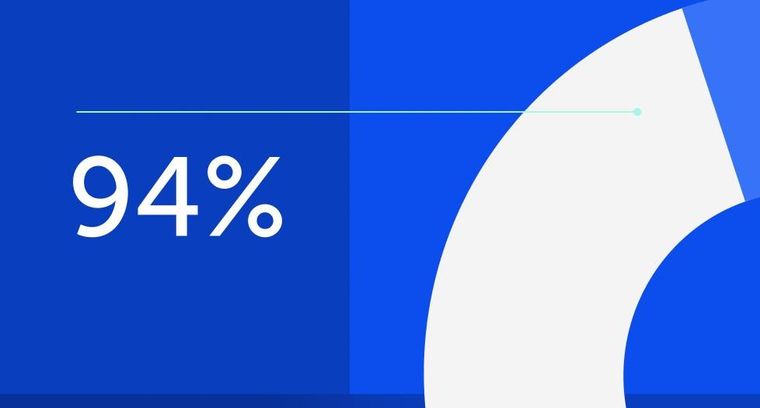
94% of researchers rate our articles as excellent or good
Learn more about the work of our research integrity team to safeguard the quality of each article we publish.
Find out more
ORIGINAL RESEARCH article
Front. Microbiol., 25 May 2020
Sec. Microbial Symbioses
Volume 11 - 2020 | https://doi.org/10.3389/fmicb.2020.00846
This article is part of the Research TopicNew Antimicrobial Peptides From Bacteria/Invertebrate Obligate Symbiotic AssociationsView all 11 articles
Spätzle (Spz) is a dimeric ligand that responds to the Gram-positive bacterial or fungal infection by binding Toll receptors to induce the secretion of antimicrobial peptides. However, whether the Toll-like signaling pathway mediates the innate immunity of Rhynchophorus ferrugineus to modulate the homeostasis of gut microbiota has not been determined. In this study, we found that a Spz homolog, RfSpätzle, is a secretory protein comprising a signal peptide and a conservative Spz domain. RT-qPCR analysis revealed that RfSpätzle was significantly induced to be expressed in the fat body and gut by the systemic and oral infection with pathogenic microbes. The expression levels of two antimicrobial peptide genes, RfColeoptericin and RfCecropin, were downregulated significantly by RfSpätzle knockdown, indicating that their secretion is under the regulation of the RfSpätzle-mediated signaling pathway. After being challenged by pathogenic microbes, the cumulative mortality rate of RfSpätzle-silenced individuals was drastically increased as compared to that of the controls. Further analysis indicated that these larvae possessed the diminished antibacterial activity. Moreover, RfSpätzle knockdown altered the relative abundance of gut bacteria at the phylum and family levels. Taken together, these findings suggest that RfSpätzle is involved in RPW immunity to confer protection and maintain the homeostasis of gut microbiota by mediating the production of antimicrobial peptides.
Spätzle (Spz), a dimeric ligand of the Toll receptor, binds the Toll receptor to initiate the secretion of antimicrobial peptides via activating the immune signaling pathway (Lemaitre and Hoffmann, 2007). In invertebrates, genomic studies have revealed that different invertebrate species have various numbers of Spz gene copies. For example, Drosophila melanogaster has six Spz isoforms (DmSpz1–6) (Parker et al., 2001), while Aedes aegypti (Shin et al., 2006), black tiger shrimp Peneaus monodon (Boonrawd et al., 2017), and white shrimp Litopenaeus vannamei (Wang et al., 2012) have three Spz proteins. However, only one Spz gene, MrSpz, was identified from the freshwater prawn Macrobrachium rosenbergii (Vaniksampanna et al., 2019). Furthermore, previous evidence indicated that BmSpz1 of Bombyx mori (Wang et al., 2007), MsSpz1 in Manduca sexta (An et al., 2010), and ApSpz in oak silkworm Antheraea pernyi (Sun et al., 2016) were markedly induced by microbial infection to activate their immune responses. In D. melanogaster, the Spz-mediated Toll pathway has been well determined to mediate the synthesis of antimicrobial peptides, such as drosomycin (Weber et al., 2003; Valanne et al., 2011; Stokes et al., 2015), and activate the cellular immunity (Hultmark, 2003).
Insects harbor diverse species of symbiotic microorganisms in their guts that strongly affect host physiological traits, including nutrition metabolism (Wong et al., 2014; Janssen and Kersten, 2015; Muhammad et al., 2017; Hebineza et al., 2019), detoxification (Engel and Moran, 2013), protection from natural enemies (Kim et al., 2015; Park et al., 2018), growth and development (Blatch et al., 2010; Wong et al., 2014; Hebineza et al., 2019), mating and foraging behavior (Ami et al., 2010), and gut homeostasis (Engel and Moran, 2013; Douglas, 2015). However, the exact mechanism by which these insect hosts modulate the intensity of gut immunity to maintain the homeostasis of gut microbiota is still poorly understood outside D. melanogaster. In D. melanogaster, it is well-known that gut epithelial cells can secrete antimicrobial peptides and reactive oxygen species (ROS), which provide colonization resistance to non-commensal bacteria (Ha et al., 2005; Ryu et al., 2008; Lee et al., 2017). The production of these two immune effectors is under the control of the IMD (immune deficiency) pathway and dual oxidase (DUOX) signaling system, respectively (Lemaitre and Hoffmann, 2007; Ryu et al., 2008; Lee et al., 2017; Lin et al., 2018). Moreover, the IMD pathway has been shown to control the excessive proliferation of residential gut microbiota, as the IMD mutant exhibited an increased gut bacterial load (Guo et al., 2014). Intestinal immunity is regulated to maintain the homeostasis of the gut microbiota. For instance, a number of recognition proteins, including PGRP-LB, PGRP-SC1a, PGRP-SC1b, and PGRP-SC2, negatively regulate the IMD signaling pathway due to their amidase activity, degrading gut bacteria-derived peptidoglycan to avoid the excessive production of immune effectors in gut epithelial cells (Bischoff et al., 2006; Zaidman-Rémy et al., 2006; Paredes et al., 2011; Guo et al., 2014; Dawadi et al., 2018; Maire et al., 2018, 2019). Additionally, negative regulation can also be achieved by the regulator PIRK, interacting with PGRP-LC, PGRP-LE, and Imd to prevent excessive activation of the IMD signaling pathway (Aggarwal and Silverman, 2008).
To the best of our knowledge, the interactions between the gut microbiota and host immune system in many insects are far from well understood. Red palm weevil (RPW), Rhynchophorus ferrugineus (Olivier) (Coleoptera: Dryophthoridae), is an immensely destructive pest for palm plants in China and other tropical countries (Ju et al., 2011; Shi et al., 2014; Al-Dosary et al., 2016). The RPW gut is colonized by a complex gut bacterial community that is involved in the degradation of plant polysaccharides to impact host nutrition metabolism (Jia et al., 2013; Tagliavia et al., 2014; Montagna et al., 2015; Muhammad et al., 2017; Hebineza et al., 2019). Interestingly, RPW also houses an intracellular symbiont, Nardonella, within a specialized organ, the bacteriome (Hosokawa et al., 2015). Nardonella provides its host with tyrosine, which is used for cuticle synthesis and hardening (Anbutsu et al., 2017). Recently, we found that RfPGRP-LB acts as a negative immunity regulator to inhibit the chronic activation of the immune response by degrading peptidoglycan (Dawadi et al., 2018). It has also been found that weevil pgrp-lb prevents endosymbiont-released immunological molecules from escaping the bacteriocytes to chronically activate host systemic immunity (Maire et al., 2019). Furthermore, an NF-κB-like transcription factor, RfRelish, has been demonstrated to mediate the intestinal immunity to modulate the homeostasis of the RPW gut microbiota (Xiao et al., 2019). However, the role of the Spz-mediated Toll-like pathway in RPW immunity has not been determined. In this study, a Spz homolog, RfSpätzle, was characterized and its role in modulating the composition of the RPW gut microbiota was determined. Our evidence indicated that the RfSpätzle-mediated Toll signaling pathway regulates the synthesis of antimicrobial peptides to confer the protection against microbial infection and modulates the proportion of RPW gut bacteria.
The RPW laboratory population was established and maintained by adults trapped in the Pingtan District (119°32′ E, 25°31′ N) of Fuzhou city, Fujian Province and Jinshan campus of Fujian Agriculture and Forestry University (119°30′ E, 26°08′ N). RPW individuals were fed with sugarcane stems in an incubator (Saifu ZRX-260, Ninbo Experimental Instrument Co. Ltd., China) at 27 ± 1°C, 75 ± 5% relative humidity, and a photoperiod of 24 h dark for larvae and 12 h light/12 h dark for adults (Muhammad et al., 2017).
The fourth instar RPW larvae were dissected in a sterilized dish plate containing 2 ml of phosphate buffered saline (PBS, NaCl 137 mM, KCl 2.7 mM, Na2HPO4 10 mM, K2HPO4 2 mM, pH 7.2). Three guts were pooled as replicates and homogenized with a tissue lyser (Ningbo Scientz BioTech. Company Limited, China). Total RNA was extracted with TRIzol Reagent (Invitrogen, Carlsbad, CA, United States) following the manufacturers’ instructions. RNA concentration and integrity were determined with NanoDrop2000 (Thermo Fisher Scientific Inc., Waltham, MA) and 1% agarose gel electrophoresis, respectively. The cDNA template was prepared with the TransScript® All-in-One First Strand cDNA Synthesis Kit (Takara Bio Inc., Dalian, China). The core sequence of RfSpätzle was cloned with a pair of specific primers (Table 1). The 25-μl PCR mix consisted of 1 μl of cDNA, 12.5 μl of 2 × TaqPCR mix, 1 μl each of forward and reverse primer (10 μM) and 9.5 μl of RNase-free water. Thermal conditions were set as follows: the initial denaturation at 95°C for 2 min followed by 35 cycles at 95°C for 30 s, 60°C for 30 s, 72°C for 1 min, and a final extension at 68°C for 7 min. PCR products were confirmed by 1% agarose gel electrophoresis. The full cDNA length of RfSpätzle was obtained through rapid amplification of cDNA ends (RACE), which was completed by nested PCR with a SMARTer® RACE kit (Takara Bio Inc., Dalian, China) according to manufacturer guidelines. PCR products were purified with an EasyPure® Quick Gel Extraction Kit (TransGen Biotech, Beijing, China). Subsequently, the purified PCR products were ligated into the pEASY® -T1 cloning vector (TransGen Biotech, Beijing, China). The full cDNA sequence of RfSpätzle was analyzed with ExPASY tool1 to determine its open reading frame (ORF). Conservative domains of RfSpätzle were predicted with running the SMART program2.
BLASTX3 was run to find the annotated Spz sequences from other insect species for the following multiple alignments. These Spz sequences were retrieved from the NCBI database. Multiple sequence alignments were performed with the Clustal Omega Program4. Phylogenetic analysis was completed with MEGA 5.05.
The healthy fourth instar larvae were dissected for collecting various tissues, including head, epidermis, hemolymph, fat body and gut, to determine the expression profile of RfSpätzle. Three larvae were dissected as a replicate and each treatment comprised four replicates. Total RNA was extracted from the tissues with TRIzol Reagent (Invitrogen, Carlsbad, CA, United States). Total RNA (1 μg) was used to synthesize cDNA with a Thermo Fisher Scientific Verso cDNA Kit (Thermo Fisher Scientific, United States). To detect the transcript abundance of RfSpätzle, RT-qPCR was performed with FastStart Universal SYBR Green Master (Roche, United States) with a 20-μl reaction system, including 1 μl of cDNA and 125 nM specific primers. Rfβ-Actin was employed as the internal reference gene. The qPCR reactions were completed with the following thermal cycling conditions: denaturation at 95°C for 10 min, 40 cycles of 95°C for 15 s, amplification at 60°C for 1 min, and with a dissociation step. The 2–ΔΔCt method was used to calculate the relative expression level of our target genes.
To investigate the potential role of RfSpätzle in RPW immunity, its transcriptional response to microbial infection was examined. Systemic and oral infections were established with injecting or feeding Gram-positive bacteria Staphylococcus aureus and Gram-negative bacteria Escherichia coli DH5α as described by Dawadi et al. (2018). Bacillus thuringiensis is a biological control agent against RPW larvae (Pu et al., 2017). Beauveria bassiana was also used as fungal challenge and cultured on potato dextrose agar (PDA) for 2 weeks at 25°C. The conidial concentration of B. bassiana (1 × 108 spores/ml) was determined with a Neubauer hemocytometer as described by Hussain et al. (2016). Fat bodies and guts of the microbe-challenged larvae were obtained at different time points (6, 12, and 24 h). To detect the transcript changes of RfSpätzle by microbial challenge, RT-qPCR was performed as described above.
To investigate the function of RfSpätzle in RPW immunity, the RNAi technique was used to silence this target gene. Following the manufacturer’s protocols, the MEGA script® RNAi Kit (Thermo Fisher Scientific, United States) was used to synthesize RfSpätzle and eGFP dsRNA. In this study, eGFP dsRNA was employed as the control for our RNAi experiments. dsRNA (1 μg) was injected into the body cavity of fourth instar larvae. According to our previous investigations (Dawadi et al., 2018; Xiao et al., 2019), fat bodies, and guts were dissected 48 h after dsRNA delivery to verify RNAi efficiency by RT-qPCR.
To evaluate the effect of RfSpätzle knockdown on the survival rate of RPW larvae, 48 h after the delivery of dsRNA, RfSpätzle-silenced individuals were challenged with entomopathogenic bacteria, B. thuringiensis strain HA (Pu et al., 2017). Five microliters of bacterial suspension (1.0 × 108 CFU/ml) or the same volume of sterile PBS (as control) was injected into the hemocoel of the fourth instar larvae (n = 30) and the survival rate was monitored every 6 h. The effect of RfSpätzle knockdown on the survival rate was determined with the Kaplan–Meier Log Rank survival test (IBM SPSS Statistics 22.0).
To reveal the impact of RfSpätzle knockdown on gut bacterial load and composition, guts were aseptically pulled out from RPW larvae at 48 h after dsRNA delivery and homogenized. Three larvae were dissected as a replicate and each treatment comprised at least three replicates. After serial dilution, gut homogenate was spread on LB agar plates and incubated for 16 h at 37°C as described by Dawadi et al. (2018). The number of colony-forming units (CFUs) of gut bacteria from the treated insects was counted. Furthermore, gut homogenate was processed for extracting total bacterial genomic DNA as described by Muhammad et al. (2017). Additionally, a blank DNA extraction was conducted as explained above but without any gut samples in each replicate. The bacterial 16S rRNA hypervariable region (V3–V4) was amplified with gene-specific primers 338F 5′-ACTCCTACGGGAGGCAGCAG-3′ and 5′-GGACTACHVGGGTWTCTAAT-3′. In the negative control, an equal volume of sterilized ddH2O was used as the template to check for laboratory contamination. No target PCR products were detected by 1% agarose gel electrophoresis in any negative controls. The sequencing was completed with the Majorbio I-Sanger (China) Illumina MiSeq platform. The OTUs (operational taxonomic units) were clustered at the similarity threshold of 97% with the Usearch program (version 7.05). Taxonomic analysis was performed with RDP Classifier6 against the Bacteria database (Silva: Release1287 and Greengene: Release 13.58) at the confidence threshold of 0.7. The sequencing data were processed and analyzed with the methods as described by Muhammad et al. (2017) and Dawadi et al. (2018). Briefly, beta diversity analysis of RPW gut bacteria was completed through principal coordinate analysis (PCoA) and principal component analysis (PCA). ANOSIM (analysis of similarity) and DEseq analysis were employed to detect the differences in the gut bacterial community between the two groups.
The full-length cDNA sequence of RfSpätzle is 1865 bp long with a 1270-bp ORF that encodes a putative protein of 353 amino acids (Figure 1A), comprising an N-terminal signal peptide and a Spz domain of 97 amino acids at the C-terminus (Figure 1B). The presence of the Spz domain indicated that RfSpätzle is a member of the Spz family, mediating the Toll signaling pathway by binding the Toll receptors. The calculated mass and isoelectric point of this mature proSpätzle are 40,122.18 Da and 8.81. The putative activation cleavage site is located after VRSKR246 in pro-RfSpätzle, implying that pro-RfSpätzle might be activated by a clip-domain serine proteinase with trypsin-like specificity via limited proteolysis immediately after Arg246 (Jang et al., 2006; Wang et al., 2007; An et al., 2010). Interestingly, some residues, including six cysteines, two prolines, and one glutamine, are conserved in four insect species (Figure 2A). It has been confirmed that these six conserved cysteines are crucial to disulfide formation and fold stability of the neurotrophin-like cysteine knot (CK) structural motif (Jang et al., 2006; An et al., 2010). Moreover, most Spz members have an orphan cysteine before Cys5, which can establish an intermolecular linkage with its counterpart in another subunit to form a disulfide-linked homodimer (Hoffmann et al., 2008; Wang and Zhu, 2009; An et al., 2010). Furthermore, when 19 Spz sequences were taken into consideration for phylogenetic analysis, these sequences were split into three distinct clusters (Spz3, Spz4, and Spz5, Figure 2B). The inclusion of RfSpätzle in the same branch as Drosophila Spz-5, exhibiting a bootstrap value of 100, indicates that RfSpätzle is an ortholog of Drosophila Spz-5.
Figure 1. Full-length cDNA nucleotide and deduced amino acid sequence of RfSpätzle (A) and schematic representation of the conserved functional domains in its deduced polypeptide sequence (B). The start and stop codons are underlined and shown in bold letters. The signal peptide is shaded in green, and the conserved domain is dark gray. In the schematic representation, the signal peptide and Spätzle (Spz) domain are shown with the red and dark gray rectangles, respectively.
Figure 2. Multiple alignments (A) and phylogenetic analysis (B) of RfSpätzle with other Spz proteins. The arrow indicates the predicted cleavage site in RfSpätzle. The maximum likelihood (ML) method of MEGA 5.05 was employed to construct the phylogenetic tree of Spz sequences with 5000 bootstrap replicates. The following Spz were retrieved for our phylogenetic analysis: Bm-Spaetzle 5 [Bombyx mori AMR08002.1], Sl-Spaetzle 5 [Spodoptera litura XP_022816314.1], Zn-Spaetzle 3 [Zootermopsis nevadensis XP_021922319.1], Dm-Spaetzle 5 [Drosophila melanogaster NP_647753.1], Ds-Spaetzle 5 [Drosophila serrata XP_020809778.1], Do-Spaetzle 5 [Drosophila obscura XP_022208379.1], Dw-Spaetzle 5 [Drosophila willistoni XP_002062599.1], Dp-Spaetzle 3 [Dendroctonus ponderosae XP_019758543.1], Ag-Spaetzle 3 [Anoplophora glabripennis XP_018561823.2], Tc-Spaetzle 3 [Tribolium castaneum NP_001153625.1], Ap-Spaetzle 3 [Agrilus planipennis XP_025831835.1], Dp-Spaetzle 4 [Dendroctonus ponderosae XP_019771425.1], At-Spaetzle 4 [Aethina tumida XP_019880911.1], Ap-Spaetzle 4 [Agrilus planipennis XP_018325475.1], Ag-Spaetzle 4 [Anoplophora glabripennis XP_018575280.1], Dp spaetzle 5 [Dendroctonus ponderosae XP_019764273.1], Ag-Spaetzle 5 [Anoplophora glabripennis XP_018568892.1], and At-Spaetzle 5 [Aethina tumida XP_019879590.1].
RT-qPCR analysis showed that RfSpätzle was constitutively expressed in the tested tissues at significantly different levels (ANOVA: F5,18 = 45.11, P < 0.001) (Figure 3). The expression level of RfSpätzle in the hemolymph and gut was 8.38- and 5.00-fold higher than that in the epidermis. RfSpätzle was significantly induced in the fat body and gut upon the systemic and oral pathogenic challenge of S. aureus, E. coli, and B. bassiana as compared to the controls (Figures 4, 5). These results suggest that RfSpätzle might mediate the systemic and gut immune responses to fight against microbial intruders.
Figure 3. Expression level of RfSpätzle in various tissues of healthy RPW larvae was quantified by RT-qPCR. The expression level of RfSpätzle was normalized to the Rfβ-actin gene. Different letters represent the significance, which was determined by Tukey’s HSD (Honest Significant Difference) test at P < 0.05. The data are shown as the mean ± SD of four independent replicates.
Figure 4. Transcriptional response of RfSpätzle in fat body (A) and gut (B) of RPW larvae after the systemic challenge of microbial pathogens at different time points. The expression level of RfSpätzle was normalized to the Rfβ-actin gene. Uppercase letters indicate significant differences across all pathogen-challenged samples at the same point, while the lowercase letters represent the significance of the same treatment across different time points. The significance was determined by Tukey’s HSD test at P < 0.05. The data are shown as the mean ± SD of three independent replicates.
Figure 5. Transcriptional response of RfSpätzle in fat body (A) and guts (B) of RPW larvae at different time points after being orally infected by S. aureus and E. coli. The expression level of RfSpätzle was normalized to the Rfβ-actin gene. Uppercase letters indicate significant differences across the different challenged samples at the same time point, while the lowercase letters represent the significance of the same treatment across different time points. The significance was detected by Tukey’s HSD test at P < 0.05. The data are shown as the mean ± SD of three independent replicates.
Compared to the controls, the expression levels of RfSpätzle in the fat body and gut were significantly reduced by 83.23 and 69.26% at 48 h after dsRNA injection (t test for fat body: t = −8.95, df = 6, P < 0.001; gut: t = −2.92, df = 6, P < 0.05) (Figure 6). After being challenged by B. thuringiensis, RfSpätzle-silenced individuals (dsSPZ) succumbed significantly faster than their counterparts (P < 0.05, Figure 7). Further investigations revealed that RfSpätzle silencing led to the significant downregulation of RfColeoptericin (t test for fat body: t = −4.18, df = 6, P = 0.006; gut: t = −2.77, df = 6, P < 0.05) and RfCecropin (t test for fat body: t = −3.34, df = 6, P < 0.05; gut: t = −2.83, df = 6, P < 0.05) in fat body and gut, but the expression level of RfDefensin (t test for fat body: t = −8.95, df = 6, P = 0.06; gut: t = −1.55, df = 6, P = 0.17) and RfAttacin (t test for fat body: t = −1.05, df = 6, P = 0.33; gut: t = −2.92, df = 6, P = 0.70) was not affected by RfSpätzle knockdown (Figures 8A,B). These findings suggested that RfSpätzle mediates the expression of these two antimicrobial peptide genes (RfColeoptericin and RfCecropin) to confer RPW protection against the pathogen invasion.
Figure 6. Verification of the RfSpätzle RNAi efficiency in the fat body and gut of the fourth instar RPW larvae 48 h after dsRNA injection. The data are shown as the mean ± SD of four independent replicates. The significant differences were determined by an independent sample t test (*P < 0.05 and ***P < 0.001).
Figure 7. Survival rate of RfSpätzle-silenced individuals after being challenged by the pathogenic bacteria Bacillus thuringiensis strain HA. Survival analysis was performed with Kaplan-Meir survival log rank (Mantel-Cox) test (*P < 0.05). The experiment was repeated three times with 30 fourth instar larvae from each cohort.
Figure 8. Effects of RfSpätzle knockdown on the transcript abundance of four antimicrobial peptide genes, RfColeoptericin, RfDefensin, RfAttacin, and RfCecropin in the fat body (A) and gut (B) of the fourth instar RPW larvae 48 h after dsRNA delivery. The data are shown as the mean ± SD of four independent replicates. The significant differences were detected with an independent sample t test (ns, non-significant, P > 0.05, *P < 0.05, and **P < 0.01).
The role of the RfSpätzle-mediated Toll-like pathway in regulating the composition and proportion of RPW gut bacteria was determined. Comparative analysis of the gut bacterial load showed a numerically higher number of CFUs in the RfSpätzle-silenced individuals although no significance was detected (t test: t = −1.22, df = 16, P = 0.24) (Figure 9). Bacterial 16S rRNA-based high-throughput sequencing yielded 431,163 reads. At the 97% similarity threshold, these reads were binned into 414 OTUs, which contained 243 OTUs from the dseGFP group and 171 OTUs from the dsSPZ group. Seventy OTUs were shared between the two groups (Supplementary Figure S1). Diversity analysis revealed that the community diversity (Shannon and Simpson) and species richness (ACE, Chao, and Sobs) did not vary significantly between the two groups (Table 2 and Supplementary Figure S2). Proteobacteria and Tenericutes represented the bulk (95%) of the RPW gut microbiota, while the relative abundance of Bacteroidetes and Firmicutes was less than 5%. At the family level, the microbiota was dominated (>98%) by Enterobacteriaceae, Entomoplasmataceae, Pseudomonadaceae, Acetobacteraceae, Porphyromonadaceae, Spiroplasmataceae, and Enterococcaceae. ANOSIM analysis revealed that no significance was determined in the RPW gut bacterial community between the two groups (P = 0.75). However, the relative abundance of gut bacteria at different taxonomic levels was altered by RfSpätzle silencing. For example, the percentage of Proteobacteria (t test: t = 4.12, df = 4, P < 0.05) in RfSpätzle-silenced RPW larvae was decreased by 20% compared to that of controls, while the relative abundance of Tenericutes (t test: t = −5.27, df = 4, P < 0.05) was increased to 23.86% by RfSpätzle knockdown (Figure 10A). Similarly, the relative abundance of Acetobacteraceae (t test: t = 3.84, df = 4, P < 0.05) was significantly less than that of controls. However, Entomoplasmataceae (t test: t = −4.71, df = 4, P < 0.05) represented a higher percentage of RfSpätzle-silenced RPW larvae (Figure 10B). At the OTU level, DEseq analysis revealed that the abundance of OTU306 (Cellulomonadaceae, G+), OTU279 (Gallionellaceae, G–), and OTU283 (Ferriphaselus, G–) was significantly increased while that of OTU200 (Acetobacterium, G+) was decreased by RfSpätzle silencing (Supplementary Table S1). Collectively, these data indicated that RfSpätzle knockdown can cause some significant changes in the proportion of RPW gut bacteria, suggesting that the Spz -mediated Toll-like pathway is involved in modulating the homeostasis of the RPW gut microbiota.
Table 2. Community diversity and richness of microbiota associated with the guts of control (dseGFP) and Spz knockdown (dsSPZ) RPW individuals.
Figure 10. Influence of RfSpätzle silencing on the relative abundance of gut bacteria at the phylum (A) and family (B) levels. The data are shown as the mean ± SD of three independent replicates. The significant differences were detected with an independent sample t test (ns, non-significant, P > 0.05, *P < 0.05, and **P < 0.01).
Recently, the immune responses of RPW larvae to some biocontrol agents, including the entomopathogenic nematode Steinernema carpocapsae (Mastore et al., 2014), bacteria (Shi et al., 2014), and fungi (Hussain et al., 2016), have been investigated. However, the underlying molecular mechanisms conferring RPW larvae to ward off the microbial pathogens have not been fully elucidated. In this work, a homolog of D. melanogaster Spz, RfSpätzle, was first cloned and characterized from R. ferrugineus. RfSpätzle encodes a protein that consists of two typical conserved functional domains, a signal peptide and a Spz domain. Multiple sequence comparisons showed that a putative activation cleavage site of RfSpätzle is directly after R246, suggesting that an activating proteinase could cleave pro-RfSpätzle after this specific Arg to release the activated form of RfSpätzle. Interestingly, seven Cys residues, which are found in nearly all known Spz cysteine-knot domains, were also found in the C-terminal active cystine-knot domain of RfSpätzle. These data suggested that RfSpätzle is a secretory cysteine-knot protein. Moreover, the conserved features of this protein implied that the RfSpätzle homodimer can be formed via intermolecular Cys-Cys disulfide bonds to activate the immune signaling pathway (Ferrandon et al., 2007; An et al., 2010; Zhong et al., 2012; Vaniksampanna et al., 2019).
RfSpätzle is constitutively expressed at different levels in all tested tissues of RPW larvae. The expression level of RfSpätzle was significantly higher in gut and hemolymph compared to other tissues, indicating that it might be involved in the systemic and gut immunity of RPW larvae. Moreover, the challenge of S. aureus, E. coli, and B. bassiana strongly induced the expression of RfSpätzle in the fat body and gut of RPW larvae. Additionally, we found that RfSpätzle-silenced individuals were more vulnerable to pathogenic infection than controls. Further analysis confirmed that RfSpätzle silencing resulted in the significant downregulation of RfColeoptericin and RfCecropin, suggesting that RfSpätzle knockdown could compromise RPW innate immunity. Interestingly, the secretion of cecropin is IMD-dependent in D. melanogaster (Önfelt Tingvall et al., 2001), and coleoptericins have been shown to be IMD-dependent in the cereal weevil Sitophilus (Maire et al., 2018). Recently, a similar role of the Spz gene was also revealed in shrimp Litopenaeus vannamei (Yuan et al., 2017), the black tiger shrimp P. monodon (Boonrawd et al., 2017), and freshwater prawn M. rosenbergii (Vaniksampanna et al., 2019). It is known that D. melanogaster Spz, an extracellular ligand of the Toll receptor, is required to activate the production of antimicrobial peptides via the Toll pathway (Valanne et al., 2011). Collectively, these data indicate that RfSpätzle can mediate the secretion of some antimicrobial peptides of RPW larvae to defend against microbial intruders.
The gut microbiota has been confirmed to profoundly affect host physiological fitness, including development, nutrition metabolism, and immunity, in many metazoan animals (Muhammad et al., 2017; Zheng et al., 2017; Kamareddine et al., 2018; Hebineza et al., 2019). Importantly, the healthy homeostasis of gut microbiota is critical for proper growth and development of the host. However, the exact mechanisms by which animals tolerate the commensal bacteria while eliminating the transient pathogenic bacteria are still not well understood. In D. melanogaster, it is well-known that dual oxidase-mediated ROS production and the IMD pathway play vital roles in regulating the homeostasis of gut microbiota (Ryu et al., 2008; Lee et al., 2017). More recently, the RfRelish-mediated IMD-like pathway has been determined to be involved in modulating the homeostasis of RPW gut bacteria (Dawadi et al., 2018; Xiao et al., 2019). Insect immune responses are mainly under the control of two signaling pathways, the Toll and IMD pathways (Hetru and Hoffmann, 2009). However, the Toll pathway does not mediate local gut immunity in D. melanogaster (Davis and Engström, 2012). Previously, our transcriptome analysis found the essential elements for the Toll signaling pathway, including SPs, Spz-like proteins, Toll receptors, Tube-1, MyD88, TRAF, and Cactus, in the RPW gut (Muhammad et al., 2019). In the present investigation, we found that the expression levels of RfSpätzle and some antimicrobial peptides were drastically induced in the fat body and gut upon microbial infection. Specifically, it was found that the relative abundance of some gut bacteria in RPW larvae was significantly altered by RfSpätzle knockdown. The expression levels of antimicrobial peptides in the gut, RfColeoptericin and RfCecropin, were also reduced by RfSpätzle silencing. Antimicrobial peptides are one of the vital effectors to maintain insect-microbe symbiosis (Ryu et al., 2008; Login et al., 2011; Vigneron et al., 2012; Masson et al., 2015; Lee et al., 2017; Dawadi et al., 2018; Zaidman-Rémy et al., 2018; Xiao et al., 2019). Consequently, our data suggested that the RfSpätzle-mediated Toll-like signaling pathway could regulate the proportion of RPW gut bacteria by mediating the synthesis of antimicrobial peptides. To the best of our knowledge, this report is the first to reveal the possible role of the Spz-mediated Toll-like pathway in regulating the homeostasis of insect gut microbiota. However, RfSpätzle knockdown only differentially affected the relative abundance of four OTUs, including gram-positive and gram-negative bacteria, suggesting that the effects of the Toll-like signaling pathway on gut bacterial composition are highly limited and non-specific. It has been revealed that the majority of RPW gut bacteria are Gram-negative (Muhammad et al., 2017). This finding might explain the dominant role of the IMD-like pathway in maintaining the homeostasis of RPW gut bacteria.
It has been well defined that many weevils are associated with the γ-proteobacterial endosymbiont lineage Nardonella, which produces tyrosine for host cuticle formation and hardening (Hosokawa et al., 2015; Anbutsu et al., 2017). Although the weevil–Nardonella coevolution has lasted more than 100 million years, Nardonella infections in a number of weevil lineages have been lost or replaced by different bacterial lineages (Lefèvre et al., 2004; Conord et al., 2008). Therefore, although we still did not find Nardonella in RPW populations of our country in this study, it would be worthwhile to intensively investigate the presence of Nardonella in other beetle species to uncover the strikingly dynamic aspect of the endosymbiotic evolution in insects. The topic of insect immunity preserving endosymbionts and controlling their load and location while keeping the ability to cope with potential environmental infections by microbial intruders is highly interesting. Recently, the IMD-like pathway has been confirmed to mediate the control of Sodalis pierantonius in the bacteriome of Sitophilus spp. (Maire et al., 2018). However, whether the Toll pathway is involved in modulating the endosymbionts in bacteriome has not been determined. Moreover, the IMD and Toll pathways in hemipterans and other arthropods might be intertwined to target wider and overlapping arrays of microbes (Nishide et al., 2019). Therefore, further studies can detect whether there is any crosstalk between the IMD and Toll signaling pathways in this pest to regulate the homeostasis of RPW gut bacteria.
In conclusion, we determined that RfSpätzle is involved in the innate immunity of RPW by mediating the secretion of the antimicrobial peptides RfColeoptericin and RfCecropin. The RfSpätzle-mediated Toll-like signaling pathway not only confers protection against pathogen invasion but may also modulate the proportion of some gut bacteria.
The datasets generated for this study can be found in the NCBI database and the submission number of our sequence data is SAMN11959955-11959960.
ZS conceived and designed the research. ZS and YH provided the reagents. AM, PH, RX, and TJ completed the experiments. AM, XW, and ZS analyzed the data. ZS and AM prepared the manuscript. All authors have read and approved the final manuscript.
This work was supported by the National Natural Science Foundation of China (31470656), the National Key Research and Development Project of China (2017YFC1200605), and the Natural Science Foundation of Fujian Province (2018J01705).
The authors declare that the research was conducted in the absence of any commercial or financial relationships that could be construed as a potential conflict of interest.
The Supplementary Material for this article can be found online at: https://www.frontiersin.org/articles/10.3389/fmicb.2020.00846/full#supplementary-material
FIGURE S1 | Bacterial OTUs (operational taxonomic units) were recovered from the control insects (dseGFP) and RfSpätzle-silenced individuals (dsSPZ). Venn diagram indicates the unique and shared OTUs between the two groups.
FIGURE S2 | Principal coordinate analysis of the phylogenetic β-diversity matrix obtained starting from the OTU table. The explained variance is as follows: 62.71% 1st component, 27.53% 2nd component.
TABLE S1 | Effect of RfSpätzle knockdown on the reads number of OTUs between the two groups.
Aggarwal, K., and Silverman, N. (2008). Positive and negative regulation of the Drosophila immune response. BMB Rep. 41, 267–277. doi: 10.5483/bmbrep.2008.41.4.267
Al-Dosary, N. M. N., Al-Dobai, S., and Faleiro, J. R. (2016). Review on the management of red palm weevil Rhynchophorus ferrugineus Olivier in date palm Phoenix dactylifera L. emirates. J. Food Agri. 28:34. doi: 10.9755/ejfa.2015-10-897
Ami, E. B., Yuval, B., and Jurkevitch, E. (2010). Manipulation of the microbiota of mass-reared mediterranean fruit flies Ceratitis capitata (Diptera: Tephritidae) improves sterile male sexual performance. ISME J. 4, 28–37. doi: 10.1038/ismej.2009.82
An, C., Jiang, H., and Kanost, M. R. (2010). Proteolytic activation and function of the cytokine Spätzle in the innate immune response of a lepidopteran insect, Manduca sexta. FEBS J. 277, 148–162. doi: 10.1111/j.1742-4658.2009.07465.x
Anbutsu, H., Moriyama, M., Nikoh, N., Hosokawa, T., Futahashi, R., Tanahashi, M., et al. (2017). Small genome symbiont underlies cuticle hardness in beetles. Proc. Natl. Acad. Sci. U.S.A. 114, E8382–E8391. doi: 10.1073/pnas.1712857114
Bischoff, V., Vignal, C., Duvic, B., Boneca, I. G., Hoffmann, J. A., and Royet, J. (2006). Downregulation of the Drosophila immune response by peptidoglycan recognition proteins SC1 and SC2. PLoS Pathog. 2:e14. doi: 10.1371/journal.ppat.0020014
Blatch, S. A., Meyer, K. W., and Harrison, J. F. (2010). Effects of dietary folic acid level and symbiotic folate production on fitness and development in the fruit fly Drosophila melanogaster. Fly 4, 312–319. doi: 10.4161/fly.4.4.13258
Boonrawd, S., Mani, R., Ponprateep, S., Supungul, P., Masrinoul, P., Tassanakajon, A., et al. (2017). Characterization of PmSp?tzle 1 from the black tiger shrimp Peneaus monodon. Fish Shellf. Immunol. 65, 88–95. doi: 10.1016/j.fsi.2017.04.005
Conord, C., Depres, L., Vallier, A., Balmand, S., Miquel, C., Zundel, S., et al. (2008). Long-term evolutionary stability of bacterial endosymbiosis in curculionoidea: additional evidence of symbiont replacement in the Dryophthoridae family. Mol. Biol. Evol. 25, 859–868. doi: 10.1093/molbev/msn027
Davis, M. M., and Engström, Y. (2012). Immune response in the barrier epithelia: lessons from the fruit fly Drosophila melanogaster. J. Innate Immun. 4, 273–283. doi: 10.1159/000332947
Dawadi, B., Wang, X., Xiao, R., Muhammad, A., Hou, Y., and Shi, Z. (2018). PGRP-LB homolog acts as a negative modulator of immunity in maintaining the gut-microbe symbiosis of red palm weevil, Rhynchophorus ferrugineus Olivier. Dev. Comp. Immunol. 86, 65–77. doi: 10.1016/j.dci.2018.04.021
Douglas, A. E. (2015). Multiorganismal insects: diversity and function of resident microorganisms. Annu. Rev. Entomol. 60, 17–34. doi: 10.1146/annurev-ento-010814-020822
Engel, P., and Moran, N. A. (2013). The gut microbiota of insects - diversity in structure and function. FEMS Microbiol. Rev. 37, 699–735. doi: 10.1111/1574-6976.12025
Ferrandon, D., Imler, J.-L., Hetru, C., and Hoffmann, J. A. (2007). The Drosophila systemic immune response: sensing and signalling during bacterial and fungal infections. Nature Rev. Immunol. 7:862. doi: 10.1038/nri2194
Guo, L., Karpac, J., Tran, S. L., and Jasper, H. (2014). PGRP-SC2 promotes gut immune homeostasis to limit commensal dysbiosis and extend lifespan. Cell 156, 109–122. doi: 10.1016/j.cell.2013.12.018
Ha, E.-M., Oh, C.-T., Bae, Y. S., and Lee, W.-J. (2005). A direct role for dual oxidase in Drosophila gut immunity. Science 310, 847–850. doi: 10.1126/science.1117311
Hebineza, P., Muhammad, A., Ji, T., Xiao, R., Yin, X., Hou, Y., et al. (2019). The promoting effect of gut microbiota on growth and development of red palm weevil, Rhynchophorus ferrugineus Olivier (Coleoptera: Dryophthoridae) by modulating nutritional metabolism. Front. Microbiol. 10:1212. doi: 10.3389/fmicb.2019.01212
Hetru, C., and Hoffmann, J. A. (2009). NF-κB in the immune response of Drosophila. Cold Spring Harb. Perspect. Biol. 1:a000232. doi: 10.1101/cshperspect.a000232
Hoffmann, A., Funkner, A., Neumann, P., Juhnke, S., Walther, M., Schierhorn, A., et al. (2008). Biophysical characterization of refolded Drosophila Spätzle, a cystine knot protein, reveals distinct properties of three isoforms. J. Biol. Chem. 283, 32598–32609. doi: 10.1074/jbc.M801815200
Hosokawa, T., Koga, R., Tanaka, K., Moriyama, M., Anbutsu, H., and Fukatsu, T. (2015). Nardonella endosymbionts of Japanese pest and non-pest weevils (Coleoptera: Curculionidae). Appl. Entomol. Zool. 50, 223–229.
Hultmark, D. (2003). Drosophila immunity: paths and patterns. Curr. Opin. Immunol. 15, 12–19. doi: 10.1016/s0952-7915(02)00005-5
Hussain, A., Rizwan-ul-Haq, M., Al-Ayedh, H., and AlJabr, A. M. (2016). Susceptibility and immune defence mechanisms of Rhynchophorus ferrugineus (Olivier)(Coleoptera: Curculionidae) against entomopathogenic fungal infections. Int. J. Mol. Sci. 17:1518. doi: 10.3390/ijms17091518
Jang, I.-H., Chosa, N., Kim, S.-H., Nam, H.-J., Lemaitre, B., Ochiai, M., et al. (2006). A Spätzle-processing enzyme required for toll signaling activation in Drosophila innate immunity. Dev. Cell 10, 45–55. doi: 10.1016/j.devcel.2005.11.013
Janssen, A. W., and Kersten, S. (2015). The role of the gut microbiota in metabolic health. J. Fed. Am. Soc. Exp. Biol. 29, 3111–3123.
Jia, S., Zhang, X., Zhang, G., Yin, A., Zhang, S., Li, F., et al. (2013). Seasonally variable intestinal metagenomes of the red palm weevil (Rhynchophorus ferrugineus). Environ. Microbiol. 15, 3020–3029. doi: 10.1111/1462-2920.12262
Ju, R., Wang, F., Wan, F., and Li, B. (2011). Effect of host plants on development and reproduction of Rhynchophorus ferrugineus (Olivier) (Coleoptera: Curculionidae). J. Pest Sci. 84, 33–39. doi: 10.1007/s10340.010.0323.4
Kamareddine, L., Robins, W. P., Berkey, C. D., Mekalanos, J. J., and Watnick, P. I. (2018). The Drosophila immune deficiency pathway modulates enteroendocrine function and host metabolism. Cell Metabol. 28, 449–462. doi: 10.1016/j.cmet.2018.05.026
Kim, J. K., Lee, J. B., Huh, Y. R., Jang, H. A., Kim, C-H., Yoo, J. W., et al. (2015). Burkholderia gut symbionts enhance the innate immunity of host Riptortus pedestris. Dev. Comp. Immunol. 53, 265–269. doi: 10.1016/j.dci.2015.07.006
Lee, J.-H., Lee, K.-A., and Lee, W.-J. (2017). Microbiota, gut physiology, and insect immunity. Adv. Insect Physiol. 52, 111–138. doi: 10.1016/bs.aiip.2016.11.001
Lefèvre, C., Charles, H., Vallier, A., Delobel, B., Farrell, B., and Heddi, A. (2004). Endosymbiont phylogenesis in the dryophthoridae weevils: evidence for bacterial replacement. Mol. Biol. Evol. 21, 965–973. doi: 10.1093/molbev/msh063
Lemaitre, B., and Hoffmann, J. (2007). The host defense of Drosophila melanogaster. Annu. Rev. Immunol. 25, 697–743. doi: 10.1146/annurev.immunol.25.022106.141615
Lin, J., Xia, X., Yu, X.-Q., Shen, J., Li, Y., Lin, H., et al. (2018). Gene expression profiling provides insights into the immune mechanism of Plutella xylostella midgut to microbial infection. Gene 647, 21–30. doi: 10.1016/j.gene.2018.01.001
Login, F. H., Balmand, S., Vallier, A., Vincent-Monégat, C., Vigneron, A., Weiss-Gayet, M., et al. (2011). Antimicrobial peptides keep insect endosymbionts under control. Science 334, 362–365. doi: 10.1126/science.1209728
Maire, J., Vincent-Monégat, C., Balmand, S., Vallier, A., Hervé, M., Masson, F., et al. (2019). Weevil pgrp-lb prevents endosymbiot TCT dissemination and chronic host systemic immune activation. Proc. Natl. Acad. Sci. U.S.A. 116, 5623–5632. doi: 10.1073/pnas.1821806116
Maire, J., Vincent-Monégat, C., Masson, F., Zaidman-Rémy, A., and Heddi, A. (2018). An IMD-like pathway mediates both endosymbiont control and host immunity in the cereal weevil Sitophilus spp. Mcirobiome 6:6. doi: 10.1186/s40168-017-0397-9
Masson, F., Vallier, A., Vigneron, A., Balmand, S., Vincent-Monégat, C., Zaidman-Rémy, L., et al. (2015). Systemic infection generates a local-like immune response of the bacteriome organ in insect symbiosis. J. Innate Immunol. 7, 290–301. doi: 10.1059/000368928
Mastore, M., Ariza, V., Manachini, B., and Brivio, M. (2014). Modulation of immune responses of Rhynchophorus ferrugineus (Insecta: Coleoptera) induced by the entomopathogenic nematode Steinernema carpocapsae (Nematoda: Rhabditida). Insect Sci. 22, 748–760. doi: 10.1111/1744-7917.12141
Montagna, M., Chouaia, B., Mazza, G., Prosdocimi, E. M., Crotti, E., Mereghetti, V., et al. (2015). Effects of the diet on the microbiota of the red palm weevil (Coleoptera: Dryophthoridae). PLoS One 10:e0117439. doi: 10.1371/journal.pone.0117439
Muhammad, A., Fang, Y., Hou, Y., and Shi, Z. (2017). The gut entomotype of red palm weevil Rhynchophorus ferrugineus Olivier (Coleoptera: Dryophthoridae) and their effect on host nutrition metabolism. Front. Microbiol. 8:2291. doi: 10.3389/fmicb.2017.02291
Muhammad, A., Prosper, H., Ji, T., Hou, Y., and Shi, Z. (2019). Intestinal microbiota confer protection by priming the immune systme of red palm weevil Rhynchophorus ferrugineus Olivier (Coleoptera: Dryophthoridae). Front. Physiol. 10:1303. doi: 10.3389/fphys.2019.01303
Nishide, Y., Kageyama, D., Yokoi, K., Jouraku, A., Tanaka, H., Futahashi, R., et al. (2019). Functional crosstalk across IMD and Toll pathways: insight into the evolution of incomplete immune cascades. Proc. R. Soc. B. 286:20182207. doi: 10.1098/rspb.2018.2207
Önfelt Tingvall, T., Roos, E., and Engström, Y. (2001). The imd gene is required for local Cecropin expression in Drosophila barrier epithelia. EMBO Rep. 2, 239–243. doi: 10.1093/embo-reports/kve048
Paredes, J. C., Welchman, D. P., Poidevin, M., and Lemaitre, B. (2011). Negative regulation by amidase PGRPs shapes the Drosophila antibacterial response and protects the fly from innocuous infection. Immunity 35, 770–779. doi: 10.1016/j.immuni.2011.09.018
Park, K-E., Jang, S. H., Lee, J., Lee, S. A., Kikuchi, Y., Seo, Y-S., et al. (2018). The roles of antimicrobial peptide, rip-thanatin, in the midgut of Riptortus pedestris. Dev. Comp. Immunol. 78, 83–90. doi: 10.1016/j.dci.2017.09.009
Parker, J. S., Mizuguchi, K., and Gay, N. J. (2001). A family of proteins related to Spätzle, the toll receptor ligand, are encoded in the Drosophila genome. Proteins 45, 71–80. doi: 10.1002/prot.1125
Pu, Y., Ma, T., Hou, Y., and Sun, M. (2017). An entomopathogenic bacterium strain, Bacillus thuringiensis, as a biological control agent against the red palm weevil, Rhynchophorus ferrugineus (Coleoptera: Curculionidae). Pest Manag. Sci. 73, 1494–1502. doi: 10.1002/ps.4485
Ryu, J.-H., Kim, S.-H., Lee, H.-Y., Bai, J. Y., Nam, Y.-D., Bae, J.-W., et al. (2008). Innate immune homeostasis by the homeobox gene caudal and commensal-gut mutualism in Drosophila. Science 319, 777–782. doi: 10.1126/science.1149357
Shi, Z. H., Lin, Y. T., and Hou, Y. M. (2014). Mother-derived trans-generational immune priming in the red palm weevil, Rhynchophorus ferrugineus olivier (Coleoptera, Dryophthoridae). Bull. Entomol. Res. 104, 742–750. doi: 10.1017/S0007485314000583
Shin, S. W., Bian, G., and Raikhel, A. S. (2006). A toll receptor and a cytokine, Toll5A and Spz1C, are involved in toll anti-fungal immune signaling in the mosquito Aedes aegypti. J. Biol. Chem. 281, 39388–39395. doi: 10.1074/jbc.M608912200
Stokes, B. A., Yadav, S., Shokal, U., Smith, L., and Eleftherianos, I. (2015). Bacterial and fungal pattern recognition receptors in homologous innate signaling pathways of insects and mammals. Front. Microbiol. 6:19. doi: 10.3389/fmicb.2015.00019
Sun, Y., Jiang, Y., Wang, Y., Li, X., Yang, R., Yu, Z., et al. (2016). The toll signaling pathway in the chinese oak silkworm, Antheraea pernyi: innate immune responses to different microorganisms. PLoS One 11:e0160200. doi: 10.1371/journal.pone.0160200
Tagliavia, M., Messina, E., Manachini, B., Cappello, S., and Quatrini, P. (2014). The gut microbiota of larvae of Rhynchophorus ferrugineus Oliver (Coleoptera: Curculionidae). BMC Microbiol. 14:136. doi: 10.1186/1471-2180-14-136
Valanne, S., Wang, J.-H., and Rämet, M. (2011). The Drosophila toll signaling pathway. J. Immunol. 186, 649–656. doi: 10.4049/jimmunol.1002302
Vaniksampanna, A., Longyant, S., Charoensapsri, W., Sithigorngul, P., and Chaivisuthangkura, P. (2019). Molecular isolation and characterization of a spätzle gene from Macrobrachium rosenbergii. Fish Shellf. Immunol. 84, 441–450. doi: 10.1016/j.fsi.2018.10.015
Vigneron, A., Charif, D., Vincent-Monégat, C., Vallier, A., Gavory, F., Wincker, P., et al. (2012). Host gene response to endosymbiont and pathogen in the cereal weevil Sitophilus oryzae. BMC Microbiol. 12(Suppl.):S14. doi: 10.1186/1471-2180-12-S1-S14
Wang, P., Liang, J., Gu, Z., Wan, D., Weng, S., Yu, X., et al. (2012). Molecular cloning, characterization and expression analysis of two novel Tolls (LvToll2 and LvToll3) and three putative Spätzle-like Toll ligands (LvSpz1–3) from Litopenaeus vannamei. Dev. Comp. Immunol. 36, 359–371. doi: 10.1016/j.dci.2011.07.007
Wang, Y., Cheng, T., Rayaprolu, S., Zou, Z., Xia, Q., Xiang, Z., et al. (2007). Proteolytic activation of pro-spätzle is required for the induced transcription of antimicrobial peptide genes in Lepidopteran insects. Dev. Comp. Immunol. 31, 1002–1012. doi: 10.1016/j.dci.2017.01.001
Wang, Y., and Zhu, S. (2009). Evolutionary and functional epitopes of the Spätzle protein: new insights into activation of the toll receptor. Cell. Mol. Life Sci. 66, 1595–1602. doi: 10.1007/s00018-009-9028-3
Weber, A. N., Tauszig-Delamasure, S., Hoffmann, J. A., Lelièvre, E., Gascan, H., Ray, K. P., et al. (2003). Binding of the Drosophila cytokine Spätzle to Toll is direct and establishes signaling. Nat. Immunol. 4:794. doi: 10.1038/ni955
Wong, A. C., Dobson, A. J., and Douglas, A. E. (2014). Gut microbiota dictates the metabolic response of Drosophila to diet. J. Exp. Biol. 217, 1894–1901. doi: 10.1242/jeb.101725
Xiao, R., Wang, X., Xie, E., Ji, T., Li, X., Muhammad, A., et al. (2019). An IMD-like pathway mediates the intestinal immunity to modulate the homeostasis of gut microbiota in Rhynchophorus ferrugineus Olivier (Coleoptera: Dryophthoridae). Dev. Comp. Immunol. 97, 20–27. doi: 10.1016/j.dci.2019.03.013
Yuan, K., Yuan, F., Weng, S., He, J., and Chen, Y. (2017). Identification and functional characterization of a novel Spätzle gene in Litopenaeus vannamei. Dev. Comp. Immunol. 68, 46–57. doi: 10.1016/j.dci.2016.11.016
Zaidman-Rémy, A., Hervé, M., Poidevin, M., Pili-Floury, S., Kim, M.-S., Blanot, D., et al. (2006). The Drosophila amidase PGRP-LB modulates the immune response to bacterial infection. Immunity 24, 463–473. doi: 10.1016/j.immuni.2006.02.012
Zaidman-Rémy, A., Vigneron, A., Weiss, L. B., and Heddi, A. (2018). Whant can a weevil teach a fly, and reciprocally? Interaction of host immune systems with endosymbionts in Glossina and Sitophilus. BMC Microbiol. 18(Suppl. 1):150. doi: 10.1186/s12866-018-1278-5
Zheng, H., Powell, J. E., Steele, M. I., Dietrich, C., and Moran, N. A. (2017). Honeybee gut microbiota promotes host weight gain via bacterial metabolism and hormonal signaling. Proc. Natl. Acad. Sci. U.S.A. 114, 4775–4780. doi: 10.1073/pnas.1701819114
Keywords: Rhynchophorus ferrugineus, Spätzle, toll pathway, antimicrobial peptides, gut microbiota
Citation: Muhammad A, Habineza P, Wang X, Xiao R, Ji T, Hou Y and Shi Z (2020) Spätzle Homolog-Mediated Toll-Like Pathway Regulates Innate Immune Responses to Maintain the Homeostasis of Gut Microbiota in the Red Palm Weevil, Rhynchophorus ferrugineus Olivier (Coleoptera: Dryophthoridae). Front. Microbiol. 11:846. doi: 10.3389/fmicb.2020.00846
Received: 08 September 2019; Accepted: 08 April 2020;
Published: 25 May 2020.
Edited by:
David Clarke, University College Cork, IrelandReviewed by:
Ioannis Eleftherianos, The George Washington University, United StatesCopyright © 2020 Muhammad, Habineza, Wang, Xiao, Ji, Hou and Shi. This is an open-access article distributed under the terms of the Creative Commons Attribution License (CC BY). The use, distribution or reproduction in other forums is permitted, provided the original author(s) and the copyright owner(s) are credited and that the original publication in this journal is cited, in accordance with accepted academic practice. No use, distribution or reproduction is permitted which does not comply with these terms.
*Correspondence: Youming Hou, eW1ob3VAZmFmdS5lZHUuY24=; Zhanghong Shi, c2hpemhAZmFmdS5lZHUuY24=
Disclaimer: All claims expressed in this article are solely those of the authors and do not necessarily represent those of their affiliated organizations, or those of the publisher, the editors and the reviewers. Any product that may be evaluated in this article or claim that may be made by its manufacturer is not guaranteed or endorsed by the publisher.
Research integrity at Frontiers
Learn more about the work of our research integrity team to safeguard the quality of each article we publish.