, C., Pasco, M., Mollé, D., Désert, C., Croguennec, T., and Nau, F. M. (2006). Proteomic analysis of hen egg white. J. Agric. Food Chem. 54, 3901–3910. doi: 10.3382/ps.2012-02986
Haginaka, J., Seyama, C., and Kanasugi, N. (1995). Ovoglycoprotein-Bonded HPLC Stationary Phases for Chiral Recognition. Anal. Chem. 67, 2539–2547. doi: 10.1021/ac00111a008
Hantke, K., Nicholson, G., Rabsch, W., and Winkelmann, G. (2003). Salmochelins, siderophores of Salmonella enterica and uropathogenic Escherichia coli strains, are recognized by the outer membrane receptor IroN. Proc. Natl. Acad. Sci. U.S.A. 100, 3677–3682. doi: 10.1073/pnas.0737682100
Holmes, M. A., Paulsene, W., Jide, X., Ratledge, C., and Strong, R. K. (2005). Siderocalin (Lcn 2) also binds carboxymycobactins, potentially defending against mycobacterial infections through iron sequestration. Structure 13, 29–41. doi: 10.1016/j.str.2004.10.009
Humphrey, T. J., Whitehead, A., Gawler, A. H. L., and Henley, A. (1991). Numbers of Salmonella enteritidis in the contents of naturally contaminated hens’ eggs. Epidemiol. Infect. 106, 489–496. doi: 10.1017/s0950268800067546
Johnstone, T. C., and Nolan, E. M. (2015). Beyond iron: non-classical biological functions of bacterial siderophores. Dalton trans. 44, 6320–6339. doi: 10.1039/c4dt03559c
Julien, L. A., Baron, F., Bonnassie, S., Nau, F., Guérin, C., Jan, S., et al. (2019). The anti-bacterial iron-restriction defence mechanisms of egg white; the potential role of three lipocalins in resistance against Salmonella. Biometals 32, 453–467. doi: 10.1007/s10534-019-00180-w
Keller, L. H., Benson, C. E., Krotec, K., and Eckroade, R. J. (1995). Salmonella Enteritidis colonization of the reproductive tract and forming and freshly laid eggs of chickens. Infect. Immun. 63, 2443–2449.
Kelly, A. U., McSorley, S. T., Patel, P., and Talwar, D. (2017). Interpreting iron studies. BMJ 357, 1–6.
Kovacs-Nolan, J., Phillips, M., and Mine, Y. (2005). Advances in the value of eggs and egg components for human health. J. Agric. Food Chem. 53, 8421–8431. doi: 10.1021/jf050964f
Li, W., Cui, T., Hu, L., Wang, Z., Li, Z., and He, Z. G. (2015). Cyclic diguanylate monophosphate directly binds to human siderocalin and inhibits its antibacterial activity. Nat. Commun. 6, 1–9. doi: 10.1038/ncomms9330
Liu, Y., Zhang, Q., Hu, M., Yu, K., Fu, J., Zhou, F., et al. (2015). Proteomic analyses of intracellular Salmonella enterica serovar typhimurium reveal extensive bacterial adaptations to infected host epithelial cells. Infect. Immun. 83, 2897–2906. doi: 10.1128/IAI.02882-14
Lock, J. L., and Board, R. G. (1992). Persistence of contamination of hens’ egg albumen in vitro with Salmonella serotypes. Epidemiol. Infect. 108, 389–396. doi: 10.1017/s095026880004989x
Louden, B. C., Haarmann, D., and Lynne, A. M. (2011). Use of blue agar CAS assay for siderophore detection. J. Microbiol. Biol. Educ. 12, 51–53. doi: 10.1128/jmbe.v12i1.249
Luo, M., Lin, H., Fischbach, M. A., Liu, D. R., Walsh, C. T., and Groves, J. T. (2006). Enzymatic tailoring of enterobactin alters membrane partitioning and iron acquisition. ACS Chem. Biol. 1, 29–32. doi: 10.1021/cb0500034
Mahmood, T., and Yang, P. C. (2012). Western blot: technique, theory, and trouble shooting. N. Am. J. Med. Sci. 4, 429–434. doi: 10.4103/1947-2714.128482
Mann, K., and Mann, M. (2011). In-depth analysis of the chicken egg white proteome using an LTQ Orbitrap Velos. Proteome Sci. 9, 1–6. doi: 10.1186/1477-5956-9-7
Müller, S. I., Valdebenito, M., and Hantke, K. (2009). Salmochelin, the long-overlooked catecholate siderophore of Salmonella. BioMetals 22, 691–695. doi: 10.1007/s10534-009-9217-4
Pollack, J. R., Ames, B. N., and Neilands, J. B. (1970). Iron transport in Salmonella typhimurium: mutants blocked in the biosynthesis of enterobactin. J. Bacteriol. 104, 635–639.
Raffatellu, M., George, M. D., Akiyama, Y., Hornsby, M. J., Nuccio, S. P., Paixao, T. A., et al. (2009). Lipocalin-2 resistance confers an advantage to Salmonella enterica serotype typhimurium for growth and survival in the inflamed intestine. Cell Host Microbe 5, 476–486. doi: 10.1016/j.chom.2009.03.011
Sambrook, J., and Russell, D. W. (2001). Molecular Cloning: A Laboratory Manual. Cold Spring Harbor: Cold Spring Harbor Laboratory Press.
Schade, A., and Caroline, L. (1944). Raw hen egg white and therole of iron in growth inhibition of Shigella dysenteriae, Staphylococcus aureus, Escherichia coli and Saccharomyces cerevisiae. Science 100, 14–15. doi: 10.1126/science.100.2584.14
Valdebenito, M., Müller, S. I., and Hantke, K. (2007). Special conditions allow binding of the siderophore salmochelin to siderocalin (NGAL-lipocalin). FEMS Microbiol. Lett. 277, 182–187. doi: 10.1111/j.1574-6968.2007.00956.x
Vylder, J., De, Raspoet, R., Dewulf, J., Haesebrouck, F., Ducatelle, R., et al. (2013). Salmonella Enteritidis is superior in egg white survival compared with other Salmonella serotypes. Poult. Sci. Assoc. Inc. 92, 842–845. doi: 10.3382/ps.2012-02668
Watts, R. E., Totsika, M., Challinor, V. L., Mabbett, A. N., Ulett, G. C., Voss, J. J., et al. (2012). Contribution of siderophore systems to growth and urinary tract colonization of asymptomatic bacteriuria Escherichia coli. Infect. Immun. 80, 333–344. doi: 10.1128/IAI.05594-11
Keywords: salmochelin, enterobactin, Ex-FABP, Cal-γ, α-1-ovoglycoprotein, egg white, Salmonella Enteritidis
Citation: Julien LA, Fau C, Baron F, Bonnassie S, Guérin-Dubiard C, Nau F, Gautier M, Karatzas KA, Jan S and Andrews SCGuérin-Dubiard C, Nau F, Gautier M, Karatzas KA, Jan S and Andrews SC (2020) The Three Lipocalins of Egg-White: Only Ex-FABP Inhibits Siderophore-Dependent Iron Sequestration by Salmonella Enteritidis. Front. Microbiol. 11:913. doi: 10.3389/fmicb.2020.00913
Received: 14 February 2020; Accepted: 17 April 2020;
Published: 15 May 2020.
Edited by:
Chrysoula C. Tassou, Hellenic Agricultural Organization – ELGO, GreeceReviewed by:
Charles Martin Dozois, Institut National de la Recherche Scientifique (INRS), CanadaSean Doyle, Maynooth University, Ireland
Copyright © 2020 Julien, Fau, Baron, Bonnassie, Guérin-Dubiard, Nau, Gautier, Karatzas, Jan and Andrews. This is an open-access article distributed under the terms of the Creative Commons Attribution License (CC BY). The use, distribution or reproduction in other forums is permitted, provided the original author(s) and the copyright owner(s) are credited and that the original publication in this journal is cited, in accordance with accepted academic practice. No use, distribution or reproduction is permitted which does not comply with these terms.
*Correspondence: Simon Colin Andrews, s.c.andrews@reading.ac.uk