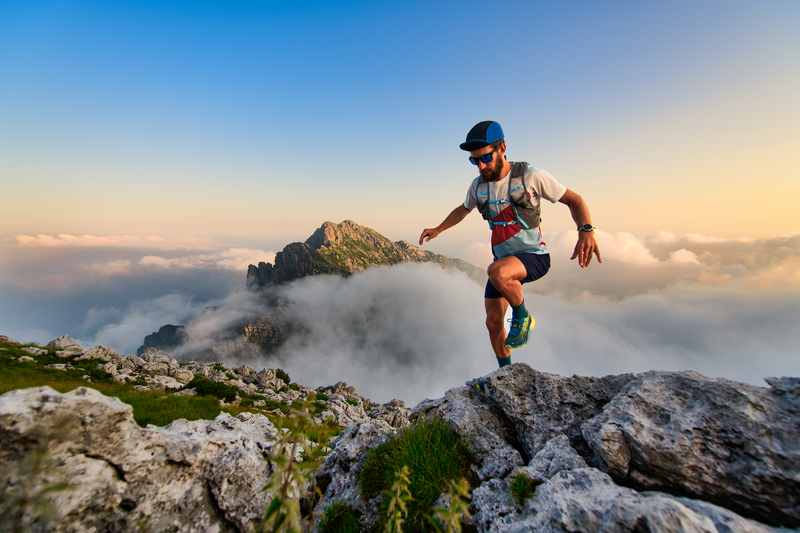
94% of researchers rate our articles as excellent or good
Learn more about the work of our research integrity team to safeguard the quality of each article we publish.
Find out more
REVIEW article
Front. Microbiol. , 29 April 2020
Sec. Infectious Agents and Disease
Volume 11 - 2020 | https://doi.org/10.3389/fmicb.2020.00435
Tuberculosis (TB) disease is an international health concern caused by the bacteria Mycobacterium tuberculosis (Mtb). Evolution of multi-drug-resistant strains may cause bacterial persistence, rendering existing antibiotics ineffective. Hence, development of new or repurposing of currently approved drugs to fight Mtb in combination with existing antibiotics is urgently needed to cure TB which is refractory to current therapy. The shortening of TB therapy and reduction in lung injury can be achieved using adjunctive host-directed therapies. There is a wide range of probable candidates which include numerous agents permitted for the treatment of other diseases. One potential candidate is metformin, a Food and Drug Administration (FDA)-approved drug used to treat type 2 diabetes mellitus (DM). However, there is a scarcity of evidence supporting the biological basis for the effect of metformin as a host-directed therapy for TB. This scoping review summarizes the current body of evidence and outlines scientific gaps that need to be addressed in determining the potential role of metformin as a host-directed therapy.
Tuberculosis (TB), although largely a curable disease, remains the leading cause of mortality globally, fueled by the unprecedented increase in anti-mycobacterial drug resistance. In 2018, the World Health Organization (WHO) reported 10 million people falling ill with active TB (World Health Organization, 2018). Of these, 558,000 were new cases resistant to rifampicin–a key drug in the current first-line treatment. Eighty-two percent of the rifampicin-resistant cases had multi-drug-resistant TB (MDR-TB), which includes, at a minimum, resistance to the first line drug isoniazid (World Health Organization, 2018). While the incidence of drug resistance is approximately 5% of total TB cases, 17% of TB deaths were due to drug-resistant strains (World Health Organization, 2018).
Treatment regimens for TB consist of combinations of anti-mycobacterial drugs aimed at eradicating infection while preventing the development of resistance and recurrent infection. Anti-TB regimens are lengthy and associated with high toxicity, driving poor treatment adherence and development of MDR and extensively drug-resistant (XDR) TB (Shenoi et al., 2009; Calver et al., 2010; Zumla et al., 2013; Millington, 2018). Current treatment regimens are pathogen-targeted strategies, which are able to be severely compromised by the development of drug resistance by the pathogen (Millington, 2018). Resistance in Mycobacterium tuberculosis (Mtb) has been attributed to the development of resistance-conferring mutations in genes that encode drug targets or drug activating enzymes (Gygli et al., 2017). Another phenomenon also exists which participates in the development of drug resistance. A study by Sharma et al. (2015) showed ion metabolism to play a crucial role in contributing to amikacin and kanamycin resistance (Sharma et al., 2015). Furthermore, proteomics and bioinformatics expression studies of drug-resistant Mtb have defined the overexpression of uncharacterized and hypothetical proteins to possibly counteract the effect of anti-TB drugs (Jiang et al., 2006; Sharma et al., 2010; Kumar et al., 2013; Sharma and Bisht, 2017). As a result, further exploitation is necessary for the advancement of novel therapeutic agents which can directly be targeted to a protein and/or a gene accountable for resistance (Sharma et al., 2016, 2017). In addition, antibiotic pressure induces a stress response in Mtb. This, in combination with the capability of bacteria to engage into a dormant state, creates a subpopulation of Mtb with decreased sensitivity to TB chemotherapy which preferentially targets dividing bacteria (Balaban et al., 2004; Lewis, 2007; Wakamoto et al., 2013; Gygli et al., 2017). Current therapeutic strategies include pretreatment drug susceptibility screening for only one anti-TB drug. Hence, the standard-of-care multidrug treatment regimens comprise drugs of uncertain efficacy.
In 1991, the “World Health Assembly Resolution” identified TB as a “major global health problem,” and several strategies such as the “WHO Directly Observed Treatment, Short-course (DOTS) strategy” and the “Stop TB strategy” have been implemented (Uplekar and Raviglione, 2015). Between 2000 and 2015, an estimated 49 million lives were saved, with a 22% drop in TB deaths (World Health Organization, 2016a). However, only one in five people needing MDR-TB treatment initiated such therapy (World Health Organization, 2016a). In May 2014, a new strategy and targets for TB prevention, the End TB Strategy, were set. The purpose of this strategy is to terminate the international TB epidemic, with the following targets compared to 2015: “90% reduction in number of TB deaths, 90% reduction in TB incidence rate, and 90% reduction in TB affected families facing unfortunate costs due to TB” by 2035 (Uplekar et al., 2015; World Health Organization, 2016b). The currently adopted End TB strategy, while ambitious, can only be realized through novel approaches in treating and preventing TB.
Host-directed therapy (HDT) provides a largely unexploited approach as adjunctive anti-TB therapy. Firstly, HDT may impair Mtb replication and survival by disrupting Mtb manipulation of macrophage pathways, thus rendering the bacteria more sensitive to host defenses (Hawn et al., 2015). The current search for novel therapeutics has focused on the use of repurposed drugs aimed at optimizing the host’s response against the mycobacterium (Sharma et al., 2017). HDT has been proposed as an adjuvant therapy for TB, to improve the efficacy of current treatment outcomes. One plausible resolution to the challenge of antibiotic resistance and non-replicating bacterial death is targeting the host as opposed to the pathogen (Rayasam and Balganesh, 2015) because it depends neither on bacterial division nor on the bacterial susceptibility to drugs. Metformin (MET) is a Food and Drug Administration (FDA)-approved drug, well-known for its glucose-lowering effect on type 2 diabetes (T2D) individuals (Foretz et al., 2014). A group of studies have reported the potential role of MET as an adjunctive therapy for TB (Singhal et al., 2014; Singhal and De Libero, 2017; Degner et al., 2018). However, relatively little is known on how MET modulates the cellular interaction between Mtb and macrophages. We therefore pursued to amalgamate the evidence base on MET as an adjunctive therapy for TB using a scoping study methodology to identify gaps to be attained in future research.
The Arksey and O’Malley framework guided this research (Arksey and O’Malley, 2005). The framework involves: (1) finding the research question; (2) finding relevant studies; (3) study choice; (4) recording the data; and (5) organizing, summarizing, and reporting the results.
We reviewed existing literature with the aim of exploring what is known about the cellular interaction of MET, specifically defining the role of MET in mediating host–pathogen defense. The primary research question was guided by the subsequent sub-research questions: (1) “What is identified in the literature?”; (2) “What are the research gaps?”; and (3) “What are future research desires?” The results from the scoping review will be used to answer the above questions, to enable a better understanding of the research gaps and to guide future work in determining the potential use of MET as HDT for TB.
An amended PICOS (Population/Problem, Intervention, Comparison, Outcomes and Study setting) framework was used to identify the suitability of the research question (Table 1). We used the PICOS framework to clearly define a well-focused research question for us to obtain appropriate resources and search for relevant evidence in the literature.
We included primary studies demonstrating clear empiric evidence published in peer-reviewed journals. Qualitative, quantitative, or mixed methods studies that addressed the research question from January 2007 to July 2019 were included. For this scoping review, English electronic academic databases, Google scholar, Ovid, PubMed, Science direct, Scopus, Web of science, and the WHO website, were reviewed. The search phrases included MET as HDT, MET and macrophages, and MET as adjunctive therapy.
A set of eligibility criteria for studies to be included was developed to warrant consistent study selection applicable to our research question. Studies that met the following inclusion criteria were selected:
Inclusion:
• Focus on MET as a possible HDT agent
• Studies evaluating the cellular interaction of MET with Mtb and macrophages
• Published from January 2007 to July 2019
• No language restriction provided that the articles can be translated to English
The following studies were excluded:
• Studies which do not focus on MET as a possible HDT agent
• Studies which do not focus on cellular interaction of MET with Mtb and/or macrophages
• Studies published before January 2007
• If the article cannot be translated to English
Relevant data were then extracted from articles that screened successfully.
As per the recommendations by Arksey and O’Malley (2005), data were extracted from eligible articles. Recording the data involved consolidating and understanding data as per key themes. A charting data form was generated to ensure that the most suitable variables and themes are included to answer the research question. The data extraction tool on excel was used to confirm applicable and resourceful data extraction of evidence associated with MET and HDT. Recording of the data included the use of excel spreadsheets for data capturing for each article and to ensure the extracted data were comparable among articles.
To increase consistency in reporting of results, we used the recommended three-step approach, i.e., exploring the data, writing of results, and applying sense to the results (Levac et al., 2010). Following data collection and abstraction, synopses were produced and collated for reporting purposes. Research gaps were recognized grounded on recommendations from “authors, key findings, gaps, and lack of evident research.”
Selected studies included in vitro, in vivo, and retrospective studies (Figure 1). MET as an adjunctive therapy was demonstrated by Singhal et al. (2014) and Lee et al. (2018) using an in vitro model and in a retrospective cohort study, respectively. The in vitro model showed MET’s ability to facilitate phagosome–lysosome fusion, reduce chronic inflammation in the lung, enhance immune response, and potentiate the effectiveness of standard TB drugs. In addition, the retrospective cohort demonstrated improved 2 months sputum culture conversion with adjunctive MET treatment, underscoring its potential utility in TB (Singhal et al., 2014; Lee et al., 2018). Conversely, Dutta et al. (2017) provided evidence that contradicted the above results. This group observed MET to have no significant effect on mice treated with MET in combination with the first-line TB regimen (Dutta et al., 2017).
Figure 1. Flowchart depicting the process of article selection for scoping study assessing the role of Metformin (MET) in tuberculosis.
Evidence showing the effect of MET on bacterial replication was reported in three studies. Authors concluded that MET controlled Mtb replication via different methods. Singhal et al. (2014) demonstrated that MET increased mitochondrial reactive species production which led to the growth control of intracellular drug-resistant Mtb strains; this facilitated phagosome–lysosome fusion in macrophages. Furthermore, MET promoted accumulation of CD8+ and CD4+ T cells in the lung autonomously of infection. Antimicrobial activity observed in two in silico systems (Vashisht et al., 2014; Vashisht and Brahmachari, 2015) (a) elucidated prospective molecular processes and pathways to circumvent Mtb persistence; (b) provided evidence that bacterial NDH-I complex is analogous to mitochondrial complex-I in structure and function (Vashisht et al., 2014; Vashisht and Brahmachari, 2015). Complex-I of the mitochondria is the first enzymatic complex in the respiratory chain, accountable for the generation and transport of electrons forming a proton gradient across the inner membrane of the mitochondria which drives the production of ATP. ATP is the main energy source for metabolic functions. Similarly, bacterial NDH-I is accountable for an assortment of functions such as respiration and cyclic electron flow (Yagi et al., 1998), making NDH-I a potential target to inhibit bacteria.
Two of the 10 studies reported the impact of MET on mortality during TB therapy. Degner et al. (2017) observed a decrease in mortality rate among diabetic TB patients receiving MET concomitantly with TB treatment. Additional findings from this study included reduced likelihood of remaining culture positive at 2 months and improved glycemic control. Degner et al. (2018) also reported reduced mortality rate during TB treatment among patients receiving both MET and TB treatment. Both studies concluded that MET is a potential adjunctive HDT for TB therapy.
Studies by Alisjahbana et al. (2007) and Singhal et al. (2014) observed reduced TB disease severity when MET was used with first line TB therapy. Alisjahbana et al. (2007) studied TB patients with and without diabetes mellitus (DM) and showed a greater likelihood of delayed smear and culture conversion and unfavorable outcomes among patients with both TB and T2D. In two retrospective cohort studies, Singhal et al. (2014) showed a statistically significant reduction in TB severity. Improved clinical outcomes and fewer pulmonary cavities were observed among TB patients receiving MET (Singhal et al., 2014). Of note, studies done in different population groups concur with findings of reduced mortality during TB treatment among T2D patients cotreated with MET (Degner et al., 2017, 2018). Benefits of MET were observed by Srujitha et al. (2017) who observed a protective role of MET against TB infection and showed that lack of glycemic control is a hazard for TB incidence. Additional benefits of MET such as reduced rates of TB infection (Lee et al., 2018) and increased sputum culture conversion in those with higher bacillary burden (Degner et al., 2018; Novita et al., 2019) have also been observed.
MET alters the amount and dispersal of circulating immune cells. Lachmandas et al. (2019) observed that following MET exposure, there was a temporary elevation in overall white blood cell and neutrophil counts (increase over 6 days with return to pre-MET treatment baseline by 21 days). The ratio of monocytes, the precursor cells for macrophages, to lymphocytes was increased in peripheral blood (Lachmandas et al., 2019). MET was observed to modulate the host’s innate immune response (Singhal et al., 2014; Lachmandas et al., 2019; Novita et al., 2019), including MET-induced increases in whole-blood reactive oxygen species (ROS) production and strong upregulation of genes responsible for ROS production (Lachmandas et al., 2019). Furthermore, Singhal et al. (2014) showed in vitro that MET restricts bacterial growth by increasing the production of ROS. ROS are known to promote naive T cell proliferation and play a critical role in modulating the development and effector functions of various T lymphocyte subsets (Yarosz and Chang, 2018). Arai et al. (2010) identified an underlying mechanism of MET inhibition of tumor necrosis factor (TNF) production and transcription factor expression by the ability of MET to inhibit TNF and tissue factor production with the addition of the stimulant lipopolysaccharide (LPS) or oxidized low-density lipoprotein (Lachmandas et al., 2019). Novita et al. (2019) measured levels of microtubule-associated Protein 1 light chain 3B (MAP1LC3B), superoxide dismutase (SOD), and interferon in a small group of 22 patients with both TB and T2D. This group observed an increased MAP1LC3B, SOD, and interferon before and after MET treatment (Novita et al., 2019). This is important since MAP1LC3B is a key protein representing autophagy; SOD is an important antioxidant that is also thought to enhance the bactericidal effects of isoniazid, and interferon-gamma levels are known to play an important role in autophagy (Novita et al., 2019). In two in silico system studies Vashisht et al. identified metabolic mechanisms related to NAD biosynthesis during Mtb infection and observed directive detouring of metabolic fluidities via NAD biosynthesis pathway (Vashisht et al., 2014; Vashisht and Brahmachari, 2015). Pathogens possess the ability to hijack enzymes responsible for maintaining the NAD+ homeostasis of the host this leads to a metabolic dysfunction allowing for the survival and persistence of the pathogen (Grady et al., 2012; Imai, 2016; Mesquita et al., 2016; Matalonga et al., 2017). Dissimilar classes of pathogens have been reported to cause fluctuations in NAD+ levels within infected cells. Variations of NAD+ levels are projected to disturb effector functions of immune cells and, subsequently, disturb the clearance or perseverance of infections. NAD+ levels are largely reliant on the enzyme IDO and the “NAD+-consuming enzymes SIRTs, PARPs, and CD38” (Grady et al., 2012; Imai, 2016; Mesquita et al., 2016; Matalonga et al., 2017). These catalysts play imperative roles in “energy metabolism, cell survival, proliferation and effector functions,” rendering these enzymes as probable targets for HDT.
Reviewed published literature supports a biologic basis for the use of MET as HDT for Mtb infection (Alisjahbana et al., 2007). Evidence supporting MET use as adjunctive therapy for TB was observed in vitro, in vivo, in retrospective, and in modeling studies. Taken collectively, this review suggests that MET has the potential to improve existing shortfalls of current TB therapy (Figure 2). Metformin was first approved by the US FDA in 1994 for DM and insulin resistance and is incorporated within the “WHO’s List of Essential Medicines,” as it is regarded among the most effective and safe medicines needed within health systems (Crofford, 1995; World Health Organization, 2013). Experiments conducted in preclinical models aimed at assessing the biologic efficacy of MET in TB remain warranted, as repurposing of MET for TB is being explored (World Health Organization, 2013). It is well-established that HDT agents produce effects on multiple host targets both related and unrelated to a specific pathway. Hence, HDT studies in TB need to first establish the mechanism, i.e., the specific molecular and/or cellular target within specific host immune response pathway for TB. The development of MET as a potential HDT for TB can be accelerated by identifying biomarkers that specify immediate effects on these targets in vivo. Despite the absence of HDT-specific biomarkers, traditional treatment response markers such as clinical response, radiologic improvement, and microbiologic clearance of TB remain potential surrogate markers of anti-TB HDT efficacy.
Figure 2. The effect of metformin on macrophage function and clinical outcomes. Studies labeled A, C, F, G, H, I, and J represent the effect of metformin on respective clinical outcomes. These include reduced mortality, 2-month culture conversion and toxicity, and improved glycemic control. Studies B and L: outcome 1 show the effect of metformin on tumor necrosis factor (TNF)-α and interferon γ. TNF-α acts together with interferon γ, causing the production of reactive nitrogen intermediates and facilitating the tuberculostatic function of macrophages and the migration of immune cells to the infection site, contributing to granuloma formation. Study C shows metformin to increase macrophage reactive oxygen species (ROS) production. Evidence suggests that macrophage-produced ROS is responsible for increasing macrophage microbicidal activity by directly killing bacteria (Tan et al., 2016). This study is not part of the inclusion criteria. Study K observes metformin increases superoxide dismutase (SOD) and microtubule-associated proteins 1A/1B light chain 3B (MAP1LC3B). Mycobacterium tuberculosis (Mtb) binds the pattern recognition receptor (PRR) on the macrophage which initiates phagocytosis. Superoxide dismutase (SOD) (produced as a by-product of oxygen metabolism within cells) enables clearance of bacteria and restricts inflammation in response to infection by encouraging bacterial phagocytosis, and MAP1LC3B is representative of autophagy while SOD induces autophagy. Study L: outcome 2 shows metformin to increase lactate production within cells. Lactate is formed in large quantities by innate immune cells during inflammatory activation. Lactate modulates the immune cell metabolism which translates to decreased inflammation and ultimately functions as a negative feedback signal to avoid unwarranted inflammatory responses. Study L: outcome 3 observed a macrophage-targeting mechanism for the anti-inflammatory effects of metformin via polarization.
The evaluation of MET during 2-month culture conversion, illness, and death exhibited that diabetes independently presents an amplified risk of death during TB therapy (Alisjahbana et al., 2007; Degner et al., 2017, 2018; Srujitha et al., 2017). Age, cancer, and chronic kidney disease are associated with increased odds of mortality and morbidity during TB treatment (Alisjahbana et al., 2007; Degner et al., 2017, 2018). Upon secondary analysis, the odds of mortality were decreased with concurrent MET use among T2D patients; this was accompanied by a “positive correlation” of only one and/or interim exposure of MET to extended “immunity against TB infection” (Degner et al., 2018). MET was also safe for T2D-TB patients showing no increase in lactate levels, suggesting a potential adjunctive role of MET for TB-DM comorbidity.
Direct modulation of the host immune response by TB HDT agents may change the relationship between infection and swelling by changing the anticipating importance of the host-directed response in TB disease. Thus, advancement of TB HDT agents may require interchange evaluations of irritation, tissue harm, defensive invulnerability, and general antimicrobial adequacy. Compelling evidence using MET as an adjunctive agent to a single anti-tubercular drug showed restricted intracellular growth of Mtb within the human macrophage cell line (Singhal et al., 2014). In this study, MET was found to mediate intracellular phagosome–lysosome fusion and induce autophagy of Mtb-infected macrophages. Interestingly, blockade of these two mechanisms did not alter MET-mediated inhibition of bacterial growth (Singhal et al., 2014). Additional studies also show that in the presence of MET, there is evidence of increased macrophage autophagy leading to killing or containment of Mtb, evidence of control of inflammation seen by elevated TNF-α, interferon-γ, and interleukin-β and initiation of innate and adaptive responses within the host (Arai et al., 2010; Lachmandas et al., 2019). Extreme pro-inflammatory reactions are troublesome as they bring about broad tissue harm before the improvement of Mtb-specific adaptive immunity (Singhal et al., 2014). Adjunctive treatment with MET was found to lessen the tissue pathology and to advance immune responses (Singhal et al., 2014). In silico analysis provides potential insights into the antimicrobial activity of MET. These analyses found direct “re-routing of metabolic fluxes” via “NAD biosynthesis pathway” and NDH-1 in Mtb, thereby presenting a probable alternate mechanism for ATP production, supporting persistence of bacilli (Vashisht et al., 2014). Interestingly, utilizing the mechanical and functional comparisons between NDH-1 of the bacteria and complex-1 of the mitochondria, accompanied by its effect on the host complex-1 supports MET’s role in also inhibiting Mtb NDH-1, suggesting a potential mechanism of impact of MET on Mtb persisters (Vashisht and Brahmachari, 2015). These studies all suggest potential effectiveness of MET as HDT for TB and underscore the need for further research aimed at establishing how MET elicits these responses.
Pilot preclinical and observational research recommends the application of MET as an adjunctive, host-directed agent for TB therapy. As previously shown in a retrospective analysis of 220 individuals with DM and TB, MET dosed at 250 mg/kg enhanced the efficacy of conventional anti-TB drugs (Singhal et al., 2014). This was also related to reduced TB severity and enhanced clinical outcomes (Singhal et al., 2014). Another retrospective study showed MET to be related to a 3.9-fold decrease in TB incidence with DM patients (Srujitha et al., 2017). Following these deductions, this preclinical study theorized that MET adjunct therapy would supplement the “bactericidal and sterilizing” activities of conventional therapy (Cohn et al., 1990; Ahmad et al., 2010) against chronic TB infection in mice and reduce the period of therapeutic treatment evaluated by decreased microbiological decline (Dutta et al., 2017). However, the study found that MET did not demonstrate activity in mice with chronic TB infection. During therapy, mice treated with MET showed no obvious toxicity (Dutta et al., 2017), but a trend toward enhanced bactericidal activity in the MET group was observed through the “continuation phase” of treatment (Dutta et al., 2017).
Several favorable conclusions have emerged from clinical and experimental studies that suggest improved TB treatment outcomes with concurrent MET use (Tables 2, 3). TB disease progression is extremely influenced by the Mtb–host interaction, thus the modulation of cellular host defenses may have a significant contribution in containment of Mtb infection. Modulation of biological procedures such as “inflammatory responses, signaling, metabolism or cellular procedures” like autophagy have arose as hopeful targets for HDT. To define the definitive role of MET as adjunctive TB therapy, an assessment of the effect of MET on the ability of macrophages to control intracellular Mtb needs to be established. This needs to be supported by further assessing the additive effect of MET on the ability of macrophages to control intracellular Mtb in the presence of TB therapy. Major implications of MET HDT would assist in shortening TB treatment, improving TB treatment outcomes and early host eradication of TB infection with potential to reduce TB transmission. All the studies and/or trials published so far are retrospective in nature. The establishment of a role for MET HDT in TB control would initiate a well-designed prospective randomized human study in well-defined populations.
Table 2. In vitro, in vivo, prospective, and retrospective studies evaluating the role of metformin in TB: 2007–2019.
NN developed the research question, collected and analyzed the data, and compiled the review. KN and AS provided comments and alterations to the study design and proofread the review.
This review manuscript was supported by the Strategic Health Innovation Partnerships (SHIP) Unit of the South African Medical Research Council.
The authors declare that the research was conducted in the absence of any commercial or financial relationships that could be construed as a potential conflict of interest.
Ahmad, Z., Nuermberger, E. L., Tasneen, R., Pinn, M. L., Williams, K. N., Peloquin, C. A., et al. (2010). Comparison of the ‘Denver regimen’ against acute tuberculosis in the mouse and guinea pig. J. Antimicrob. Chemother. 65, 729–734. doi: 10.1093/jac/dkq007
Alisjahbana, B. E., Sahiratmadja, E. J., Nelwan, A. M., Purwa, Y., Ahmad, T. H., Ottenhoff, R. H., et al. (2007). The effect of type 2 diabetes mellitus on the presentation and treatment response of pulmonary tuberculosis. Clin. Infect. Dis. 45, 428–435. doi: 10.1086/519841
Arai, M., Uchiba, M., Komura, H., Mizuochi, Y., Harada, N., and Okajima, K. (2010). Metformin, an antidiabetic agent, suppresses the production of tumor necrosis factor and tissue factor by inhibiting early growth response factor-1 expression in human monocytes in Vitro. J. Pharmacol. Exp. Ther. 334, 206–213. doi: 10.1124/jpet.109.164970
Arksey, H., and O’Malley, L. (2005). Scoping studies: towards a methodological framework. Int. J. Soc. Res. Methodol. 8, 19–32. doi: 10.1080/1364557032000119616
Balaban, N. Q., Merrin, J., Chait, R., Kowalik, L., and Leibler, S. (2004). Bacterial persistence as a phenotypic switch. Science 305, 1622–1625. doi: 10.1126/science.1099390
Calver, A. D., Falmer, A. A., Murray, M., Strauss, O. J., Streicher, E. M., Hanekom, M., et al. (2010). Emergence of increased resistance and extensively drug-resistant tuberculosis despite treatment adherence, South Africa. Emerg. Infect. Dis. 16, 264–271. doi: 10.3201/eid1602.090968
Cohn, D. L., Catlin, B. J., Peterson, K. L., Judson, F. N., and Sbarbaro, J. A. (1990). A 62-dose, 6-month therapy for pulmonary and extrapulmonary tuberculosis. A twice-weekly, directly observed, and cost-effective regimen. Ann. Intern. Med. 112, 407–415.
Degner, N. R., Wang, J.-Y., Golub, J. E., and Karakousis, P. C. (2017). The effect of diabetes and comorbidities on tuberculosis treatment outcomes. A62. Treatment of tuberculosis. Am. Thorac. Soc. 195:A7587.
Degner, N. R., Wang, J. Y., Golub, J. E., and Karakousis, P. C. (2018). Metformin use reverses the increased mortality associated with diabetes mellitus during tuberculosis treatment. Clin. Infect. Dis. 66, 198–205. doi: 10.1093/cid/cix819
Dutta, N. K., Pinn, M. L., and Karakousis, P. C. (2017). Metformin adjunctive therapy does not improve the sterilizing activity of the first-line antitubercular regimen in mice. Antimicrob. Agents Chemother. 61:e00652-17.
Foretz, M., Guigas, B., Bertrand, L., Pollak, M., and Viollet, B. (2014). Metformin: from mechanisms of action to therapies. Cell Metab. 20, 953–966. doi: 10.1016/j.cmet.2014.09.018
Grady, S. L., Hwang, J., Vastag, L., Rabinowitz, J. D., and Shenk, T. (2012). Herpes simplex virus 1 infection activates poly(ADP-ribose) polymerase and triggers the degradation of poly(ADP-ribose) glycohydrolase. J. Virol. 86, 8259–8268. doi: 10.1128/jvi.00495-12
Gygli, S. M., Borrell, S., Trauner, A., and Gagneux, S. (2017). Antimicrobial resistance in Mycobacterium tuberculosis: mechanistic and evolutionary perspectives. FEMS Microbiol. Rev. 41, 354–373. doi: 10.1093/femsre/fux011
Hawn, T. R., Shah, J. A., and Kalman, D. (2015). New tricks for old dogs: countering antibiotic resistance in tuberculosis with host-directed therapeutics. Immunol. Rev. 264, 344–362. doi: 10.1111/imr.12255
Imai, S. I. (2016). The NAD World 2.0: the importance of the inter-tissue communication mediated by NAMPT/NAD(+)/SIRT1 in mammalian aging and longevity control. NPJ Syst. Biol. Appl. 2:16018.
Jiang, X., Zhang, W., Gao, F., Huang, Y., Lv, C., and Wang, H. (2006). Comparison of the proteome of isoniazid-resistant and -susceptible strains of Mycobacterium tuberculosis. Microb. Drug Resist. 12, 231–238. doi: 10.1089/mdr.2006.12.231
Kumar, B., Sharma, D., Sharma, P., Katoch, V. M., Venkatesan, K., and Bisht, D. (2013). Proteomic analysis of Mycobacterium tuberculosis isolates resistant to kanamycin and amikacin. J. Proteomics 94, 68–77. doi: 10.1016/j.jprot.2013.08.025
Lachmandas, E., Eckold, C., Böhme, J., Koeken, V. A. C. M., Marzuki, M. B., Blok, B., et al. (2019). Metformin alters human host responses to Mycobacterium tuberculosis in healthy subjects. J. Infect. Dis. 220, 139–150. doi: 10.1093/infdis/jiz064
Lee, Y. J., Han, S. K., Park, J. H., Lee, J. K., Kim, D. K., Chung, H. S., et al. (2018). The effect of metformin on culture conversion in tuberculosis patients with diabetes mellitus. Korean J. Intern. Med. 33, 933–940. doi: 10.3904/kjim.2017.249
Levac, D., Colquhoun, H., and O’Brien, K. K. (2010). Scoping studies: advancing the methodology. Implement. Sci. 5:69.
Lewis, K. (2007). Persister cells, dormancy and infectious disease. Nat. Rev. Microbiol. 5:48. doi: 10.1038/nrmicro1557
Matalonga, J., Glaria, E., Bresque, M., Escande, C., Carbo, J. M., Kiefer, K., et al. (2017). The nuclear receptor LXR limits bacterial infection of host macrophages through a mechanism that impacts cellular NAD metabolism. Cell Rep. 18, 1241–1255. doi: 10.1016/j.celrep.2017.01.007
Mesquita, I., Varela, P., Belinha, A., Gaifem, J., Laforge, M., Vergnes, B., et al. (2016). Exploring NAD+ metabolism in host-pathogen interactions. Cell. Mol. Life Sci. 73, 1225–1236. doi: 10.1007/s00018-015-2119-4
Millington, K. A. (2018). Ending Tuberculosis, K4D Emerging Issues Report. Brighton: Institute of Development Studies, 1–43.
Novita, B. D., Ali, M., Pranoto, A., Soediono, E. I., and Mertaniasih, N. M. (2019). Metformin induced autophagy in diabetes mellitus – tuberculosis co-infection patients: a case study. Indian J. Tuberc. 66, 64–69. doi: 10.1016/j.ijtb.2018.04.003
Novita, B. D., Pranoto, A., Wuryani, E., Soediono, I., and Mertaniasih, N. M. (2018). A case risk study of lactic acidosis risk by metformin use in type 2 diabetes mellitus tuberculosis coinfection patients. Indian J. Tuberc. 65, 252–256. doi: 10.1016/j.ijtb.2017.05.008
Rayasam, G. V., and Balganesh, T. S. (2015). Exploring the potential of adjunct therapy in tuberculosis. Trends Pharm. Sci. 36, 506–513. doi: 10.1016/j.tips.2015.05.005
Sharma, D., and Bisht, D. (2017). M. tuberculosis hypothetical proteins and proteins of unknown function: hope for exploring novel resistance mechanisms as well as future target of drug resistance. Front. Microbiol. 8:465. doi: 10.3389/fmicb.2017.00465
Sharma, D., Dhuriya, Y. K., Deo, N., and Bisht, D. (2017). Repurposing and revival of the drugs: a new approach to combat the drug resistant tuberculosis. Front. Microbiol. 8:2452. doi: 10.3389/fmicb.2017.02452
Sharma, D., Kumar, B., Lata, M., Joshi, B., Venkatesan, K., Shukla, S., et al. (2015). Comparative proteomic analysis of aminoglycosides resistant and susceptible Mycobacterium tuberculosis clinical isolates for exploring potential drug targets. PLoS One 10:e0139414. doi: 10.1371/journal.pone.0139414
Sharma, D., Lata, M., Singh, R., Deo, N., Venkatesan, K., and Bisht, D. (2016). Cytosolic proteome profiling of aminoglycosides resistant Mycobacterium tuberculosis clinical isolates using MALDI-TOF/MS. Front. Microbiol. 7:1816. doi: 10.3389/fmicb.2016.01816
Sharma, P., Kumar, B., Gupta, Y., Singhal, N., Katoch, V. M., Venkatesan, K., et al. (2010). Proteomic analysis of streptomycin resistant and sensitive clinical isolates of Mycobacterium tuberculosis. Proteome Sci. 8:59. doi: 10.1186/1477-5956-8-59
Shenoi, S., Heysell, S., Moll, A., and Friedland, G. (2009). Multidrug-resistant and extensively drug-resistant tuberculosis: consequences for the global HIV community. Curr. Opin. Infect. Dis. 22, 11–17. doi: 10.1097/qco.0b013e3283210020
Singhal, A., and De Libero, G. (2017). Methods to Inhibit Intracellular Growth of Bacteria and to Treat Bacteria-mediated Diseases, Google Patents. U.S. Patent No 9539222. Fusionopolis: Agency for Science, Technology and Research. doi: 10.1097/qco.0b013e3283210020
Singhal, A., Jie, L., Kumar, P., Hong, G. S., Leow, M. K., Paleja, B., et al. (2014). Metformin as adjunct antituberculosis therapy. Sci. Transl. Med. 6:263ra159. doi: 10.1126/scitranslmed.3009885
Srujitha, M., Padmanav, S., Swathi, P., Sonal Sekhar, M., Unnikrishnan, M. K., and Manu Mohan, K. (2017). Protective effect of metformin against tuberculosis infections in diabetic patients: an observational study of south Indian tertiary healthcare facility. Braz. J. Infect. Dis. 21, 312–316. doi: 10.1016/j.bjid.2017.01.001
Tan, H.-Y., Wang, N., Li, S., Hong, M., Wang, X., and Feng, Y. (2016). The reactive oxygen species in macrophage polarization: reflecting its dual role in progression and treatment of human diseases. Oxidat. Med. Cell. Longev. 2016:16.
Uplekar, M., and Raviglione, M. (2015). WHO’s end TB strategy: from stopping to ending the global TB epidemic. Indian J. Tuberc. 196–199. doi: 10.1016/j.ijtb.2015.11.001
Uplekar, M., Weil, D., Lonnroth, K., Jaramillo, E., Lienhardt, C., Dias, H. M., et al. (2015). WHO’s new End TB Strategy. Lancet 385, 1799–1801.
Vashisht, R., Bhat, A. G., Kushwaha, S., Bhardwaj, A., Consortium, O., and Brahmachari, S. K. (2014). Systems level mapping of metabolic complexity in Mycobacterium tuberculosis to identify high-value drug targets. J. Transl. Med. 12:263.
Vashisht, R., and Brahmachari, S. K. (2015). Metformin as a potential combination therapy with existing front-line antibiotics for Tuberculosis. J. Transl. Med. 13:83. doi: 10.1186/s12967-015-0443-y
Wakamoto, Y., Dhar, N., Chait, R., Schneider, K., Signorino-Gelo, F., Leibler, S., et al. (2013). Dynamic persistence of antibiotic-stressed mycobacteria. Science 339, 91–95. doi: 10.1126/science.1229858
World Health Organization (2013). WHO Model List of Essential Medicines. Geneva: World Health Organization.
World Health Organization (2016a). Global Tuberculosis Report. Geneva: World Health Organization, 1–214.
World Health Organization (2018). Global Tuberculosis Report. Geneva: World Health Organization, 1–277.
Yagi, T., Yano, T., Bernardo, S., and Matsuno-Yagi, A. (1998). Procaryotic complex I (NDH-1), an overview. Biochim. Biophys. Acta Bioenergetics 1364, 125–133. doi: 10.1016/s0005-2728(98)00023-1
Yarosz, E. L., and Chang, C. H. (2018). The role of reactive oxygen species in regulating T cell-mediated immunity and disease. Immune Netw. 18:e14.
Keywords: tuberculosis, metformin, host-directed therapy, Mycobacterium tuberculosis, adjuvant
Citation: Naicker N, Sigal A and Naidoo K (2020) Metformin as Host-Directed Therapy for TB Treatment: Scoping Review. Front. Microbiol. 11:435. doi: 10.3389/fmicb.2020.00435
Received: 03 December 2019; Accepted: 02 March 2020;
Published: 29 April 2020.
Edited by:
Pere-Joan Cardona, Institute for Health Science Research Germans Trias i Pujol (IGTP), SpainReviewed by:
Delia Goletti, Istituto Nazionale per le Malattie Infettive Lazzaro Spallanzani (IRCCS), ItalyCopyright © 2020 Naicker, Sigal and Naidoo. This is an open-access article distributed under the terms of the Creative Commons Attribution License (CC BY). The use, distribution or reproduction in other forums is permitted, provided the original author(s) and the copyright owner(s) are credited and that the original publication in this journal is cited, in accordance with accepted academic practice. No use, distribution or reproduction is permitted which does not comply with these terms.
*Correspondence: Nikita Naicker, bmlraXRhLm5haWNrZXJAY2FwcmlzYS5vcmc=
Disclaimer: All claims expressed in this article are solely those of the authors and do not necessarily represent those of their affiliated organizations, or those of the publisher, the editors and the reviewers. Any product that may be evaluated in this article or claim that may be made by its manufacturer is not guaranteed or endorsed by the publisher.
Research integrity at Frontiers
Learn more about the work of our research integrity team to safeguard the quality of each article we publish.