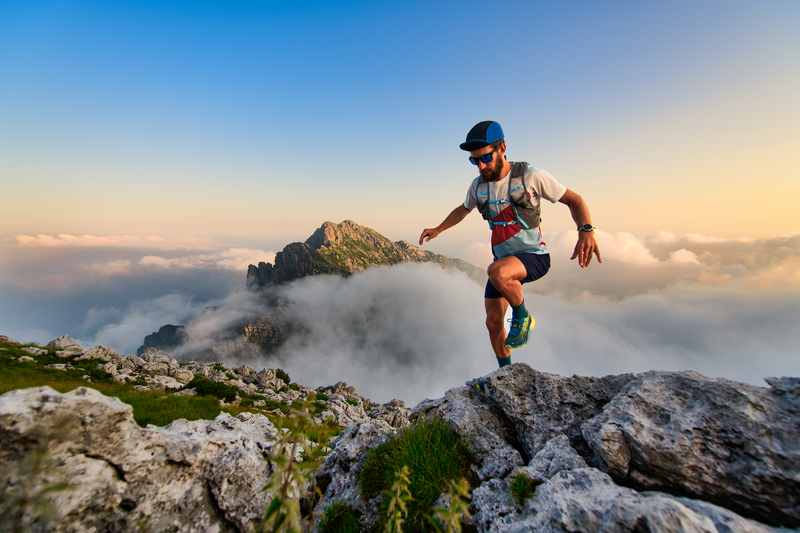
94% of researchers rate our articles as excellent or good
Learn more about the work of our research integrity team to safeguard the quality of each article we publish.
Find out more
ORIGINAL RESEARCH article
Front. Microbiol. , 07 May 2020
Sec. Microbial Physiology and Metabolism
Volume 11 - 2020 | https://doi.org/10.3389/fmicb.2020.00804
In this study, we investigated the global gene expression responses of Elizabethkingia anophelis to iron fluxes in the midgut of female Anopheles stephensi mosquitoes fed sucrose or blood, and in iron-poor or iron-rich culture conditions. Of 3,686 transcripts revealed by RNAseq technology, 218 were upregulated while 112 were down-regulated under iron-poor conditions. Hemolysin gene expression was significantly repressed when cells were grown under iron-rich or high temperature (37°C) conditions. Furthermore, hemolysin gene expression was down-regulated after a blood meal, indicating that E. anophelis cells responded to excess iron and its associated physiological stress by limiting iron loading. By contrast, genes encoding respiratory chain proteins were up-regulated under iron-rich conditions, allowing these iron-containing proteins to chelate intracellular free iron. In vivo studies showed that growth of E. anophelis cells increased 3-fold in blood-fed mosquitoes over those in sucrose-fed ones. Deletion of siderophore synthesis genes led to impaired cell growth in both iron-rich and iron-poor media. Mutants showed more susceptibility to H2O2 toxicity and less biofilm formation than did wild-type cells. Mosquitoes with E. anophelis experimentally colonized in their guts produced more eggs than did those treated with erythromycin or left unmanipulated, as controls. Results reveal that E. anophelis bacteria respond to varying iron concentration in the mosquito gut, harvest iron while fending off iron-associated stress, contribute to lysis of red blood cells, and positively influence mosquito host fecundity.
Elizabethkingia anophelis is an aerobic, non-fermenting, Gram-negative rod (Kämpfer et al., 2011). It is ubiquitously distributed in diverse natural environments including water, soil, sediment, plants, and animal digestive tracts (Wang et al., 2011; Breurec et al., 2016). E. anophelis and/or its close relative E. meningoseptica associate symbiotically with the gut lumen environment of Anopheles (Kämpfer et al., 2011) and Aedes mosquitoes, whether in wild-caught individuals or those from insectary colonies (Terenius et al., 2012; Pei et al., 2017). E. anophelis enriched in the larval Anopheles gut during filter feeding from the surrounding water medium, transmitted transtadially from larval to adult gut lumen during metamorphosis, and transmitted vertically to the next generation through an uncharacterized mechanism (Wang et al., 2011). E. anophelis infection resulted in high mortality in adult Anopheles when injected through the cuticle into the mosquito hemocoel, exhibiting pathogenesis, but stabilized as non-pathogenic symbionts when fed to the mosquitoes and confined to gut (Akhouayri et al., 2013). These findings indicate that E. anophelis can be opportunistically pathogenic in mosquitoes but that the gut provides a barrier against systemic infection (Akhouayri et al., 2013). Hospital-acquired infections of E. anophelis occur in sick or immunocompromised individuals, sometimes leading to death (Teo et al., 2014; Sun et al., 2015; Breurec et al., 2016). E. anophelis was the cause of a recent outbreak of nosocomial illness in the Upper Midwest region of the United States (Wisconsin, Illinois, and Michigan) (Breurec et al., 2016; Figueroa Castro et al., 2017; Perrin et al., 2017). Other outbreaks have occurred in Africa, Singapore, Taiwan and Hong Kong (Teo et al., 2014; Lau et al., 2016; Lin et al., 2017). A survey of five hospitals in Hong Kong showed that bacteremia in patients due to E. anophelis was often associated with severe clinical outcomes including mortality (Lau et al., 2016). Bacteria disseminated from hospital water service lines, especially sink faucets, during handwashing to the hands of healthcare workers, who subsequently exposed patients when providing care (Chee-Fu et al., 2018). The association between bacterial infection in mosquitoes and infection in human beings is not established (Lau et al., 2016).
Regular but episodic influxes of blood enter the female mosquito midgut, greatly but temporarily altering gut physiology and environmental conditions, including an increase in proteolytic enzyme activity associated with blood meal digestion, formation of a chitinous peritrophic matrix around the blood meal, a sudden and large increase in temperature, and large flux of iron from heme and ferric-transferrin sources (DeJong et al., 2007; Champion and Xu, 2018). Mosquitoes also take sugar meals, whose first destination is the foregut “crop” from where sugar moves to the midgut for digestion and assimilation (Foster, 1995). Iron is practically nil in the midgut after a sugar meal and between blood meals, but spikes to ca. 600 ng iron per ul after a blood meal (Zhou et al., 2007). The community structure of the microbiome in the mosquito gut varies temporally with episodes of blood and sugar feeding (Wang et al., 2011; Chen et al., 2016). Our specific interest in E. anophelis focuses on understanding the survival mechanisms and physiological adaptations as it establishes and maintains infection in the mosquito gut, where environmental conditions such as iron flux are highly variable.
Iron is an essential cofactor in many enzymes that are involved in maintaining cell homeostasis and functions (Zhang, 2014). Thus, bioavailability of iron greatly influences bacterial metabolism, growth and transcription (Expert et al., 1996; Andrews, 1998; Andrews et al., 2003). In the mosquito midgut, the microbiota meet two very different iron concentrations. Very limited iron (non-heme form, Fe3+ or Fe2+) will be available in the female midgut when only nectar is imbibed (Zhou et al., 2007). Microorganisms might employ various mechanisms to scavenge iron under those conditions (Andrews et al., 2003). One of the most efficient strategies to sequester iron is to secrete iron chelator siderophores (Frawley and Fang, 2014). Iron-bound siderophores are transported into periplasm through specific siderophore receptors (TonB-dependent iron transports) or other transport systems (Molina et al., 2005). Heme/iron increases suddenly when a mosquito ingests and digests erythrocytes, increasing oxidative stress in the gut lumen (Benoit et al., 2011; Oliveira et al., 2011). Bacteria secrete hemophores to capture hemin from hemoproteins (released from hemoglobin when erythrocytes are disrupted) and deliver it to bacterial periplasm (Cescau et al., 2007).
The ability to scavenge iron, manage iron-induced stress, and minimize damage from reactive oxygen radicals generated by iron could influence bacterial colonization and survivorship in the mosquito gut (Pumpuni et al., 1996; Molina et al., 2005; Frawley and Fang, 2014; Ledala et al., 2014). In this study, we hypothesize that gene expression revealing these processes in E. anophelis will be significantly influenced by iron availability in the mosquito midgut, depending on the two normal types of meals (blood vs sugar). To explore this hypothesis, we analyzed global transcriptomic changes in E. anophelis under iron-replete (or iron-rich) and iron-depleted (or iron-poor) conditions. We developed a genetic manipulation system to knock out siderophore synthesis genes whose expression was significantly regulated by iron. Furthermore, we labeled wild-type and mutant bacteria with sensitive luciferase-based reporters for purposes of quantifying their growth and gene expression in mosquitoes. Hemolysin genes responding to iron availability in vitro and blood meals in vivo were investigated in detail. The goal was to use a combination of transcriptomic and genetic analyses of iron metabolism in E. anophelis to expand our understanding of bacterial survival mechanisms and physiological functions in the mosquito gut.
Strains and molecular reagents used in this study are listed in Table 1. Escherichia coli JM109 was used for cloning. E. coli S17 (λ pir) was used for conjugation. Luria-Bertani (LB) media were used for E. coli cultures. E. anophelis Ag1 was isolated from the mosquito A. gambiae (Kukutla et al., 2014) and was cultured in tryptic soy broth (TSB) or LB media (Chen et al., 2017c). Liquid cultures were grown with shaking (ca. 200 rpm) at either 30°C (E. anophelis and Flavobacterium johnsoniae) or 37°C (E. coli). For solid LB media, Bacto-Agar (Difco, Detroit, Michigan) was added to a final concentration of 20 g/L. Whenever necessary, erythromycin (100 μg/ml) (abbreviation, Em), kanamycin (50 μg/ml) (abbreviation, Km) or ampicillin (100 μg/ml) (abbreviation, Amp) was added to media to screen transconjugants or bacteria with plasmids, respectively.
Genomic DNA was prepared using a genomic DNA extraction kit (Promega, Madison, WI, United States), and plasmid DNA was purified with the QIAprep spin miniprep kit (QIAGEN, Germantown, MD, United States). PCR amplifications were done with the Failsafe PCR system (Epicenter Technology, Madison, WI, United States). Amplicons were separated in 0.7–1.0% (w/v) agarose gels, and DNA fragments were purified with the QIAquick gel extraction system (QIAGEN). Restriction and modification enzymes were purchased from Promega (Madison, WI, United States) or New England Biolabs (Beverly, MA, United States). Ligation mixtures were transformed into E. coli cells, and transformants were plated onto LB plates with appropriate antibiotic selection. Resistant colonies were isolated, and then screened for the acquisition of plasmids. All constructs were sequenced to verify the structure.
Mosquito rearing, blood feeding and bacterial infection procedures were described in detail by Chen et al. (2015). Briefly, for erythromycin-treatment of the mosquito gut environment, sterilized 10% sugar solution mixed with erythromycin antibiotic (200 μg/ml) was provided to the mosquitoes through cotton wicks for 7 days, with fresh antibiotic administered daily. After that procedure, sterilized 10% sugar only was used prior to the blood meal. To estimate the erythromycin treatment efficiency, midgut contents were plated on LB agar for CFU calculation. Compared to the control group (6.7 × 105 CFU/ml), the bacterial CFUs significantly decreased (2.2 × 105 CFU/ml) but bacterial burden was not eliminated. For E. anophelis infection treatment, pure culture was added to the 10% sterile sucrose solution (2.4 × 103 CFU/ml, final concentration) and fed to antibiotic pretreated mosquitoes for 1 day. All the female mosquitoes obtained above (conventionally reared, Em-treated, and Elizabethkingia-infected) were starved from sugar for 12 h before blood meal. Unfed and partially fed mosquitoes were removed and replete females used for fecundity assessment. Individuals were transferred to 50 ml sterile tubes, covered with a netting material for easy access of sucrose. Each mosquito was provided with an autoclaved wet filter paper and cotton wool substrate, held at 27oC in an incubator, and allotted 2 days for oviposition. Filter papers were removed and the number of eggs counted under a stereoscopic microscope. Ten replicates for each treatment were used.
Hemolysin production in E. anophelis was demonstrated by growing bacteria on Remel Blood Agar plate (Thermo Scientific, Lenexa, KS, United States) as described previously (Kukutla et al., 2014). Bovine whole blood cells (Hemostat Laboratories, Dixon, CA, United States) were washed with phosphate buffered saline (PBS) and re-suspended in 1 mL of PBS. E. anophelis (final concentration, 7.27 × 106 cells/mL) was incubated with the above washed blood cells at 37°C and 100 rpm for 4 days. The numbers of erythrocytes were counted using a hemocytometer under microscopy. The negative control was the same as the treatment without introduction of bacteria.
Elizabethkingia anophelis cultures were grown overnight in LB and OD600nm was adjusted to 0.1 by diluting the cells into 20 ml of LB supplemented with either 12 μM of FeCl3 or 200 μM of 2, 2-dipyridyl (to create iron-deficient condition) in 250 ml flasks (Chen et al., 2010a). Cells were grown at 30°C with shaking (200 rpm) for 3 h to a final OD of 0.2 or 0.5 for iron-depleted cultures or iron-replete ones, respectively. Cells grown in log-phase were re-suspended in RNAlater and frozen immediately after being harvested. RNA was isolated using Trizol reagents following the manufacturer’s manual. Residual DNA in the samples was removed using Dnase I. The integrity of the RNA was analyzed using an Agilent bioanalyzer (Agilent Technologies). The Ribo-Zero rRNA removal kit (Gram-negative bacteria, Epicentre) was used to remove the ribosomal RNA species (23S and 16S rRNA) from total RNA in samples. Library construction and sequencing were performed by Beijing Genomics Institute (BGI) using TruSeq RNA sample preparation v2 guide (Illumina). Three biological replicates of each treatment were used for RNA-Seq. The libraries were sequenced using the Illumina HiSeq 2000 platform with a paired-end protocol and read lengths of 100 bps.
Data from RNA-seq were checked for quality control (QC), pre-trimming, using Fast QC1. Raw reads were subjected to trimming of low-quality bases and removal of adapter sequences using Trimmomatic (v0.32) with a 4-bp sliding window (Bolger et al., 2014) when the read quality was below 15 (using the Phred64 quality scoring system) or read length was less than 50 bp. The trimming process improved the quality of the data as evidenced by comparing Fast QC reports pre- and post-trimming. Forward and reverse read pairs were aligned to the reference genome using Bowtie2 (v2.2.3) with the –S option to produce SAM files as output2. SAM files were converted to BAM format, sorted by name using the –n option and converted back to SAM format using SAMTools (Li et al., 2009). Aligned reads were then counted per gene feature in the E. anophelis Ag1 genome using the HTSeq Python library (Anders et al., 2015). Specifically, counting was performed using the htseq-count function within the HTSeq suite of tools using the “–r” name and “–s” no options. Differential gene expression was calculated by normalizing the data utilizing the trimmed mean of M-values normalization method and filtering out genes that had <10 counts per million (CPM) within the edgeR package (Robinson et al., 2010). Statistical analysis was performed in RStudio (v 0.98.1102) (RStudio, 2012) by the exact test with a negative binomial distribution for each set of conditions and testing for differential gene expression using edgeR (Robinson et al., 2010). Differentially expressed genes (DEG) were determined to be statistically significant based on an adjusted P < 0.05. Magnitude amplitude (MA) plots were generated by modifying a function within the edgeR package (Robinson et al., 2010). Red dots indicate significantly expressed genes (adjusted P < 0.05) and black dots are those not significantly expressed. Blue lines indicate two-fold changes either up- or down-regulated.
Elizabethkingia anophelis possessed at least three genes encoding putative hemolysins (here named Elilysin1, EAAG1_002100; Elilysin2, EAAG1_002105; Elilysin3, EAAG1_016825) (see section “Results”). Among them, EAAG1_11032 was chosen for this study because it was highly regulated by iron (Supplementary Table S1). Moreover, the promoter of the Elilysin2 gene (EAAG1_002105) carried a typical promoter motif conserved in Bacteroidetes (Supplementary Figure S1). Identification of the transcriptional start site, promoter, and regulatory region prediction is described in Supplementary Figure S1. A 757-bp fragment spanning the 5′-end of EAAG1_002105 and the 3′-end of a hypothetical protein was amplified with primers Walker183 and Walker185 using genomic DNA as template. The amplicon was cloned into the T-easy vector (pSCH893) (Table 1). The insert was released from pSCH893 by restriction enzymes SmaI and SacII and ligated into the same sites on the transposon pSCH801 (Chen et al., 2015), creating pSCH905 (Table 1). pSCH905 was conjugatively transferred into E. anophelis and colonies with erythromycin resistance and luciferase production were selected, leading to the reporter strain SCH908 (Table 1). pSCH908 was inserted into a gene encoding the hypothetical protein, which did not affect bacterial growth compared to the WT (data not shown).
For determination of NanoLuc reporter activity in vitro, the properly diluted cells were added to an equal volume of NanoLuc assay buffer (Promega, Madison, WI). The light intensity was measured in 96-well microtiter plates by using a plate reader following the manufacturer’s protocol. For determination of NanoLuc reporter activity in vivo, The mosquitoes were homogenized with a sterile pestle, centrifuged, washed with phosphate-buffered saline (PBS), resuspended in PBS, and subjected to NanoLuc reporter analysis as described above. Meanwhile, colony forming units were determined by plating mosquito suspensions onto LB agar supplemented with erythromycin (100 μg/ml). Standard curves were established each time to quantify the relationship between bacterial density and luminescence.
The siderophore synthesis gene cluster consisting of iucA/iucC (EAAG1_002765), iucB (EAAG1_002760) and iucD (EAAG1_002755) was targeted for deletion. To accomplish it, upstream (1963-bp) and downstream (1657-bp) gene fragments were amplified with primers Walker277/Walker278 and Walker285/Walker 287, respectively, by using E. anophelis genomic DNA as the template. Amplicons were gel purified and separately cloned into pGEM-T easy (pSCH1038 and pSCH1033). Upstream fragments were released by BamHI/SalI digestion, and downstream fragments were released by SalI/SphI from pGEM-T easy and then sequentially assembled at the same sites on the suicide plasmid pYT313 (pSCH1034).
Plasmid pSCH1034 was conjugatively transferred into E. anophelis through procedures described elsewhere (Zhu et al., 2017). Merodiploids were selected on LB plates supplemented with Em. The Em-resistant merodiploids were resolved by plating single colonies onto LB agar medium containing 10% (wt/vol) sucrose and Em according to a previously described method (Zhu et al., 2017). Putative (ΔiucA_iucC/iucB/iucD) clones were identified by screening with PCR with primers Walker295/Walker296 (Table 1) and checked for susceptibility to Em and sucrose; one confirmed (ΔiucA_iucC/iucB/iucD) clone (SCH1065) was chosen for further analysis.
The CAS solution was prepared by following procedures previously described (Li et al., 2015). The CAS solution consisted of HDTMA (0.6 mM), FeCl3 (15 μm), CAS (0.15 mM) and piperazine (500 mM). The buffer system was PIPES (1 mM, pH 5.6). Due to the lack of the commercially available aerobactin as a reference, we utilized the purified deferoxamine mesylate salt (Sigma-Aldrich, United States) as a standard to measure siderophore activity. CAS solution was mixed with the equivalent volume of the filtered supernatants from various cultures, incubated at 37°C for 3 h, and the OD630nm determined on a microplate reader.
E. anophelis cultures grown overnight (16 h) were diluted 10-fold in 20 ml of LB supplemented with either 40 μM FeCl3, 10 μM hemoglobin, or 1.28 mM 2, 2-dipyridyl in a 250 ml flask. After 3 h, the log-phase cells were harvested and washed with 1 × PBS. After OD600nm was adjusted to 0.1, the washed E. anophelis cells were treated with 20 μM of H2O2 (final concentration) for 20 min. Then cells were extensively washed with 1 × PBS three times before plating on LB agar for viable counts.
E. anophelis was cultured in TSB broth at 37°C with agitation. Cultures grown overnight were diluted in TSB broth supplemented with either 40 μM of FeCl3 or 1.28 mM of 2, 2-dipyridyl, respectively. 200 μl of the above bacterial suspensions were inoculated into individual wells of the 96-well polystyrene microtiter plates and statically cultured overnight. TSB broth without bacterial inoculation was used as the negative control. A modified biofilm assay was carried out according to published methods (Basson et al., 2008; Jacobs and Chenia, 2011). Planktonic cells were removed and the absorbed cells were air dried for 30 min at room temperature. 0.5% crystal violet was added into the wells, incubated for 15 min at room temperature, and rinsed thoroughly with distilled water. After air drying, crystal violet was solubilized in 200 μl of ethanol acetone (80:20, vol/vol) for 30 min, and the OD570nm was measured by using a SpectraMax M5 microplate reader (Molecular Devices, Sunnyvale, CA, United States). 12 replicates were used for each treatment.
The RNA-seq data were submitted to the NCBI Gene Expression Omnibus, BioProject, and The Sequence Read Archive, under accession numbers GSE132933, PRJNA549490, and SRP201789 respectively.
The raw sequence output of the transcriptomes included 150 million reads in total. Reads were well matched (100%) to the published E. anophelis Ag1 genome (Wang et al., 2011). Out of the 3,686 transcribed genes detected in this study (Supplementary Table S1), 330 displayed a significant change (more than 4-fold, adjusted P value <0.01), which counted for 9% of total transcripts in E. anophelis (Supplementary Table S2). Among the genes significantly regulated by iron, 218 transcripts displayed decreased expression and 112 showed increased expression (Figure 1 and Supplementary Tables S2, S3). Multidimensional scaling analysis of the matrix of up- and down-regulated genes by experimental category of high- or low-iron treatment samples showed that the transcriptomes of the biological replicates in iron-rich samples grouped together while they were well separated from those grown under iron-poor conditions (Supplementary Figure S2). Enrichment analysis of KEGG pathways (Figure 2A) showed that the majority of up-regulated DEGs was assigned to “energy metabolism” (Andrews, 1998) including oxidative phosphorylation (NADH-quinone oxidoreductase). Next to “energy metabolism,” the enriched DEGs were involved in “environmental information” (Teo et al., 2014) or “genetic information processing” (Akhouayri et al., 2013). In contrast to up-regulated DEGs, down-regulated genes (Figure 2B) were predominantly enriched in the “genetic information” (Molina et al., 2005) and “environmental information” (Expert et al., 1996) processing categories, including translation and amino acid metabolism. Further, metabolic analyses by STRING (Figures 2C,D) indicated that many genes involved in oxidative phosphorylation (e.g., NADH:quinone oxidoreductases) were clustered together in high iron cells (Figure 2C). It is very likely that they interact with each other due to similar functions and cellular compartments. Many gene products involved in lipid, porphyrin and phenylalanine metabolism also formed clusters with possible interactions (Figure 2C). By contrast, amino acid synthesis (aromatic amino acid biosynthesis), iron uptake (siderophore, enterobactin and receptors), vitamin (B12) synthesis, ribosome proteins and thioredoxin were enriched and clustered together in iron-restricted cells (Figure 2D).
Figure 1. Comparison of differential gene expression between E. anophelis Ag1 cultures held in low- and high-iron culture conditions. (A) Heat maps of 100 genes with significant regulation by iron availability. Left, high-iron condition; right, low-iron condition. (B) Magnitude amplitude plots generated by a modifying function within the edgeR package. Red dots indicate statistically significant genes (adjusted P < 0.05) and black dots are non-statistically significant differentially regulated genes. Blue lines indicate two-fold changes either up-regulated or down-regulated.
Figure 2. KEGG and STRING analysis of differentially regulated genes in E. anophelis Ag1. (A) KEGG pathway enrichment analysis of up-regulated DEGs. Most of up-regulated DEGs were enriched to Energy Metabolism. (B) KEGG pathway enrichment analysis of down-regulated DEGs. Down-regulated genes were predominantly enriched in the Genetic Information Processing. (C) STRING analysis of up-regulated DEGs. Up-regulated DEGs were clustered to oxidative phosphorylation (circled). (D) STRING analysis of down-regulated DEGs. Down-regulated DEGs were clustered to ribosome and the biosynthesis of phenylalanine, tyrosine and tryptophan (circled).
Genes encoding the respiratory chain protein components were significantly up-regulated in iron-replete media (Table 2). Related to this, the gene cluster related to quinol:cytochrome c oxidoreductase (bc1 complex or complex III) synthesis and assembly in E. anophelis was further studied in details (Figure 3A). The bc1 cluster consists of cytochrome c2, quinol:cytochrome c oxidoreductase iron-sulfur protein precursor, hydrogenase, monoheme cytochrome subunit, quinol:cytochrome C oxidoreductase subunit II, and quinol:cytochrome c oxidoreductase membrane protein. Transcription levels of these genes were 4.1∼8.6-fold higher in cells in iron-rich compared to iron-limited conditions (Figure 3A). Besides the bc1 gene cluster, there was a cytochrome cbb3 gene cluster (Figure 3B) consisting of 8 genes encoding oxidase subunit I/II, subunit III, copper exporting ATPase, copper tolerance proteins, assembly and maturation. The expression of these genes in the cbb3 gene operon under iron-rich condition was 1.0∼3.1-fold of that compared to the iron-limited conditions (Figure 3B).
Figure 3. Genes encoding respiratory chain complex bc1 and cbb3 in response to iron availability. (A) The scheme of genome organization in the bc1 operon, with heat map of the cytochrome bc1 gene expression under the low iron and high iron conditions. (B) The scheme of genome organization in the cbb3 operon, with heat map of the cytochrome cbb3 gene expression under the low iron and high iron conditions.
Expression of luciferase in SCH908 (Figure 4A) determined by luciferase reporter analysis was 13.9-fold and 20.8-fold higher in iron-low than that in iron-rich cultures at 22°C (Beck-Johnson et al., 2013) (represents the malaria transmission temperature in nature) and 37°C (represents the immediate midgut temperature after blood meals), respectively. Moreover, under iron-low conditions, the relative reporter activity in SCH908 cells cultured at 22°C was 29.1-fold higher than at 37°C; under iron-rich conditions, luciferase activity in SCH908 cells grown at 22°C was 43.2-fold higher than at 37°C. When SCH908 cells were introduced into female mosquitoes by the oral feeding route, luciferase activity measured from dissected guts of sugar-fed mosquitoes was 2.4-fold higher than it was from guts of blood-fed ones, indicating that this promoter was regulated by relative iron availability (Figure 4B). Our results (Figure 4C) further demonstrated that SCH908 cell density in blood-fed A. stephensi mosquitoes was 3.3-fold higher than that in the mosquitoes fed with sugar meal (P < 0.05), suggesting E. anophelis utilized iron and/or other nutrients from animal blood cells for fast growth in insect host.
Figure 4. Response of hemolysin genes to temperature, iron stress and diet changes in mosquitoes. (A) Effects of iron and temperature on hemolysin gene expression by E. anophelis in vitro. The high or low temperature was 37 or 22°C, respectively. High-iron medium was established with 12 μM of iron (final concentration), while the low iron medium was established with 200 mM of 2,2-dipyridyl (final concentration). Values are means ± standard deviation. (B) Comparison of hemolysin gene expression in E. anophelis when mosquitoes were fed sugar and blood meals. A. stephensi were fed with 10% sucrose supplemented with E. anophelis for 24 h (NanoLuc reporter strain). For blood meals, mosquitoes were fed bovine blood through a membrane. Values are means ± standard deviation. (C) Density of E. anophelis in mosquitoes given a blood meal or sugar meal. Cell growth was expressed relative to that measured with sugar meal as 100%. Values are means ± standard deviation. Differences were significant at P < 0.05.
E. anophelis produced alpha-hemolysin on blood agar (Supplementary Figure S3A), which was consistent with previous observations (Kukutla et al., 2014). Visual inspection of liquid culture with or without E. anophelis Ag1 cells showed less heme color in the former condition, indicating heme utilization by cells (Supplementary Figure S3B). Under conditions with E. anophelis Ag1 cells, 14% and 34% of the initial erythrocytes (day 0) were disrupted after 2- and 4-day incubation in vitro, respectively. By contrast, in cell-free controls erythrocyte density decreased by 5% and 15% of initial RBC count, respectively (Figure 5A), which was significantly lower than that with the supplement of E. anophelis Ag1 cells (P < 0.05). In female mosquitoes not treated with erythromycin or treated with it but after having been fed E. anophelis Ag1 via a sugar meal, fecundity averaged 30 eggs per A. stephensi (Figure 5B). By contrast, there was an average of 60 eggs per A. stephensi after having been fed E. anophelis Ag1 in a sugar meal, but not given erythromycin, indicating that E. anophelis Ag1 contributed to host fecundity (Figure 5B).
Figure 5. Effects of added E. anophelis cells on red blood cell lysis and mosquito fecundity. (A) Time course of the digestion of red blood cells by E. anophelis. Values are means ± standard deviation. Comparisons that were significantly different (P < 0.05) were concentrations of red blood cells incubated without E. anophelis addition on day 2 and day 4 versus day 0 or incubated with E. anophelis on day 2 and day 4 versus day 0, respectively. (B) Fecundity of A. stephensi after a bovine blood meal given by membrane feeding. Comparisons that were significantly different (P < 0.05) were the eggs produced by mosquitoes previously provided E. anophelis in a sugar meal, E. anophelis in sugar meal supplemented with erythromycin versus sugar only (indicated by “a”), respectively.
Siderophore biosynthesis genes including iucA/iucC, iucB and iucD were significantly upregulated under iron-poor conditions; their expression level was respectively 53.6, 45.2 and 69.2-fold higher than that in cells held in iron-rich conditions (Supplementary Table S3). When the three genes ΔiucA_iucC/iucB/iucD were intact in wild type E. anophelis Ag1, the expected gene fragments were amplified in PCR with appropriate primers; when deleted, these gene fragments were absent, confirming successful construction of deletion mutants (Figures 6A,B). No significant siderophore production in WT was observed in cells grown in iron-rich conditions (Supplementary Figure S4). However, the blue CAS solution turned to an orange/brown color and the OD630nm decreased when exposed to filtered supernatants of E. anophelis grown in ABTGC media with no iron addition, demonstrating significant siderophore activity (Supplementary Figure S4). E. anophelis produced approximately 27 μM of siderophore when deferoxamine was used as an iron siderophore standard (Supplementary Figure S4). The supernatants of SCH1065 (ΔiucA_iucC/iucB/iucD) from the iron-replete culture had a similar OD630nm to the control (no inoculation) when mixed with CAS solution (Supplementary Figure S4), showing that the function of siderophore synthesis was impaired. The growth of SCH1065 (revealed by OD600nm) was comparable to the WT in the first 4 h under iron-replete conditions (Figure 6C), indicating that E. anophelis recycled intracellular iron or scavenged iron from the media using other iron uptake pathways (e.g., direct uptake of available Fe2+). However, growth stopped after 4-h incubation in SCH1065 cells when the culture medium was iron-poor. Under the same conditions, the WT cell density continued to increase and was significantly higher than the mutant cells after 8 h. When cultured under iron-rich conditions, both mutant and WT cells grew to stationary phase at 8 h. However, the final cell density in the mutant was slightly lower than that in WT (Figure 6C).
Figure 6. Deletion of the siderophore synthesis gene cluster (ΔiucA_iucC/iucB/iucD) led to impaired growth under iron stress conditions. (A) Organization of the iucA/iucC, iucB and iucD gene cluster was shown as the wide solid arrows (up panel). The locations and amplification directions of the primers were indicated using the thin arrows (low panel). hp, hypothetic protein; ddc, putative L-2,4-diaminobutyrate decarboxylase. (B) Detection of gene fragments of iucA/iucC, iucB and iucD genes in the WT and the mutant. Lane M, molecular marker. WT, wild-type; Mut, mutant. (C) The growth curve of WT and siderophore synthesis mutant in high-iron and low iron media. WT−, wild-type grown in the low iron media; Mut−, mutant grown in the low iron media; WT+, wild-type grown in the high-iron media; Mut+, mutant grown in the high-iron media.
When wild-type cells were cultured in LB broth, only 30% of the original cells were viable after treatment with 20 μM of H2O2 for 20 min (Figure 7A). By contrast, 60% viability was observed when cells cultured in LB media with the addition of FeCl3 or hemin were exposed to H2O2 (Figure 7A), showing that the preloaded cellular iron is critical for bacterial tolerance of the H2O2 toxicity. Cells grown in hemoglobin had an even better protection from H2O2 toxicity with a bacterial surviving rate of up to 80% (Figure 7A). Nearly all (97.5%) of cells grown under iron-poor conditions died, indicating that iron-depleted cells were highly susceptible to H2O2 toxicity (Figure 7A). Further, only 1.1% of the original cells were retained when the siderophore mutant was grown in LB media (Figure 7B). We were only able to recover 0.037% of the original cells when the mutant was cultured in iron-poor medium, showing that cells without efficient uptake of iron were more susceptible to H2O2 than WT (Figure 7B). Incubation of mutants with hemin, Fe3+ or hemoglobin retained at least 0.2, 4, and 3.3% of the original cells without H2O2 treatment, indicating that iron is important for protection from H2O2 toxicity (Figure 7B).
Figure 7. Deficiency in siderophore synthesis was susceptible to H2O2 damage and led to the attenuated biofilm formation. (A) The survival rate of WT cells in low iron media (LB added with 2′2-dipyridyl), LB media, high iron media, and LB media supplemented with hemoglobin. (B) The survival rate of mutants in low iron media (LB added with 2′2-dipyridyl), LB media, high iron media, and LB media supplemented with hemoglobin. (C) Biofilm formation of the WT and mutant cells grown in the low iron and high iron media. Values are means ± standard deviation. Asterisks indicate there was a significant difference (P < 0.05).
Biofilm formation in mutant SCH1065 was only 71.8% of the WT when grown in iron-depleted media (Figure 7C). However, the deficiency in the siderophore synthesis (Figure 7C) did not affect biofilm formation if the cultures (WT and mutant) were grown in iron-replete media (P > 0.05).
The female mosquito gut is characterized by slightly acidic pH with a tendency to alkalinity after blood meals, a small volume, and frequent nutritional fluctuations (Nepomuceno et al., 2017). Iron availability contributes importantly to the assemblage of microorganisms in the mosquito midgut (Wang et al., 2011; Chen et al., 2016; Champion and Xu, 2017). Bacterial inhabitants of the adult mosquito midgut experience extreme conditions with relationship to iron availability, either having insufficient supply prior to and between blood meals, or excessive free iron and associated free radicals during and after blood meals (Champion and Xu, 2017; Daschner et al., 2017). Our results showed that E. anophelis, a commensal organism of the mosquito midgut, responds to these extreme conditions and utilizes multiple iron uptake pathways, TonB-dependent transporters, and iron chelating proteins in response to iron stress. We further demonstrated that siderophores are crucial for iron uptake, alleviation of oxidative damage by H2O2, and biofilm formation. E. anophelis facilitated RBC lysis with several hemolysins, which possibly contributed to the mosquito’s fecundity. Collectively, our results provide new insights into the colonization and survival mechanisms for this predominant commensal bacterium in the mosquito gut.
A global gene response occurred in response to iron stress in E. anophelis and involved least 9% of the total transcribed genes. In particular, the expression level of several genes involved in “the respiratory chain complex” was notably affected by iron availability. Bacteria utilize several iron-storage proteins (DNA binding protein from starved cells, ferritins and/or bacterioferritin) to remove excessive iron that produces radicals and the oxidative damage inside the cells (Andrews, 1998; Chasteen and Harrison, 1999; Carrondo, 2003). However, de novo synthesis of iron storage proteins is too slow and energy-consuming to manage a sudden, high concentration of free iron (Andrews, 1998). The expression of iron storage protein genes is frequently detected during stationary growth phase (regulated by transcription sigma factor σ54) (Crosa, 1997; Carrondo, 2003). Incorporating excessive free iron into proteins/enzymes may be a better strategy than de novo synthesis of iron storage proteins, when a sudden high load of iron is encountered, because it allows bacteria to remove reactive oxygen radicals quickly, minimizing damage to the cell (Tsou et al., 2008; Tu et al., 2012; Toukoki and Gryllos, 2013). Among the iron-containing proteins (using iron as cofactors), the ETC components are good candidates because they utilize iron/heme as the cofactors and are highly demanded to maintain the cell metabolism (Durham and Millett, 2011). Besides the electron transport chain (ETC) components, expression of genes involved in TCA cycle and/or Fe-S protein synthesis was observed to be significantly elevated in the high iron cells. Liu et al. (2017) also demonstrated that the transcription level of ETC genes was significantly higher (up to 5-fold) in iron-rich cells than that in iron-poor cells in R. anatipestifer CH-1 (Liu et al., 2017). The transcription level of genes encoding ETC component proteins (such as cytochrome-c peroxidase, cytochrome c oxidase subunit CcoP and cytochrome c oxidase subunit CcoN) was remarkably lower in H2O2-treated cells than in untreated E. anophelis (Li et al., 2015). E. anophelis dramatically decreased iron uptake, likely to avoid more intracellular reactive oxygen species (ROS) production when high oxidative stress (presented here experimentally by H2O2 treatment) is encountered in the environment (Li et al., 2015). Therefore, the rapid conversion of toxic free iron into non-toxic forms, and minimizing iron uptake, substantially contributes to cellular tolerance to the high oxidative stress.
Hemolysin production has been widely reported in many pathogens (Portnoy et al., 1988; Simi et al., 2003; Chen et al., 2017a, b). E. anophelis produced α-hemolysin(s) to facilitate digestion of animal erythrocytes in vitro. Restriction of environmental iron increased hemolysin gene transcription, demonstrating that they were actively involved in iron metabolism. Once erythrocytes are lysed, iron/heme becomes available in the mosquito midgut (Gaio Ade et al., 2011). In our experiments, E. anophelis dramatically lowered hemolysin gene expression, perhaps to avoid excessive hemoglobin release. By contrast, in S. marcescens, expression of hemolysin genes greatly increased when iron in the medium was limited (Pramanik et al., 2014). In Yersinia ruckeri, the promoter activity of these genes was regulated by both iron concentration and temperature (Fernández et al., 2007). Infection was more efficient at low temperature (18°C), which induced higher expression of hemolysin, protease (Yrp1), ruckerbactin and other toxin genes than at elevated temperature (37°C) (Fernández et al., 2007). Our results demonstrate that expression of hemolysin genes was remarkably depressed by increasing environmental temperature though the bacteria grow faster at 37°C. For Elizabethkingia, the temperature-dependent modulation of hemolysin in the blood feeding course may be similar to the scenario encountered by the Y. ruckeri in the blood stream (Fernández et al., 2007). When the female mosquito ingests blood cells from warm-blooded animals, the midgut epithelial cells respond with rapid expression of heat shock proteins (Benoit et al., 2011). The higher temperature could be a signal for Elizabethkingia in the midgut to decrease hemolysin synthesis. However, further experiments are warranted to test this hypothesis.
Supplementation of E. anophelis in the diet of adult female A. stephensi increased fecundity. Gaio Ade et al. (2011) investigated the effects of the gut bacteria on the blood digestion and egg production in A. aegypti (Gaio Ade et al., 2011). Elimination of the gut bacteria slowed digestion of erythrocyte protein components (Gaio Ade et al., 2011). It is well established that blood components induce release of insulin-like peptides (ILPs) and ovary ecdysteroidogenic hormone in mosquitoes and stimulate ecdysone production (Gulia-Nuss et al., 2015; Vogel et al., 2015; Coon et al., 2016). Mosquitoes require ecdysone and ILPs to produce yolk proteins which are incorporated into primary oocytes forming mature eggs (Gulia-Nuss et al., 2015; Vogel et al., 2015). Consequently, retarded release of essential nutrients involved in the vitellogenic cycle due to the removal of gut microbiota possibly affected oocyte maturation, resulting in the production of less viable eggs (Gulia-Nuss et al., 2015; Vogel et al., 2015). To efficiently digest proteins from whole blood cells, the anautogenous mosquito hosts (such as A. aegypti and A. stephensi) and their associated gut bacteria are required to work in synergism (Coon et al., 2016).
When mosquitoes are only fed a sugar meal, Elizabethkingia may access ferrous iron through ferrous transporters, ferric iron using siderophores, and other iron sources by exosiderophore (i.e., ferrichrome, see below) in the gut. Due to the aerobic environment in mosquito gut, ferric iron may be one of the prevalent iron species in sugar-fed mosquito. However, the bioavailability of ferric iron in water is extremely low (about 10–17 M) (Ilbert and Bonnefoy, 2013). Secretion of efficient siderophores (such as aerobactin) from the mosquito-associated bacteria is important for competing for the very limited iron resource in the mosquito midgut (Li et al., 2015). The siderophore synthesis deficient mutants were particularly vulnerable to oxidative damage, indicating that siderophore production in E. anophelis can protect the cells from H2O2 damage (Li et al., 2015). The ability to remove free radicals is seriously compromised if hosts cannot produce iron-containing peroxidases and catalases. As an opportunistic and emerging pathogen, Elizabethkingia may utilize aerobactin-like siderophore(s) as an important virulence factor. Among the different siderophores (i.e., aerobactin, salmochelin, enterobactin and yersiniabactin), aerobactin was one of the most important virulence factors in systemic infections (Russo et al., 2015).
Hemin, Fe3+ or hemoglobin (Hgb) attenuated cytotoxicity by H2O2 (Li et al., 2015). Addition of hemoglobin to E. anophelis NUHP1 significantly enhanced H2O2 resistance (Li et al., 2015). Exposure to H2O2 accumulates ROS and nitric oxide (NO), decreases mitochondrial membrane potential, and triggers caspase-3/7 activity in vertebrates (Espinosa-Diez et al., 2015). Hemoglobin remarkably attenuated the overproduction of ROS and NO, reverted mitochondrial membrane potential, and repressed caspase-3/7 (Espinosa-Diez et al., 2015). It is possible that the hemin/iron from degraded hemoglobin can immediately be transported into cells and incorporated to some of the heme-containing antioxidative enzymes (such as heme-containing catalases) when bacteria suffer the crisis of strong reactive oxygen radicals (Champion and Xu, 2017; Whiten et al., 2018). Similarly, some of the non-heme proteins (with iron as the cofactor) can remove the ROS (Whiten et al., 2018). Despite that the current transcriptomic study was carried out in vitro, the recent meta-transcriptomic data showed that a large number of gene transcripts (involved in iron metabolism) from Elizabethkingia were discovered in mosquito midgut after the blood meal (Sharma et al., 2020). Our observations here and others indicated that Elizabethkingia played an important role in iron metabolism and oxidative stress management.
Iron availability impacted biofilm formation early in its development (Oliveira et al., 2017). In this study, the siderophore-deficient mutant formed less biofilm than the WT under iron-depleted conditions. Iron-responsive genes such as siderophore synthesis and iron uptake genes were strongly induced by biofilm formation rather than by planktonic growth in Mycobacterium smegmatis (Ojha and Hatfull, 2007). Further, deficiency in the exochelin biosynthesis or uptake systems led to poor biofilm formation, the viability and cultivability of biofilm cells under iron-limiting conditions (Ojha and Hatfull, 2007; Oliveira et al., 2017). Biofilm formation in vitro often led to weaker ability to attach to animal cells in Elizabethkingia. Thus, our observations here indicate that siderophore synthesis is also important for successful colonization in mosquitoes.
Environmental stress (e.g., pH and temperature), immune defense, as well as nutritional variations for commensal Elizabethkingia in mosquitoes with sudden blood meals are similar to those invading the bloodstream (Chen et al., 2015, 2016). Moreover, genome contexts and gene sequences between the mosquito-associated E. anophelis and clinical isolates are conserved (Kukutla et al., 2014; Li et al., 2015; Lin et al., 2017; Perrin et al., 2017). Thus, the investigation of the molecular mechanisms of the iron metabolism in mosquito-associated E. anophelis provides the insights into the pathogenesis progress in Elizabethkingia species and related bacteria.
The datasets generated in this study are publicly available in the NCBI Gene Expression Omnibus, BioProject, and The Sequence Read Archive, under accession numbers GSE132933, PRJNA549490, and SRP201789 respectively.
SC and EW conceived the study and participated in its design and coordination, and wrote the manuscript. SC and BN performed the experiments. SC, BJ, and TY conducted the transcriptomics analysis. All authors have read and approved the manuscript.
This project was funded by NIH grant R37AI21884.
The authors declare that the research was conducted in the absence of any commercial or financial relationships that could be construed as a potential conflict of interest.
The authors thank Dr. Mark McBride at University of Wisconsin-Milwaukee and Dr. Jiannong Xu at the New Mexico State University for the plasmid pYT313 and the strain E. anophelis Ag1. This manuscript has been released as a Pre-Print at Biorxiv.
The Supplementary Material for this article can be found online at: https://www.frontiersin.org/articles/10.3389/fmicb.2020.00804/full#supplementary-material
Akhouayri, I. G., Habtewold, T., and Christophides, G. K. (2013). Melanotic pathology and vertical transmission of the gut commensal Elizabethkingia meningoseptica in the major malaria vector Anopheles gambiae. PLoS One 8:e77619. doi: 10.1371/journal.pone.0077619
Anders, S., Pyl, P. T., and Huber, W. (2015). HTSeq—a Python framework to work with high-throughput sequencing data. Bioinformatics 31, 166–169. doi: 10.1093/bioinformatics/btu638
Andrews, S. C., Robinson, A. K., and Rodruez-Quines, F. (2003). Bacterial iron homeostasis. FEMS Microbiol. Rev. 27, 215–237.
Basson, A., Flemming, L. A., and Chenia, H. Y. (2008). Evaluation of adherence, hydrophobicity, aggregation, and biofilm development of Flavobacterium johnsoniae-like isolates. Microb. Ecol. 55, 1–14.
Beck-Johnson, L. M., Nelson, W. A., Paaijmans, K. P., Read, A. F., Thomas, M. B., and Bjørnstad, O. N. (2013). The effect of temperature on Anopheles mosquito population dynamics and the potential for malaria transmission. PLoS One 8:e79276. doi: 10.1371/journal.pone.0079276
Benoit, J. B., Lopez-Martinez, G., Patrick, K. R., Phillips, Z. P., Krause, T. B., and Denlinger, D. L. (2011). Drinking a hot blood meal elicits a protective heat shock response in mosquitoes. Proc. Natl. Acad. Sci. U.S.A. 108, 8026–8029. doi: 10.1073/pnas.1105195108
Bolger, A. M., Lohse, M., and Usadel, B. (2014). Trimmomatic: a flexible trimmer for Illumina sequence data. Bioinformatics 30, 2114–2120. doi: 10.1093/bioinformatics/btu170
Breurec, S., Criscuolo, A., Diancourt, L., Rendueles, O., Vandenbogaert, M., Passet, V., et al. (2016). Genomic epidemiology and global diversity of the emerging bacterial pathogen Elizabethkingia anophelis. Sci. Rep. 6:30379. doi: 10.1038/srep30379
Carrondo, M. A. (2003). Ferritins, iron uptake and storage from the bacterioferritin viewpoint. EMBO J. 22, 1959–1968.
Cescau, S., Cwerman, H., Létoffé, S., Delepelaire, P., Wandersman, C., and Biville, F. (2007). Heme acquisition by hemophores. Biometals 20:603.
Champion, C. J., and Xu, J. (2017). The impact of metagenomic interplay on the mosquito redox homeostasis. Free Radic. Biol. Med. 105, 79–85. doi: 10.1016/j.freeradbiomed.2016.11.031
Champion, C. J., and Xu, J. (2018). Redox state affects fecundity and insecticide susceptibility in Anopheles gambiae. Sci. Rep. 8:13054. doi: 10.1038/s41598-018-31360-2
Chasteen, N. D., and Harrison, P. M. (1999). Mineralization in ferritin: an efficient means of iron storage. J. Struct. Biol. 126, 182–194.
Chee-Fu, Y., Matthias, M., Liat, H. L., Han, Y. S., Chin, B. T., Phaik, K. L., et al. (2018). Elizabethkingia anophelis and association with tap water and handwashing, Singapore. Emerg. Infect. Dis. J. 24:1730. doi: 10.3201/eid2409.171843
Chen, S., Bagdasarian, M., and Walker, E. D. (2015). Elizabethkingia anophelis: molecular manipulation and interactions with mosquito hosts. Appl. Environ. Microbiol. 81, 2233–2243. doi: 10.1128/aem.03733-14
Chen, S., Bleam, W. F., and Hickey, W. J. (2010a). Molecular analysis of two bacterioferritin genes, bfrα and bfrβ, in the model rhizobacterium Pseudomonas putida KT2440. Appl. Environ. Microbiol. 76, 5335–5343.
Chen, S., Blom, J., Loch, T. P., Faisal, M., and Walker, E. D. (2017a). The emerging fish pathogen Flavobacterium spartansii isolated from chinook salmon: comparative genome analysis and molecular manipulation. Front. Microbiol. 8:2339. doi: 10.3389/fmicb.2017.02339
Chen, S., Blom, J., and Walker, E. D. (2017b). Genomic, physiologic, and symbiotic characterization of Serratia marcescens strains isolated from the mosquito Anopheles stephensi. Front. Microbiol. 8:1483. doi: 10.3389/fmicb.2017.01483
Chen, S., Kaufman, M. G., Bagdasarian, M., Bates, A. K., and Walker, E. D. (2010b). Development of an efficient expression system for Flavobacterium strains. Gene 458, 1–10.
Chen, S., Soehnlen, M., Downes, F. P., and Walker, E. D. (2017c). Insights from the draft genome into the pathogenicity of a clinical isolate of Elizabethkingia meningoseptica Em3. Stand. Genomic Sci. 12:56. doi: 10.1186/s40793-017-0269-8
Chen, S., Zhao, J., Joshi, D., Xi, Z., Norman, B., and Walker, E. D. (2016). Persistent infection by Wolbachia wAlbB has no effect on composition of the gut microbiota in adult female Anopheles stephensi. Front. Microbiol. 7:1485. doi: 10.3389/fmicb.2016.01485
Coon, K. L., Brown, M. R., and Strand, M. R. (2016). Gut bacteria differentially affect egg production in the anautogenous mosquito Aedes aegypti and facultatively autogenous mosquito Aedes atropalpus (Diptera: Culicidae). Parasit. Vect. 9:375. doi: 10.1186/s13071-016-1660-9
Crosa, J. H. (1997). Signal transduction and transcriptional and posttranscriptional control of iron-regulated genes in bacteria. Microbiol. Mol. Biol. Rev. 61, 319–336.
Daschner, P. J., Grisham, M. B., and Espey, M. G. (2017). Redox relationships in gut-microbiome interactions. Free Radic. Biol. Med. 105, 1–2.
DeJong, R. J., Miller, L. M., Molina-Cruz, A., Gupta, L., Kumar, S., and Barillas-Mury, C. (2007). Reactive oxygen species detoxification by catalase is a major determinant of fecundity in the mosquito Anopheles gambiae. Proc. Natl. Acad. Sci. U.S.A. 104, 2121–2126.
Durham, B., and Millett, F. S. (2011). Iron: Heme Proteins & Electron Transport, Encyclopedia of Inorganic and Bioinorganic Chemistry. Hoboken, NJ: John Wiley & Sons, Ltd.
Espinosa-Diez, C., Miguel, V., Mennerich, D., Kietzmann, T., Sánchez-Pérez, P., Cadenas, S., et al. (2015). Antioxidant responses and cellular adjustments to oxidative stress. Redox Biol. 6, 183–197.
Expert, D., Enard, C., and Masclaux, C. (1996). The role of iron in plant host-pathogen interactions. Trends Microbiol. 4, 232–237.
Fernández, L., Prieto, M., and Guijarro, J. A. (2007). The iron- and temperature-regulated haemolysin YhlA is a virulence factor of Yersinia ruckeri. Microbiology 153, 483–489. doi: 10.1099/mic.0.29284-0
Figueroa Castro, C. E., Johnson, C., Williams, M., VanDerSlik, A., Graham, M. B., Letzer, D., et al. (2017). Elizabethkingia anophelis: clinical experience of an academic health system in Southeastern Wisconsin. Open Forum Infect. Dis. 4:ofx251. doi: 10.1093/ofid/ofx251
Foster, W. A. (1995). Mosquito sugar feeding and reproductive energetics. Annu. Rev. Entomol. 40, 443–474.
Frawley, E. R., and Fang, F. C. (2014). The ins and outs of bacterial iron metabolism. Mol. Microbiol. 93, 609–616. doi: 10.1111/mmi.12709
Gaio Ade, O., Gusmão, D. S., Santos, A. V., Berbert-Molina, M. A., Pimenta, P. F., and Lemos, F. J. (2011). Contribution of midgut bacteria to blood digestion and egg production in Aedes aegypti (diptera: culicidae) (L.). Parasit. Vect. 4:105. doi: 10.1186/1756-3305-4-105
Gulia-Nuss, M., Elliot, A., Brown, M. R., and Strand, M. R. (2015). Multiple factors contribute to anautogenous reproduction by the mosquito Aedes aegypti. J. Insect Physiol. 82, 8–16. doi: 10.1016/j.jinsphys.2015.08.001
Ilbert, M., and Bonnefoy, V. (2013). Insight into the evolution of the iron oxidation pathways. Biochim. Biophys. Acta Bioenerget. 1827, 161–175. doi: 10.1016/j.bbabio.2012.10.001
Jacobs, A., and Chenia, H. Y. (2011). Biofilm formation and adherence characteristics of an Elizabethkingia meningoseptica isolate from Oreochromis mossambicus. Ann. Clin. Microbiol. Antimicrob. 10:16. doi: 10.1186/1476-0711-10-16
Kämpfer, P., Matthews, H., Glaeser, S. P., Martin, K., Lodders, N., and Faye, I. (2011). Elizabethkingia anophelis sp. nov., isolated from the midgut of the mosquito Anopheles gambiae. Int. J. Syst. Evol. Microbiol. 61, 2670–2675. doi: 10.1099/ijs.0.026393-0
Kukutla, P., Lindberg, B. G., Pei, D., Rayl, M., Yu, W., Steritz, M., et al. (2014). Insights from the genome annotation of Elizabethkingia anophelis from the malaria vector Anopheles gambiae. PLoS One 9:e0097715. doi: 10.1371/journal.pone.0097715
Lau, S. K. P., Chow, W.-N., Foo, C.-H., Curreem, S. O. T., Lo, G. C.-S., Teng, J. L. L., et al. (2016). Elizabethkingia anophelis bacteremia is associated with clinically significant infections and high mortality. Sci. Rep. 6:26045. doi: 10.1038/srep26045
Ledala, N., Zhang, B., Seravalli, J., Powers, R., and Somerville, G. A. (2014). Influence of iron and aeration on Staphylococcus aureus growth, metabolism, and transcription. J. Bacteriol. 196, 2178–2189. doi: 10.1128/JB.01475-14
Li, H., Handsaker, B., Wysoker, A., Fennell, T., Ruan, J., Homer, N., et al. (2009). The Sequence Alignment/Map format and SAMtools. Bioinformatics 25, 2078–2079. doi: 10.1093/bioinformatics/btp352
Li, Y., Liu, Y., Chew, S. C., Tay, M., Salido, M. M. S., Teo, J., et al. (2015). Complete genome sequence and transcriptomic analysis of the novel pathogen Elizabethkingia anophelis in response to oxidative stress. Genome Biol. Evol. 7, 1676–1685. doi: 10.1093/gbe/evv101
Lin, J.-N., Lai, C.-H., Yang, C.-H., Huang, Y.-H., and Lin, H.-H. (2017). Genomic features, phylogenetic relationships, and comparative genomics of Elizabethkingia anophelis strain EM361-97 isolated in Taiwan. Sci. Rep. 7:14317. doi: 10.1038/s41598-017-14841-8
Liu, M., Huang, M., Zhu, D., Wang, M., Jia, R., Chen, S., et al. (2017). Identifying the genes responsible for iron-limited condition in Riemerella anatipestifer CH-1 through RNA-Seq-Based analysis. BioMed Res. Int. 2017:8682057. doi: 10.1155/2017/8682057
Molina, M. A., Godoy, P., Ramos-Gonzalez, M. I., Munoz, N., Ramos, J. L., and Espinosa-Urgel, M. (2005). Role of iron and the TonB system in colonization of corn seeds and roots by Pseudomonas putida KT2440. Environ. Microbiol. 7, 443–449.
Nepomuceno, D. B., Santos, V. C., Araújo, R. N., Pereira, M. H., Sant’Anna, M. R., Moreira, L. A., et al. (2017). pH control in the midgut of Aedes aegypti under different nutritional conditions. J. Exp. Biol. 220, 3355–3362. doi: 10.1242/jeb.158956
Ojha, A., and Hatfull, G. F. (2007). The role of iron in Mycobacterium smegmatis biofilm formation: the exochelin siderophore is essential in limiting iron conditions for biofilm formation but not for planktonic growth. Mol. Microbiol. 66, 468–483.
Oliveira, F., França, Â, and Cerca, N. (2017). Staphylococcus epidermidis is largely dependent on iron availability to form biofilms. Int. J. Med. Microbiol. 307, 552–563.
Oliveira, J., Goncalves, R., Lara, F., Dias, F., Gandara, A., Menna-Barreto, R., et al. (2011). Blood meal-derived heme decreases ROS levels in the midgut of Aedes aegypti and allows proliferation of intestinal microbiota. PLoS Pathog. 7:e1001320. doi: 10.1371/journal.ppat.1001320
Pei, D., Nicholson, A. C., Jiang, J., Chen, H., Whitney, A. M., Villarma, A., et al. (2017). Complete circularized genome sequences of four strains of Elizabethkingia anophelis, including two novel strains isolated from wild-caught Anopheles sinensis. Genome Announc. 5:e01359-17.
Perrin, A., Larsonneur, E., Nicholson, A. C., Edwards, D. J., Gundlach, K. M., Whitney, A. M., et al. (2017). Evolutionary dynamics and genomic features of the Elizabethkingia anophelis 2015 to 2016 Wisconsin outbreak strain. Nat. Commun. 8:15483. doi: 10.1038/ncomms15483
Portnoy, D. A., Jacks, P. S., and Hinrichs, D. J. (1988). Role of hemolysin for the intracellular growth of Listeria monocytogenes. J. Exp. Med. 167:1459.
Pramanik, A., Könninger, U., Selvam, A., and Braun, V. (2014). Secretion and activation of the Serratia marcescens hemolysin by structurally defined ShlB mutants. Int. J. Med. Microbiol. 304, 351–359. doi: 10.1016/j.ijmm.2013.11.021
Pumpuni, C. B., Demaio, J., Kent, M., Davis, J. R., and Beier, J. C. (1996). Bacterial population dynamics in three anopheline species: the impact on plasmodium sporogonic development. Am. J. Trop. Med. Hyg. 54, 214–218.
Robinson, M. D., McCarthy, D. J., and Smyth, G. K. (2010). edgeR: a Bioconductor package for differential expression analysis of digital gene expression data. Bioinformatics 26, 139–140. doi: 10.1093/bioinformatics/btp616
RStudio (2012). RStudio: Integrated development environment for R (Version 0.96.122) [Computer software]. Boston, MA: RStudio.
Russo, T. A., Olson, R., MacDonald, U., Beanan, J., and Davidson, B. A. (2015). Aerobactin, but not yersiniabactin, salmochelin, or enterobactin, enables the growth/survival of hypervirulent (Hypermucoviscous) Klebsiella pneumoniae ex vivo and in vivo. Infect. Immun. 83, 3325–3333. doi: 10.1128/IAI.00430-15
Sharma, P., Rani, J., Rawal, C., and Seena, Y. (2020). Altered gut microbiota and immunity defines Plasmodium vivax survival in Anopheles stephensi. Front. Immunol. (in press). doi: 10.3389/fimmu.2020.00609
Simi, S., Carbonell, G. V., Falcón, R. M., Gatti, M. S. V., Joazeiro, P. P., Darini, A. L., et al. (2003). A low molecular weight enterotoxic hemolysin from clinical Enterobacter cloacae. Can. J. Microbiol. 49, 479–482.
Sun, G., Wang, L., Bao, C., Li, T., Ma, L., and Chen, L. (2015). Complete genome sequence of Elizabethkingia meningoseptica, isolated from a T-cell non-Hodgkin’s lymphoma patient. Genome Announc. 3:e00673-15.
Teo, J., Tan, S. Y.-Y., Liu, Y., Tay, M., Ding, Y., Li, Y., et al. (2014). Comparative genomic analysis of malaria mosquito vector associated novel pathogen Elizabethkingia anophelis. Genome Biol. Evol. 6, 1158–1165. doi: 10.1093/gbe/evu1094
Terenius, O., Lindh, J. M., Eriksson-Gonzales, K., Bussière, L., Laugen, A. T., Bergquist, H., et al. (2012). Midgut bacterial dynamics in Aedes aegypti. FEMS Microbiol. Ecol. 80, 556–565. doi: 10.1111/j.1574-6941.2012.01317.x
Toukoki, C., and Gryllos, I. (2013). PolA1, a putative DNA polymerase I, is coexpressed with PerR and contributes to peroxide stress defenses of group A Streptococcus. J. Bacteriol. 195, 717–725. doi: 10.1128/JB.01847-12
Tsou, C.-C., Chiang-Ni, C., Lin, Y.-S., Chuang, W.-J., Lin, M.-T., Liu, C.-C., et al. (2008). An iron-binding protein, Dpr, decreases hydrogen peroxide stress and protects Streptococcus pyogenes against multiple stresses. Infect. Immun. 76, 4038–4045. doi: 10.1128/IAI.00477-08
Tu, W. Y., Pohl, S., Gray, J., Robinson, N. J., Harwood, C. R., and Waldron, K. J. (2012). Cellular iron distribution in Bacillus anthracis. J. Bacteriol. 194, 932–940. doi: 10.1128/JB.06195-11
Vogel, K. J., Brown, M. R., and Strand, M. R. (2015). Ovary ecdysteroidogenic hormone requires a receptor tyrosine kinase to activate egg formation in the mosquito Aedes aegypti. Proc. Natl. Acad. Sci. U.S.A. 112, 5057–5062. doi: 10.1073/pnas.1501814112
Wang, Y., Gilbreath, T. M., Kukutla, P., Yan, G., and Xu, J. (2011). Dynamic gut microbiome across life history of the malaria mosquito Anopheles gambiae in Kenya. PLoS One 6:e24767. doi: 10.21371/journal.pone.0024767
Whiten, S. R., Eggleston, H., and Adelman, Z. N. (2018). Ironing out the details: exploring the role of iron and heme in blood-sucking arthropods. Front. Physiol. 8:1134. doi: 10.3389/fphys.2017.01134
Zhang, C. (2014). Essential functions of iron-requiring proteins in DNA replication, repair and cell cycle control. Protein Cell 5, 750–760. doi: 10.1007/s13238-014-0083-7
Zhou, G., Kohlhepp, P., Geiser, D., Frasquillo, M. D. C., Vazquez-Moreno, L., and Winzerling, J. J. (2007). Fate of blood meal iron in mosquitoes. J. Insect. Physiol. 53, 1169–1178.
Keywords: Elizabethkingia, mosquito microbiota, iron, transcriptomics and genetics, physiology, oxidative stress
Citation: Chen S, Johnson BK, Yu T, Nelson BN and Walker ED (2020) Elizabethkingia anophelis: Physiologic and Transcriptomic Responses to Iron Stress. Front. Microbiol. 11:804. doi: 10.3389/fmicb.2020.00804
Received: 27 December 2019; Accepted: 03 April 2020;
Published: 07 May 2020.
Edited by:
Catherine Ayn Brissette, University of North Dakota, United StatesReviewed by:
Emily Derbyshire, Duke University, United StatesCopyright © 2020 Chen, Johnson, Yu, Nelson and Walker. This is an open-access article distributed under the terms of the Creative Commons Attribution License (CC BY). The use, distribution or reproduction in other forums is permitted, provided the original author(s) and the copyright owner(s) are credited and that the original publication in this journal is cited, in accordance with accepted academic practice. No use, distribution or reproduction is permitted which does not comply with these terms.
*Correspondence: Shicheng Chen, c2hpY2hlbmdAbXN1LmVkdQ==; Y2hlbnNoaWNoZW5nQGdtYWlsLmNvbQ==
Disclaimer: All claims expressed in this article are solely those of the authors and do not necessarily represent those of their affiliated organizations, or those of the publisher, the editors and the reviewers. Any product that may be evaluated in this article or claim that may be made by its manufacturer is not guaranteed or endorsed by the publisher.
Research integrity at Frontiers
Learn more about the work of our research integrity team to safeguard the quality of each article we publish.