- 1Department of Sustainable Agro-Ecosystems and Bioresources, Research and Innovation Centre, Fondazione Edmund Mach, San Michele all’Adige, Italy
- 2Biological Products for Agriculture (Bi-PA), Londerzeel, Belgium
- 3Department of Plant Induced Resistance and Bioprotection, University of Reims Champagne-Ardenne, Reims, France
- 4Department of Biology, University of Padua, Padua, Italy
- 5Botanical Garden, University of Padua, Padua, Italy
- 6Center Agriculture Food Environment (C3A), University of Trento, San Michele all’Adige, Italy
Rare sugars are monosaccharides with limited availability in nature and their biological functions are largely unknown. Among them, tagatose was developed as a low-calorie sweetener and showed beneficial effects on human health. Tagatose is metabolized by only certain microbial taxa and inhibits the growth of important crop pathogens (e.g., Phytophthora infestans), but its mode of action and the microbial responses are unknown. The aim of this study was to understand the tagatose mode of action against Phytophthora spp., with the final aim of developing new plant protection products. Tagatose inhibited P. infestans growth in vitro and caused severe ultrastructural alterations, with the formation of circular and concentric mitochondrial cristae. Decreased ATP content and reduced oxygen consumption rate (OCR) were found in tagatose-incubated P. infestans as compared to the control, with the consequent accumulation of reactive oxygen species (ROS) and induction of genes related to apoptosis and oxidative stress response. On the other hand, tagatose did not, or only slightly, affect the growth, cellular ultrastructure and mitochondrial processes in Phytophthora cinnamomi, indicating a species-specific response to this rare sugar. The mode of action of tagatose against P. infestans was mainly based on the inhibition of mitochondrial processes and this rare sugar seems to be a promising active substance for the further development of eco-friendly fungicides, thanks to its anti-nutritional properties on some phytopathogens and low risk for human health.
Introduction
Rare sugars are monosaccharides and their derivatives that rarely exist in nature (Granström et al., 2004). The ecological role of rare sugars is not fully understood and their promising biological properties are underestimated, mainly due to their limited availability in terms of quantity in nature (Li et al., 2013). The implementation of industrial enzymatic and microbial processes lowered the cost of rare sugar synthesis (Granström et al., 2004; Izumori, 2006; Oh, 2007) and made scientific studies and technological applications of these carbohydrates more accessible (Oh, 2007; Li et al., 2013). Twenty hexoses (e.g., tagatose, allose, gulose, and sorbose) and nine pentoses (e.g., lyxose, xylulose, and xylitol) have been classified as rare sugars by the international society of rare sugars (Ahmed, 2001; Jayamuthunagai et al., 2017). Among them, tagatose is a ketohexose that was found naturally at low concentration (<3 mg/g) in many foods, such as apples, oranges, and milk (Vastenavond et al., 2011). Tagatose was “generally recognized as safe” by the Food and Drug Administration as it does not have negative impacts on human health (Levin, 2002; Vastenavond et al., 2011). Thanks to its safety for human health, reduced caloric value and physical properties similar to those of sucrose (sweetness, color, and texture), tagatose was approved for use as low-calorie sweetener in several countries, European Union and United States included (Vastenavond et al., 2011).
Tagatose also shows beneficial effects and therapeutic properties on humans and it was proposed for the treatment of “type 2” diabetes, hyperglycemia, anemia, and hemophilia (Levin, 2002). Moreover, tagatose affects the growth of human-associated microorganisms, inhibiting biofilm formation and co-aggregation of the oral bacteria (streptococci and actinomycetes) responsible for dental plaque formation (Levin and Lu, 2007). In particular, prebiotic properties on the human gut microbiome were attributed to tagatose, for example it increases the abundance of beneficial bacteria, such as Enterococcus spp. and Lactobacillus spp. (Bertelsen et al., 1999; Vastenavond et al., 2011; Hasibul et al., 2018). On the other hand, tagatose inhibits the growth of human pathogenic bacteria, such as Streptococcus mutants and Salmonella enterica serovar Typhimurium (Lobete et al., 2017; Hasibul et al., 2018). Likewise, tagatose is not catabolized by some human pathogens, such as Bacillus cereus, Escherichia coli, Listeria monocytogenes, Staphylococcus aureus, and Yersinia enterocolitica (Bautista et al., 2000), indicating its nutritional or anti-nutritional effects on specific microbial taxa. Tagatose can be utilized as a carbohydrate source by only certain microbial taxa, such as Exiguobacterium spp., Lactobacillus spp., and Lactococcus spp. (Raichand et al., 2012; Martinussen et al., 2013; Van Der Heiden et al., 2013; Wu and Shah, 2017). In particular, tagatose can be transported into microbial cells by the phosphotransferase uptake systems and used as an intermediate in the lactose, galactose, and galactitol catabolism by some bacterial species (Van Der Heiden et al., 2013). For example, the Lactobacillus spp. and Lactococcus spp. metabolism includes the tagatose-6-phosphate pathway (Martinussen et al., 2013; Wu and Shah, 2017) and the incubation of Lactobacillus rhamnosus with tagatose triggered a complex transcriptional reprograming of the carbohydrate metabolism with activation of the phosphotransferase system (Koh et al., 2013).
In plants, tagatose inhibits the growth of some phytopathogens and it was patented to control important crop diseases, such as tomato and potato late blight (P. infestans), cucumber downy mildew (Pseudoperonospora cubensis), grape downy mildew (Plasmopara viticola), cucumber powdery mildew (Sphaerotheca fuliginea), wheat fusarium blight (Puccinia recondita), cabbage downy mildew (Peronospora parasitica), rice and cucumber damping-off disease (Pythium graminicola and Pythium aphanidermatum) (Ohara et al., 2008). Among them, P. infestans causes severe economic losses on potato, tomato, and eggplant (Fry et al., 2015) and the Phytophthora genus comprises some of the most aggressive and widespread plant pathogens (Kamoun, 2000). For example, Phytophthora cinnamomi causes considerable damage to agricultural, horticultural and forest plants, with more than 3000 host species, including avocado, chestnut, and pineapple (Hardham, 2005).
Potato late blight, caused by P. infestans, has an estimated cost for growers of about 5 billion dollars per year and requires frequent applications of plant-protection products (Judelson and Blanco, 2005) with a consequent negative impact on human health and the environment (Fantke et al., 2012). Thus, tagatose has been previously suggested as a possible alternative to synthetic chemical fungicides (Ohara et al., 2008), thanks to the absence of deleterious effects on human health (Levin, 2002; Vastenavond et al., 2011). Tagatose showed also possible plant prebiotic effects on the phyllosphere microbiota and modified the balance of potential pathogenic and potential beneficial microorganisms by selective nutritional and anti-nutritional properties for some specific microbial taxa (Perazzolli et al., 2020). However, deeper investigations are required to clarify the growth inhibition properties of tagatose on phytopathogens, because the mechanism of action is still unknown. In addition, tagatose did not inhibit the mycelial growth of Aspergillus niger, Cladosporium cladosporioides, and Penicillium chrysogenum (Izumori et al., 2008) and it promoted the spore germination of A. niger (Hayer et al., 2013), indicating the absence of growth inhibition on some plant-associated microorganisms. More specifically, tagatose supported the growth of Trichoderma harzianum and Trichoderma pleuroticola, but not that of Trichoderma pleurotum (Komon-Zelazowska et al., 2007), indicating nutritional or anti-nutritional effects also within species belonging to the same genus. The variability in the response of plant-associated microorganisms to tagatose requires more information on its physiological and molecular effects, to further develop innovative biopesticides based on this active substance. The aim of this study was to clarify the mode of action of tagatose and the cellular responses in two phytopathogenic Phytophthora spp. in vitro, in order to provide deeper knowledge for the further development of eco-friendly fungicides for sustainable plant protection.
Materials and Methods
Biological Material, Growth Conditions and Treatments
Phytophthora infestans strain VB3 and P. cinnamomi strain CBS 144.22 were stored in glycerol at −80°C in the fungal collection of the Fondazione Edmund Mach, Italy, and they are freely available upon request. P. infestans and P. cinnamomi were grown in Petri dishes on pea agar medium (PAM, 12.5% frozen peas and 1.2% agar in distilled water) at 18 ± 1 and 25 ± 1°C, respectively (Puopolo et al., 2014).
The P. infestans and P. cinnamomi mycelial suspension was prepared by collecting small mycelial fragments from 4-days-old colonies. Briefly, Petri dishes of P. infestans or P. cinnamomi colonies were filled with 2 mL pea broth (PB, 12.5% frozen peas in distilled water), small mycelia fragments were scraped with a sterile spatula and the mycelial suspension was filtered using a sterile Pasteur pipette containing a fine mesh. The liquid culture of P. infestans or P. cinnamomi was obtained in 10 mL PB inoculated with 100 μL of the mycelial suspension and incubated at 18 ± 1 and 25 ± 1°C under orbital shaking at 80 rpm, respectively.
The stock solution (50 g/L in distilled water) of each rare sugar, such as tagatose (Bi-PA, Londerzeel, Belgium), psicose (Carbosynth, Compton, United Kingdom), and sorbose (Carbosynth), was filter sterilized and added at the appropriate final concentration (5 or 10 g/L) in PAM or PB shortly before Phytophthora spp. inoculation. Filter sterilized oligomycin (Sigma-Aldrich, St. Louis, MO, United States) was used as control treatment at the final concentration of 10 μg/mL, since it is known as a growth inhibitor of fungi, such as Aspergillus spp., Candida spp., and Penicillium spp. (Eliskases-Lechner and Prillinger, 1996), through inhibition of ATP synthase activity (Manfredi et al., 2002; Kabala et al., 2014) and mitochondrial respiration (Galloway et al., 2012; Kooragayala et al., 2015).
Assessment of Rare Sugar Impact on Phytophthora spp. Radial Growth
Phytophthora spp. plugs (5 mm diameter) were cut from the edge of 14-days-old colonies and a plug was placed at the center of each Petri dish (90 mm diameter) on PAM augmented with the appropriate concentration of the rare sugar. The radial growth of P. infestans and P. cinnamomi was assessed 4 and 10 days after incubation (DAI) at 18 ± 1 and 25 ± 1°C, respectively, calculated as the average of the two perpendicular diameters of the colony, minus the plug diameter and the result divided by two. Plugs of P. infestans and P. cinnamomi mycelia developed on PAM in the presence of tagatose were transferred on new PAM dishes and the growth was then monitored as reported above. Ten replicates (dishes) were used for each treatment and the experiment was carried out twice.
Ultrastructural Analysis by Transmission Electron Microscopy
The P. infestans and P. cinnamomi liquid cultures were collected at 4 and 10 DAI in PB in the absence (control) and presence of 5 g/L tagatose or 10 μg/mL oligomycin, for transmission electron microscopy (TEM) analysis. Each mycelial sample (0.3 cm3) was transferred into a 2 mL tube and incubated with 500 μL of fixing solution (3% glutaraldehyde in 0.1 M cacodylate buffer, pH 7.4) (Zuppini et al., 2010) for 1 h at room temperature under rotary shaking at 15 rpm and then for 15 h at 4°C. Samples were washed three times in 500 μL of cacodylate buffer (0.1 M, pH 7.4), post-fixed for 2 h with 1% (w/v) osmium tetroxide in 0.1 M cacodylate buffer (pH 7.4) and dehydrated in graded ethanol series of 25, 50, 75, and 100% (v/v) with an incubation of 15 min at room temperature for each ethanol concentration (Zuppini et al., 2010). Samples were infiltrated in 1:3 (v/v) araldite resin:propylene oxide (Sigma-Aldrich) by incubating for 1 h at 40°C, followed by 1:1 (v/v) araldite resin:propylene oxide for 1 h at 40°C and 3:1 araldite resin:propylene oxide overnight at 40°C. Samples were subsequently embedded in 100% araldite resin by incubating for 24 h at 40°C and for 72 h at 60°C. Ultra-thin sections (70 nm) were obtained on a Reichert-Jung ultramicrotome (Leica Biosystems, Wetzlar, Germany) and mounted on uncoated copper grids. Sections were then stained with 1% uranyl acetate (in 50% ethanol) for 15 min and 1% lead citrate for 7 min. Observations were carried out with a Tecnai G2 transmission electron microscope (Field Electron and Ion Company, Hillsboro, OR, United States) operating at 100 kV and equipped with a Osis Veleta camera (Olympus, Tokyo, Japan). Two replicates (tubes) were analyzed for each treatment and time point and the experiment was carried out twice.
Assessment of the ATP Content
The P. infestans and P. cinnamomi mycelial suspension (200 μL) was incubated for 24 h in PB in the absence (control) and presence of 5 g/L tagatose or 10 μg/mL oligomycin in white 96-well microplate with clear flat bottom (Corning, New York, NY, United States) under orbital shaking at 80 rpm at 18 ± 1 and 25 ± 1°C, respectively. Cellular ATP content was quantified using an ATPlite luminescence assay kit (Perkin Elmer, Waltham, MA, United States) according to manufacturer’s instructions (Lis et al., 2016). Briefly, each mycelial suspension was supplemented with 100 μL of lysis buffer under vigorous shaking at 700 rpm for 10 min and 100 μL were then transferred into a 96-well microplate with a transparent flat bottom (Costar, Corning) for the subsequent protein quantification. In each well, 50 μL of substrate solution (luciferin and luciferase) were added after discarding 50 μL of each sample. The luminescence resulting from the reaction of ATP was measured using a Synergy 2 Multi-Mode Microplate Reader (Biotek, Winooski, VT, United States). An ATP standard curve (0.001, 0.01, 0.1, 1, 10, 100, and 1000 nmol/L) was used as reference to calculate the ATP content.
For protein quantification, each sample (100 μL) was mixed with 100 μL Bradford reagent (Pierce Coomassie Plus, Thermo Fisher Scientific), the 96-well microplate was incubated for 10 min at room temperature and the absorbance at 595 nm was measured with a Synergy 2 Multi-Mode Microplate Reader (Biotek). A standard curve of bovine serum albumin (Sigma-Aldrich; 0, 0.01, 0.05, 0.1, and 0.2 mg/mL) was used as reference to determine the protein concentration of each sample and the ATP content was then expressed per unit of total proteins (nmol/mg) (Smith et al., 2016). Three replicates (wells) were assessed for each treatment and the experiment was carried out twice.
Assessment of the Oxygen Consumption Rate
The P. infestans and P. cinnamomi mycelial suspension (100 μL) was incubated for 16 h in PB in the absence (control) and presence of 5 g/L tagatose or 10 μg/mL oligomycin in a black 96-well microplate with Corning under orbital shaking at 80 rpm at 18 ± 1 and 25 ± 1°C, respectively. The oxygen consumption rate (OCR) was measured using the MitoXpress Xtra Oxygen Consumption Assay (Luxcel Biosciences, Agilent, Santa Clara, CA, United States) fluorescent probe for the real-time analysis of cellular respiration (Calmes et al., 2015). An aliquot (50 μL) of the liquid media was removed from each well by aspiration with a micropipette and 50 μL of fresh PB containing 100 nM MitoXPress were added and overlaid with 100 μL of mineral oil, to exclude ambient air. Oxygen depletion in the medium was assessed as the increase in the fluorescence lifetime (FLT) of the probe, using a Synergy 2 Multi-Mode Microplate Reader equipped with a time-resolved fluorescence head (Biotek). The relative fluorescence units (RFU) (340 nm excitation, 605–705 nm emission) were recorded twice for 30 μsec, with a delay of 40 μsec (after 30 and 70 μs) at 0, 4, and 8 h of reaction time at 18 ± 1 and 25 ± 1°C for P. infestans and P. cinnamomi under orbital shaking at 80 rpm, respectively. The FLT of each sample was calculated based on the RFU with 40 μsec delay (Calmes et al., 2015) as follows:
where, 40 μsec is the delay time between the two measurements; RFU1 is the signal measured after 30 μsec and RFU2 is the signal measured after 70 μsec. Three replicates (wells) were assessed for each treatment and the experiment was carried out twice.
Quantification of Intracellular Reactive Oxygen Species
The P. infestans and P. cinnamomi mycelial suspension (100 μL) was incubated for 16 h in PB in the absence (control) and presence of 5 g/L tagatose in a black 96-well microplate with Corning under orbital shaking at 80 rpm at 18 ± 1 and 25 ± 1°C, respectively. As control treatment, 2 mM H2O2 was added to increase reactive oxygen species (ROS) generation, as previously reported for Aspergillus fumigatus (Shekhova et al., 2017). Intracellular ROS were quantified with 2′,7′-dichlorodihydrofluorescein diacetate (H2DCF-DA; Molecular Probes, Thermo Fisher Scientific) as previously described (Shekhova et al., 2017) with slight modifications. Briefly, 1 μL H2DCF-DA (300 μM) was added to each well and the 96-well microplate was incubated for 1 h in the dark under orbital shaking at 80 rpm, at 18 ± 1 and 25 ± 1°C for P. infestans and P. cinnamomi, respectively. The mycelial suspension was centrifuged at 200 rpm for 2 min, 50 μL of the liquid media were removed by aspiration with a micropipette and replaced with 50 μL of fresh PB to remove the excess of unreacted fluorescent probe. Intracellular ROS were quantified at 0, 1, and 2 h of reaction time by measuring the fluorescence intensity using a Synergy 2 Multi-Mode Microplate Reader (Biotek) with an excitation filter at 485 nm and an emission filter at 530 nm, at 18 ± 1°C for P. infestans and at 25 ± 1°C for P. cinnamomi (Wong et al., 2018). Three replicates (wells) were assessed for each treatment and the experiment was carried out twice.
Primer Design for Gene Expression Analysis
The Phytophthora spp. gene markers related to sugar metabolism, respiration process, oxidative stress response and apoptosis were selected for quantitative real-time PCR (qPCR) analysis. For each gene, a primer pair compatible for the P. infestans and P. cinnamomi sequence was designed on conserved coding regions (Supplementary Table S1) and PCR products were sequenced on both strands using an AB3730xl instrument (Applied Biosystems, Thermo Fisher Scientific) at the sequencing platform facility of Fondazione Edmund Mach as validation.
RNA Extraction and Gene Expression Analysis
The P. infestans and P. cinnamomi mycelium was collected at 4 and 10 DAI in PAM covered with sterile cellophane layers in the absence (control) and presence of 5 g/L tagatose at 18 ± 1 and 25 ± 1°C, respectively. Samples were immediately frozen in liquid nitrogen, stored at −80°C and crushed using a mixer mill disruptor (MM200, Retsch, Haan, Germany) at 25 Hz for 45 s with sterile steel jars and beads refrigerated in liquid-N2. Total RNA was extracted from 100 mg of ground Phytophthora spp. mycelium using the Spectrum Plant total RNA kit (Sigma-Aldrich). RNA was quantified by NanoDrop 8000 (Thermo Fisher Scientific, Wilmington, DE, United States), treated with DNase I (Invitrogen, Thermo Fisher Scientific) and the first strand cDNA was synthesized from 1 μg of total RNA using Superscript III (Invitrogen, Thermo Fisher Scientific) and oligo-dT primer. qPCR reactions were carried out with Platinum SYBR Green qPCR SuperMix-UDG (Invitrogen, Thermo Fisher Scientific) and specific primers (Supplementary Table S1) using the Light Cycler 480 (Roche Diagnostics, Mannheim, Germany) as previously described (Perazzolli et al., 2011). Briefly, the PCR conditions were: 50°C for 2 min and 95°C for 2 min as initial steps, followed by 40 cycles at 95°C for 15 s and at 60°C for 1 min. Each sample was examined in three technical replicates and dissociation curves were analyzed to verify the specificity of each amplification reaction. Three housekeeping genes were analyzed, β-tubulin (tub-b) (Yan and Liou, 2006), exosome complex exonuclease subunit Rrp42 and exosome complex exonuclease subunit Rrp43 (also called exosome ribonuclease) (Judelson et al., 2008), and their stability was validated using the ΔCt method described by Silver et al. (2006). Briefly, a qPCR was carried out for the three housekeeping genes on all samples and tub-b was selected as constitutive gene for normalization, because tub-b expression was not affected by the treatments (i.e., lowest standard deviation among the housekeeping genes tested). For the gene expression analysis, Light Cycler 480 SV1.5.0 software (Roche) was used to extract Ct values based on the second derivative calculation and the LinReg software version 11.0 was used to calculate reaction efficiencies for each primer pair (Ruijter et al., 2009). The relative expression level (fold change) of each gene was then calculated according to the Pfaffl equation (Pfaffl, 2001) for tagatose-incubated samples as compared to the respective control samples (calibrator) for each time point and Phytophthora spp., using tub-b as constitutive gene for normalization. Five replicates (dishes with 10 plugs in each dish) were assessed for each treatment and the experiment was carried out twice.
Statistical Analysis
All experiments were carried out twice and data were analyzed with Statistica 13.1 software (Dell, Round Rock, TX, United States). Normal distribution (Kolmogorov–Smirnov test, P > 0.05) and variance homogeneity of the data (Levene’s tests, P > 0.05) were checked and parametric tests were used when both assumptions were respected. Each experimental repetition was analyzed singularly and a two-way analysis of variance (ANOVA) was used to demonstrate non-significant differences between the two experiments (P > 0.05). Data from the two experimental repetitions were pooled and significant differences were assessed with the Student’s t-test (P ≤ 0.05) or Tukey’ test (P ≤ 0.05) in case of pairwise or multiple comparisons, respectively. Fold change values of gene expression analysis were transformed using the equation y = Log10 (1 + x) (Casagrande et al., 2011). When parametric assumptions were not respected, the Kruskal–Wallis test was used to demonstrate non-significant differences between the two experimental repetitions (P > 0.05), then data from the two experiments were pooled and a Kruskal–Wallis test was used to detect significant differences among treatments (P ≤ 0.05).
Results
Tagatose Differentially Inhibits Phytophthora infestans and P. cinnamomi Growth and Causes Ultrastructural Alterations
Phytophthora infestans growth was inhibited by tagatose at 4 and 10 DAI and the level of inhibition using 5 and 10 g/L tagatose was comparable at each time point (Figure 1A). Conversely, P. cinnamomi growth was not affected by 5 and 10 g/L tagatose at 10 DAI, and only a slight inhibition was observed with 10 g/L tagatose at 4 DAI (Figure 1B). When transferred to new PAM dishes, the growth of P. infestans plugs collected at 10 DAI with 5 and 10 g/L tagatose was comparable to the growth of P. infestans plugs collected at 10 DAI from control dishes (data not shown), indicating that the effect of tagatose was reversible.
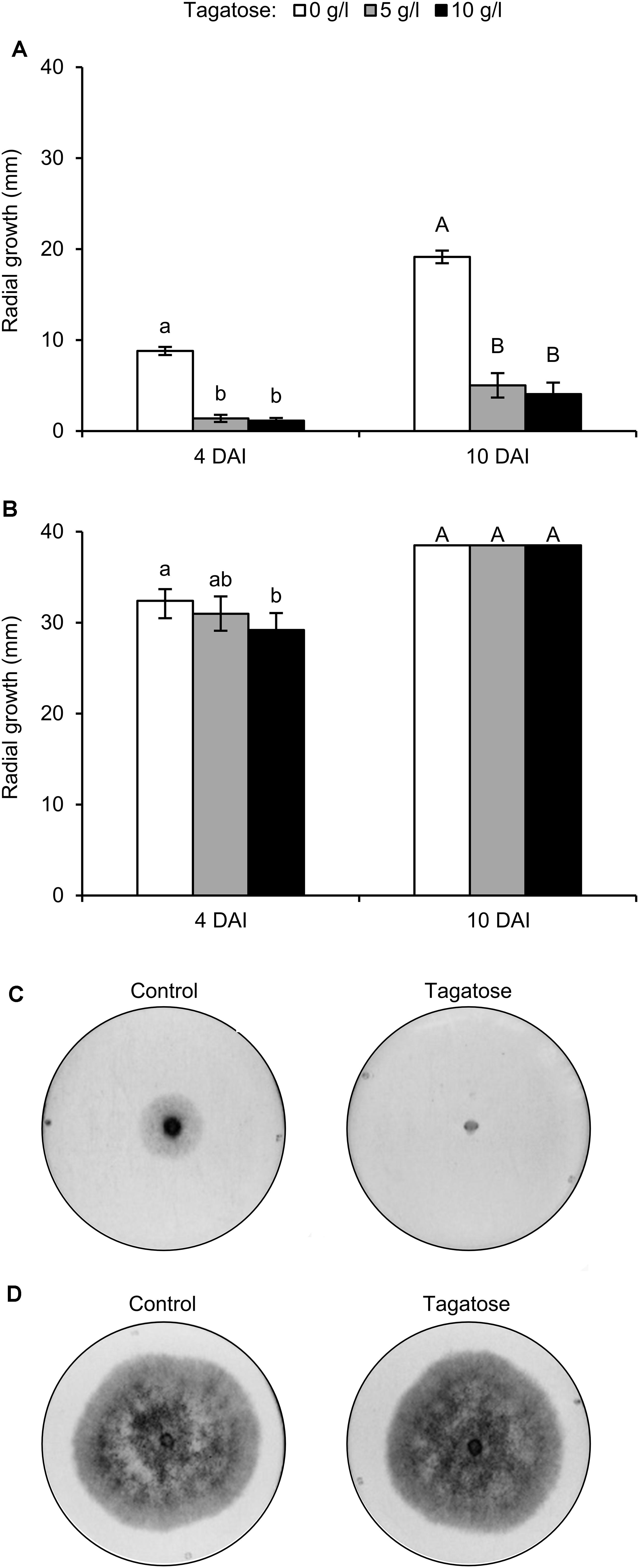
Figure 1. Effect of tagatose on Phytophthora spp. growth. Phytophthora infestans (A) and P. cinnamomi (B) growth (mm) was assessed 4 and 10 days after incubation (DAI) on pea agar medium in the absence (white, Control) and presence of 5 (gray), or 10 g/L (black) tagatose. The radial growth was calculated as the average of the two perpendicular diameters of the colony, minus the plug diameter and the result divided by two. The Kruskal–Wallis test showed no significant differences between the two experimental repetitions (P > 0.05, 10 replicates per experiment) and data from the two experiments were pooled. Mean and standard error values of 20 replicates (dishes) from the two experiments are presented for each treatment. Different lowercase and uppercase letters indicate significant differences among treatments at 4 and 10 days after incubation (DAI) according to the Kruskal–Wallis test (P ≤ 0.05), respectively. Representative pictures of P. infestans (C) and P. cinnamomi (D) growth 4 DAI in the absence (Control) and presence of 5 g/L tagatose are shown.)
In order to verify the specificity of tagatose, isomers were tested, such as fructose, psicose, and sorbose. The incubation with 5 g/L fructose, 5 g/L psicose, or 5 g/L sorbose did not inhibit P. infestans and P. cinnamomi growth at 4 DAI (Supplementary Figure S1) and 10 DAI (data not shown). Because the minimum dosage showing a differential effect on Phytophthora spp. growth was 5 g/L tagatose (Figures 1C,D), this quantity was selected for the following experiments.
In order to investigate morphological impacts of tagatose incubation on cellular structures, TEM analyses were carried out. The typical ultrastructure of Phytophthora spp. (Xu et al., 2007) was observed by TEM analysis of P. infestans collected at 4 DAI (Figures 2A,B) and 10 DAI in PB (Figures 2C,D). Conversely, the structure of mitochondria was severely altered in tagatose-incubated P. infestans samples, displaying the reorganization of mitochondrial cristae at 4 DAI (Figures 2E,F) that leads to circular and concentric cristae at 10 DAI (Figures 2G,H). In the case of P. cinnamomi, tagatose did not affect cellular structures at 4 DAI (Figures 2M,N) as compared to the control (Figures 2I–L). Slight mitochondrial alterations, consisting of a less dense mitochondrial matrix with a sporadic occurrence of circular cristae, were found in P. cinnamomi only at 10 DAI (Figures 2O,P). The ATP synthase inhibitor oligomycin (Manfredi et al., 2002; Kabala et al., 2014), known to impair fungal growth (Eliskases-Lechner and Prillinger, 1996) and mitochondrial respiration (Galloway et al., 2012; Kooragayala et al., 2015), caused severe mitochondrial alterations in both P. infestans and P. cinnamomi already at 4 DAI and showed either the disappearance or profound rearrangement of the mitochondrial cristae (Supplementary Figure S2). Taken together, these observations indicated that tagatose altered the mitochondrial structure of P. infestans with consequent inhibition of radial growth.
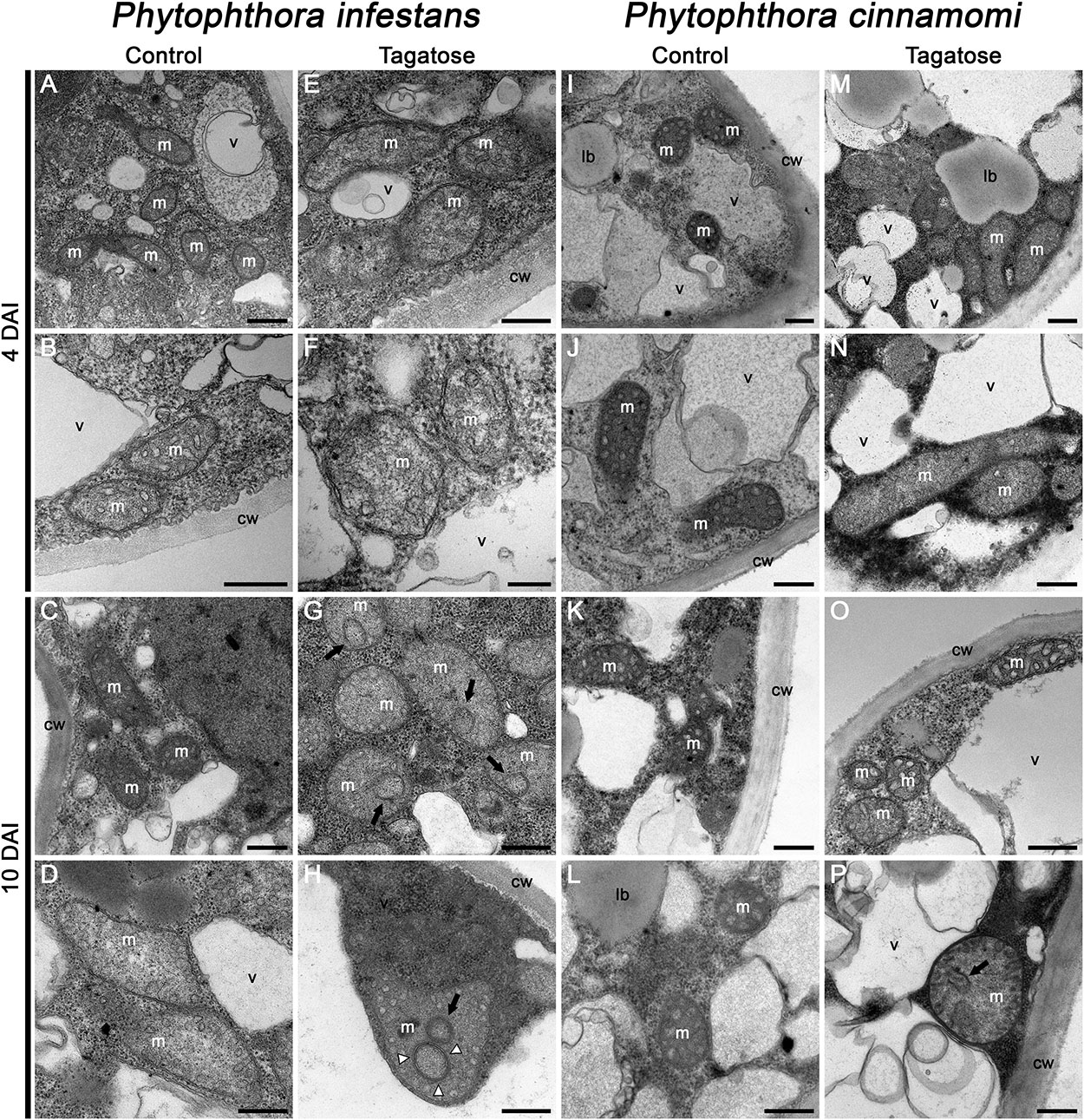
Figure 2. Effect of tagatose on Phytophthora spp. ultrastructure. Transmission electron microscopy observations of P. infestans (A–H) and P. cinnamomi (I–P) liquid culture were carried out 4 and 10 days after incubation (DAI) in pea broth either in the absence (Control) or the presence of 5 g/L tagatose. Two replicates (tubes) were analyzed for each treatment and the experiment was carried out twice with similar results. Circular (black arrows) and concentric (white arrowheads) mitochondrial cristae are indicated. cw, cell wall; lb, lipid bodies; m, mitochondria; v, vacuoles. Bars correspond to 500 nm (A–E, G–P) or 200 nm (F). Two representative pictures are reported for each treatment and time point as follow: A,B: P. infestans control 4 DAI; C,D: P. infestans control 10 DAI; E,F: P. infestans tagatose 4 DAI; G,H: P. infestans tagatose 10 DAI; I,J: P. cinnamomi control 4 DAI; K,L: P. cinnamomi control 10 DAI; M,N: P. cinnamomi tagatose 4 DAI; O,P: P. cinnamomi tagatose 10 DAI.
Tagatose Negatively Affects Mitochondrial Activities in Phytophthora infestans and Not P. cinnamomi
Since mitochondrial cristae alterations have been associated with the dysfunction of ATP synthase activity in yeast (Paumard et al., 2002; Gavin et al., 2004; Weimann et al., 2008), the ATP content of Phytophthora spp. was assessed. Tagatose decreased the ATP content of P. infestans as compared to the control and the effect was comparable to that obtained with the ATP synthase inhibitor oligomycin (Figure 3A). The ATP content of P. cinnamomi was not affected by tagatose and it was decreased by only the ATP synthase inhibitor oligomycin (Figure 3B).
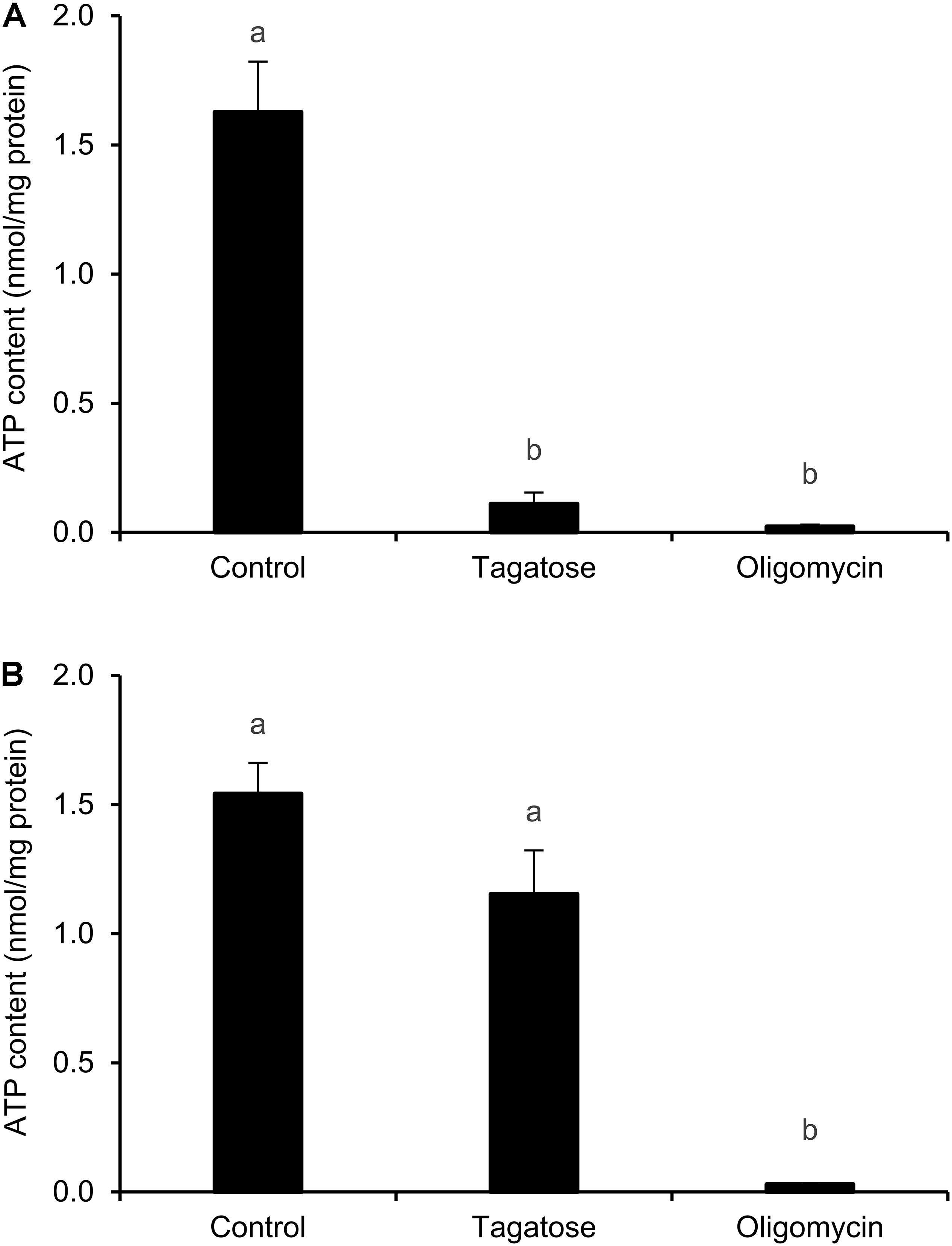
Figure 3. Effect of tagatose on ATP content of Phytophthora spp. The ATP content of P. infestans (A) and P. cinnamomi (B) mycelial suspension was assessed 24 h after incubation in pea broth in the absence (Control) and presence of 5 g/L tagatose or 10 μg/mL oligomycin. The ATP content was quantified using the luminescence assay and expressed per unit of total proteins (nmol/mg). The Kruskal–Wallis test showed no significant differences between the two experimental repetitions (P > 0.05, three replicates per experiment) and data from the two experiments were pooled. Mean and standard error values of six replicates (wells) from the two experiments are presented for each treatment. Different letters indicate significant differences among treatments according to the Kruskal–Wallis test (P ≤ 0.05).
The ATP synthase inhibition has been commonly linked to dysfunctions of the OCR (Galloway et al., 2012; Kooragayala et al., 2015) and ROS homeostasis (Martinez-Reyes and Cuezva, 2014) of eukaryotic cells. In P. infestans, we found that the OCR was inhibited by tagatose at 4 and 8 h reaction time and the FLT of tagatose-incubated samples was intermediate between that of control samples and samples incubated with the ATP synthase inhibitor oligomycin (Figure 4A). Conversely, P. cinnamomi OCR was not affected by tagatose and it was impaired by only the ATP synthase inhibitor oligomycin (Figure 4B). As possible consequence of ATP synthase and OCR inhibition, the ROS generation was increased by tagatose in P. infestans (Figure 4C), but not in P. cinnamomi (Figure 4D). As for other systems (Shekhova et al., 2017), H2O2 incubation increased the ROS level in both Phytophthora spp. In particular, the ROS fluorescence intensity of tagatose-incubated P. infestans was intermediate between that of control and H2O2-incubated samples. Taken together, these results showed that tagatose decreased the ATP content and OCR with a consequent increase of ROS accumulation in P. infestans, but not in P. cinnamomi.
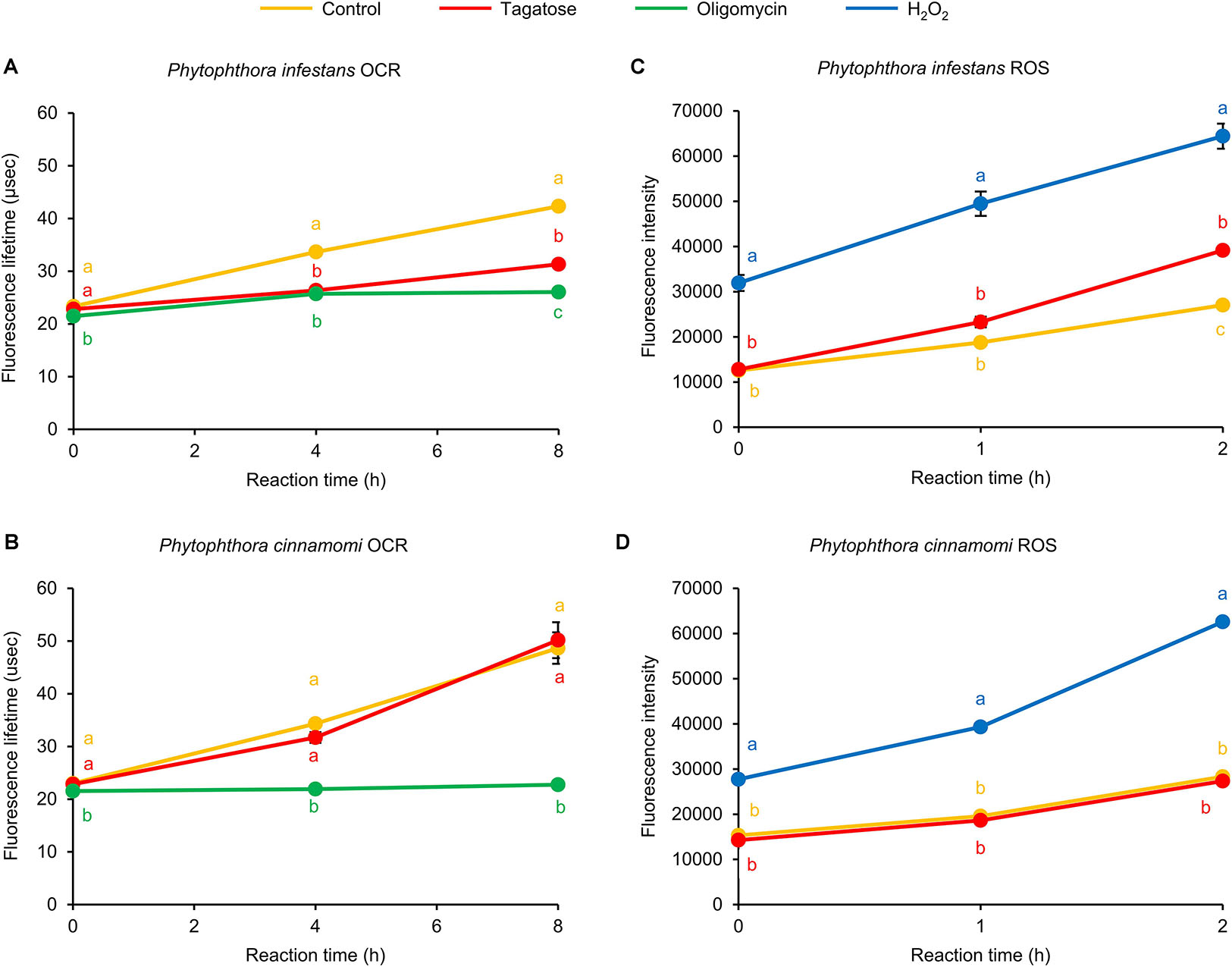
Figure 4. Effect of tagatose on Phytophthora spp. respiration processes and accumulation of reactive oxygen species (ROS). The oxygen consumption rate (OCR) and the generation of ROS of the P. infestans (A,C) and P. cinnamomi (B,D) mycelial suspension were assessed 16 h after incubation in pea broth in the absence (Control, orange) and presence of 5 g/L of tagatose (red). Oligomycin (10 μg/mL, green) and hydrogen peroxide (2 mM H2O2, blue) were used as control treatments of the OCR and ROS assay, respectively. The OCR was assessed as increase of fluorescence lifetime (μsec) at 0, 4, and 8 h of reaction time and ROS generation was quantified as fluorescence intensity at 0, 1, and 2 h of reaction time using fluorescent probes. For each assay, the factorial analysis of variance showed no significant differences between the two experimental repetitions (P > 0.05, three replicates per experiment) and data from the two experiments were pooled. Mean and standard error values of six replicates (wells) from the two experiments are presented for each treatment. Different letters indicate significant differences among treatments, according to the Tukey test (P ≤ 0.05).
Tagatose Modulates the Expression of Phytophthora spp. Genes
Phytophthora spp. genes encoding key enzymes of glycolysis were analyzed by qPCR (Supplementary Table S1), such as glucose-6-phosphate dehydrogenase (g6pd) and phosphofructokinase (pfk). Moreover, genes encoding ATP synthase subunits, responsible for ATP production (Yoshida et al., 2001) were selected, since their activity was previously linked to mitochondrial structure biogenesis in Saccharomyces cerevisiae (Paumard et al., 2002; Lefebvre-Legendre et al., 2005), such as the ATP synthase subunit 4 (atp4) and ATP synthase subunit beta (atpB). Maleylacetoacetate isomerase (maai) was analyzed and it shares sequence homology and key domains with glutathione S-transferase genes (gst) upregulated by oxidative stresses (Montibus et al., 2015). The apoptosis-inducing factor (aif) was analyzed as marker of ROS scavenging (Klein et al., 2002) and mitochondrial cristae regulation (Cheung et al., 2006), since it was upregulated in response to farnesol in Aspergillus nidulans (Savoldi et al., 2008). The pro-apoptotic serine protease (nma111) and cytochrome c (cytc) were selected as upregulated genes in response to graphene oxide (Zhu et al., 2017) and acetic acid in S. cerevisiae (Ludovico et al., 2002), respectively. Possible markers of oxidative phosphorylation were selected, such as genes encoding NADH dehydrogenase ubiquinone flavoprotein (ndufv) (Kuhn et al., 2015) and cytochrome c oxidase (cox) (Dufour et al., 2000), as well as the cellulose synthase (ces) responsible for cell wall biosynthesis (Blum et al., 2010).
Expression levels of genes related to apoptosis (aif and nma111) and oxidative stress response (maai) were upregulated by tagatose in P. infestans at 4 and 10 DAI (Table 1). The atp4 expression was upregulated by tagatose at 4 DAI, suggesting an attempted P. infestans response to the ATP decrease and ROS increase caused by tagatose. On the other hand, the expression of atpB, ces, cytc, cox, g6pd, ndufv, and pfk was not affected by tagatose in P. infestans. In P. cinnamomi, no genes of glycolysis (g6pd and pfk), ATP synthesis (atp4 and atpB), apoptosis (aif), oxidative phosphorylation (ndufv and cox), cellulose biosynthesis (ces), and oxidative stress response (maai and cytc) were modulated by tagatose at 4 DAI and 10 DAI, except for the nma111 downregulation at 10 DAI, as corroboration of slight tagatose effects on P. cinnamomi.
Discussion
Tagatose is a rare sugar that can be metabolized by only certain microbial taxa (Raichand et al., 2012; Martinussen et al., 2013; Van Der Heiden et al., 2013; Wu and Shah, 2017) and inhibits some important crop pathogens, P. infestans included (Ohara et al., 2008). Nutritional and anti-nutritional effects of tagatose have been shown on human-associated microorganisms (Van Der Heiden et al., 2013; Lobete et al., 2017; Wu and Shah, 2017; Hasibul et al., 2018) and plant-associated microorganisms (Komon-Zelazowska et al., 2007; Izumori et al., 2008; Ohara et al., 2008; Hayer et al., 2013; Perazzolli et al., 2020). We showed that tagatose inhibited the growth of P. infestans, but it had only slight effects on P. cinnamomi, with a species-specific impact on the mitochondrial processes. P. infestans and P. cinnamomi were grown at the respective optimum temperature commonly used for fungicide assays in vitro (Coffey and Joseph, 1985; Groves and Ristaino, 2000; Yuan et al., 2006; Hu et al., 2010) and the contribution of temperature to the differential effect of tagatose cannot be totally excluded. The selectivity of action was previously observed on Trichoderma spp., where tagatose supported the growth of T. harzianum and T. pleuroticola, but not that of T. pleurotum (Komon-Zelazowska et al., 2007). Similar selectivity was reported in some bacterial genera, for example tagatose can be assimilated by Bacillus licheniformis (Van Der Heiden et al., 2013), Lactobacillus plantarum, Lactobacillus acidophilus, Lactobacillus brevis (Bautista et al., 2000), Lactobacillus casei, and L. rhamnosus (Koh et al., 2013), but not by B. cereus, Bacillus subtilis, and Lactobacillus buchneri (previously called Lactobacillus frigidus) (Bautista et al., 2000). Although all the rare sugars tested in this work were epimers (psicose, sorbose, and tagatose), only tagatose inhibited P. infestans growth, suggesting that structural differences among epimers may affect the inhibitory activities of rare sugars. The inhibition of P. infestans growth was reversible and tagatose-incubated plugs can normally grow when transferred on a new growth medium free of tagatose. This reversible effect raises the question of how to keep the persistence of tagatose on treated crops at constant and sufficient levels, when looking at a possible tagatose application as a plant protection product, and suggest that appropriated formulations should be probably developed for its application under field conditions.
Severe mitochondrial alterations with concentric cristae were found in tagatose-incubated P. infestans. Similar alterations have been previously observed in mammalian (Florea and Craciun, 2011) and yeast (Arselin et al., 2004) cells treated with inhibitors of mitochondrial activities, such as Apis mellifera venom and doxycycline, respectively. Likewise, some toxic compounds are known to form mitochondrial concentric cristae in target organisms, such as a phosphocholine derivative in Leishmania amazonensis (Godinho et al., 2013), benzimidazole anthelmintic in Haemonchus contortus (Cristina et al., 2015), and ethidium bromide in green Euglena spp. cells (Nass and Ben-Shaul, 1973). Moreover, xylitol and sorbose caused structural alterations of Coprinus lagopus cell wall (Moore and Stewart, 1972), Streptococcus mutans cell membrane (Nayak et al., 2014), and Neurospora crassa vesicle number and size (Trinci and Collinge, 1973), respectively. Mitochondrial cristae alterations were previously associated with the dysfunction of ATP synthase activity (Paumard et al., 2002; Gavin et al., 2004; Weimann et al., 2008) and with the reduction of mitochondrial bioenergetic status (Zick et al., 2009) in yeast cells. In particular, the formation of concentric cristae was associated with dimerization and oligomerization disorders of the ATP synthase in S. cerevisiae (Zick et al., 2009) with the consequent uncontrolled biogenesis of the inner mitochondrial membrane (Velours et al., 2009). The ATP synthase dimerization is associated to disulfide bonds between subunits codified by atp4 in S. cerevisiae (Paumard et al., 2002) and the upregulation of atp4 in P. infestans suggested an attempted cellular response against tagatose, in order to mitigate ATP4 dimerization effects. As consequence of mitochondrial alterations, the ATP content was decreased by tagatose in P. infestans, as previously reported in human subjects exposed to tagatose (Buemann et al., 2000) and isolated perfused liver treated with xylitol (Woods and Krebs, 1973). The ATP synthase inhibition has been commonly linked to the OCR reduction (Galloway et al., 2012; Kooragayala et al., 2015) and cellular redox state alteration (Martinez-Reyes and Cuezva, 2014), indicating that OCR inhibition and ROS increase in tagatose-incubated P. infestans can be ascribed to severe inhibition of mitochondrial processes.
The attempted cellular responses of P. infestans against tagatose included the upregulation of maai, which is homologous to gst genes commonly upregulated by oxidative stresses (Montibus et al., 2015). The maai gene is a marker of stress responses and its expression was also upregulated by the biocontrol agent Lysobacter capsici AZ78 in P. infestans (Tomada et al., 2017) and by copper sulfate in Saprolegnia parasitica (Hu et al., 2016). In addition, tagatose upregulated the expression of aif in P. infestans and it encoded a protein involved in ROS scavenging (Klein et al., 2002) and mitochondrial cristae regulation (Cheung et al., 2006) in mammalian cells. The expression of nma111 was upregulated and downregulated by tagatose in P. infestans and P. cinnamomi, respectively, and its expression was previously linked to ROS accumulation in Saccharomyces spp. (Wang et al., 2014), demonstrating a strong connection of physiological effects and transcriptional changes observed in tagatose-incubated P. infestans and P. cinnamomi. Therefore, further transcriptomic and metabolomic studies are required, in order to better understand the species-specific response of Phytophthora spp. to tagatose incubation and to estimate the risk of evolution of P. cinnamomi-like tolerance to tagatose in P. infestans.
Conclusion
Tagatose inhibited P. infestans growth in vitro and caused severe ultrastructural alterations, with the formation of circular and concentric mitochondrial cristae. In addition, the ATP content and OCR were decreased, while the ROS accumulation and expression of apoptosis- and oxidative stress-related genes were increased, suggesting the induction of severe deficiencies in the mitochondrial processes of tagatose-incubated P. infestans. On the other hand, P. cinnamomi growth and mitochondrial ultrastructure were only slightly affected by tagatose with no significant impacts on respiration processes and ROS accumulation, suggesting species-specific responses to this rare sugar. A partial or total selectivity of a fungicide is commonly regarded as a positive trait, because of the reduction of possible side effects on other microorganisms. For this reason, besides the good toxicological profile, tagatose seems to be a promising active substance for the further development of plant protection products to control P. infestans. The mode of action of tagatose against P. infestans is mainly based on the inhibition of mitochondrial processes, but further transcriptomic and metabolomic analyses are required to fully clarify the molecular determinants and pathways affected by this rare sugar in Phytophthora spp. In addition, its reversible effects suggest that efficacy trials of formulated products under field conditions are required, in order to better verify the stability and persistence of tagatose against target phytopathogens.
Data Availability Statement
All datasets generated for this study are included in the article/Supplementary Material.
Author Contributions
AC carried out the functional experiments and wrote the manuscript. AN carried out the functional experiments. LN and BB carried out ultrastructural analysis and revised the manuscript. IB carried out growth experiments and an ultrastructural analysis. EA revised the manuscript. IP revised the manuscript and analyzed the data. GP conceived the study, analyzed the data and revised the manuscript. MP conceived the study, supervised the experiments, analyzed the data and wrote the manuscript.
Funding
This project has received funding from the European Union’s Horizon 2020 Research and Innovation Program under the Marie Skłodowska-Curie grant agreement no. 722642 (project INTERFUTURE). Ultrastructural analysis were supported by funding from The University of Padua (PRID 2018 prot. BIRD180317 to LN) and the Electron Microscopy Service of the Department of Biology, University of Padua (Italy), for technical assistance.
Conflict of Interest
AC and AN were employed by Biological Products for Agriculture (Bi-PA).
The remaining authors declare that the research was conducted in the absence of any commercial or financial relationships that could be construed as a potential conflict of interest.
Acknowledgments
We thank Dr. Valerio Battaglia (Council for Agricultural Research and Agricultural Economics Analysis) for providing the Phytophthora infestans strain VB3.
Supplementary Material
The Supplementary Material for this article can be found online at: https://www.frontiersin.org/articles/10.3389/fmicb.2020.00128/full#supplementary-material
References
Ahmed, Z. (2001). Production of natural and rare pentoses using microorganisms and their enzymes. Electron. J. Biotechnol. 4, 1–9. doi: 10.2225/vol4-issue2-fulltext-7
Arselin, G., Vaillier, J., Salin, B., Schaeffer, J., Giraud, M. F., Dautant, A., et al. (2004). The modulation in subunits e and g amounts of yeast ATP synthase modifies mitochondrial cristae morphology. J. Biol. Chem. 279, 40392–40399. doi: 10.1074/jbc.M404316200
Bautista, D. A., Pegg, R. B., and Shand, P. J. (2000). Effect of L-glucose and D-tagatose on bacterial growth in media and a cooked cured ham product. J. Food Prot. 63, 71–77. doi: 10.4315/0362-028X-63.1.71
Bertelsen, H., Jensen, B. B., and Buemann, B. (1999). D-tagatose a novel low-calorie bulk sweetener with prebiotic properties. World Rev. Nutr. Diet. 85, 98–109. doi: 10.1159/000059685
Blum, M., Boehler, M., Randall, E., Young, V., Csukai, M., Kraus, S., et al. (2010). Mandipropamid targets the cellulose synthase-like PiCesA3 to inhibit cell wall biosynthesis in the oomycete plant pathogen, Phytophthora infestans. Mol. Plant Pathol. 11, 227–243. doi: 10.1111/j.1364-3703.2009.00604.x
Buemann, B., Toubro, S., Raben, A., Blundell, J., and Astrup, A. (2000). The acute effect of D-tagatose on food intake in human subjects. Br. J. Nutr. 84, 227–231. doi: 10.1017/s000711450000146x
Calmes, B., N’guyen, G., Dumur, J., Brisach, C. A., Campion, C., Iacomi, B., et al. (2015). Glucosinolate-derived isothiocyanates impact mitochondrial function in fungal cells and elicit an oxidative stress response necessary for growth recovery. Front. Plant Sci. 6:414. doi: 10.3389/fpls.2015.00414
Casagrande, K., Falginella, L., Castellarin, S. D., Di Gaspero, G., and Testolin, R. (2011). Defence responses in Rpv3-dependent resistance to grapevine downy mildew. Planta 234, 1097–1109. doi: 10.1007/s00425-011-1461-1465
Cheung, E. C. C., Joza, N., Steenaart, N. A. E., Mcclellan, K. A., Neuspiel, M., Mcnamara, S., et al. (2006). Dissociating the dual roles of apoptosis-inducing factor in maintaining mitochondrial structure and apoptosis. EMBO J. 25, 4061–4073. doi: 10.1038/sj.emboj.7601276
Coffey, M. D., and Joseph, M. C. (1985). Effects of phosphorous acid and fosetyl-Al on the life cycle of Phytophthora cinnamomi and Phytophthora citricola. Phytopathology 75, 1042–1046. doi: 10.1094/phyto-75-1042
Cristina, R. T., Dumitrescu, E., Pentea, M. C., Stancu, A. C., and Muselin, F. (2015). Albendazole sensitive vs. resistant nematodes – the mitochondrial ultra-structural changes. J. Fac. Vet. Med. Istanbul. Univ. 41, 43–49. doi: 10.16988/iuvfd.2015.34662
Dufour, E., Boulay, J., Rincheval, V., and Sainsard-Chanet, A. (2000). A causal link between respiration and senescence in Podospora anserina. Proc. Natl. Acad. Sci. U.S.A. 97, 4138–4143. doi: 10.1073/pnas.070501997
Eliskases-Lechner, F., and Prillinger, H. (1996). Inhibition of mould growth by oligomycin during quantitative analysis of yeasts. J. Dairy Res. 63, 483–448. doi: 10.1017/S0022029900032003
Fantke, P., Friedrich, R., and Jolliet, O. (2012). Health impact and damage cost assessment of pesticides in Europe. Environ. Int. 49, 9–17. doi: 10.1016/j.envint.2012.08.001
Florea, A., and Craciun, C. (2011). Abnormal mitochondrial cristae were experimentally generated by high doses of Apis mellifera venom in the rat adrenal cortex. Micron 42, 434–442. doi: 10.1016/j.micron.2010.12.006
Fry, W. E., Birch, P. R., Judelson, H. S., Grunwald, N. J., Danies, G., Everts, K. L., et al. (2015). Five reasons to consider Phytophthora infestans a reemerging pathogen. Phytopathology 105, 966–981. doi: 10.1094/PHYTO-01-15-0005-FI
Galloway, C. A., Lee, H., Nejjar, S., Jhun, B., Yu, T., Hsu, W., et al. (2012). Transgenic control of mitochondrial fission induces mitochondrial uncoupling and relieves diabetic oxidative stress. Diabetes 61, 2093–2104. doi: 10.2337/db11-1640/-/DC1
Gavin, P. D., Prescott, M., Luff, S. E., and Devenish, R. J. (2004). Cross-linking ATP synthase complexes in vivo eliminates mitochondrial cristae. J. Cell Sci. 117, 2333–2343. doi: 10.1242/jcs.01074
Godinho, J. L., Georgikopoulou, K., Calogeropoulou, T., De Souza, W., and Rodrigues, J. C. (2013). A novel alkyl phosphocholine-dinitroaniline hybrid molecule exhibits biological activity in vitro against Leishmania amazonensis. Exp. Parasitol. 135, 153–165. doi: 10.1016/j.exppara.2013.06.015
Granström, T. B., Takata, G., Tokuda, M., and Izumori, K. (2004). Izumoring. J. Biosci. Bioeng. 97, 89–94. doi: 10.1016/s1389-1723(04)70173-5
Groves, C. T., and Ristaino, J. B. (2000). Commercial fungicide formulations induce in vitro oospore formation and phenotypic change in mating type in Phytophthora infestans. Phytopathology 90, 1201–1208. doi: 10.1094/phyto.2000.90.11.1201
Hardham, A. R. (2005). Phytophthora cinnamomi. Mol. Plant Pathol. 6, 589–604. doi: 10.1111/j.1364-3703.2005.00308.x
Hasibul, K., Nakayama-Imaohji, H., Hashimoto, M., Yamasaki, H., Ogawa, T., Waki, J., et al. (2018). D-Tagatose inhibits the growth and biofilm formation of Streptococcus mutans. Mol. Med. Rep. 17, 843–851. doi: 10.3892/mmr.2017.8017
Hayer, K., Stratford, M., and Archer, D. B. (2013). Structural features of sugars that trigger or support conidial germination in the filamentous fungus Aspergillus niger. Appl. Environ. Microbiol. 79, 6924–6931. doi: 10.1128/AEM.02061-13
Hu, J., Hong, C., Stromberg, E. L., and Moorman, G. W. (2010). Mefenoxam sensitivity in Phytophthora cinnamomi isolates. Plant Dis. 94, 39–44. doi: 10.1094/pdis-94-1-0039
Hu, K., Ma, R. R., Cheng, J. M., Ye, X., Sun, Q., Yuan, H. L., et al. (2016). Analysis of Saprolegnia parasitica transcriptome following treatment with copper sulfate. PLoS One 11:e0147445. doi: 10.1371/journal.pone.0147445
Izumori, K. (2006). Izumoring: a strategy for bioproduction of all hexoses. J. Biotechnol. 124, 717–722. doi: 10.1016/j.jbiotec.2006.04.016
Izumori, K., Akimitsu, K., Tajima, S., Agarie, M., Yanagi, T., and Mochioka, R. (2008). Utilization of Rare Sugars in Plant or Microorganism. Patent No.: US 2008/0182752 A1. Takamatsu: Kagawa University.
Jayamuthunagai, J., Gautam, P., Srisowmeya, G., and Chakravarthy, M. (2017). Biocatalytic production of D-tagatose: a potential rare sugar with versatile applications. Crit. Rev. Food. Sci. Nutr. 57, 3430–3437. doi: 10.1080/10408398.2015.1126550
Judelson, H. S., Ah-Fong, A. M., Aux, G., Avrova, A. O., Bruce, C., Cakir, C., et al. (2008). Gene expression profiling during asexual development of the late blight pathogen Phytophthora infestans reveals a highly dynamic transcriptome. APS 21, 433–447. doi: 10.1094/MPMI
Judelson, H. S., and Blanco, F. A. (2005). The spores of Phytophthora: weapons of the plant destroyer. Nat. Rev. Microbiol. 3, 47–58. doi: 10.1038/nrmicro1064
Kabala, A. M., Lasserre, J. P., Ackerman, S. H., di, Rago JP, and Kucharczyk, R. (2014). Defining the impact on yeast ATP synthase of two pthogenic human mitochondrial DNA mutations, T9185C and T9191C. Biochimie 100, 200–206. doi: 10.1016/j.biochi.2013.11.024
Kamoun, S. (2000). “Phytophthora,” in Fungal Pathology, 1 Edn, ed. J. W. Kronstad, (Dordrecht: Springer), 237–265.
Klein, J. A., Longo-Guess, C. M., Rossmann, M. P., Seburn, K. L., Hurd, R. E., Frankel, W. N., et al. (2002). The harlequin mouse mutation down-regulates apoptosis-inducing factor. Nature 419, 367–374. doi: 10.1038/nature01034
Koh, J. H., Choi, S. H., Park, S. W., Choi, N. J., Kim, Y., and Kim, S. H. (2013). Synbiotic impact of tagatose on viability of Lactobacillus rhamnosus strain GG mediated by the phosphotransferase system (PTS). Food Microbiol. 36, 7–13. doi: 10.1016/j.fm.2013.03.003
Komon-Zelazowska, M., Bissett, J., Zafari, D., Hatvani, L., Manczinger, L., Woo, S., et al. (2007). Genetically closely related but phenotypically divergent Trichoderma species cause green mold disease in oyster mushroom farms worldwide. Appl. Environ. Microbiol. 73, 7415–7426. doi: 10.1128/AEM.01059-07
Kooragayala, K., Gotoh, N., Cogliati, T., Nellissery, J., Kaden, T. R., French, S., et al. (2015). Quantification of oxygen consumption in retina ex vivo demonstrates limited reserve capacity of photoreceptor mitochondria. Biochem. Mol. Biol. 56, 8428–8436. doi: 10.1167/iovs.15-17901
Kuhn, K., Obata, T., Feher, K., Bock, R., Fernie, A. R., and Meyer, E. H. (2015). Complete mitochondrial complex I deficiency induces an up-regulation of respiratory fluxes that is abolished by traces of functional complex I. Plant Physiol. 168, 1537–1549. doi: 10.1104/pp.15.00589
Lefebvre-Legendre, L., Salin, B., Schaeffer, J., Brethes, D., Dautant, A., Ackerman, S. H., et al. (2005). Failure to assemble the alpha 3 beta 3 subcomplex of the ATP synthase leads to accumulation of the alpha and beta subunits within inclusion bodies and the loss of mitochondrial cristae in Saccharomyces cerevisiae. J. Biol. Chem. 280, 18386–18392. doi: 10.1074/jbc.M410789200
Levin, G. V. (2002). Tagatose, the new GRAS sweetener and health product. J. Med. Food 5, 23–36. doi: 10.1089/109662002753723197
Levin, G. V., and Lu, Y. (2007). D-tagatose as an Anti-Biofilm Agent. Patent No.: US 7,189,351 B2. Virginia: SPHERIX Inc.
Li, Z., Gao, Y., Nakanishi, H., Gao, X., and Cai, L. (2013). Biosynthesis of rare hexoses using microorganisms and related enzymes. Beilstein J. Org. Chem. 9, 2434–2445. doi: 10.3762/bjoc.9.281
Lis, P., Jurkiewicz, P., Cal-Ba̧kowska, M., Ko, Y. H., Pedersen, P. L., Goffeau, A., et al. (2016). Screening the yeast genome for energetic metabolism pathways involved in a phenotypic response to the anti-cancer agent 3-bromopyruvate. Oncotarget 7, 10153–10173. doi: 10.18632/oncotarget.7174
Lobete, M. M., Noriega, E., Batalha, M. A., De Beurme, S., Van De Voorde, I., and Van Impe, J. F. (2017). Effect of tagatose on growth dynamics of Salmonella Typhimurium and Listeria monocytogenes in media with different levels of structural complexity and in UHT skimmed milk. Food Control 73, 31–42. doi: 10.1016/j.foodcont.2016.05.049
Ludovico, P., Rodrigues, F., Almeida, A., Silva, M. T., Barrientos, A., and Corte-Real, M. (2002). Cytochrome c release and mitochondria involvement in programmed cell death induced by acetic acid in Saccharomyces cerevisiae. Mol. Biol. Cell 13, 2598–2606. doi: 10.1091/mbc.e01-12-0161
Manfredi, G., Yang, L., Gajewski, C. D., and Mattiazzi, M. (2002). Measurements of ATP in mammalian cells. AP 26, 317–326. doi: 10.1016/S1046-2023(02)00037-3
Martinez-Reyes, I., and Cuezva, J. M. (2014). The H(+)-ATP synthase: a gate to ROS-mediated cell death or cell survival. Biochim. Biophys. Acta 1837, 1099–1112. doi: 10.1016/j.bbabio.2014.03.010
Martinussen, J., Solem, C., Holm, A. K., and Jensen, P. R. (2013). Engineering strategies aimed at control of acidification rate of lactic acid bacteria. Curr. Opin. Biotechnol. 24, 124–129. doi: 10.1016/j.copbio.2012.11.009
Montibus, M., Pinson-Gadais, L., Richard-Forget, F., Barreau, C., and Ponts, N. (2015). Coupling of transcriptional response to oxidative stress and secondary metabolism regulation in filamentous fungi. Crit. Rev. Microbiol. 41, 295–308. doi: 10.3109/1040841X.2013.829416
Moore, D., and Stewart, G. R. (1972). Effects of 2-deoxy-D-glucose, D-glucosamine, and L-sorbose on the growth of Coprinus lagopus hyphae. J. Gen. Microbiol. 71, 333–342. doi: 10.1099/00221287-71-2-333
Nass, M. M. K., and Ben-Shaul, Y. (1973). Effects of ethidium bromide on growth, chlorophyll synthesis, ultrastructure and mitochondrial DNA in green and bleached mutant Euglena gracilis. J. Cell. Sci. 13, 567–590.
Nayak, P. A., Nayak, U. A., and Khandelwal, V. (2014). The effect of xylitol on dental caries and oral flora. Clin. Cosmet. Investig. Dent. 6, 89–94. doi: 10.2147/CCIDE.S55761
Oh, D. K. (2007). Tagatose: properties, applications, and biotechnological processes. Appl. Microbiol. Biotechnol. 76, 1–8. doi: 10.1007/s00253-007-0981-1
Ohara, T., Ishida, Y., Kudou, R., Kakibuchi, K., Akimitsu, K., Izumori, K., et al. (2008). Plant Disease Control Agent Comprising D-Tagatose as Active Ingredient, and Plant Disease Control Method. Patent No.: EP 2 329 713 A1, 1-46. Takamatsu: Kagawa University.
Paumard, P., Vaillier, J., Coulary, B., Schaeffer, J., Soubannier, V., Mueller, D. M., et al. (2002). The ATP synthase is involved in generating mitochondrial cristae morphology. EMBO J. 21, 221–230. doi: 10.1093/emboj/21.3.221
Perazzolli, M., Nesler, A., Giovannini, O., Antonielli, L., Puopolo, G., and Pertot, I. (2020). Ecological impact of a rare sugar on grapevine phyllosphere microbial communities. Microbiol Res. 232, 126387. doi: 10.1016/j.micres.2019.126387
Perazzolli, M., Roatti, B., Bozza, E., and Pertot, I. (2011). Trichoderma harzianum T39 induces resistance against downy mildew by priming for defense without costs for grapevine. Biol. Control 58, 74–82. doi: 10.1016/j.biocontrol.2011.04.006
Pfaffl, M. W. (2001). A new mathematical model for relative quantification in real-time RT–PCR. Nucleic Acids Res. 29, 2002–2007. doi: 10.1093/nar/29.9.e45
Puopolo, G., Cimmino, A., Palmieri, M. C., Giovannini, O., Evidente, A., and Pertot, I. (2014). Lysobacter capsici AZ78 produces cyclo(L-Pro-L-Tyr), a 2,5-diketopiperazine with toxic activity against sporangia of Phytophthora infestans and Plasmopara viticola. J. Appl. Microbiol. 117, 1168–1180. doi: 10.1111/jam.12611
Raichand, R., Pareek, S., Singh, N. K., and Mayilraj, S. (2012). Exiguobacterium aquaticum sp. nov., a member of the genus Exiguobacterium. Int. J. Syst. Evol. Microbiol. 62, 2150–2155. doi: 10.1099/ijs.0.035790-0
Ruijter, J. M., Ramakers, C., Hoogaars, W. M., Karlen, Y., Bakker, O., Van Den Hoff, M. J., et al. (2009). Amplification efficiency: linking baseline and bias in the analysis of quantitative PCR data. Nucleic Acids Res. 37:e45. doi: 10.1093/nar/gkp045
Savoldi, M., Malavazi, I., Soriani, F. M., Capellaro, J. L., Kitamoto, K., Da Silva Ferreira, M. E., et al. (2008). Farnesol induces the transcriptional accumulation of the Aspergillus nidulans Apoptosis-Inducing Factor (AIF)-like mitochondrial oxidoreductase. Mol. Microbiol. 70, 44–59. doi: 10.1111/j.1365-2958.2008.06385.x
Shekhova, E., Kniemeyer, O., and Brakhage, A. A. (2017). Induction of mitochondrial reactive oxygen species production by itraconazole, terbinafine, and amphotericin B as a mode of action against Aspergillus fumigatus. Antimicrob. Agents Chemother. 61:e00978-17. doi: 10.1128/AAC.00978-17
Silver, N., Best, S., Jiang, J., and Thein, S. L. (2006). Selection of housekeeping genes for gene expression studies in human reticulocytes using real-time PCR. BMC Mol. Biol. 7:33. doi: 10.1186/1471-2199-7-33
Smith, M. R., Vayalil, P. K., Zhou, F., Benavides, G. A., Beggs, R. R., Golzarian, H., et al. (2016). Mitochondrial thiol modification by a targeted electrophile inhibits metabolism in breast adenocarcinoma cells by inhibiting enzyme activity and protein levels. Redox Biol. 8, 136–148. doi: 10.1016/j.redox.2016.01.002
Tomada, S., Sonego, P., Moretto, M., Engelen, K., Pertot, I., Perazzolli, M., et al. (2017). Dual RNA-Seq of Lysobacter capsici AZ78 - Phytophthora infestans interaction shows the implementation of attack strategies by the bacterium and unsuccessful oomycete defense responses. Environ. Microbiol. 19, 4113–4125. doi: 10.1111/1462-2920.13861
Trinci, A. P., and Collinge, A. (1973). Influence of L-sorbose on the growth and morphology of Neurospora crassa. J. Gen. Microbiol. 78, 179–192. doi: 10.1099/00221287-78-1-179
Van Der Heiden, E., Delmarcelle, M., Lebrun, S., Freichels, R., Brans, A., Vastenavond, C. M., et al. (2013). A pathway closely related to the D-tagatose pathway of Gram-negative enterobacteria identified in the Gram-positive bacterium Bacillus licheniformis. Appl. Environ. Microbiol. 79, 3511–3515. doi: 10.1128/AEM.03918-12
Vastenavond, C., Bertelsen, H., Hansen, S. R., Laursen, R., Saunders, J., and Eriknauer, K. (2011). “Tagatose (D-tagatose),” in Alternative Sweeteners, 4 Edn, ed. L. O’brien-Nabors, (New York, NY: CRC Press), 197–222. doi: 10.1201/b11242-15
Velours, J., Dautant, A., Salin, B., Sagot, I., and Brethes, D. (2009). Mitochondrial F1F0-ATP synthase and organellar internal architecture. Int. J. Biochem. Cell Biol. 41, 1783–1789. doi: 10.1016/j.biocel.2009.01.011
Wang, C. Q., Li, X., Wang, M. Q., Qian, J., Zheng, K., Bian, H. W., et al. (2014). Protective effects of ETC complex III and cytochrome c against hydrogen peroxide-induced apoptosis in yeast. Free Radic. Res. 48, 435–444. doi: 10.3109/10715762.2014.885116
Weimann, T., Vaillier, J., Salin, B., and Velours, J. (2008). The intermembrane space loop of subunit b (4) is a major determinant of the stability of yeast oligomeric ATP synthases. Biochemistry 47, 3556–3563. doi: 10.1021/bi702000g
Wong, S. S. W., Rani, M., Dodagatta-Marri, E., Ibrahim-Granet, O., Kishore, U., Bayry, J., et al. (2018). Fungal melanin stimulates surfactant protein D–mediated opsonization of and host immune response to Aspergillus fumigatus spores. J. Biol. Chem. 293, 4901–4912. doi: 10.1074/jbc.M117.815852
Woods, H. F., and Krebs, H. A. (1973). Xylitol metabolism in the isolated perfused rat liver. Biochem. J. 134, 437–443. doi: 10.1042/bj1340437
Wu, Q., and Shah, N. P. (2017). The potential of species-specific tagatose-6-phosphate (T6P) pathway in Lactobacillus casei group for galactose reduction in fermented dairy foods. Food Microbiol. 62, 178–187. doi: 10.1016/j.fm.2016.10.027
Xu, J., Zhao, X., Han, X., and Du, Y. (2007). Antifungal activity of oligochitosan against Phytophthora capsici and other plant pathogenic fungi in vitro. Pestic. Biochem. Physiol. 87, 220–228. doi: 10.1016/j.pestbp.2006.07.013
Yan, H. Z., and Liou, R. F. (2006). Selection of internal control genes for real-time quantitative RT-PCR assays in the oomycete plant pathogen Phytophthora parasitica. Fungal Genet. Biol. 43, 430–438. doi: 10.1016/j.fgb.2006.01.010
Yoshida, M., Muneyuki, E., and Hisabori, T. (2001). ATP synthase — a marvellous rotary engine of the cell. Nat. Rev. Mol. Cell Biol. 2, 669–677. doi: 10.1038/35089509
Yuan, S. K., Liu, X. L., Si, N. G., Dong, J., Gu, B. G., and Jiang, H. (2006). Sensitivity of Phytophthora infestans to flumorph: in vitro determination of baseline sensitivity and the risk of resistance. Plant Pathol. 55, 258–263. doi: 10.1111/j.1365-3059.2006.01338.x
Zhu, S., Luo, F., Zhu, B., and Wang, G. X. (2017). Toxicological effects of graphene oxide on Saccharomyces cerevisiae. Toxicol. Res. 6, 535–543. doi: 10.1039/c7tx00103g
Zick, M., Rabl, R., and Reichert, A. S. (2009). Cristae formation-linking ultrastructure and function of mitochondria. Biochim. Biophys. Acta Mol. Cell. Res. 1793, 5–19. doi: 10.1016/j.bbamcr.2008.06.013
Keywords: rare sugar, Phytophthora spp., biological control, mitochondrial alteration, antioomycete activity, oxidative stress
Citation: Chahed A, Nesler A, Navazio L, Baldan B, Busato I, Ait Barka E, Pertot I, Puopolo G and Perazzolli M (2020) The Rare Sugar Tagatose Differentially Inhibits the Growth of Phytophthora infestans and Phytophthora cinnamomi by Interfering With Mitochondrial Processes. Front. Microbiol. 11:128. doi: 10.3389/fmicb.2020.00128
Received: 25 October 2019; Accepted: 20 January 2020;
Published: 06 February 2020.
Edited by:
Brigitte Mauch-Mani, Université de Neuchâtel, SwitzerlandReviewed by:
Silvia Restrepo, University of Los Andes, ColombiaHazel McLellan, University of Dundee, United Kingdom
Copyright © 2020 Chahed, Nesler, Navazio, Baldan, Busato, Ait Barka, Pertot, Puopolo and Perazzolli. This is an open-access article distributed under the terms of the Creative Commons Attribution License (CC BY). The use, distribution or reproduction in other forums is permitted, provided the original author(s) and the copyright owner(s) are credited and that the original publication in this journal is cited, in accordance with accepted academic practice. No use, distribution or reproduction is permitted which does not comply with these terms.
*Correspondence: Michele Perazzolli, bWljaGVsZS5wZXJhenpvbGxpQHVuaXRuLml0