- 1Nanoscience Institute, CNR and NEST, Scuola Normale Superiore, Pisa, Italy
- 2The BioRobotics Institute, Polo Sant’Anna Valdera, Scuola Superiore Sant’Anna, Pontedera, Italy
The rise of antibiotic resistance is the main cause for the failure of conventional antibiotic therapy of Helicobacter pylori infection, which is often associated with severe gastric diseases, including gastric cancer. In the last years, alternative non-pharmacological approaches have been considered in the treatment of H. pylori infection. Among these, antimicrobial PhotoDynamic Therapy (aPDT), a light-based treatment able to photoinactivate a wide range of bacteria, viruses, fungal and protozoan parasites, could represent a promising therapeutic strategy. In the case of H. pylori, aPDT can exploit photoactive endogenous porphyrins, such as protoporphyrin IX and coproporphyrin I and III, to induce photokilling, without any other exogenous photosensitizers. With the aim of developing an ingestible LED-based robotic pill for minimally invasive intragastric treatment of H. pylori infection, it is crucial to determine the best illumination parameters to activate the endogenous photosensitizers. In this study the photokilling effect on H. pylori has been evaluated by using a novel LED-based device, designed for testing the appropriate LEDs for the pill and suitable to perform in vitro irradiation experiments. Exposure to visible light induced bacterial photokilling most effectively at 405 nm and 460 nm. Sub-lethal light dose at 405 nm caused morphological changes on bacterial surface indicating the cell wall as one of the main targets of photodamage. For the first time endogenous photosensitizing molecules other than porphyrins, such as flavins, have been suggested to be involved in the 460 nm H. pylori photoinactivation.
Introduction
Helicobacter pylori is the major causative agent of gastric chronic infection leading to severe gastric diseases such as atrophic gastritis, peptic ulcer, MALT (Mucosa-Associated Lymphoid Tissue) lymphoma, and significantly increasing the risk of developing gastric adenocarcinoma. H. pylori infection represents a risk factor in almost 90% of cases of gastric cancer, which is the third leading cause of cancer-related deaths worldwide according to World Health Organization (Wroblewski et al., 2010; Moss, 2016; Burkitt et al., 2017). In fact, the International Agency for Research on Cancer has classified H. pylori as a group I carcinogen (World Health Organization [WHO] and International Agency for Research on Cancer [IARC], 2019). The clinical treatment involves the use of several antimicrobials such as clarithromycin, amoxicillin and levofloxacin or metronidazole with a combined intake of a proton pump inhibitor. However, the alarming antibiotic resistance in H. pylori is the main reason for the failure of this conventional therapy. As a consequence, many research efforts have been devoted to find alternative non-antibiotic therapies (Bush et al., 2011).
PhotoDynamic Therapy (PDT), largely employed as a clinical treatment for several malignant and premalignant pathologies, has gained importance also as a promising antimicrobial approach (Dougherty, 2002; Kharkwal et al., 2011; Van Straten et al., 2017; Cieplik et al., 2018; Ferreira Dos Santos et al., 2019; Quilbe et al., 2020). Antimicrobial PhotoDynamic Therapy (aPDT) relies on the application of a photosensitizer able to absorb appropriate wavelengths in the visible light range and to react with oxygen molecules inside and around cells, resulting in the production of singlet oxygen or other cytotoxic reactive oxygen species (ROS), which lead to cell death, after inducing photodamage. aPDT can efficiently kill a wide range of bacteria (both antibiotic-susceptible and multi-resistant strains), viruses, fungal, and protozoan parasites (Smijs and Pavel, 2011; Thomas et al., 2015; Wang et al., 2017; Alves et al., 2018; Andrade et al., 2018; Namvar et al., 2019) without causing development of resistance (Al-Mutairi et al., 2018; Ma et al., 2018). This approach is particularly advantageous against bacteria naturally producing and accumulating endogenous photosensitizers such as porphyrins and flavins (Plavskii et al., 2018), physiologically involved in several essential biological functions (e.g., respiration, biological oxidation, photosynthesis, sulfate reduction, metabolism of fats, carbohydrates, and proteins) (Shu et al., 2013; García-Angulo, 2017; Sepúlveda Cisternas et al., 2018). Several studies determined the presence of endogenous photosensitizing porphyrins and/or flavins in Pseudomonas aeruginosa (Dai et al., 2013; Wang et al., 2016), Acinetobacter baumannii (Zhang et al., 2014; Wang et al., 2016), Candida albicans (Zhang et al., 2016), Aggregatibacter actinomycetemcomitans (Cieplik et al., 2014; Fyrestam et al., 2015), methicillin-resistant Staphylococcus aureus (Biener et al., 2017), Porphyromonas gingivalis (Fyrestam et al., 2015; Yoshida et al., 2017), Saccharomyces cerevisiae (Fyrestam et al., 2015; Hoenes et al., 2018), Legionella rubrilucens (Schmid et al., 2019), and Neisseria gonorrhoeae (Wang et al., 2019). The susceptibility to PDT has been demonstrated in Propionibacterium acnes, the etiological agent of acne, which produces porphyrins exploitable as photosensitizers (Romiti et al., 2000; Ashkenazi et al., 2003).
It has long been known that H. pylori also spontaneously produces porphyrins, making it a suitable target for PDT (Hamblin et al., 2005). In previous studies, we have analyzed the composition of H. pylori endogenous porphyrins and their photophysical characteristics in bacterial extracts as well as within planktonic and biofilm growing cells. H. pylori accumulates a porphyrin mixture mainly composed by protoporphyrin IX (PPIX) and coproporphyrin I and III (CPI and CPIII). The absorption spectrum of the mixture shows a main peak at 405 nm with the characteristic porphyrin absorption bands at longer wavelengths (Battisti et al., 2017a, b). Preliminary clinical studies have been conducted on H. pylori infected patients by administering a porphyrin precursor and blue light through laser endoscopy, highlighting a significant reduction of H. pylori in biopsies after the treatment (Wilder-Smith et al., 2002). Later, other authors have shown remarkable reduction of antrum bacterial load after endoscopic treatment with a violet light source only (Ganz et al., 2005; Lembo et al., 2009). The possible collateral effects of aPDT on healthy gastric mucosa have also been investigated: the phototreatments did not cause side effects, even when the release of porphyrins from bacteria in the surrounding tissues was simulated (Faraoni et al., 2018). Nevertheless, the endoscopic treatment is invasive and associated to a poor patient compliance. Thus, alternative devices have been taken into account (Li et al., 2016; Romano et al., 2016). In the CapsuLight project, a swallowable pill containing LED sources has been proposed to perform intragastric H. pylori phototherapy in a minimally invasive way (Tortora et al., 2016). For this purpose it is crucial to determine the best illumination parameters able to activate the H. pylori photosensitizers, and to evaluate the LED emission module before proceeding to the integration in the final ingestible device, in order to maximize the bacteria photokilling during aPDT while taking care of battery power needs. Hence, the present study is aimed at evaluating the bactericidal effect of aPDT on H. pylori strains by using a novel LED-based device, developed to perform in vitro irradiation tests on bacterial cultures, as a first necessary step for further in vivo studies.
Materials and Methods
Bacterial Strains and Cultivation Conditions
Two bacterial strains, purchased from LGC Standards S.r.l. (Milan, Italy), were used in this study: a laboratory-adapted strain, ATCC 43504, and a virulent strain (cagA+ and vacA+), ATCC 700824 (J99). Both strains were stored at −80°C in Brucella Broth (BB, Thermo Fisher Scientific Remel Products, Lenexa, KS, United States) supplemented with 10% (v/v) heat-inactivated fetal bovine serum (FBS, Gibco, Life Technologies, Carlsbad, CA, United States) and 20% (v/v) glycerol. From frozen stocks, bacteria were cultured overnight in BB supplemented with 10% (v/v) FBS at 37°C in a microaerophilic atmosphere (CampyGen Compact, Oxoid, Hampshire, United Kingdom) with shaking at 170 rpm in the dark.
After incubation, H. pylori cells were harvested in mid-log growth phase by centrifugation at 4000 × g for 10 min, washed to remove trace amounts of culture medium, and to avoid the influence of medium pigments. Bacterial suspensions at a concentration of 2 × 106 CFU/ml in Phosphate-Buffered Saline (PBS; pH 7.4) were prepared for photoinactivation assays.
LED-Based Device and Lighting Conditions for the Photoinactivation Assays
Bacterial suspensions were irradiated by means of a customized LED-based illuminating prototype. Selected LEDs (Nichia Corporation Ltd., Tokushima, Japan) were mounted on a board coupled with a programmable microcontroller (LPC81xM, NXP Semiconductors); two lithium batteries (CR2, 3 V) provide suitable power according to experimental needs. The assembled electronic elements are located inside a light-absorbent case (4 cm × 4 cm; height: 2.5 cm), topped with a ring-shaped holder where the sample dish is placed at a distance of 1 cm from the LED board and irradiated from below (Figure 1). Before the experiment the device was programmed (via a dedicated software) to set the desired control current, the number and position of the active LEDs and the irradiation time. Four different boards were used, each one mounting identical LEDs, whose number and position were designed to obtain comparable light intensities and a homogeneous irradiation field on the sample. The selected LEDs were: NVSU233A-U405 (Violet, 405 nm, FWHM ≈ 12 nm, 8 LEDs per board), NSSC146AT (Blue, 460 nm, FWHM ≈ 18 nm, 40 LEDs per board), NCSE119BT-V1 (Bluish-Green, 500 nm, FWHM ≈ 30 nm, 8 LEDs per board), NCSR219BT-V1-E (Red, 630 nm, FWHM ≈ 16 nm, 8 LEDs per board). These LEDs were selected to match the Soret band (405 nm), the shortest (500 nm), and the longest wavelength (630 nm) Q bands of porphyrin absorption spectrum and a spectral range (460 nm) where the porphyrin absorption spectrum has a minimum.
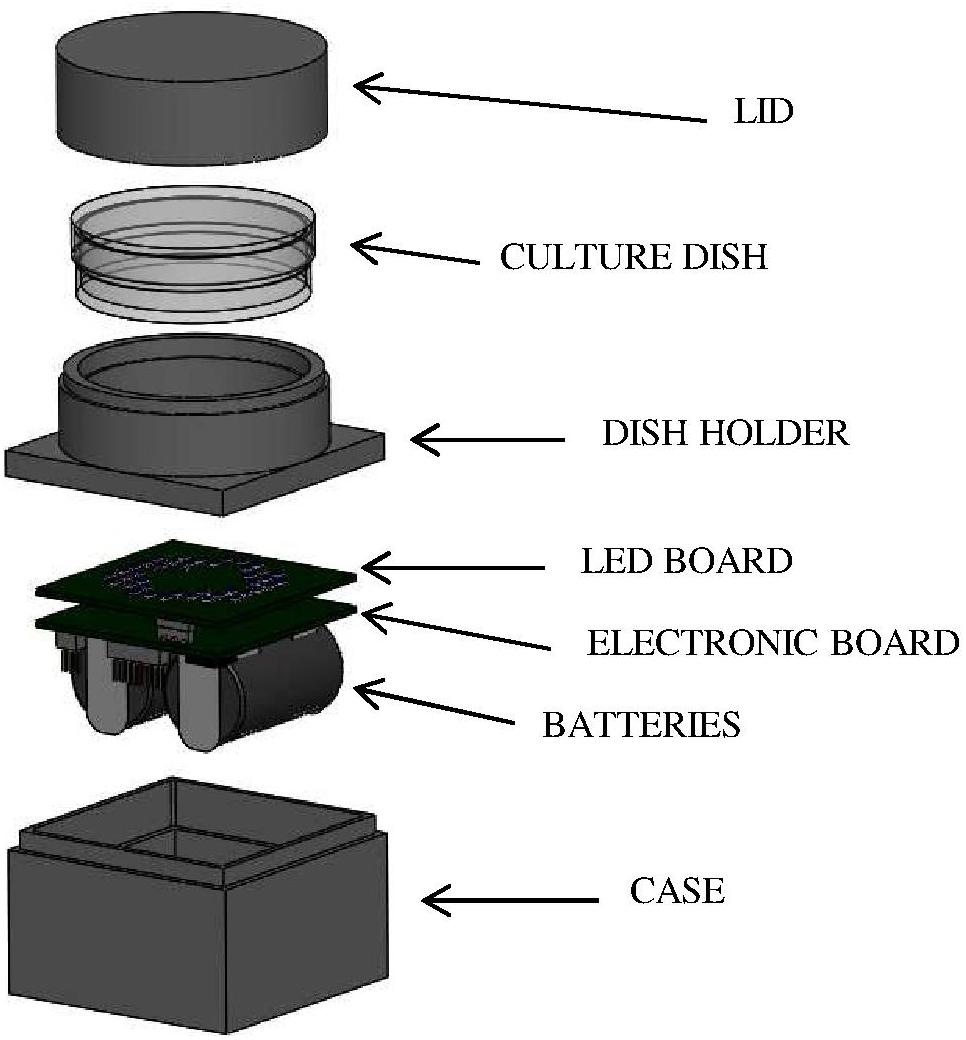
Figure 1. LED-based device for in vitro irradiation tests. LEDs were mounted on a board (LED BOARD) coupled with a programmable microcontroller (ELECTRONIC BOARD); two lithium BATTERIES provide suitable power according to experimental needs. The assembled electronic elements are located inside a light-absorbent CASE topped with a ring-shaped holder (DISH HOLDER) where the sample dish is placed at a distance of 1 cm from the LED board and irradiated from below. During irradiation experiments, the culture dish was covered with a LID to avoid light scattering.
Control measurements were performed to assure the stability of the control current, and consequently of the irradiance, in the time range of the designed experiments. Furthermore, preliminary tests allowed the determination of the maximum control current and the appropriate time extent of the irradiation in order to keep the sample temperature in the physiological range. Control current values were tested in steps of 25 mA up to 100 mA and the corresponding irradiance values, used throughout the experiments, were measured using a light spectroradiometer (SAMA Tools by S.A.M.A Italia Srl, Lucca, Italy) (Battisti et al., 2018).
Photoinactivation Assays
For the photoinactivation assays, an aliquot (2 ml) of a bacterial suspension (2 × 106 CFU/ml) was transferred to a Petri dish (35 mm) and irradiated in aerobic atmosphere at room temperature, according to the absorption spectrum of H. pylori endogenous porphyrins. At least three independent replicates were performed for each experiment. Following light irradiation assays, the illumination efficacy was assessed in comparison with the dark control by plating serial dilutions of each sample on Brucella agar plates with 10% FBS as described previously (Jett et al., 1997). Plates were incubated at 37°C in microaerophilic atmosphere for 4 days. After incubation, surviving bacterial cells (CFU/ml) were counted and, when indicated, survival fractions were determined relatively to untreated bacterial suspensions and expressed as Log CFU/ml.
Scanning Electron Microscopy
In order to examine the effects of irradiation at 405 nm on bacterial surface morphology, non-irradiated and irradiated samples were observed by scanning electron microscopy. A bacterial suspension of H. pylori ATCC 43504 (1 × 107 cells/ml) was exposed to sublethal light dose at 405 nm (9.3 J/cm2), as described above. Then, non-irradiated and irradiated samples were centrifuged and the pellet was fixed with 2% glutaraldehyde and 1% paraformaldehyde in 0.1 M cacodylate buffer for 1 h at room temperature and then overnight at 4°C. Samples were washed three times for 5 min in 0.1 M cacodylate buffer and postfixed in 1% OsO4 and 1% K3Fe(CN)6. After washing with distilled water, samples were dehydrated through increasing ethanol concentrations (30%, 50%, 70%, 90%, and 100%) in sequential steps of 10 min incubation. Finally, an aliquot of each sample was transferred on a silicon wafer, dried at the critical point and sputter coated with gold (8 nm) before imaging.
Fluorescence Characterization of H. pylori Extracts
Bacterial extracts from H. pylori cultures (100 ml) were prepared as previously described (Battisti et al., 2017a, b). H. pylori cells were harvested by centrifugation (7000 × g at 4°C for 10 min), washed in 20 ml pre-chilled buffer (0.05 M Tris pH 8.2–2 mM EDTA) and suspended in 10 ml of the same buffer. An aliquot (1.5 ml) of a mixture of ethyl acetate and acetic acid (3:1, v/v) was added and bacterial cells were lysed by sonication in ice. Then, the organic phase was extracted with 100 μl of HCl 3 M. After vigorous vortexing, this solution was centrifuged (7000 × g for 5 min) and then the bottom layer was collected for spectroscopic analysis and diluted in HCl 3M/MeOH 1:3. Fluorescence measurements were carried out with a Cary Eclipse fluorometer (Varian, Palo Alto, CA, United States) using 5 nm excitation band-pass, 5 nm emission band-pass, 0.5 s integration time.
High Performance Liquid Chromatography and Mass Spectrometry
High Performance Liquid Chromatography-Mass Spectrometry analyses were performed on a Shimadzu Nexera UHPLC chromatograph interfaced with an Ab Sciex 3200 QTRAP mass spectrometer (AB SCIEX, Toronto, ON, Canada). HPLC analyses were performed using a Phenomenex Kinetex PFP column (3 × 150 mm) using water/formic acid 100/0.1 v/v (A) and acetonitrile/formic acid 100/0.1 v/v (B) as mobile phases at 0.8 ml/min flow. Runs were performed under the following conditions: 2 min at 25% B, then a linear gradient to 95% B in 26 min, followed by a 4-min purge step at 95% B and by a 8-min re-equilibration step to the starting conditions. MS analyses were performed under the following conditions: ion spray voltage: 5000 V, source temperature 350°C, declustering potential 50 V, ion source gas 20 l/min, curtain gas: 25 l/min. m/z ratios between 200 and 1300 were monitored.
Statistical Analysis
The results of photoinactivation were expressed as mean ± standard error of the means (SEM). Differences between illuminated and unilluminated samples were evaluated with one-way ANOVA test, followed by Tukey-Kramer post hoc test using GraphPad Instat software (version 6.05 for Windows, La Jolla, CA, United States). The level of significance was set at a P value of <0.05.
Results
H. pylori Photoinactivation at 405 nm
A first set of measurements was performed irradiating the two H. pylori strains (ATCC 43504 and the virulent ATCC 700824) at 405 nm, the most effective wavelength in bacterial photokilling (Hamblin et al., 2005). Samples were exposed to 8.64 mW/cm2 irradiance (corresponding to the control current value of 25 mA) for times ranging between 5 and 20 min thus administering the following light doses: 2.59, 5.18, 7.78, and 10.4 J/cm2.
As shown in Figure 2, the viability of both the tested H. pylori strains was similarly affected by the 405 nm irradiation. A significant photoinactivation effect was quickly achieved after 15 min illumination (7.78 J/cm2), resulting in a 2.3 Log reduction in cell viability of both H. pylori strains, as compared to the dark control. The photoinactivation effect further increased (P < 0.001) after 20 min of light exposure (10.4 J/cm2), causing about four Log reduction of bacterial count.
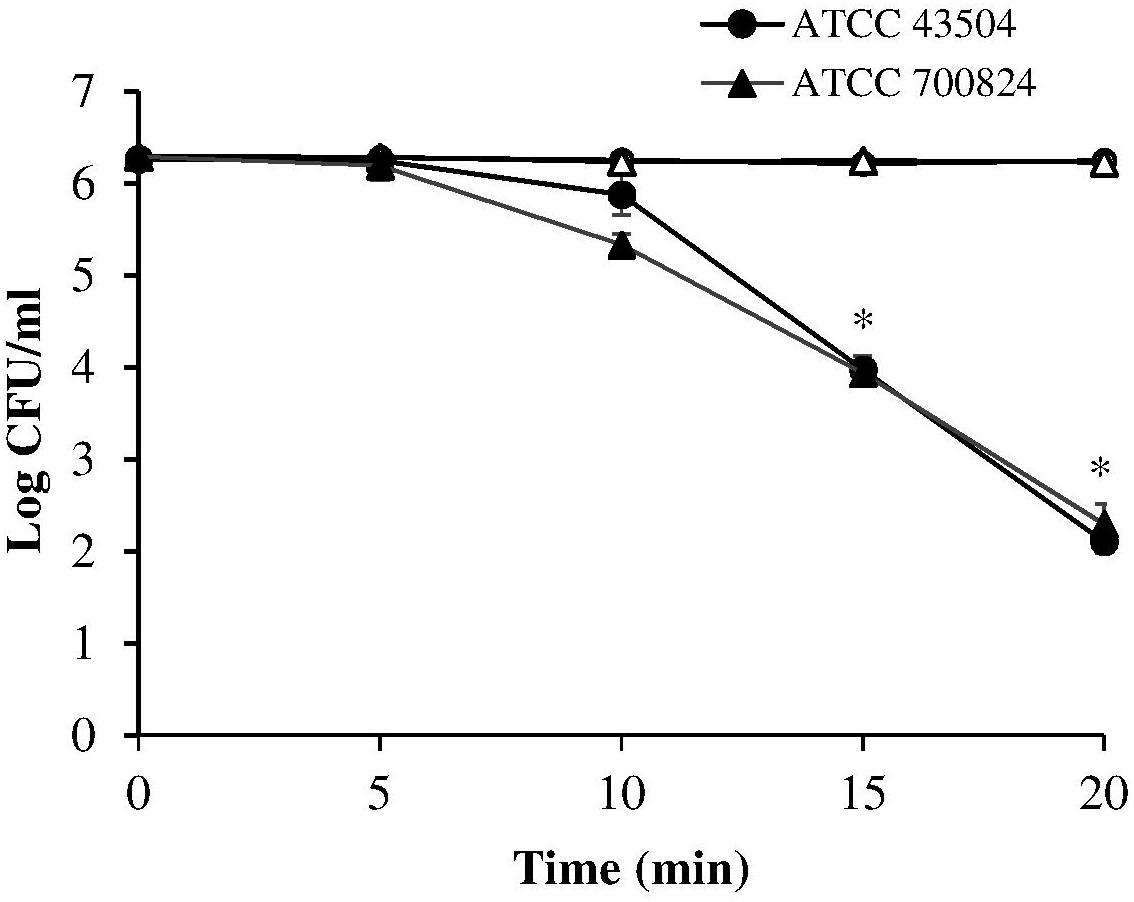
Figure 2. Photoinactivation at 405 nm. H. pylori ATCC 43504 and ATCC 700824 were illuminated at 405 nm (8.64 mW/cm2 irradiance) for different exposure time points in aerobic atmosphere at room temperature (closed circle and closed triangle for ATCC 43504 and ATCC 700824, respectively). Data are expressed as means of three independent experiments ±SEM. ∗ P < 0.001, as compared to the dark control (open symbols).
A reciprocity test of the photoinactivation effect was then performed on the ATCC 700824 strain administering various radiation doses at 405 nm, varying both irradiance and exposure time, as shown in Table 1.
The dose-response curves at the three irradiance values (reported in Figure 3, together with the results of the corresponding control measurements) show a very similar trend, indicating that in these experimental conditions the photoinactivation effect is dose-dependent regardless of the administration method (i.e., varying irradiance or irradiation time).
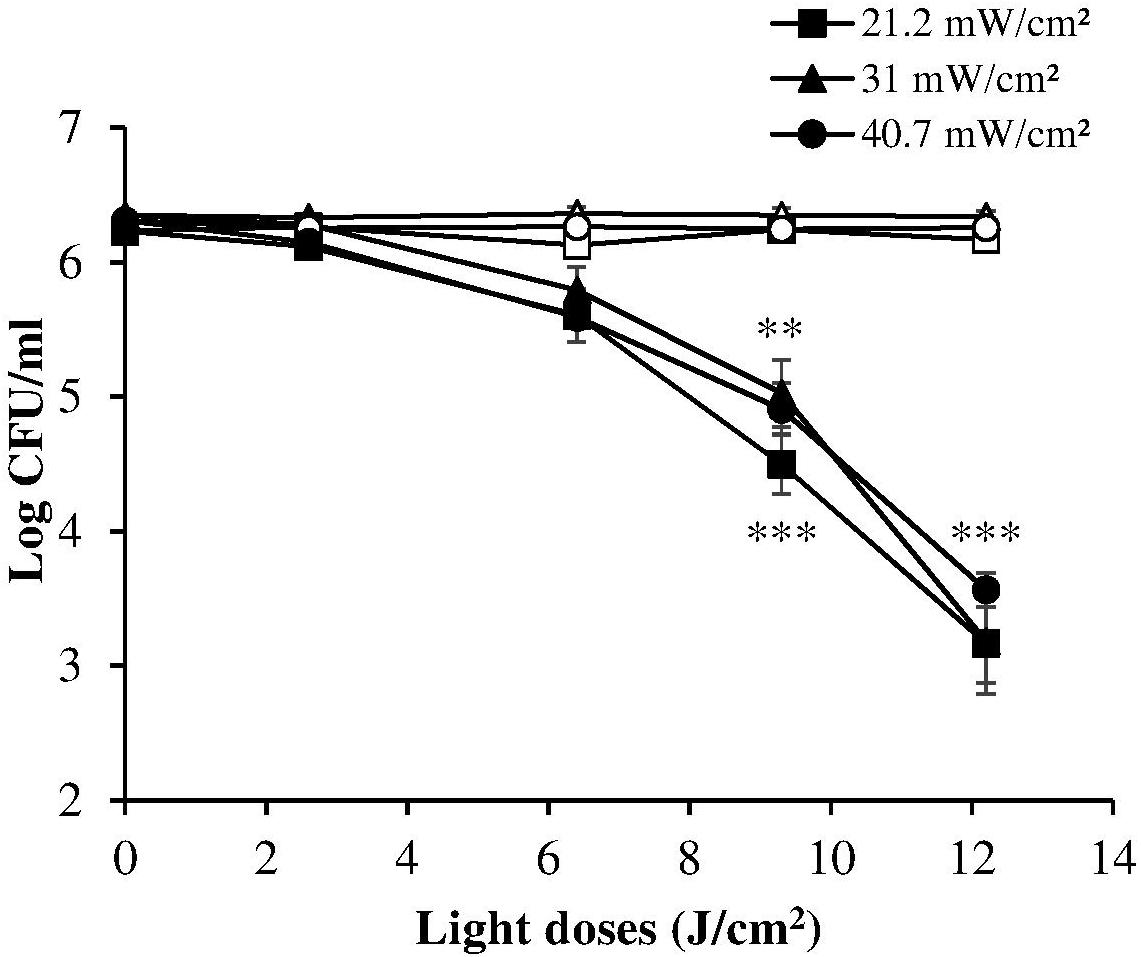
Figure 3. Comparison between dose-effect curves of H. pylori photoinactivation at 405 nm at three different irradiances. H. pylori ATCC 700824 (about 2 × 106 CFU/ml) was exposed to different light doses (405 nm) delivered at constant irradiances (21.2 mW/cm2, closed square; 31 mW/cm2, closed triangle; 40.7 W/cm2, closed circle), under aerobic atmosphere, changing the exposure time (see Table 1), thus providing the same light dose. Data are expressed as means of at least three independent experiments ±SEM. ∗∗ P < 0.01; ∗∗∗ P < 0.001, as compared to the dark control (open symbols).
Dose-Effect Curves at Different Wavelengths
Despite violet light may cause an efficient H. pylori photokilling in vitro, its effects may be limited to the most superficial layers of the gastric mucosa because of its low penetration through tissues, thus probably hampering the eradication of the infection in vivo. Porphyrins also have absorbance bands in the green and red spectral regions, and light of those wavelengths penetrates deeper into the layers of the gastric mucosa. Thus, in order to choose the most effective combination of wavelengths to implement inside the ingestible LED-equipped pill, dose-effect curves obtained by exposure of H. pylori at different wavelengths corresponding to the peaks of the Soret (405 nm) as well as Q (500 and 630 nm) porphyrin absorption bands were compared. As negative control, irradiation was also performed at 460 nm where porphyrin absorbance has a minimum (Figures 4A,B). Figure 5 shows for the two bacterial strains the relative photokilling efficiency (action spectrum) at 405 nm, 460 nm and 500 nm, plotted as the reciprocal of the light dose required to induce a 3 Log reduction in bacterial viability, which is conventionally regarded as bactericidal (Nakonieczna, 2017). These values were estimated by linear interpolation of the appropriate data points of the dose-effect curves. The action spectrum indicates that for the virulent strain ATCC 700824 the photoinactivation effect at 405 nm was about 3 and 10 times higher than the one observed at 460 nm and at 500 nm, respectively, whereas it is about 2 and 7 times higher for the laboratory-adapted strain ATCC 43504.
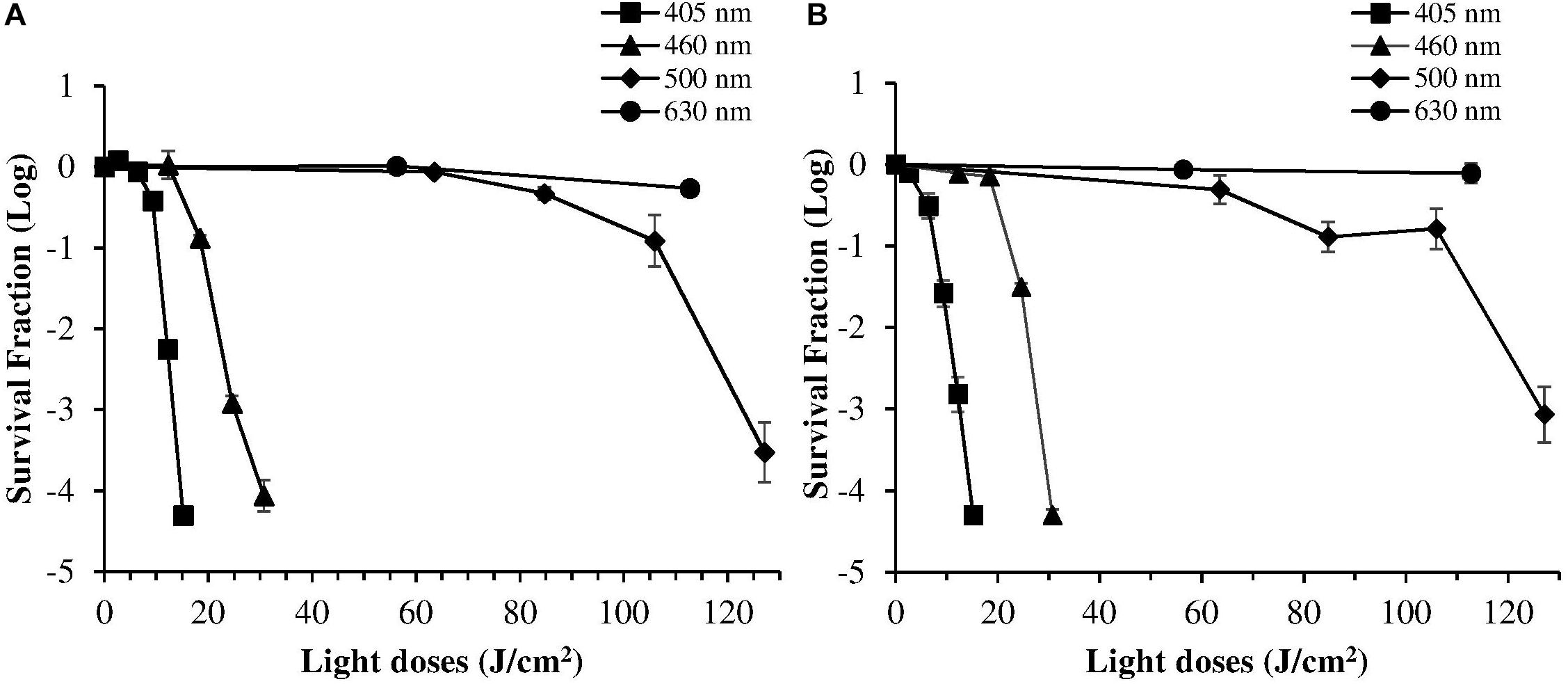
Figure 4. Comparison of photoinactivation curves of H. pylori ATCC 43504 (A) and ATCC 700824 (B) strains following exposure to light at different wavelengths: 405 nm (square), 460 nm (triangle), 500 nm (diamond), 630 nm (circle). H. pylori cells (about 2 × 106 CFU/ml) were illuminated at room temperature under aerobic atmosphere. Data are expressed as means of survival fraction of at least three independent experiments ±SEM.
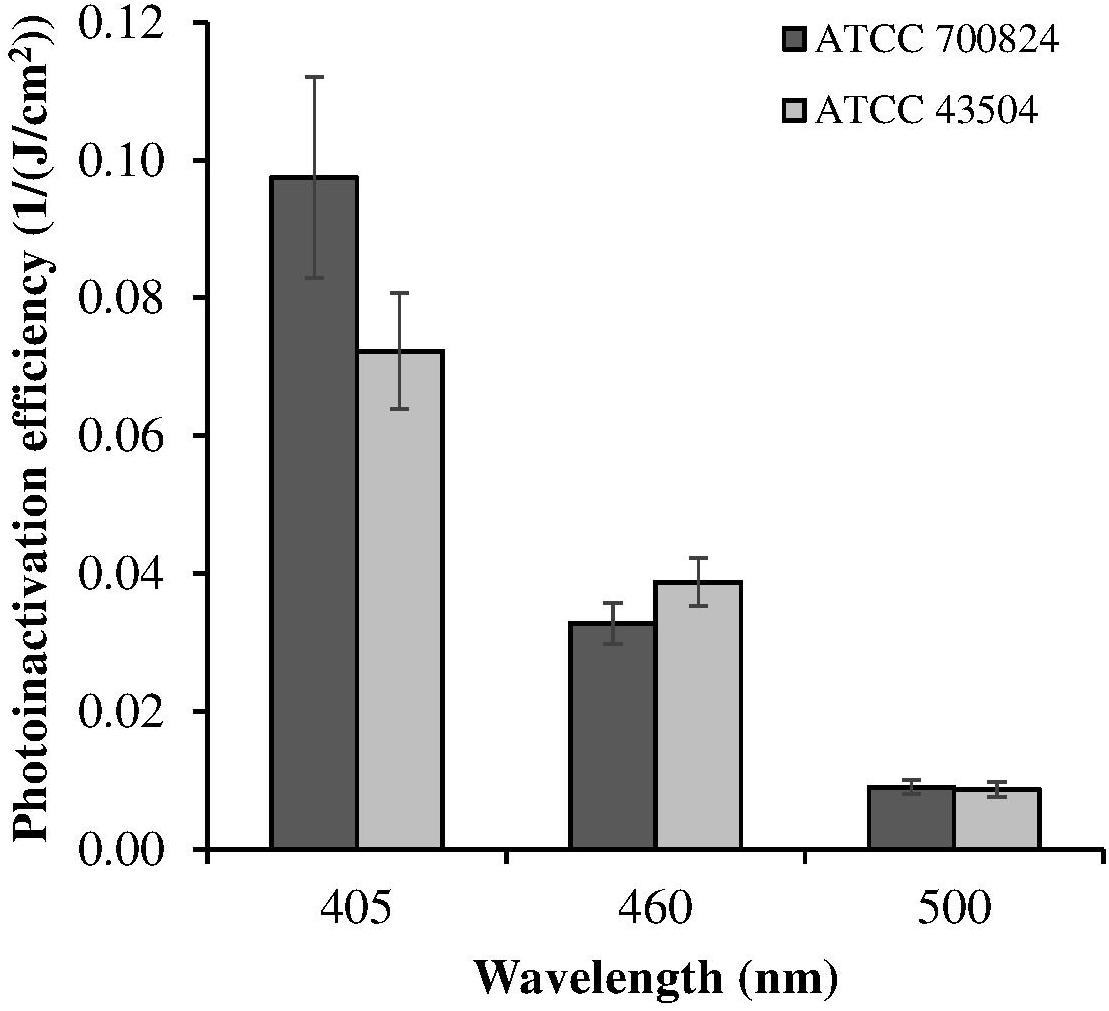
Figure 5. Relative photoinactivation efficiency at different wavelengths for H. pylori ATCC 43504 and ATCC 700824 strains. Photoinactivation efficiency is plotted as the reciprocal of the light dose required to induce a three Log reduction in bacterial viability.
The irradiation at 630 nm did not induce any photoinactivation effect, even using the maximum light dose (maximum exposure time of 1 h; LED at 630 nm; 31.3 mW/cm2 irradiance) allowed without reaching non-physiological temperatures.
Effect of Irradiation on H. pylori Cell Morphology
Scanning electron microscopy revealed morphological alterations on the bacterial surface of H. pylori cells, exposed to the sublethal light dose of 9.3 J/cm2 at 405 nm, affecting the cell viability without inducing a massive reduction of bacterial count. Non-irradiated bacteria, used as control, showed a curved or spiral shape and their bacterial surface appeared intact, with one or a few flagella at one end (Figure 6A). Conversely, irradiated H. pylori cells were damaged showing holes on the bacterial surface (Figure 6B), evidence of cellular suffering and prelude to bacterial death. No coccoid form was observed in both non-irradiated and irradiated cells.
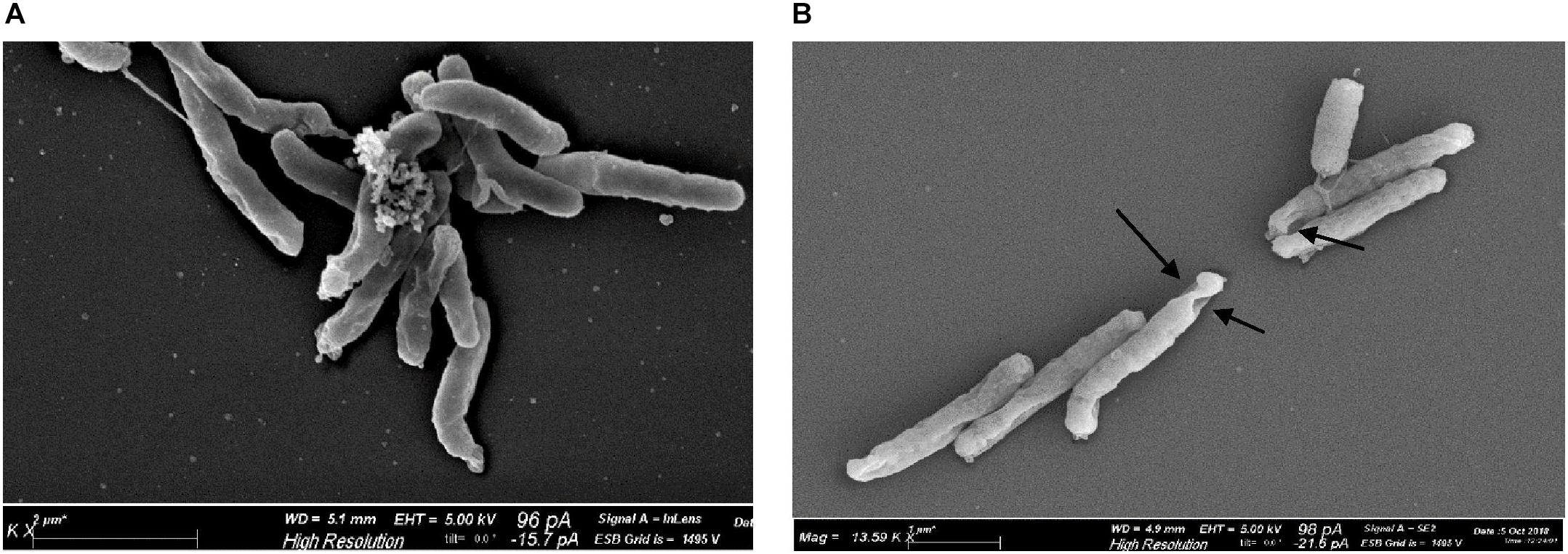
Figure 6. Effect of illumination at 405 nm on bacterial morphology. Representative scanning electron microscope images of H. pylori ATCC 43504 (5 × 107 cells/ml): (A) non-irradiated and (B) irradiated cells at 405 nm (9.3 J/cm2). About 100 cells/sample were analyzed. Arrows indicate the presence of holes on the surface of irradiated bacteria.
Spectroscopic Characterization of Endogenous Photosensitizers
The H. pylori photoinactivation observed at 460 nm (Figure 5) is too high to be exclusively attributed to the photosensitizing action of endogenous porphyrins. In fact, on the basis of porphyrin spectroscopic features, the difference in light absorption at 405 and 460 nm should correspond to a similar difference in bacterial photosensitivity: a tenfold reduction of the effect was expected, but only a two-threefold reduction was obtained by shifting the irradiation from 405 nm to 460 nm. In order to identify a second putative endogenous photosensitizer, fluorescence emission and excitation spectra were performed on bacteria extracts. In Figure 7A the fluorescence emission (lexc = 405 nm) and excitation (lem = 660 nm) spectra showed the typical spectroscopic features of porphyrins, as expected (Battisti et al., 2017a). Exciting H. pylori extracts at 460 nm, a broad fluorescence emission band peaked around 525 nm was observed while no porphyrin emission bands at 600 nm and 650 nm were detected (Figure 7B). As in the case of other photosensitive bacteria the broad fluorescence peak around 525 nm can be assigned to flavin compounds, namely, riboflavin, FAD, and FMN, which exhibit very similar absorption and emission characteristics (Plavskii et al., 2018). Along with the flavin-like fluorescence emission, an intense peak in the mass spectrum of the bacterial extracts, corresponding to an m/z ratio of 376, suggests the presence of riboflavin as a significant component of the mixture (Figure 8).
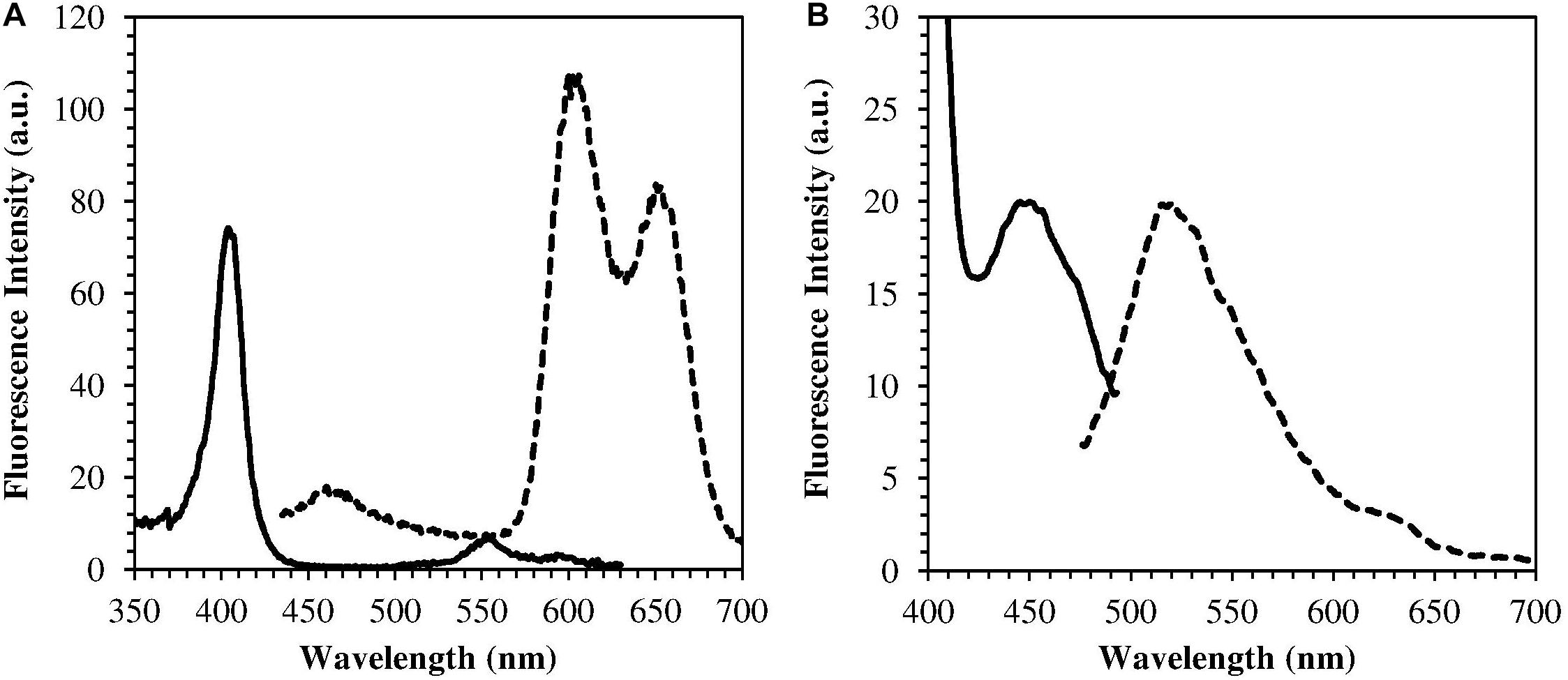
Figure 7. Emission (dotted curves) and excitation (solid curves) fluorescence spectra of H. pylori extracts. (A) lexc = 405 nm, lem = 660 nm (porphyrin fluorescence); (B) lexc = 460 nm, lem = 520 nm (flavin fluorescence).
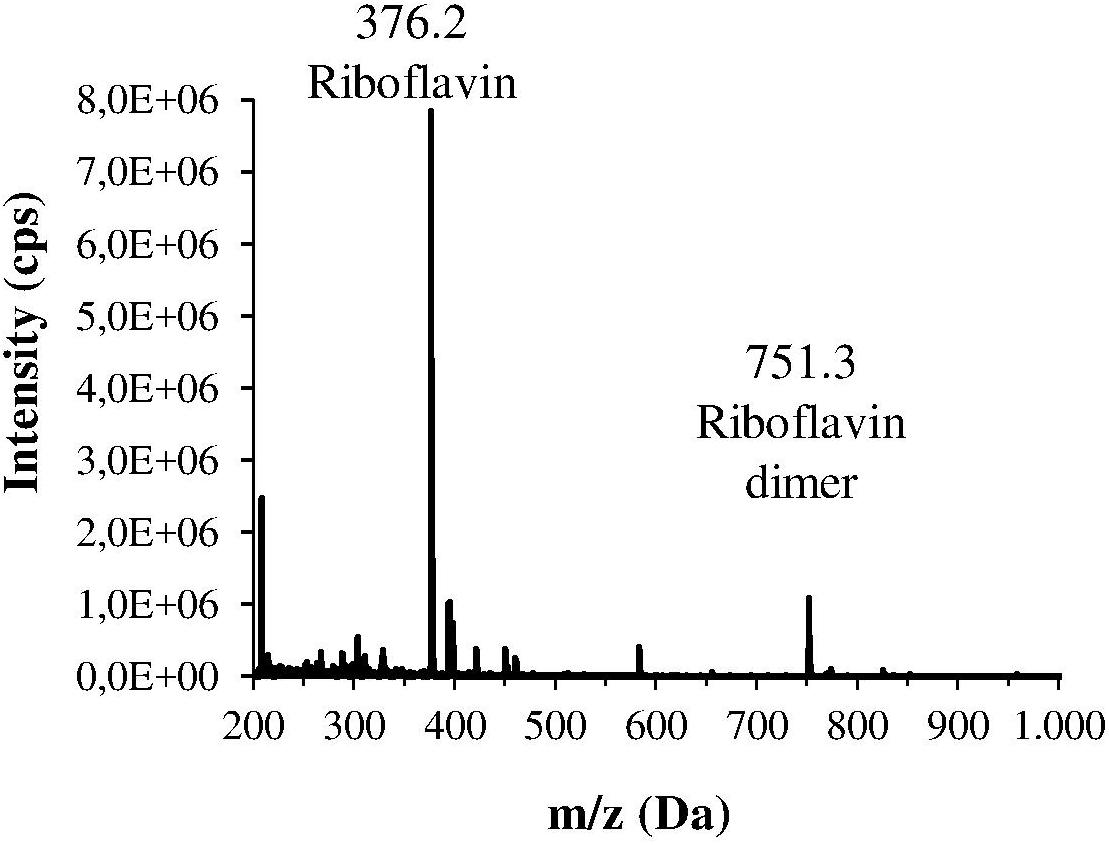
Figure 8. Mass spectrum of one the main fractions of the bacterial extract, isolated by HPLC. This spectrum corresponds to the fraction with the lowest retention time, and shows an intense m/z peak at 376.2 Da and a lower peak at 751.3 Da that could suggest the presence of riboflavin and its dimer.
Discussion
The present study shows that visible light irradiation by using an innovative LED-based device can efficiently induce in vitro photokilling in H. pylori without the administration of exogenous photosensitizers. In fact, a significant reduction of cell viability was observed after illumination with LEDs at 405 nm on both tested H. pylori strains, including the virulent one, ATCC 700824. In addition, the induced photokilling effect is mainly dependent on the delivered light dose, at least within the tested values range. Indeed, combinations of longer exposure times with lower irradiances, or shorter exposure times with higher irradiances, provided a similar photokilling effect. Thus, according to the reciprocity law of Bunsen-Roscoe, the observed photoinactivation is directly proportional to the total energy dose, regardless of the administering regime (Schindl et al., 2001). Although few data are currently available on this issue, reciprocity is often found to hold true in bacteria, unlike in eukaryotic organisms endowed with more complex mechanisms of oxidative damage repair (Farr and Kogoma, 1991; Schindl et al., 2001; Morita et al., 2010; Taraszkiewicz et al., 2015; Ogonowska et al., 2019). Considering the potential clinical application of aPDT, this aspect is crucial in defining the most effective protocol of irradiation, and thus it should be taken into account for in vitro aPDT studies.
As previously referred by Hamblin et al. (2005), also in our irradiation experiments 405 nm was the most effective wavelength in inducing photokilling in both H. pylori strains. Much higher doses of 460 nm and 500 nm wavelengths were necessary to induce a bactericidal effect, whereas the 630 nm irradiation was ineffective. Therefore, in accordance with other studies, the efficacy of bacterial photoinactivation at different wavelengths mainly correlates with the light absorption spectrum of endogenous porphyrins, decreasing with the increase in irradiation wavelength (Pummer et al., 2017). However, the observed photoinactivation of H. pylori by light of 450–470 nm is much higher than expected on the basis of porphyrin absorption characteristics, since they only exhibit a strong absorption in the spectral region of 380–420 nm and a weak absorption in the 500–650 nm range. Previous studies on other microorganisms demonstrated that significant bacterial photoinactivation around 460 nm is mediated by flavin-like molecules (Hessling et al., 2017; Hoenes et al., 2018; Tomb et al., 2018). Our spectroscopical and mass analysis studies of bacterial extracts indicate for the first time flavin compounds, along with porphyrins, as endogenous photosensitizers responsible for H. pylori photoinactivaction.
As far as the cellular targets are concerned, our scanning electron images show morphological alterations of the bacterial cell wall after administration of a sub-lethal light dose. This could be the main damage of the photodynamic action, leading to bacterial death through leakage of cellular contents or inactivation of membrane transport systems and enzymes (Caminos et al., 2008; Ma et al., 2018).
As reported by safety studies on the side effects of aPDT on host cells and tissue, our irradiation conditions are sufficient to inactivate H. pylori, but are ineffective to cause lethal oxidative bursts through excitation of endogenous pigments of mammalian cells (Wang et al., 2017).
In conclusion, the overall findings suggest that aPDT could be an alternative safe effective treatment, or an adjuvant therapy to conventional antibiotics for H. pylori eradication. Cost-effective and energy-efficient LEDs emitting in the violet (405 nm) and blue (460 nm) region could efficiently photoinactivate H. pylori and can be integrated in final ingestible pills used for gastric irradiation. The endogenous photosensitizers responsible for bacterial photokilling are not only porphyrins, PPIX and CPI and III, but also flavin-type molecules such as riboflavin.
Further studies will be aimed at evaluating the efficacy of irradiation on biofilms produced by clinical H. pylori strains since bacteria embedded therein may exhibit different characteristics as compared to their planktonic counterparts in terms of resistance toward antimicrobials (Costerton et al., 1995; Hathroubi et al., 2018).
Data Availability Statement
The raw data supporting the conclusion of this article will be made available by the authors, without undue reservation, to any qualified researcher.
Author Contributions
PM, FG, and AS conceived and designed the experiments. PM, AB, GT, and AS performed the experiments. PM, AB, FG, GC, and AS analyzed the data. PM drafted the manuscript, with the contribution of AB, GC, FG, and AS. All authors reviewed and revised the first and final drafts of this manuscript.
Conflict of Interest
The authors declare that the research was conducted in the absence of any commercial or financial relationships that could be construed as a potential conflict of interest.
Funding
Financial support was obtained from the Regione Toscana Bando FAS Salute 2014 (Italy) in the framework of the project “CapsuLight – Design of an ingestible robotic pill based on LED sources for the treatment of gastrointestinal disorders” (CUP B52I14005760002).
Acknowledgments
The authors thank Dr. Giorgio De Simoni (CNR-NANO, Pisa) for the valuable help in scanning electron microscopy imaging.
References
Al-Mutairi, R., Tovmasyan, A., Batinic-Haberle, I., and Benov, L. (2018). Sublethal Photodynamic Treatment Does Not Lead to Development of Resistance. Front. Microbiol. 9:1699. doi: 10.3389/fmicb.2018.01699
Alves, F., Alonso, G. C., Carmello, J. C., de Oliveira Mima, E. G., Bagnato, V. S., and Pavarina, A. C. (2018). Antimicrobial Photodynamic Therapy mediated by Photodithazine® in the treatment of denture stomatitis: A case report. Photodiagn. Photodyn. Ther. 21, 168–171. doi: 10.1016/j.pdpdt.2017.11.018
Andrade, C. G., Figueiredo, R. C. B. Q., Ribeiro, K. R. C., Souza, L. I. O., Sarmento-Neto, J. F., and Rebouças, J. S. (2018). Photodynamic effect of zinc porphyrin on the promastigote and amastigote forms of Leishmania braziliensis. Photochem. Photobiol. Sci. 17, 482–490. doi: 10.1039/c7pp00458c
Ashkenazi, H., Malik, Z., Harth, Y., and Nitzan, Y. (2003). Eradication of Propionibacterium acnes by its endogenic porphyrins after illumination with high intensity blue light. FEMS Immunol. Med. Microbiol. 35, 17–24. doi: 10.1111/j.1574-695X.2003.tb00644.x
Battisti, A., Morici, P., Ghetti, F., and Sgarbossa, A. (2017a). Spectroscopic characterization and fluorescence imaging of Helicobacter pylori endogenous porphyrins. Biophys Chem. 229, 19–24. doi: 10.1016/j.bpc.2017.05.010
Battisti, A., Morici, P., Signore, G., Ghetti, F., and Sgarbossa, A. (2017b). Compositional analysis of endogenous porphyrins from Helicobacter pylori. Biophys Chem. 229, 25–30. doi: 10.1016/j.bpc.2017.06.006
Battisti, A., Morici, P., Tortora, G., Menciassi, A., Checcucci, G., Ghetti, F., et al. (2018). Temperature increase inside LED-based illuminators for in vitro aPDT photodamage studies. Results Phys. 9, 680–681. doi: 10.1016/j.rinp.2018.03.032
Biener, G., Masson-Meyers, D. S., Bumah, V. V., Hussey, G., Stoneman, M. R., Enwemeka, C. S., et al. (2017). Blue/violet laser inactivates methicillin-resistant Staphylococcus aureus by altering its transmembrane potential. J. Photochem. Photobiol. B. 170, 118–124. doi: 10.1016/j.jphotobiol.2017.04.002
Burkitt, M. D., Duckworth, C. A., Williams, J. M., and Pritchard, D. M. (2017). Helicobacter pylori-induced gastric pathology: insights from in vivo and ex vivo models. Dis Model Mech. 10, 89–104. doi: 10.1242/dmm.027649
Bush, K., Courvalin, P., Dantas, G., Davies, J., Eisenstein, B., Huovinen, P., et al. (2011). Tackling antibiotic resistance. Nat. Rev. Microbiol. 9, 894–896. doi: 10.1038/nrmicro2693
Caminos, D. A., Spesia, M. B., Pons, P., and Durantin, E. N. (2008). Mechanisms of Escherichia coli photodynamic inactivation by an amphiphilic tricationic porphyrin and 5,10,15,20-tetra(4-N,N,N-trimethylammoniumphenyl) porphyrin. Photochem. Photobiol. Sci. 7, 1071–1078. doi: 10.1039/B804965C
Cieplik, F., Deng, D., Crielaard, W., Buchalla, W., Hellwig, E., Al-Ahmad, A., et al. (2018). Antimicrobial photodynamic therapy – what we know and what we don’ t. Crit. Rev. Microbiol. 44, 571–589. doi: 10.1080/1040841X.2018.1467876
Cieplik, F., Späth, A., Leibl, C., Gollmer, A., Regensburger, J., Tabenski, L., et al. (2014). Blue light kills Aggregatibacter actinomycetemcomitans due to its endogenous photosensitizers. Clin. Oral Investig. 18, 1763–1769. doi: 10.1007/s00784-013-1151-8
Costerton, J. W., Lewandowski, Z., Caldwell, D. E., Korber, D. R., and Lappin-Scott, H. M. (1995). Microbial biofilms. Annu. Rev. Microbiol. 49, 711–745. doi: 10.1146/annurev.mi.49.100195.003431
Dai, T., Gupta, A., Huang, Y. Y., Yin, R., Murray, C. K., Vrahas, M. S., et al. (2013). Blue light rescues mice from potentially fatal Pseudomonas aeruginosa burn infection: efficacy, safety, and mechanism of action. Antimicrob. Agents Chemother. 57, 1238–1245. doi: 10.1128/AAC.01652-12
Dougherty, T. J. (2002). An update on photodynamic therapy applications. J. Clin. Laser Med. Surg. 20, 3–7. doi: 10.1089/104454702753474931
Faraoni, P., Gnerucci, A., Ranaldi, F., Orsini, B., Romano, G., and Fusi, F. (2018). Side effects of intra-gastric photodynamic therapy: an in vitro study. Journal of Photochemistry and Photobiology B: Biology. 186, 107–115. doi: 10.1016/j.jphotobiol.2018.07.010
Farr, S. B., and Kogoma, T. (1991). Oxidative stress responses in Escherichia coli and Salmonella typhimurium. Microbiol. Mol. Biol. Rev. 55, 561–585. doi: 10.1128/mmbr.55.4.561-585.1991
Ferreira Dos Santos, A., Raquel Queiroz De Almeida, D., Ferreira Terra, L., Baptista, M., and Labriola, L. (2019). Photodynamic therapy in cancer treatment–an update review. J. Cancer Metast. Treat. 5, 25. doi: 10.20517/2394-4722.2018.83
Fyrestam, J., Bjurshammar, N., Paulsson, E., Johannsen, A., and Östman, C. (2015). Determination of porphyrins in oral bacteria by liquid chromatography electrospray ionization tandem mass spectrometry. Anal. Bioanal. Chem. 407, 7013. doi: 10.1007/s00216-015-8864-2
Ganz, R. A., Viveiros, J., Ahmad, A., Ahmadi, A., Khalil, A., Tolkoff, M. J., et al. (2005). Helicobacter pylori in patients can be killed by visible light. Lasers Surg. Med. 36, 260–265. doi: 10.1002/lsm.20161
García-Angulo, V. A. (2017). Overlapping riboflavin supply pathways in bacteria. Crit. Rev. Microbiol. 43, 196–209. doi: 10.1080/1040841X.2016.1192578
Hamblin, M. R., Viveiros, J., Yang, C., Ahmadi, A., Ganz, R. A., and Tolkoff, M. J. (2005). Helicobacter pylori accumulates photoactive porphyrins and is killed by visible light. Antimicrob. Agents Chemother. 49, 2822–2827. doi: 10.1128/AAC.49.7.2822-2827.2005
Hathroubi, S., Servetas, S. L., Windham, I., Merrell, D. S., and Ottemann, K. M. (2018). Helicobacter pylori Biofilm Formation and Its Potential Role in Pathogenesis. ıMicrobiol. Mol. Biol. Rev. 82, e00001–e18. doi: 10.1128/MMBR.00001-18
Hessling, M., Spellerberg, B., and Hoenes, K. (2017). Photoinactivation of bacteria by endogenous photosensitizesers and exposure to visible light of different wavelengths-a review on existing data. FEMS Microbiol. Lett. 364, 1–12. doi: 10.1093/femsle/fnw270
Hoenes, K., Hess, M., Vatter, P., Spellerberg, B., and Hessling, M. (2018). 405 nm and 450 nm photoinactivation of Saccharomyces cerevisiae. Eur. J. Microbiol. Imm. 8, 142–148. doi: 10.1556/1886.2018.00023
Jett, B. D., Hatter, K. L., Huycke, M. M., and Gilmore, M. S. (1997). Simplified agar plate method for quantifying viable bacteria. BioTechniques. 23, 648–650. doi: 10.2144/97234bm22
Kharkwal, G. B., Sharma, S. K., Huang, Y. Y., Dai, T., and Hamblin, M. R. (2011). Photodynamic therapy for infections: clinical applications. Lasers Surg. Med. 43, 755–767. doi: 10.1002/lsm.21080
Lembo, A. J., Ganz, R. A., Sheth, S., Cave, D., Kelly, C., Levin, P., et al. (2009). Treatment of Helicobacter pylori infection with intra-gastric violet light phototherapy: a pilot clinical trial. Lasers Surg. Med. 41, 337–344. doi: 10.1002/lsm.20770
Li, Z., Ren, B., Tan, H., Liu, S., Wang, W., Pang, Y. et al. (2016). Capsule design for blue light therapy agaist Helicobacter pylori. PLoS One. 11, 1–10. doi: 10.1371/journal.pone.0147531
Ma, J., Hiratsuka, T., Etoh, T., Akada, J., Fujishima, H., Shiraishi, N., et al. (2018). Anti-proliferation effect of blue light-emitting diodes against antibiotic-resistant Helicobacter pylori. J. Gastroenterol. Hepatol. 33, 1492–1499. doi: 10.1111/jgh.14066
Morita, R., Nakane, S., Shimada, A., Inoue, M., Iino, H., Wakamatsu, T., et al. (2010). Molecular Mechanisms of the Whole DNA Repair System: A Comparison of Bacterial and Eukaryotic Systems. J Nucleic Acids 2010, 179594. doi: 10.4061/2010/179594
Moss, S. F. (2016). The Clinical Evidence Linking Helicobacter pylori to Gastric Cancer. Cell. Mol. Gastroenterol. Hepatol. 3, 183–191. doi: 10.1016/j.jcmgh.2016.12.001
Nakonieczna, J. (2017). Comment on “Effectiveness of antimicrobial photodynamic therapy (AmPDT) on Staphylococcus aureus using phenothiazinecompound with red laser”. Lasers Med Sci. 32, 1667–1668. doi: 10.1007/s10103-016-2107-4
Namvar, M. N., Vahedi, M., Abdolsamadi, H., Mirzaei, A., Mohammadi, Y., and Azizi Jalilian, F. (2019). Effect of photodynamic therapy by 810 and 940 nm diode laser on Herpes Simplex Virus 1: An in vitro study. Photodiagn Photodyn. 25, 87–89. doi: 10.1016/j.pdpdt.2018.11.011
Ogonowska, P., Woźniak, A., Pierański, M., Wasylew, T., Kwiek, P., Brasel, M., et al. (2019). Application and characterization of light-emitting diodes for photodynamic inactivation of bacteria. Light Res. Technol. 51, 612–624. doi: 10.1177/1477153518781478
Plavskii, V. Y., Mikulich, A. V., Tretyakova, A. I., Leusenka, I. A., Plavskaya, L. G., Kazyuchits, O. A., et al. (2018). Porphyrins and flavins as endogenous acceptors of optical radiation of blue spectral region determining photoinactivation of microbial cells. J. Photochem. Photobiol. B. 183, 172–183. doi: 10.1016/j.jphotobiol.2018.04.021
Pummer, A., Knüttel, H., Hiller, K. A., Buchalla, W., Cieplik, F., and Maisch, T. (2017). Antimicrobial efficacy of irradiation with visible light on oral bacteria in vitro: a systematic review. Future Med. Chem. 9, 1557–1574. doi: 10.4155/fmc-2017-0051
Quilbe, A., Moralès, O., Baydoun, M., Kumar, A., Mustapha, R., Murakami, T., et al. (2020). An Efficient Photodynamic Therapy Treatment for Human Pancreatic Adenocarcinoma. J. Clin. Med. 9, 192. doi: 10.3390/jcm9010192
Romano, G., Calusi, S., Gnerucci, A., Orsini, B., Faraoni, P., Tortora, G., et al. (2016). “Optical modelling of the gastric tissue to optimize the phototherapy efficacy against H. pylori infection∗,” in Proceedings of the 18th Italian National Conference on Photonic Technologies (Fotonica 2016), (Rome).
Romiti, R., Schaller, M., Jacob, K., and Plewig, G. (2000). High-performance liquid chromatography analysis of porphyrins in Propionibacterium acnes. Arch. Dermatol. Res. 292, 320. doi: 10.1007/s004030000122
Schindl, A., Rosado-Sholosser, B., and Trautinger, F. (2001). Reciprocity in photobiology. An overview. Dermatologist. 52, 779. doi: 10.1007/s001050170065
Schmid, J., Hoenes, K., Vatter, P., and Hessling, M. (2019). Antimicrobial Effect of Visible Light—Photoinactivation of Legionella rubrilucens by Irradiation at 450, 470, and 620 nm. Antibiotics. 8, 187. doi: 10.3390/antibiotics8040187
Sepúlveda Cisternas, I., Salazar, J. C., and García-Angulo, V. A. (2018). Overview on the Bacterial Iron-Riboflavin Metabolic Axis. Front. Microbiol. 9:1478. doi: 10.3389/fmicb.2018.01478
Shu, M., Kuo, S., Wang, Y., Jiang, Y., Liu, Y. T., Gallo, R. L., et al. (2013). Porphyrin metabolisms in human skin commensal Propionibacterium acnes bacteria: potential application to monitor human radiation risk. Curr. Med. Chem. 20, 562–568. doi: 10.2174/0929867311320040007
Smijs, T. G., and Pavel, S. (2011). The Susceptibility of Dermatophytes to Photodynamic Treatment with Special Focus on Trichophyton rubrum. Photochem. Photobiol. 87, 2–13. doi: 10.1111/j.1751-1097.2010.00848.x
Taraszkiewicz, A., Szewczyk, G., Sarna, T., Bielawski, K. P., and Nakonieczna, J. (2015). Photodynamic inactivation of Candida albicans with imidazoacridinones: Influence of irradiance, photosensitizer uptake and reactive oxygen species generation. PLoS One 10:129301. doi: 10.1371/journal.pone.0129301
Thomas, M., Craik, J. D., Tovmasyan, A., Batinic-Haberle, I., and Benov, L. T. (2015). Amphiphilic cationic Zn-porphyrins with high photodynamic antimicrobial activity. Future Microbiol. 10, 709–724. doi: 10.2217/fmb.14.148
Tomb, R. M., White, T. A., Coia, J. E., Anderson, J. G., MacGregor, S. J., and Maclean, M. (2018). Review of the Comparative Susceptibility of Microbial Species to Photo-inactivation Using 380–480 nm Violet-Blue Light. Photochem. Photobiol. 94, 445–458. doi: 10.1111/php.12883
Tortora, G., Orsini, B., Pecile, P., Menciassi, A., Fusi, F., Romano, G., et al. (2016). An Ingestible Capsule for the Photodynamic Therapy of Helicobacter pylori infection.
Van Straten, D., Mashayekhi, V., De Bruijn, H. S., Oliveira, S., and Robinson, D. J. (2017). Oncologic Photodynamic Therapy: Basic Principles, Current Clinical Status and Future Directions. Cancers. 9, 19. doi: 10.3390/cancers9020019
Wang, Y., Ferrer-Espada, R., Baglo, Y., Goh, X. S., Held, K. D., Grad, Y. H., et al. (2019). Photoinactivation of Neisseria gonorrhoeae: a paradigm-changing approach for combating antibiotic-resistant gonococcal infection. J. Infect. Dis. 220, 873–881. doi: 10.1093/infdis/jiz018
Wang, Y., Wang, Y., Wang, Y., Murray, C. K., Hamblin, M. R., Hooper, D. C., et al. (2017). Antimicrobial blue light inactivation of pathogenic microbes: State of the art. Drug Resist. Updat. 3, 1–22. doi: 10.1016/j.drup.2017.10.002
Wang, Y., Wu, X., Chen, J., Amin, R., Lu, M., Bhayana, B., et al. (2016). Antimicrobial Blue Light Inactivation of Gram-Negative Pathogens in Biofilms: In Vitro and In Vivo Studies. J. Infect. Dis. 213, 1380–1387. doi: 10.1093/infdis/jiw070
Wilder-Smith, C. H., Wilder-Smith, P., Grosjean, P., van den Bergh, H., Woodtli, A., Monnier, P., et al. (2002). Photoeradication of Helicobacter pylori using 5-aminolevulinic acid: Preliminary human studies. Lasers Surg. Med. 31, 18–22. doi: 10.1002/lsm.10066
World Health Organization [Who] and International Agency for Research on Cancer [IARC] (2019). Monographs on the identification of carcinogenic hazards to humans. Geneva: WHO.
Wroblewski, L. E., Peek, R. M. Jr., and Wilson, K. T. (2010). Helicobacter pylori and gastric cancer: factors that modulate disease risk. Clin. Microbiol. Rev. 23, 713–739. doi: 10.1128/CMR.00011-10
Yoshida, A., Sasaki, H., Toyama, T., Araki, M., Fujioka, J., Tsukiyama, K., et al. (2017). Antimicrobial effect of blue light using Porphyromonas gingivalis pigment. Sci Rep. 7, 5225. doi: 10.1038/s41598-017-05706-1
Zhang, Y., Zhu, Y., Chen, J., Wang, Y., Sherwood, M. E., Murray, C. K., et al. (2016). Antimicrobial blue light inactivation of Candida albicans: In vitro and in vivo studies. Virulence. 7, 536–545. doi: 10.1080/21505594.2016.1155015
Zhang, Y., Zhu, Y., Gupta, A., Huang, Y., Murray, C. K., Vrahas, M. S., et al. (2014). Antimicrobial blue light therapy for multidrug-resistant Acinetobacter baumannii infection in a mouse burn model: implications for prophylaxis and treatment of combat-related wound infections. J. infec dis. 209, 1963–1971. doi: 10.1093/infdis/jit842
Keywords: Helicobacter pylori, antimicrobial PDT, LEDs, porphyrins, flavins
Citation: Morici P, Battisti A, Tortora G, Menciassi A, Checcucci G, Ghetti F and Sgarbossa A (2020) The in vitro Photoinactivation of Helicobacter pylori by a Novel LED-Based Device. Front. Microbiol. 11:283. doi: 10.3389/fmicb.2020.00283
Received: 10 December 2019; Accepted: 07 February 2020;
Published: 21 February 2020.
Edited by:
Fabian Cieplik, University Medical Center Regensburg, GermanyReviewed by:
Tianhong Dai, Harvard Medical School, United StatesJoanna Nakonieczna, Medical University of Gdańsk, Poland
Copyright © 2020 Morici, Battisti, Tortora, Menciassi, Checcucci, Ghetti and Sgarbossa. This is an open-access article distributed under the terms of the Creative Commons Attribution License (CC BY). The use, distribution or reproduction in other forums is permitted, provided the original author(s) and the copyright owner(s) are credited and that the original publication in this journal is cited, in accordance with accepted academic practice. No use, distribution or reproduction is permitted which does not comply with these terms.
*Correspondence: Antonella Sgarbossa, YW50b25lbGxhLnNnYXJib3NzYUBuYW5vLmNuci5pdA==