- 1Department of Crop and Soil Sciences, Washington State University, Pullman, WA, United States
- 2Wheat Health, Genetics, and Quality, USDA-ARS, Pullman, WA, United States
- 3Institute of Biological Chemistry, Washington State University, Pullman, WA, United States
Fusarium avenaceum F.a.1 is a novel strain of a fungal plant pathogen capable of preferentially decaying wild oat (Avena fatua) caryopses compared with those of wheat (Triticum aestivum). Understanding the molecular mechanisms governing weed seed-pathogen interactions is crucial to developing novel weed seed suppression technologies. Additionally, wild oat often competes with wheat in regions undergoing soil acidification, which leads to increases in soluble concentrations of many metals, including aluminum (Al). There is a dearth of information regarding the gene expression responses of Fusarium species to Al toxicity, or how metal toxicity might influence caryopsis colonization. To address this, a transcriptomic approach was used to investigate molecular responses of F.a.1 during wild oat caryopsis colonization in the presence and absence of chronic, sublethal concentrations of Al (400 μM). Caryopsis colonization was associated with induction of genes related to virulence, development, iron metabolism, oxidoreduction, stress, and detoxification, along with repression of genes associated with development, transport, cell-wall turnover, and virulence. Caryopsis colonization during Al exposure resulted in the induction of genes associated with virulence, detoxification, stress, iron metabolism, oxidoreduction, and cell wall turnover, along with repression of genes associated with cell wall metabolism, virulence, development, detoxification, stress, and transcriptional regulation. Aluminum exposure in the absence of caryopses was associated with induction of genes involved in siderophore biosynthesis, secretion, uptake, and utilization, along with several other iron metabolism-related and organic acid metabolism-related genes. The siderophore-related responses associated with Al toxicity occurred concurrently with differential regulation of genes indicating disruption of iron homeostasis. These findings suggest Al toxicity is attenuated by siderophore metabolism in F.a.1. In summary, both caryopsis colonization and Al toxicity uniquely influence transcriptomic responses of F.a.1.
Introduction
Agronomic weeds are a global issue that result in billions of dollars in annual economic losses (Pimentel et al., 2001). Weed seeds exist in high densities in soils, and may persist for many years due to long-term dormancy and decay resistance [as reviewed by Pollard (2018)]. Promoting microbial-driven seed decay is a potential ecological approach to long-term weed management by depleting the weed seedbank. In temperate regions of the world, including wheat-growing regions, wild oat (Avena fatua) is considered one of the ten worst weeds (Beckie et al., 2012). A major factor contributing to the persistence of A. fatua is that seeds can remain dormant in the soil for many years, thus generating a large soil seedbank that can readily develop herbicide resistance (Beckie et al., 2012).
Work by de Luna et al. (2011) resulted in hundreds of soil fungi isolates from dormant wild oat seeds, and it was found that Fusarium avenaceum isolate F.a.1 elicited the most rapid and pronounced decay of wild oat seeds. Successive studies in vitro showed F.a.1 is capable of preferentially decaying A. fatua compared with wheat caryopses (seeds without hulls), and that the fungus induces activity of several defense enzymes, including polyphenol oxidase, chitinase, and peroxidase, in both whole caryopses and caryopsis leachates (the soluble enzyme fraction) (Anderson et al., 2010; Fuerst et al., 2011, 2014, 2018). The latest work showed that incubation of wheat and wild oat seeds on a F.a.1 fungal mat resulted in a rapid increase in decay rating of wild oat, while wheat seeds germinated (Fuerst et al., 2018). F.a.1 exposure also resulted in increased polyphenol oxidase in wild oat and wheat caryopses, though the increase was 3.4 times that of the pathogen-free control in wild oat, and 1.8 times in wheat (Fuerst et al., 2018).
In addition to weed pressure, wheat production is often complicated by soil acidification, primarily due to the addition of ammoniacal fertilizers, which can have a strong influence on metal bioavailability, soil chemistry, and microbial communities (Schroder et al., 2011; Lewis et al., 2018). Soil acidification is a global issue currently affecting a large percentage of the world’s arable land (von Uexküll and Mutert, 1995), and the toxicity of soluble Al in acidic soils is thought to be a major factor in limiting plant growth (Foy, 1984). It has been hypothesized that many microbes can produce metal-chelating compounds, such as siderophores and organic acids, that may play a role in metal availability in the soil (Jones et al., 2003; Glick, 2010). Still, it is unclear how fungal plant pathogens respond to Al toxicity at the molecular level. In addition to revealing fundamental molecular mechanisms involved in Al toxicity, understanding how fungal plant pathogens might respond to this important aspect of soil acidification might assist in developing methods of weed seedbank control as soils acidify.
Work examining the F. avenaceum genome has shown it is enriched in transcription factors, redox-related proteins, and signal transduction proteins (Lysøe et al., 2014). Additionally, the F. avenaceum transcriptome was found to be enriched in gene ontology (GO) categories related to membrane activity, ATP/GTP binding, and calcium ion binding (Lysøe et al., 2014). One objective of the current work is to examine the transcriptomic changes associated with fungal colonization of A. fatua caryopses. Doing so should provide key insights into the molecular mechanisms governing A. fatua caryopsis colonization. Another objective is to examine the influence of Al toxicity on fungal gene expression in the absence and presence of A. fatua caryopses. Addressing this last objective would provide information regarding how F. avenaceum responds to aluminum toxicity while also examining how Al influences the fungal transcriptome during colonization of caryopses. The ultimate objective of the work is to elucidate fungal genes which may be of use in future endeavors to develop weed seed suppression technologies through promotion of weed seedbank destruction.
Materials and Methods
Fungal Culturing
Filter disk segments containing mycelium from F. avenaceum F.a.1 were transferred to potato dextrose agar (PDA: 24 g potato dextrose L–1 + 15 g agar L–1) plates (25 mL). After 13 days of growth, 6 mm plugs were taken with a sterile core sampler and placed to 25 mL PDA plates that had been amended 72 h earlier, with 1 mL of double-deionized (DDI) sterile H2O (PDA-H2O), or 1 mL of 10 mM AlCl3 (PDA-Al). All plates were incubated in dark conditions at 22°C.
Plant-Fungal Interaction and Tissue Sampling
Fungal culture diameter was measured at 2, 4, 7, and 11 days post inoculation (DPI); note that fungal colony diameter data are only shown for samples used in the RNA-Seq studies, so there are 12 replicates per treatment (water and Al) (Figure 1). After 8 days of growth on PDA-H2O or PDA-Al, 30 dry wild oat (Avena fatua) caryopses were placed along the growing edge of the fungal mycelial mat on nine plates of each treatment. Caryopses were also placed on 25 mL water agar plates (15 g agar L–1) with one mL of DDI H2O (Agar-H2O), or one mL 10 mM AlCl3 (Agar-Al), without the fungus; these plates were previously prepared and treated along with the PDA plates described above. After addition of caryopses, the plates were incubated in dark conditions at 15°C for 3 days (72 h). The decreased temperature was used to discourage germination of the caryopses.
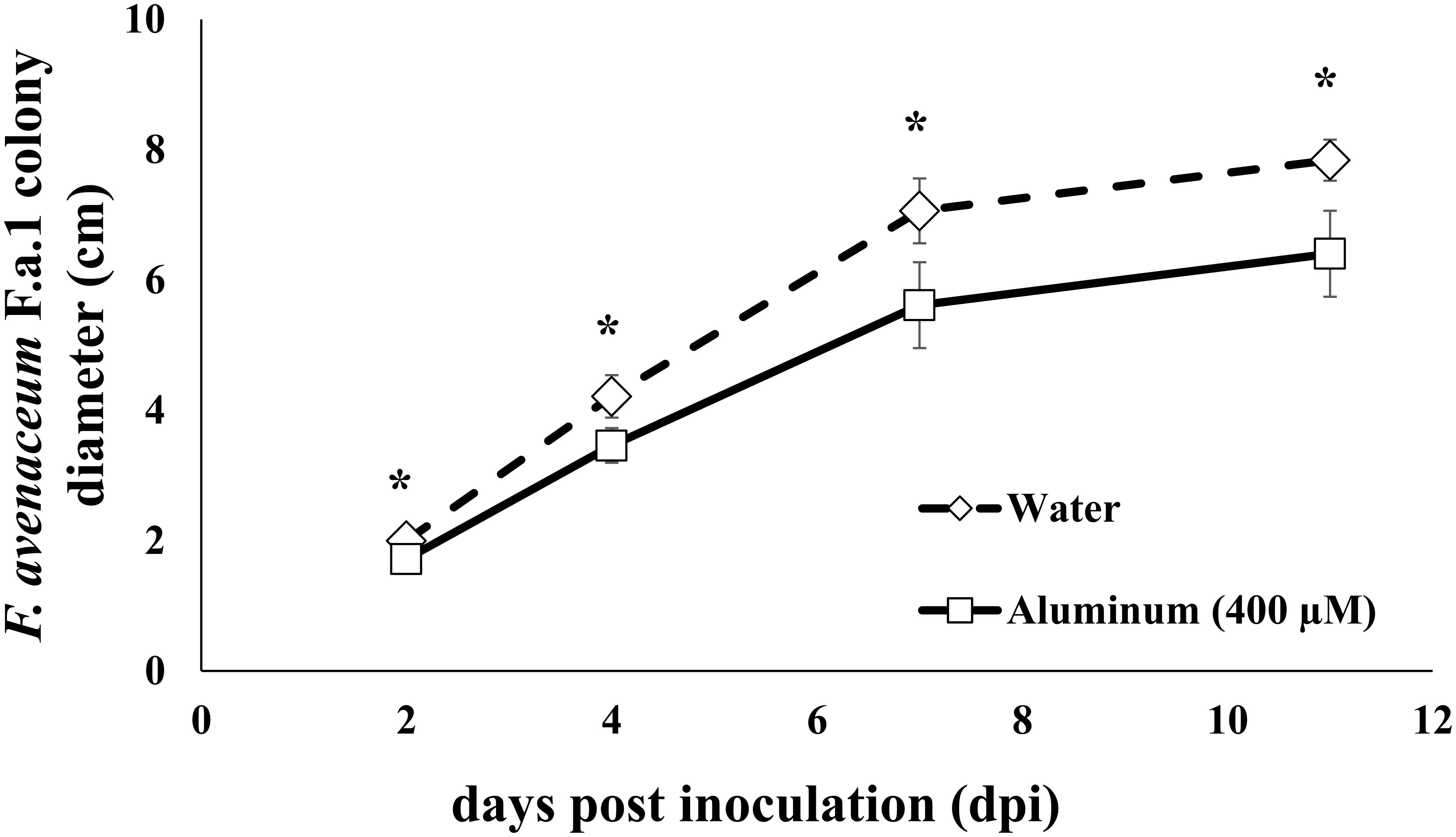
Figure 1. Fungal colony diameter of Fusarium avenaceum F.a.1 in cm. Days post inoculation (dpi) is days after transferring a 6 mm plug of colonized potato dextrose agar (PDA; 25 mL) to the center of fresh PDA (25 mL) amended with either 1 mL of water (diamond; dotted line) or 1 mL of 10 mM AlCl3 (squares; solid lines; 400 μM is final aluminum concentration). Data represent samples used in transcriptomic studies. Because caryopses were not added until day eight, colony diameter analyses have 12 replicates for each treatment (water or Al). Asterisks indicate statistical significance at alpha = 0.05.
After 72 h, caryopses were gently removed from the fungal mat using sterile forceps, large fungal fragments were carefully removed, and then the caryopses were placed in sterile 2 mL tubes. Six replicates per treatment of the caryopses samples were set aside for a separate study, and three replicates of each treatment were used for polyphenol oxidase (PPO) activity assays. The fungal tissue was gently scraped from the PDA plates using a sterile spatula, and placed in sterile 2 mL tubes. All tubes were massed before and after loading samples and were immediately placed in liquid N2 after massing. Samples were then maintained at −80°C before RNA extraction. Throughout the manuscript the treatments are indicated as follows, FOW = fungus only + water, FW = fungus + caryopsis + water, FOA = fungus only + Al, and FA = fungus + caryopsis + Al.
PPO Activity in Caryopses
Whole caryopsis PPO activity was assayed spectrophotometrically as previously described (Fuerst et al., 2018). Using forceps, caryopses were gently removed from Agar-H2O and Agar-Al plates, and from the fungal mycelial bed from the fungus grown on PDA-H2O and PDA-Al (three replicates each). Three plates per treatment were dedicated to the PPO assays, and three replicates composed of five caryopses each were gathered from each plate and transferred to a tared 2-mL microcentrifuge tube, and samples were re-weighed. Samples were incubated in 1.25 mL substrate solution consisting of 10 mM L-DOPA (L-3,4-dihydroxyphenylalanine) at pH 6.5. Samples were incubated at room temperature on an end-over-end shaker for 25 min and the reaction was terminated with 1 mM tropolone (2-hydroxy-2,4,6-cycloheptatrien-1-one). Samples were centrifuged to remove particulate contaminants and 300 μL of supernatant was transferred to a microtiter plate in duplicate. Absorbance at 475 nm was determined with a spectrophotometer (BioTek Epoch; BioTek Instruments, Inc., Winooski, VT, United States). Results are reported as change in optical density per gram fresh weight of caryopses (gfwt–1).
RNA Extraction, Sequencing, and Analysis
Six replicates from each treatment were used for fungal transcriptome studies. Samples ranging from 56 to 271 mg were collected and put into 2 mL safe-lock tubes (Eppendorf) at −80°C. Samples were prepared by precooling TissueLyser adapter sets in liquid nitrogen and adding two sterilized 2.88 mm stainless steel beads in each sample tube, then sample homogenates were generated using a TissueLyser II (Qiagen) with a frequency setting of 30 for 40 s. About 50 mg sample powder for each sample was collected in 0.5 mL Trizol (Invitrogen, Carlsbad, CA, United States). For RNA extraction, 0.3 mL chloroform was added to 0.5 mL Trizol homogenates, followed by vigorous sample shaking for 2 min. Samples were transferred to 1.5 mL tubes, and then allowed to sit for 3 min at room temperature, followed by centrifugation at 12,000 × g for 15 min at 4°C to assist with separation of organic and aqueous phases. The aqueous phase (∼250 μL) was then transferred to a new sterile RNase-free tube and an equal volume of 100 % EtOH was added, with mixing as needed. Samples were further purified using the RNeasy Mini Kit (Qiagen, Valencia, CA, United States) according to the manufacturer’s protocol. The quality and quantity of each RNA sample was assessed using a NanoDrop 2000 Spectrophotometer (Thermo Scientific, Wilmington, DE, United States), and an Agilent 2100 Bioanalyzer (Agilent, Santa Clara, CA, United States).
Libraries were prepared using the TruSeq RNA Library Prep Kit (Illumina, San Diego, CA, United States). Next-generation sequencing was performed by Novogene Inc. using an Illumina NovaSeq 6000 (paired-end, 2 × 150 bp, 20 million reads per sample). Reads were filtered by discarding those with adaptor contamination, uncertain nucleotides >10%, and/or base quality <20 for more than 50% of the read. The Fusarium avenaceum genome was used as a reference and mapping was performed using TopHat (v2.0.12) with mismatch = 2 (Trapnell et al., 2009). HTSeq (v0.6.1) was used for quantification using the “union” mode (Anders et al., 2015). DESeq (v1.120.1) was used for assessing differentially expressed genes (DEGs) with significance assessed using an adjusted p-value of 0.05 (Anders and Huber, 2010). Reported differentially expressed genes were further trimmed to include only those with |LOG2(Fold Change)| ≥2 (which is a 4-fold change), and only genes with at least 14 average reads in one of the treatments being compared were discussed. Genes were annotated using the Swiss-Prot (UniProt Consortium, 2018) and GenBank (Coordinators, 2016) databases (Supplementary Tables S1–S3). Kyoto Encyclopedia of Genes and Genomes (KEGG) (Kanehisa and Goto, 2000) enrichment analysis was performed using Fusarium graminearum (Walkowiak et al., 2016) as a reference and KOBAS (v3.0) with significance evaluated at an adjusted p-value of 0.05. GO enrichment was assessed using HMMER (v3.1b1) and significance assessed using an adjusted p-value of 0.05 (Eddy, 2011). All adjusted p-values were obtained using the FDR method. Information regarding RNA quality and RNA-Seq quality control can be found in supplemental information (Supplementary Tables S4–S6). Counts of genes that were uniquely expressed and exhibited statistically significant expression (DEGs) were summarized in Supplementary Table S7; these data were further filtered to only include gene ontology terms with ≥5 DEGs. Raw sequencing data are available via the Sequence Read Archive (SRA; SRA accession: PRJNA595343).
Results
Aluminum Influences Colony Formation but Not PPO Activity in Caryopses
Chronic sublethal exposure of F.a.1 to 400 μM Al resulted in inhibited fungal colony formation that persisted across the study, starting at 2 days post inoculation (dpi) until tissue harvesting at 11 dpi (Figure 1). Fungal colonies on control plates containing water ultimately reached an average diameter of 7.8 cm, while those exposed to Al reached an average diameter of 6.4 cm. Activity of polyphenol oxidase (PPO) was increased in the caryopses exposed to F.a.1, however, PPO activity was not influenced by addition of Al (Figure 2).
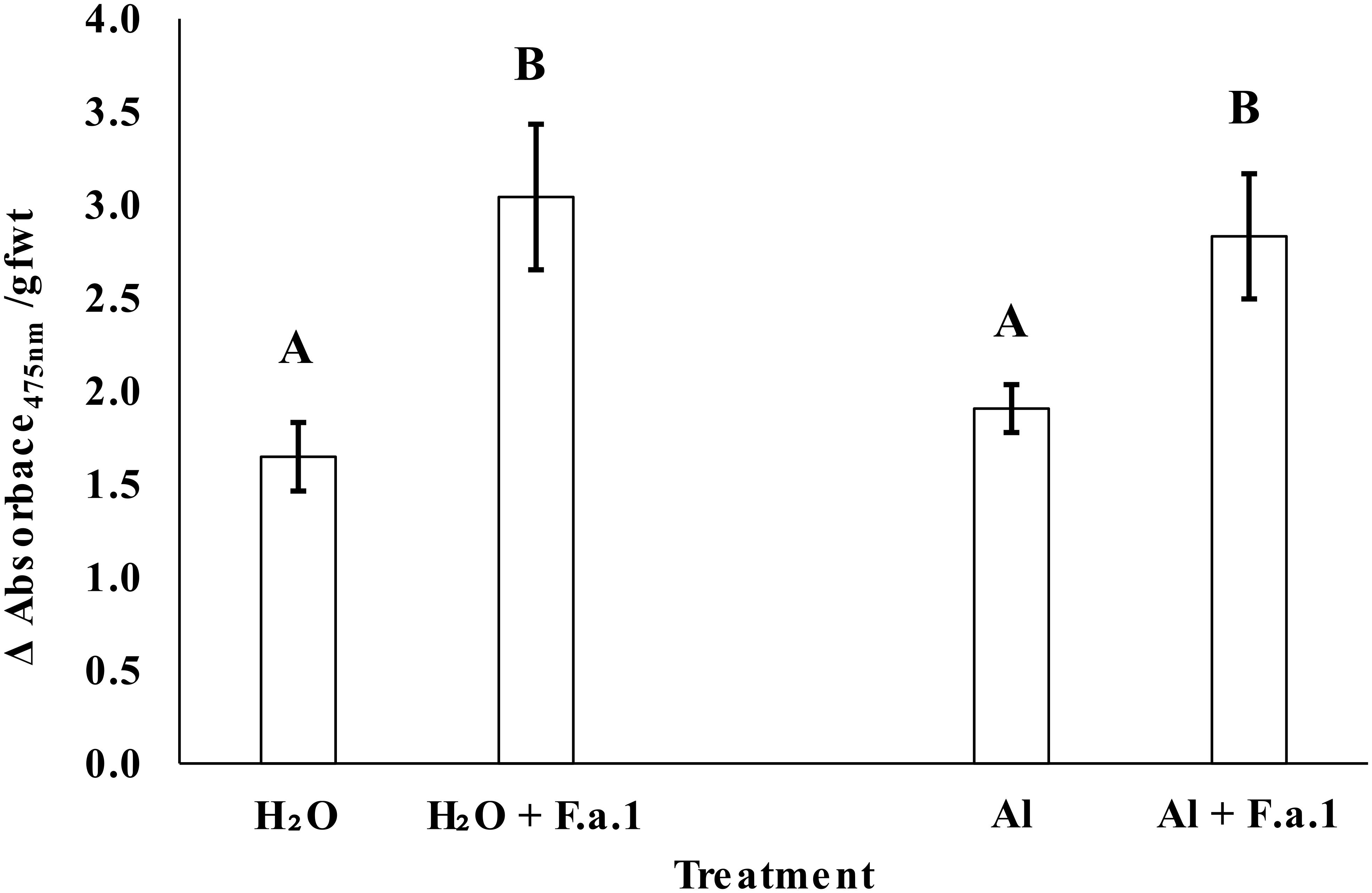
Figure 2. Polyphenol oxidase (PPO) activity in whole caryopses presented as average change (Δ) in absorbance at 475 nm divided by caryopsis grams fresh weight (gfwt). Bars are standard deviation and letters represent significance evaluated at alpha = 0.05. Caryopses in the absence of Fusarium avenaceum F.a.1 (F.a.1) were sampled from 25 mL water agar plates amended with 1 mL of water (H2O) or 10 mM AlCl3 (Al). Caryopses in the presence of F.a.1 were sampled from 25 mL PDA plates with the same amendments.
Fungal Genes Involved in Wild Oat Caryopsis Colonization
In the absence of Al, 8,249 genes were co-expressed in the fungus with (FW) or without the caryopses (FOW). In the presence or absence of the caryopses, 154 and 203 genes were uniquely expressed in the fungus (Figure 3A). Of uniquely expressed genes in the FW treatment, differentially expressed genes were associated with unique gene ontology terms; these terms included oxidation-reduction process, oxidoreductase activity, ion binding, small molecule binding, organic cyclic compound metabolic process, and more (Supplementary Table S7). Induction and repression of genes related to several biological functions were associated with A. fatua caryopsis colonization in the FW treatment, including genes involved in virulence/pathogenicity, stress detoxification responses, organic acid metabolism, metal interactions, basic metabolism, and amino acid/peptide/protein metabolism (Tables 1, 2, and Supplementary Table S1). Proteins of many of the induced and repressed genes were localized in various membrane compartments (Tables 1, 2), with several induced uncharacterized transporters being potentially localized to the vacuole membrane (Table 1).
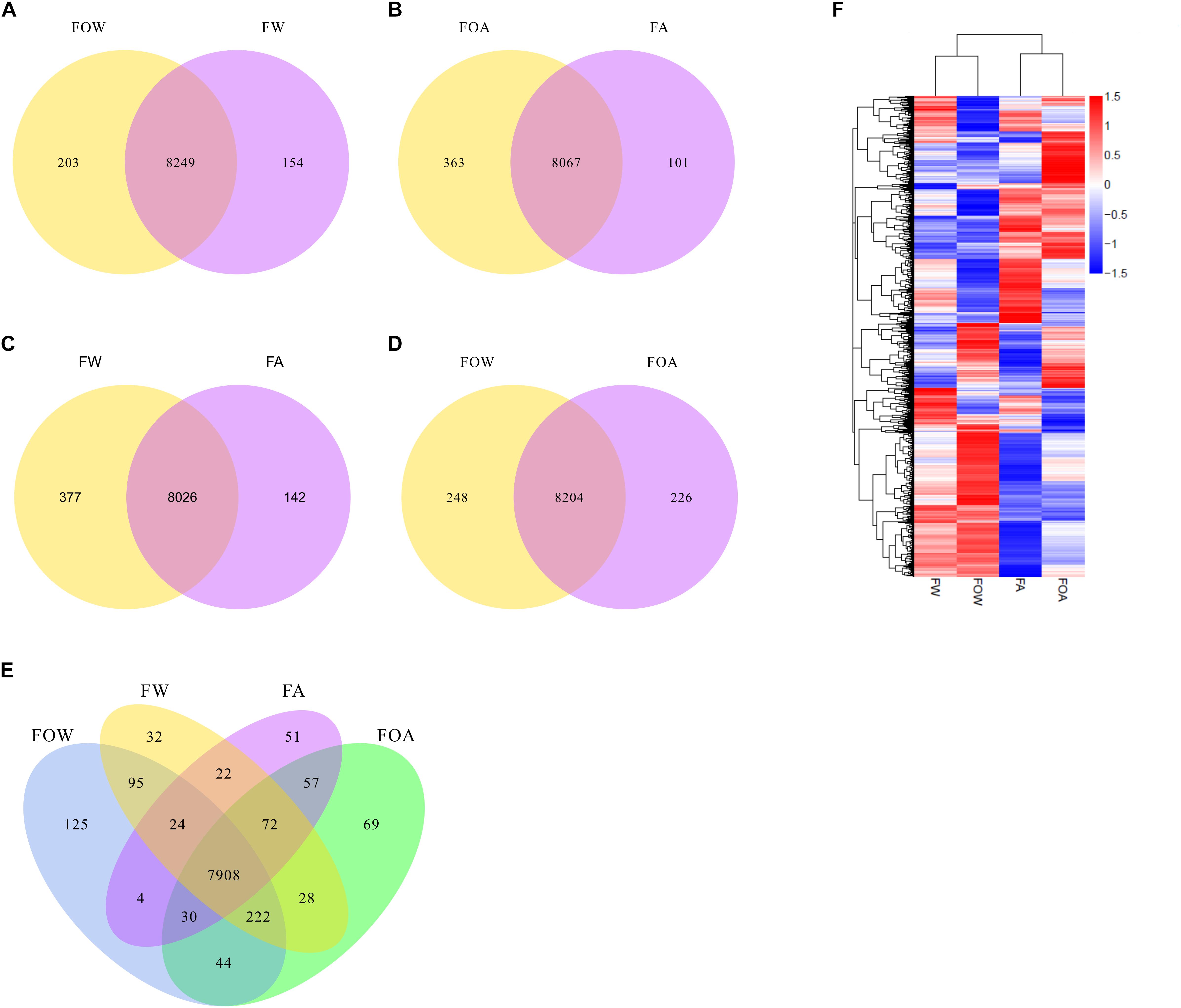
Figure 3. Influence of aluminum exposure and caryopsis colonization on global gene expression. FOW, fungus only exposed to water; FW, fungus exposed to water plus caryopses; FOA, fungus only exposed to aluminum; FA, fungus exposed to aluminum plus caryopses. Co-expression Venn diagrams are shown comparing (A) FOW vs. FW, (B) FOA vs. FA, (C) FW vs. FA (D) FOW vs. FOA), and (E) all treatments. (F) shows a clusters analysis of differentially expressed genes.
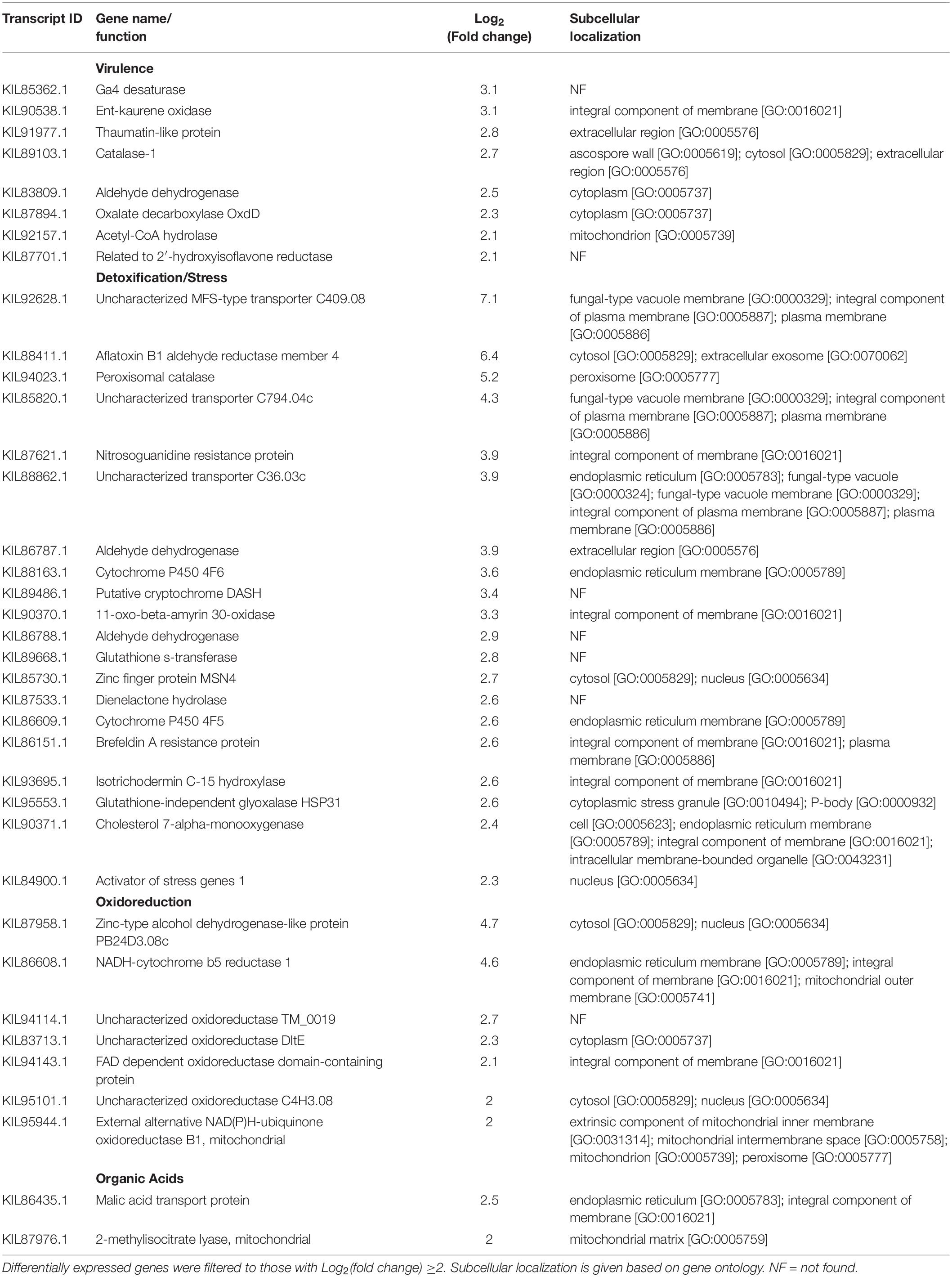
Table 1. Caryopsis-induced differentially expressed genes in F. avenaceum F.a.1. in the absence of aluminum (FW vs. FOW).
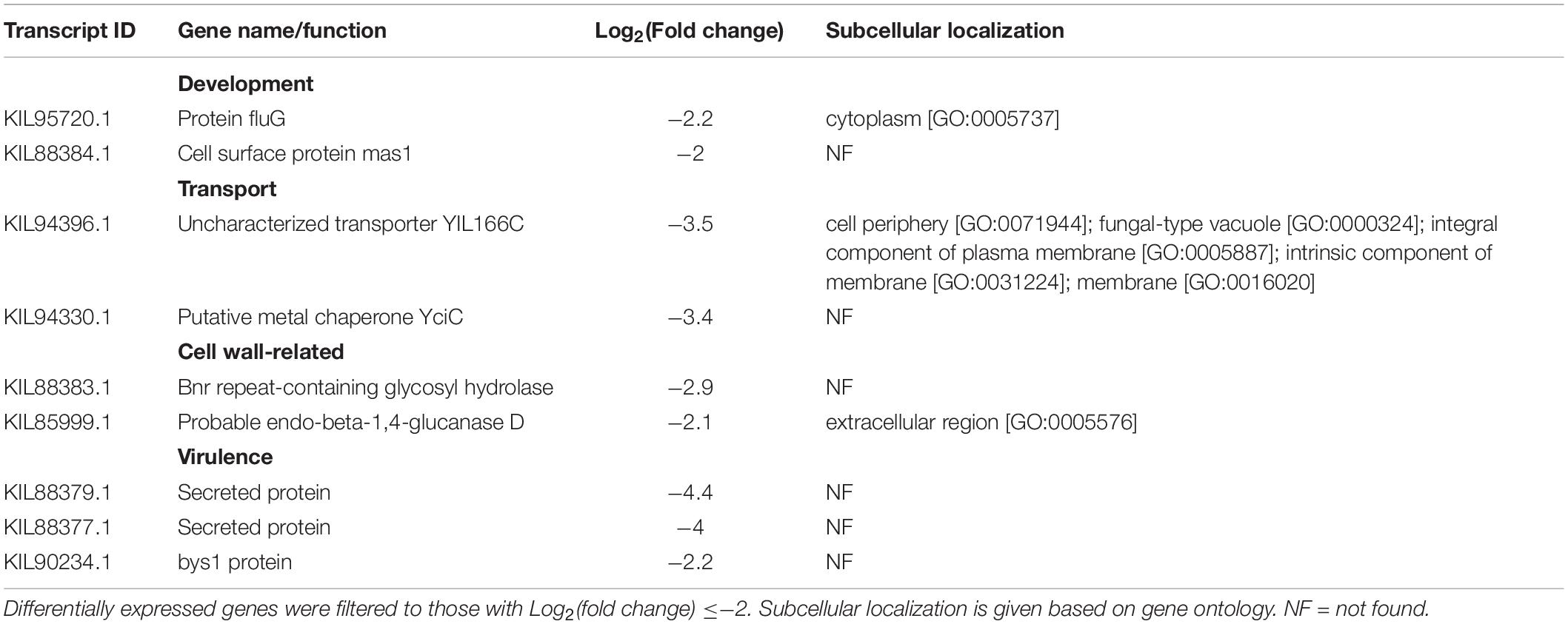
Table 2. Caryopsis-repressed differentially expressed genes in F. avenaceum F.a.1. in the absence of aluminum (FW vs. FOW).
Fungal Genes Involved in Wild Oat Caryopsis Colonization During Aluminum Exposure
Compared with the control, Al exposure led to slightly fewer genes (8,067) being co-expressed in the fungus with (FA) or without the caryopses (FOA). In the FA and FOA treatments, 101 and 363 genes were uniquely expressed in the fungus, respectively (Figure 3B). Of uniquely expressed genes in the FA treatment, differentially expressed genes were associated with transporter activity (Supplementary Table S7). In the FOW treatment, 66 gene ontology terms were unique compared with the FW treatment, including primary metabolic process, macromolecule metabolic process, nitrogen compound metabolic process, cellular aromatic compound metabolic process, cellular nitrogen compound metabolic process, and more (Supplementary Table S7).
Genes related to several biological functions were differentially expressed during A. fatua caryopsis colonization and Al exposure (FA), including genes involved in iron metabolism, stress/defense responses, basic metabolism, metal-related responses, and amino acid/peptide/protein metabolism, and phosphate-related metabolism (Tables 3, 4 and Supplementary Table S1). While many of the induced and repressed genes were found to encode proteins potentially localized in cellular membranes, several of the induced cell wall-related genes were found to be localized in the extracellular region (Tables 3, 4).
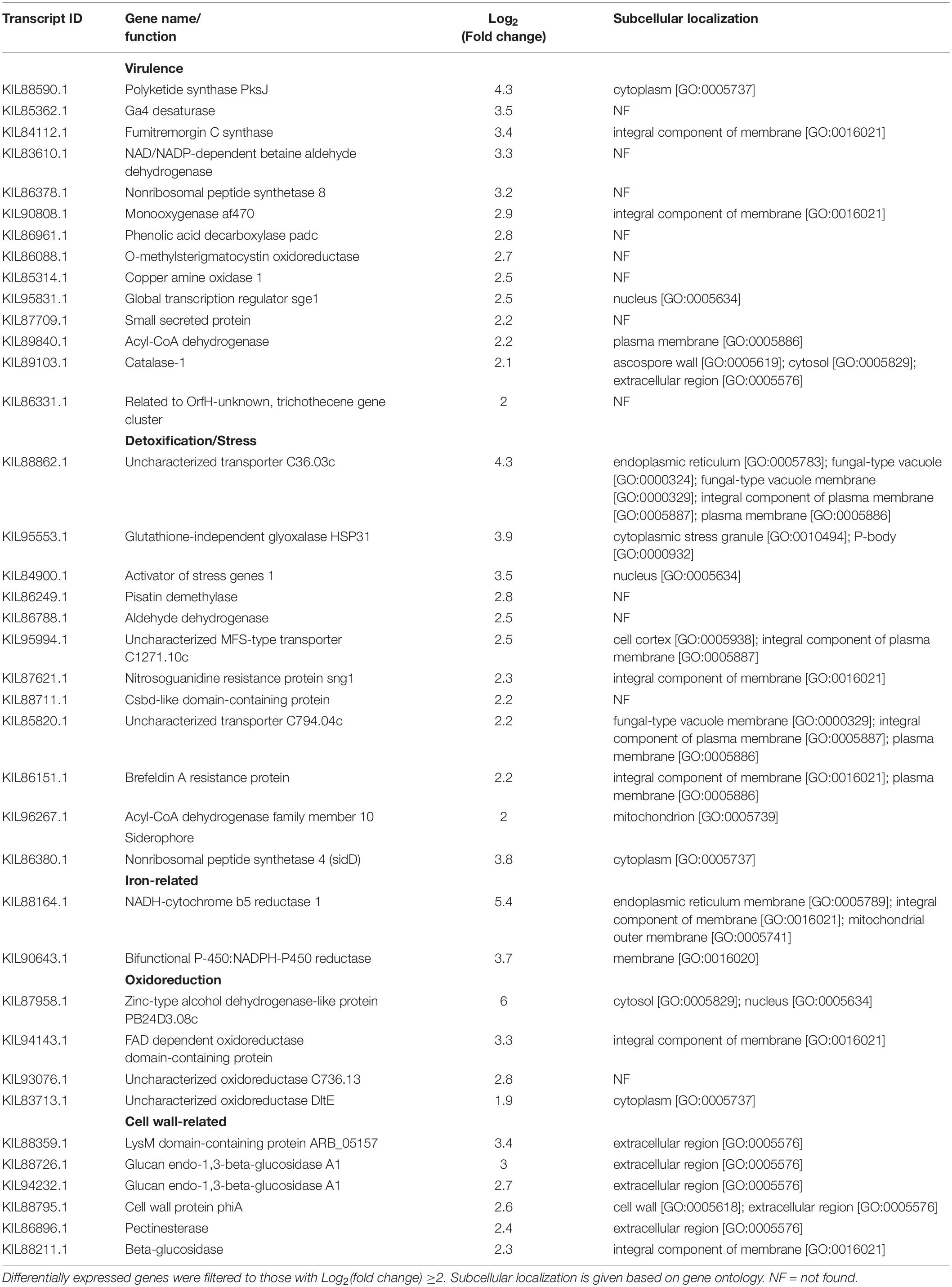
Table 3. Caryopsis-induced differentially expressed genes in F. avenaceum F.a.1, during aluminum exposure (FA vs. FOA).
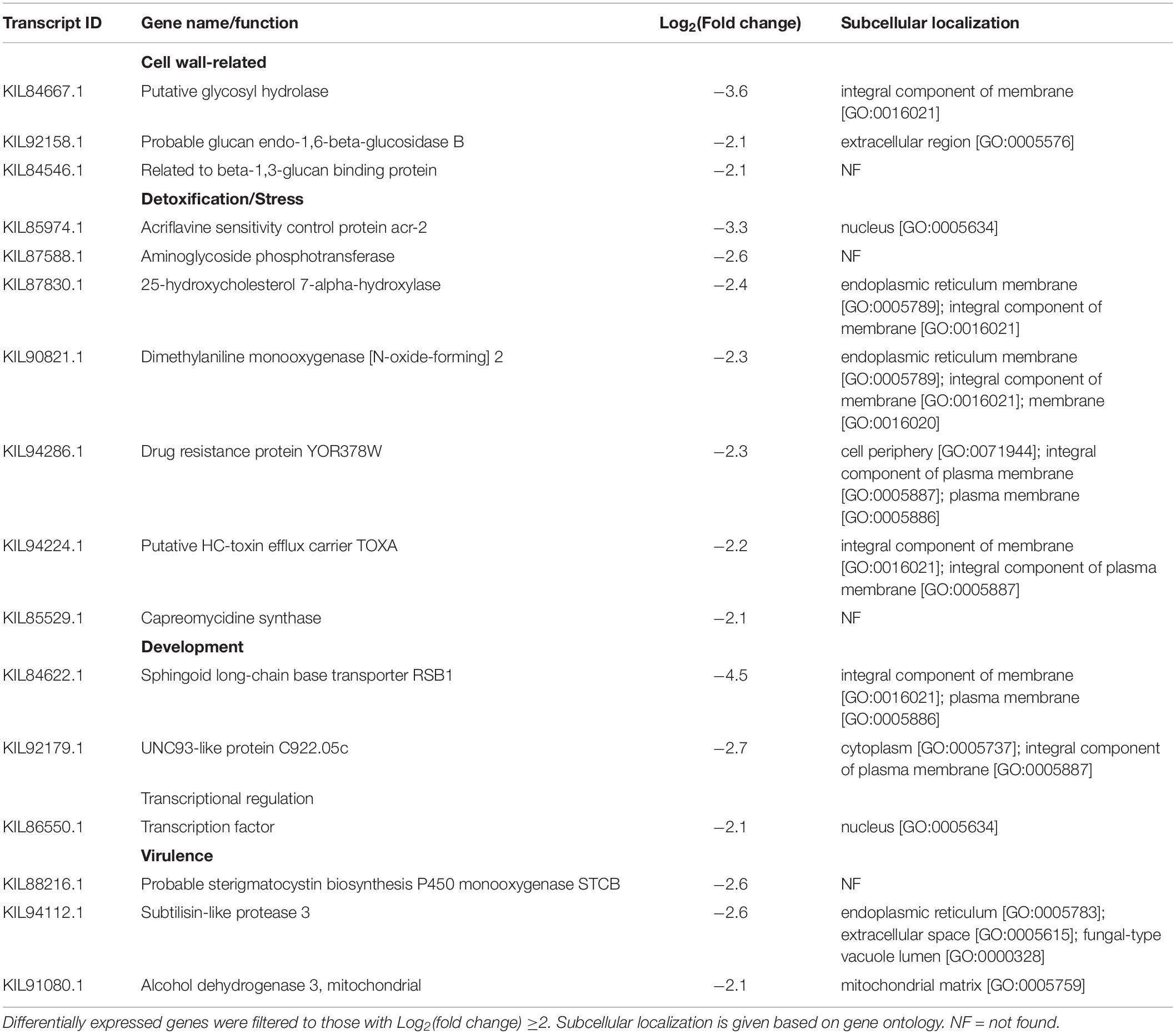
Table 4. Caryopsis-repressed differentially expressed genes in F. avenaceum F.a.1, during aluminum exposure (FA vs. FOA).
The Influence of Al on Caryopsis Colonization
Caryopsis colonization resulted in co-expression of 8,026 genes in the fungus exposed to water (FW) or Al (FA). Additionally, 142 and 377 genes were uniquely expressed in the fungus in the presence or absence of Al, respectively (Figure 3C). Compared with the FW treatment, uniquely expressed DEGs were associated with three unique gene ontology terms in the FA treatment, including cofactor binding, coenzyme binding, and ion transport (Supplementary Table S7).
Induction of genes related to several biological functions were associated with Al exposure during A. fatua caryopsis colonization (FA) when compared to the FW treatment, including genes involved in siderophore metabolism, iron metabolism, stress/defense responses, drug resistance, basic metabolism, metal-related responses, and phosphate-related metabolism (Tables 5, 6 and Supplementary Table S1). Proteins of both Al-repressed and Al-induced genes were found to be potentially localized to membranes and the cytosol/cytoplasm (Tables 5, 6). Two genes associated with transport and detoxification (uncharacterized membrane protein YJR124C and leptomycin B resistance protein pmd1, respectively), were found to be induced by Al, with the associated proteins potentially being localized in the vacuole membrane (Table 5). Laccase-2 was found to be repressed by Al in the FA treatment compared to the FW treatment, and was found to be potentially partitioned to the extracellular region (Table 6).
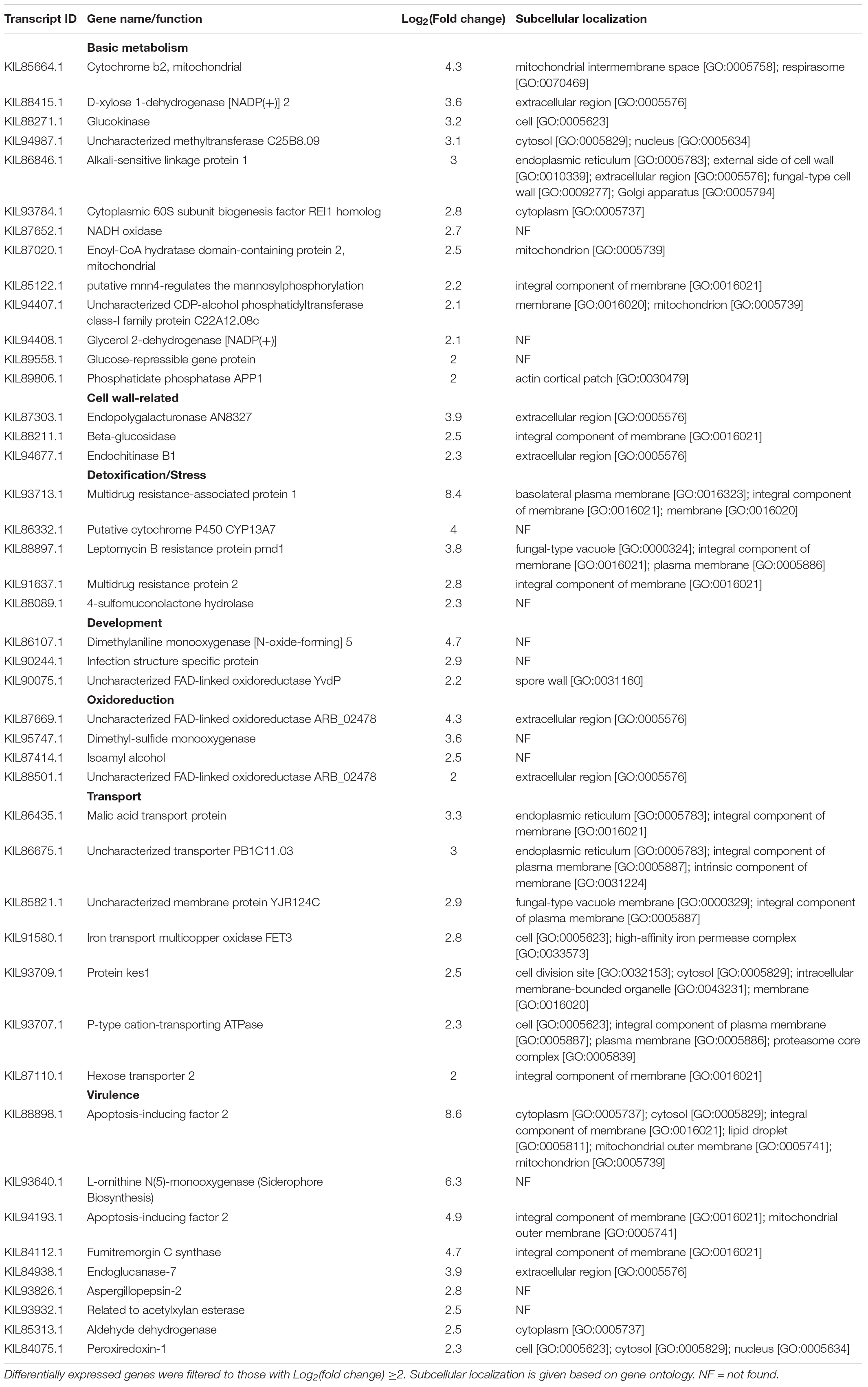
Table 5. Aluminum-induced differentially expressed genes in F. avenaceum F.a.1 during caryopsis colonization (FA vs. FW).
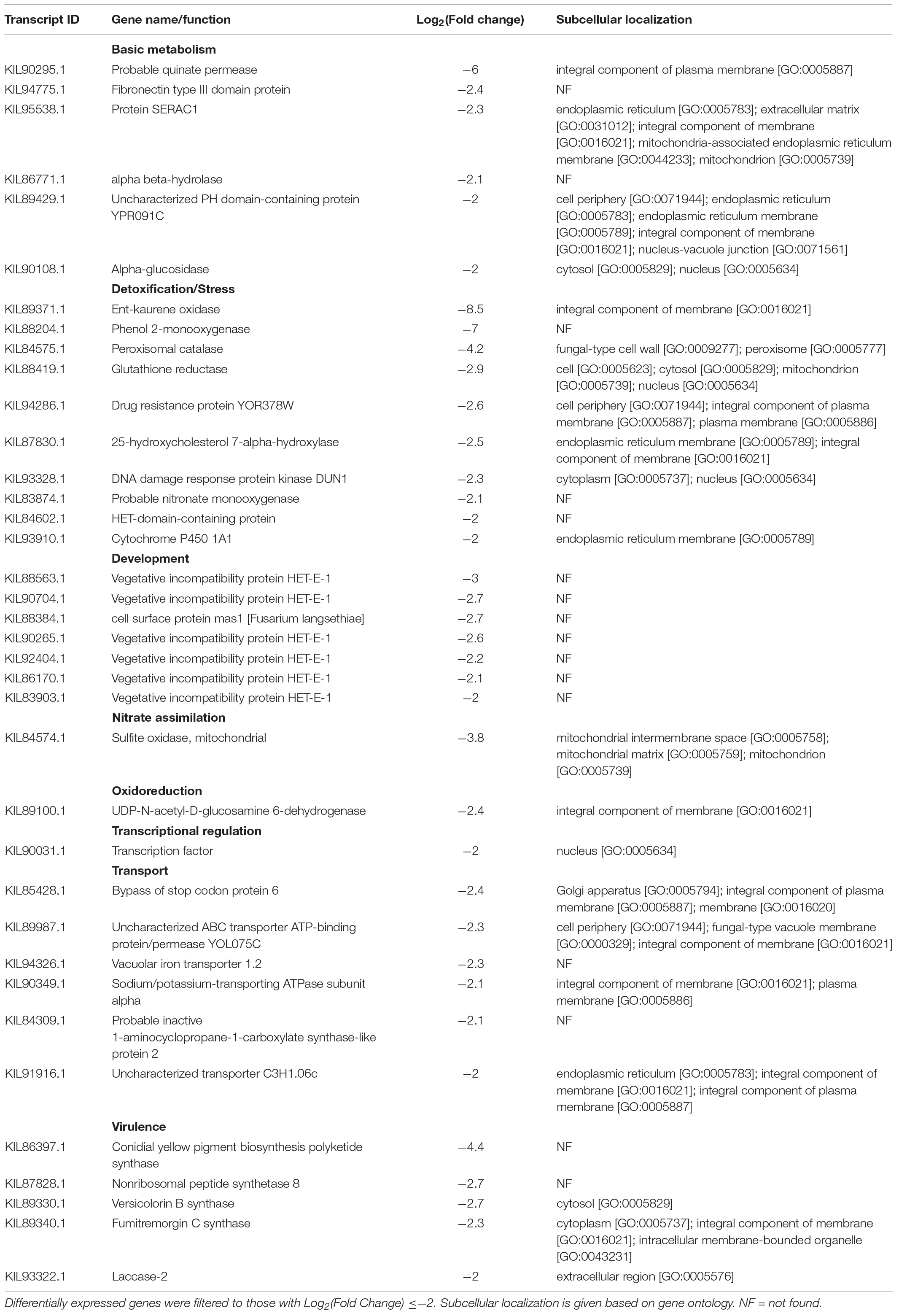
Table 6. Aluminum-repressed differentially expressed genes in F. avenaceum F.a.1 during caryopsis colonization (FA vs. FW).
Fungal Transcriptomic Responses to Aluminum Exposure
Exposure of F.a.1 to Al (FOA) or water (FOW) resulted in the co-expression of 8,204 genes in the absence of caryopses (Figure 3D). In the FOW treatment, 248 genes were uniquely expressed, while 226 genes were uniquely expressed during Al exposure (FOA). Genes uniquely expressed in the FOA treatment compared with FOW, were associated with 33 unique GO terms, including several protein-related GO terms, ion transport, organic substance transport, organonitrogen compound metabolic process, organic acid transport, and more (Supplementary Table S7). Compared with the FOA treatment, the FOW treatment was associated with 22 unique GO terms, including several related to nucleic acid metabolism, carbohydrate derivative binding, lipid biosynthetic processes, ATP binding, iron ion binding, oxidoreductase activity (acting on CH-OH group donors, and more) (Supplementary Table S7).
Additionally, Al exposure led to induction of several genes associated with siderophore transport, iron metabolism, organic acid metabolism, and metals, as well as, genes associated with stress/defense, and drug resistance (Tables 7–9 and Supplementary Table S1). Proteins of most of the induced siderophore-related genes were found to be potentially localized to various membranes throughout the cell (Tables 7, 8). Siderophore iron transporter 3 was found to potentially be localized to cell, cytoplasm, integral component of membrane, integral component of plasma membrane, and/or plasma membrane (Table 7). Siderophore iron transporter 1 was found to be potentially localized to any of several compartments, including cell, cytoplasmic vesicle, endosome, endosome membrane, fungal-type vacuole, integral component of plasma membrane, plasma membrane, and/or vacuolar membrane (Table 7). Several Al-induced virulence and detoxification genes were found to potentially encode for proteins that might partition to the extracellular space, including acetyl-coenzyme A synthetase, laccase ARB_05828, subtilisin-like protease 3, aflatoxin B1 aldehyde reductase member 4, and aldehyde dehydrogenase (Table 8).
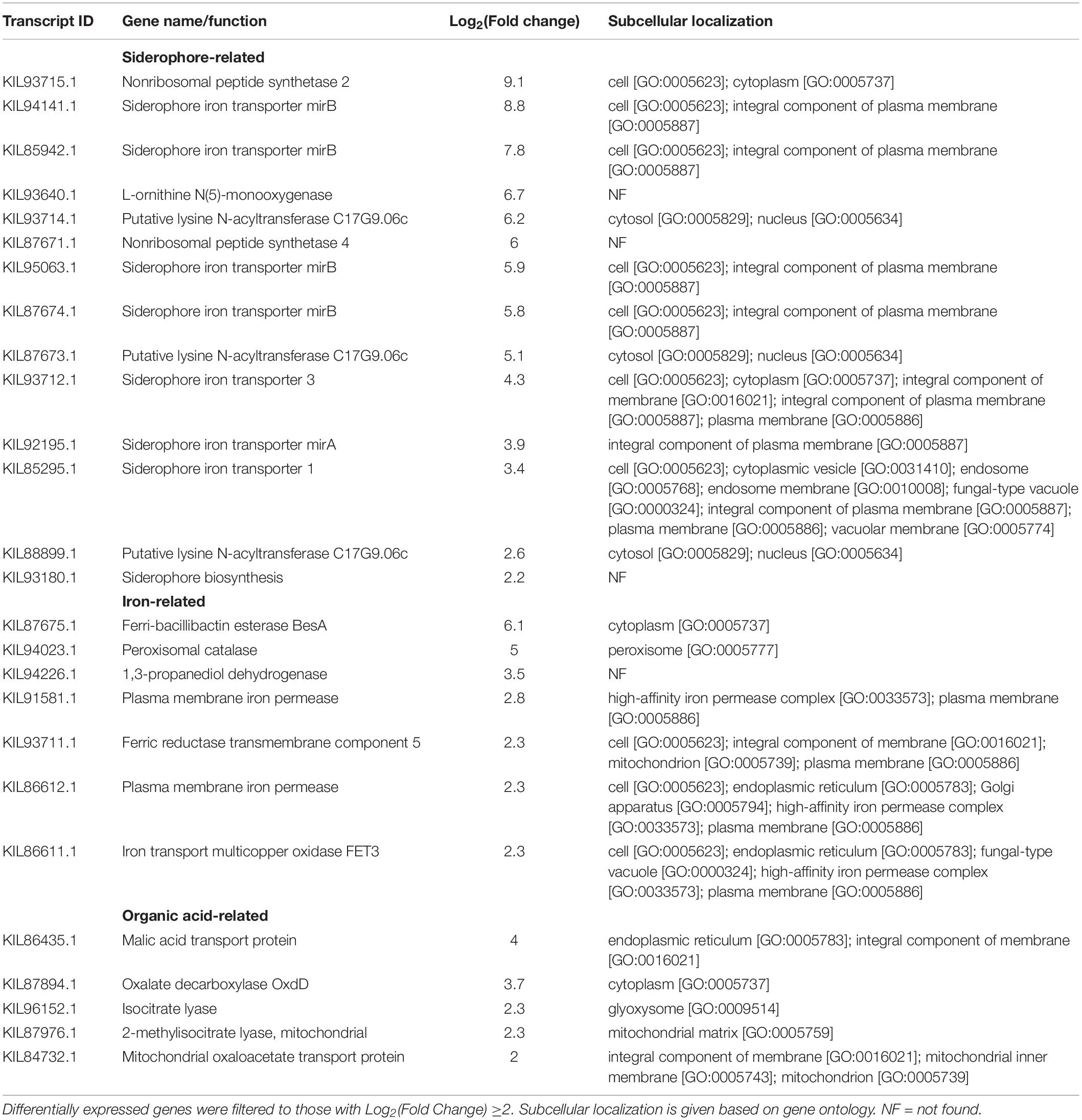
Table 7. Aluminum-induced differentially expressed genes in F. avenaceum F.a.1, in the absence of caryopsis colonization (FOA vs. FOW).
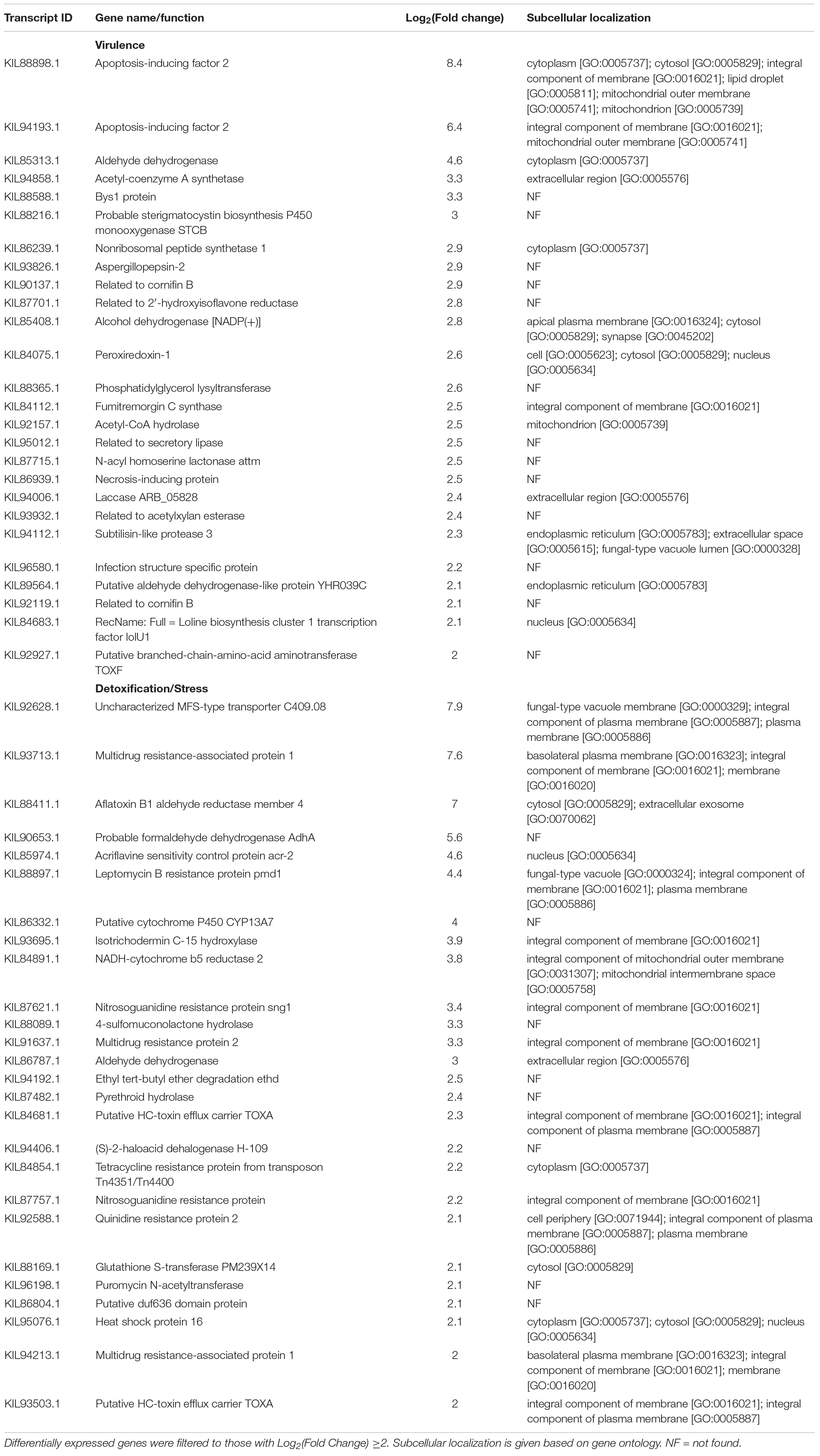
Table 8. Additional aluminum-induced differentially expressed genes in F. avenaceum F.a.1, in the absence of caryopsis colonization (FOA vs. FOW).
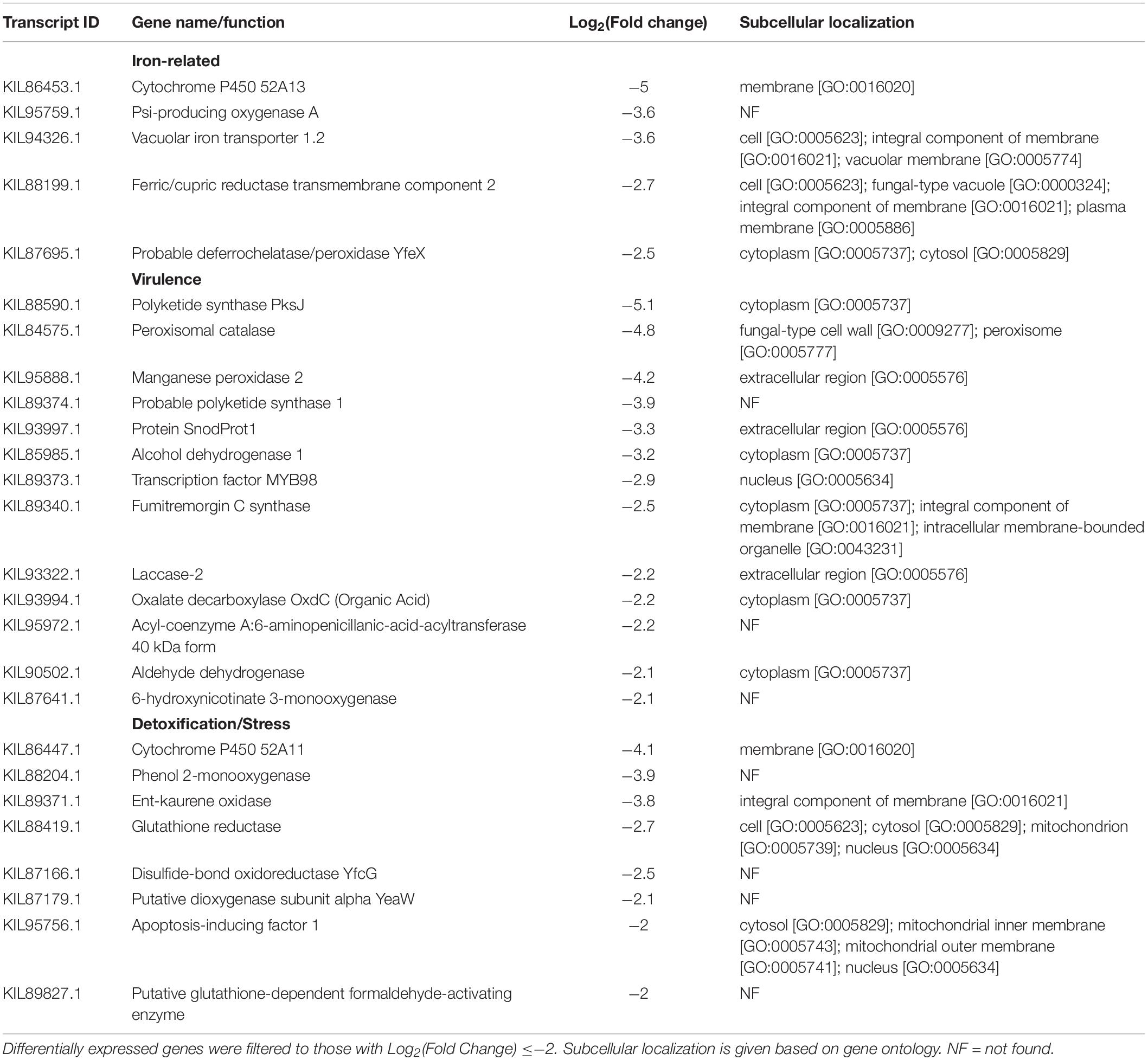
Table 9. Aluminum-repressed differentially expressed genes in F. avenaceum F.a.1 in the absence of caryopsis colonization (FOA vs. FOW).
Gene Ontology (GO) Enrichment and KEGG (Kyoto Encyclopedia of Genes and Genomes) Pathway Analysis
Chronic Aluminum Exposure Influences Gene Ontology Enrichment
Chronic Exposure of F.a.1 to sublethal concentrations of Al (FOA) was associated with changes in the enrichment of many genes from ontology groups associated with biological and molecular processes. Aluminum exposure was related to induction of genes involved in biological and metabolic processes, catalytic activity, single-organism process, single-organism metabolic process, oxidoreductase activity, oxidation-reduction process, organonitrogen compound metabolic process, small molecule metabolic process, organonitrogen compound biosynthetic process, carboxylic acid metabolic process, oxoacid metabolic process, and organic acid metabolic process (Table 10). Aluminum exposure was also related to the repression of genes associated with gene ontology groups, including biological regulation, cation binding, regulation of cellular process, regulation of biological process, metal ion binding, transition metal ion binding, zinc ion binding, nucleic acid binding transcription factor activity, transcription factor activity, and sequence-specific DNA binding (Table 10). Repression was also observed of several iron-related genes in response to Al exposure. KEGG pathway analysis showed Al exposure led to induction of pathways associated with biosynthesis of secondary metabolites, biosynthesis of amino acids, carbon metabolism, 2-oxocarboxylic acid metabolism, cysteine and methionine metabolism, propanoate metabolism, lysine biosynthesis, and, valine, leucine and isoleucine degradation (Table 11).
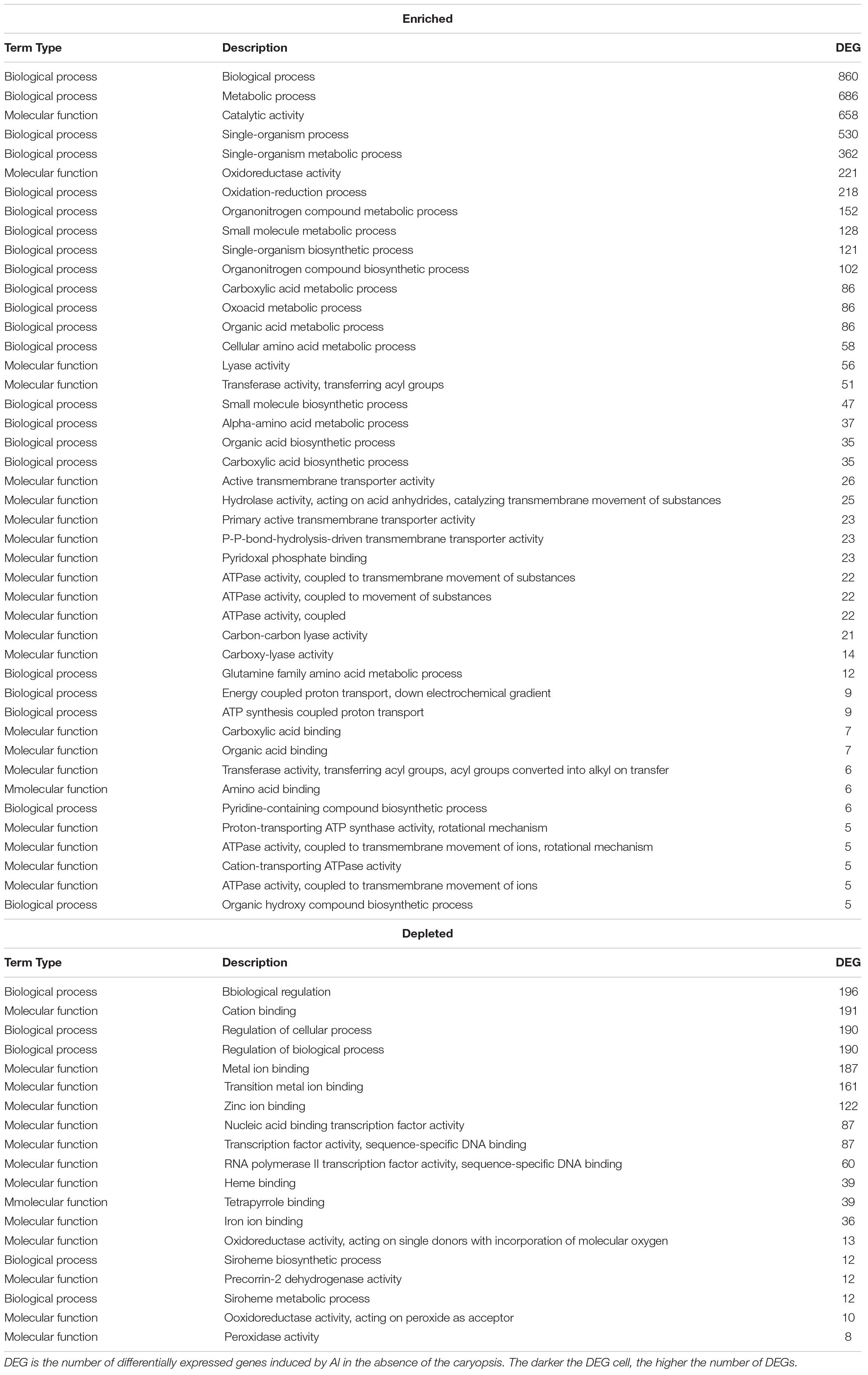
Table 10. Enriched and depleted gene ontology terms associated with Al exposure in the absence of the caryopses (FOA).
Gene Ontology Enrichment Is Influenced by Caryopsis Colonization During Aluminum Exposure
Caryopsis colonization during aluminum exposure (FA) resulted in the induction of gene ontology terms associated with biological, cellular component, and molecular functions. These gene ontology terms were associated with some general biological processes, including ion binding, transport, membrane-related functions, oxidoreductase activity, and lipid metabolic processes (Table 12).

Table 12. Enriched gene ontology terms associated with Al exposure in the presence of caryopses (FA vs. FOA).
KEGG Pathway Analysis During Caryopsis Colonization
Caryopsis colonization (in the absence of Al) was associated with an increase in 23 DEGs associated with carbon metabolism, and an increase in 10 DEGs associated with the tricarboxylic acid cycle (TCA cycle). Additionally, a decrease in 5 DEGs associated with N-Glycan biosynthesis was observed in fungal tissues samples colonizing A. fatua caryopses.
Discussion
Caryopsis colonization resulted in induction of F.a.1 genes associated with virulence, stress/defense, detoxification, organic acid metabolism, basic metabolism, transport, and amino acid/peptide/protein metabolism. At the same time, repression of genes associated with iron metabolism, stress/defense, organic acid metabolism, metal-related metabolism, and basic metabolism was observed. These results suggest a shift in gene expression related to fundamental biological functions occurs during caryopsis colonization. It should be noted that future researchers might consider sampling fungal tissues in a spatial manner that includes sampling the zone of fungal material directly in contact with the caryopsis separately from the remaining hyphal mat. A more spatially refined sampling method might also reveal more information with respect to GO enrichment and KEGG pathway analyses at specific interaction sites.
Nevertheless, the presented results reveal many biological mechanisms associated with caryopsis colonization. Notably, AKR7L: Aflatoxin B1 aldehyde reductase member 4 was strongly induced, suggesting a role for aflatoxin degradation during F. avenaceum caryopsis colonization. Previous work suggests Fusarium and Aspergillus species (which produce aflatoxins) can be the primary fungi associated with post-harvest mycotoxin contaminated wheat and corn (Ali et al., 1998; Del Palacio et al., 2016). Several oxidoreductase genes were induced during caryopsis colonization (Table 1), which is of relevance because the F. avenaceum genome has been shown to be enriched in oxidoreductase genes, several of which were induced during colonization of barley plants (Lysøe et al., 2014). Additionally, in the current study, many genes related to oxidative stress were induced during caryopsis colonization, including peroxisomal catalase and catalase-1 (cat-1). Others have found Fusarium isolates with a greater capacity to cope with oxidative stress also exhibit stronger virulence (Ponts et al., 2009). Together these results suggest oxidoreductase genes are key to F. avenaceum pathogenicity of both seeds and developed plant tissues, likely through mitigating oxidative stress. Further overlap was observed between the results of the current study and those of Lysøe et al., 2014, including induction of the same or related genes, such as, NADH-related genes, an ATP synthase subunit, an extracellular serine-rich protein, heat shock proteins, an iron-sulfur cluster assembly protein, cytochrome p450, and others (Table 1 and Supplementary Table S1). Heat shock protein 90 is required for virulence and development in F. graminearum (Bui et al., 2016), and while this heat shock protein was not induced during caryopsis colonization in the current study, several others were, with the highest expression observed in HSP31 (Table 1); this suggests a role for other heat shock proteins in the virulence of Fusarium species. Other virulence-related genes induced in the current study included ga4 desaturase and ent-kaurene oxidase; both are involved in the production of gibberellic acid, which is also thought to play a role in fungal pathogenesis (Malonek et al., 2005; Chanclud and Morel, 2016).
Many differentially induced genes were unique to the present study as compared with Lysøe et al. (2014), including those involved in the metabolism of the organic acids, oxalate and malate. Additionally, nitrogen metabolism genes were induced during caryopsis colonization, including urea amidolyase and nitrite reductase. Many genes related to carbon metabolism were induced during caryopsis colonization, including STL1 (a sugar transporter), ght1 (a glucose transporter), glcA (glucan endo-1,3-beta-glucosidase A1), grg-1 (glucose-repressible gene protein), and Gpd2 (glycerol-3-phosphate dehydrogenase). In studies with F. graminearum, STL1 has been shown to be involved in interactions with living verses dead wheat tissues, and is thought to be induced by plant signals (Boedi et al., 2016). While the precise role of grg-1 remains unknown in Fusarium species, it is known that glucose repression is intimately linked with fungal-driven cell wall degradation and is necessary for virulence (Tonukari et al., 2000; Ospina-Giraldo et al., 2003). The results suggest a role of grg-1 in A. fatua caryopsis colonization. The gpd2 gene has been shown to be necessary for glycerol utilization, and deletion of the gene results in reduced virulence of Pyricularia oryzae (Shi et al., 2018). Several phosphatase genes were induced during caryopsis colonization, which is of relevance because not only is phosphate turnover central to basic biological functions, but phosphatases have also been shown to be crucial to virulence in F. graminearum (Yun et al., 2015). Another stress-related gene that was induced during caryopsis colonization was sed1, which is thought to be involved in cell wall stability (Hagen et al., 2004).
Bioavailable Al is thought to play a major role in inhibition of many plant pathogenic fungi, including inhibition of virulence and macroconidial germination of Fusarium solani f. sp. phaseoli (Firestone et al., 1983; Kobayashi and Ko, 1985; Meyer et al., 1994; Furuya et al., 1999; Fichtner et al., 2006). Chronic exposure of F.a.1 to sublethal concentrations of Al in the current study resulted in global transcriptomic changes (Figures 3E,F). Notably, Al exposure led to induction of siderophore-, iron-, and organic acid-related genes. Fungal-derived siderophores and organic acids are known to interact with metals (Renshaw et al., 2002; Sullivan et al., 2012). Aluminum exposure-induced alterations in iron metabolism included induction and repression of genes associated with iron transport, suggesting a general disruption in iron metabolism in response to Al exposure. Several genes involved in siderophore biosynthesis were induced during Al exposure, including sidA (L-ornithine N(5)-monooxygenase), which is crucial for viability of Aspergillus nidulans (Eisendle et al., 2003), and is required for full virulence of F. graminearum (Greenshields et al., 2007). The siderophore biosynthesis genes, sidD (NRPS4) and sidC (NRPS2) were also induced in response to Al, and are known to be responsible for synthesis of fusarinine C and ferricrocin, respectively, in Aspergillus fumigatus (Schrettl et al., 2007). In both Cochliobolus heterostrophus and F. graminearum (Gibberella zeae), sidC has been found essential for sexual development, with the phenotype being partially restored in knockout mutants supplemented with iron (Oide et al., 2007). Reduced colony forming unit counts were observed in ΔsidA and ΔsidD mutants of A. fumigatus used to test the role of fungal siderophores in infecting mice corneas (Leal et al., 2013). Additionally, the transporter, mirB was induced in response to Al exposure. It has been shown that mirB is involved exclusively in transporting triacetylfusarinine C (Haas et al., 2003), which has been implicated as playing key roles in iron uptake and virulence of F. graminearum (Oide et al., 2015). Another siderophore transporter, mirA, was also induced by Al toxicity, which has been shown to exclusively transport enterobactin, a bacterial siderophore (Haas et al., 2003). The siderophore transporter sit1, was also induced by Al exposure. Greenshields et al. (2007), found sit1 was induced by low iron, but was not expressed in infected wheat, suggesting a role in iron metabolism, but not necessarily for virulence. Another siderophore transporter that was induced during Al toxicity was str3, which is known to be negatively regulated by iron (Pelletier et al., 2003), and is thought to be crucial for low-affinity heme acquisition by Schizosaccharomyces pombe (Normant et al., 2018). A transcript having 93.4% sequence similarity to besA, ferri-bacillibactin esterase, was also induced in response to Al exposure. The besA sequence has also been identified in F. oxysporum (Guo et al., 2014), while, in Bacillus species, the besA protein is involved in liberating iron from Fe-bacillibactin complexes (Abergel et al., 2009). In the context of the findings presented here, an analogous function for the protein may occur in fungal species, as well.
Organic acids are known to play important roles in metal availability through complexation with metal ions, which renders the metal less bioavailable (Jones, 1998), and the phenomenon has been observed in fungi. For instance, oxalic acid production is responsible for zinc and copper tolerance in Aspergillus niger and Penicillium citrinum, and A. niger isolated from lead contaminated soils has been found to secrete large amounts of organic acids (Sullivan et al., 2012; Sazanova et al., 2015). Additionally, malate exudation by Penicillum oxalicum was suggested to be involved in phosphate liberation from AlPO4, FePO4, and Ca3(PO4)2 (Gadagi et al., 2007). These findings, along with the fact that malate is thought to be responsible for Al tolerance in plants (Delhaize and Ryan, 1995; Ryan et al., 1995; Klugh-Stewart and Cumming, 2009), suggests the induction of malate transporters observed in F.a.1 exposed to Al is likely related to modulating Al bioavailability.
Gene ontology and KEGG pathway analyses also showed Al exposure was associated with alterations in basic biological processes, including induction of genes related to oxidoreductase activity, oxidation-reduction process, organic acid metabolism, biosynthesis of secondary metabolites, biosynthesis of amino acids, carbon metabolism, 2-oxocarboxylic acid metabolism, and cysteine and methionine metabolism. Alterations in these disparate biological processes suggests Al toxicity results in changes to the basic metabolism of the fungus. Gene ontology analyses also suggest that uniquely expressed DEGs might also be involved in unique biological processes (Supplementary Table S7), however, these data were filtered to remove GO terms with less than 5 DEGs to identify potentially unique biological functions associated with the uniquely expressed genes. The unfiltered results showing the number of unique DEGs associated with GO terms can be found in Supplementary Table S8.
These results have significance regarding the currently expanding global issue of soil acidification, and associated Al toxicity. It is unclear how soil fungi, particularly pathogens, cope with acid soils and the metals that become toxic at lower pH levels in acidified soils, at the molecular level. The results presented here suggest siderophores and organic acids are likely involved in Al toxicity responses in F.a.1. Which, in turn, brings attention to the fact that these interactions in soils and their impacts on weed seed decay have been overlooked to date. The transcriptomic responses of F.a.1 to Al suggest that Al toxicity results in dramatic changes in iron metabolism, siderophore metabolism, and organic acid metabolism, simultaneously. This is a key finding because iron homeostasis has been shown to be essential for full virulence of the related pathogenic fungus, F. oxysporum (López-Berges et al., 2012). Additionally, siderophore production is known to be important in plant-pathogen interactions, including the pathogenic activity and sexual development of F. graminearum (Greenshields et al., 2007; Oide et al., 2015). A future direction might include examining the influence of Al (and other metals) on competitiveness and virulence of F.a1 in soils.
The transcriptome of F.a.1 provided insights regarding the interactions between an oat fungal pathogen, its susceptible host, and aluminum challenge. A complex hierarchy of gene expression produced by aluminum challenge, superseded that of the host interaction. Understanding genes involved in Al tolerance may be utilized to promote fungal activity under high Al soil conditions. Additionally, better understanding of fungus-Al interactions could also assist identification and development of plant growth-promoting fungi that can tolerate increased Al bioavailability. Key genes associated with pathogen growth and life cycle, including the formation of spores, conidia, and infection structures were revealed (Table 6 and Supplementary Tables S2, S3). These genes are promising candidates for host-induced gene silencing (HIGS), an RNAi-based approach for pathogen suppression that has been deployed against Fusarium spp. (reviewed in Okubara et al., 2019). Additionally, these genes (and others identified here) may be utilized in identifying other fungal strains that may be even more effective in causing weed seedbank decay. Future work might include applying a multi-omic approach to further elucidate and clarify molecular mechanisms of caryopsis colonization and aluminum toxicity in F. avenaceum, including metabolomic and proteomic approaches. Additionally, future work could aim to examine the subcellular localization of the proteins involved in caryopsis colonization and Al toxicity to reveal how Al and seed colonization influence the subcellular organization of important metabolites, proteins, and metals.
Data Availability Statement
The RNA sequence datasets generated for this study can be found through the Sequence Read Archive (SRA; SRA accession: PRJNA595343).
Author Contributions
RL conceived and performed the experiments, analyzed the data, and was the primary author of the manuscript. PO assisted with experimental design, data analysis, and writing of the manuscript. EF assisted with experimental design, determined PPO activity, and assisted with editing the manuscript. RH assisted with experimental design, extracted RNA, and provided text regarding the RNA extraction protocol. DG assisted with experimental design. TS assisted with experimental design and editing the manuscript.
Funding
This project was funded by the USDA National Institute of Food and Agriculture (Agriculture and Food Research Initiative), 2013-02322 (Award Number: 2014- 67013-21575), and CRIS Project No. 2090-22000-017-00D (PO).
Conflict of Interest
The authors declare that the research was conducted in the absence of any commercial or financial relationships that could be construed as a potential conflict of interest.
Acknowledgments
The authors would like to thank Nandan Utgikar for assistance completing the initial stages of the experiment, and Anne T. Pollard for growing and providing seed.
Supplementary Material
The Supplementary Material for this article can be found online at: https://www.frontiersin.org/articles/10.3389/fmicb.2020.00051/full#supplementary-material
References
Abergel, R. J., Zawadzka, A. M., Hoette, T. M., and Raymond, K. N. (2009). Enzymatic hydrolysis of trilactone siderophores: where chiral recognition occurs in enterobactin and bacillibactin iron transport. J. Am. Chem. Soc. 131, 12682–12692. doi: 10.1021/ja903051q
Ali, N., Sardjono, Yamashita, A., and Yoshizawa, T. (1998). Natural co-occurrence of aflatoxins and Fusarium mycotoxins (fumonisins, deoxynivalenol, nivalenol and zearalenone) in corn from Indonesia. Food Addit. Contam. 15, 377–384. doi: 10.1080/02652039809374655
Anders, S., and Huber, W. (2010). Differential expression analysis for sequence count data. Genome Biol. 11:R106. doi: 10.1186/gb-2010-11-10-r106
Anders, S., Pyl, P. T., and Huber, W. (2015). HTSeq–a Python framework to work with high-throughput sequencing data. Bioinformatics 31, 166–169. doi: 10.1093/bioinformatics/btu638
Anderson, J. V., Fuerst, E. P., Tedrow, T., Hulke, B., and Kennedy, A. C. (2010). Activation of polyphenol oxidase in dormant wild oat caryopses by a seed-decay isolate of Fusarium avenaceum. J. Agric. Food Chem. 58, 10597–10605. doi: 10.1021/jf102625a
Beckie, H. J., Francis, A., and Hall, L. M. (2012). The biology of Canadian weeds. 27. Avena fatua L. (updated). Can. J. Plant Sci. 92, 1329–1357. doi: 10.4141/cjps2012-005
Boedi, S., Berger, H., Sieber, C., Münsterkötter, M., Maloku, I., Warth, B., et al. (2016). Comparison of Fusarium graminearum transcriptomes on living or dead wheat differentiates substrate-responsive and defense-responsive genes. Front. Microbiol. 7:1113. doi: 10.3389/fmicb.2016.01113
Bui, D.-C., Lee, Y., Lim, J. Y., Fu, M., Kim, J.-C., Choi, G. J., et al. (2016). Heat shock protein 90 is required for sexual and asexual development, virulence, and heat shock response in Fusarium graminearum. Sci. Rep. 6:28154. doi: 10.1038/srep28154
Chanclud, E., and Morel, J.-B. (2016). Plant hormones: a fungal point of view. Mol. Plant Pathol. 17, 1289–1297. doi: 10.1111/mpp.12393
Coordinators, N. R. (2016). Database resources of the national center for biotechnology information. Nucleic Acids Res. 44, D7–D19. doi: 10.1093/nar/gkv1290
de Luna, L. Z., Kennedy, A. C., Hansen, J. C., Paulitz, T. C., Gallagher, R. S., and Fuerst, E. P. (2011). Mycobiota on wild oat (Avena fatua L.) seed and their caryopsis decay potential. Plant Health Prog. 12:20. doi: 10.1094/php-2011-0210-01-rs
Del Palacio, A., Bettucci, L., and Pan, D. (2016). Fusarium and Aspergillus mycotoxins contaminating wheat silage for dairy cattle feeding in Uruguay. Braz. J. Microbiol. 47, 1000–1005. doi: 10.1016/j.bjm.2016.06.004
Delhaize, E., and Ryan, P. R. (1995). Aluminum toxicity and tolerance in plants. Plant Physiol. 107, 315–321.
Eddy, S. R. (2011). Accelerated profile HMM searches. PLoS Comput. Biol. 7:e1002195. doi: 10.1371/journal.pcbi.1002195
Eisendle, M., Oberegger, H., Zadra, I., and Haas, H. (2003). The siderophore system is essential for viability of Aspergillus nidulans: functional analysis of two genes encoding l-ornithine N 5-monooxygenase (sidA) and a non-ribosomal peptide synthetase (sidC). Mol. Microbiol. 49, 359–375. doi: 10.1046/j.1365-2958.2003.03586.x
Fichtner, E., Hesterberg, D., Smyth, T., and Shew, H. (2006). Differential sensitivity of Phytophthora parasitica var. nicotianae and Thielaviopsis basicola to monomeric aluminum species. Phytopathology 96, 212–220. doi: 10.1094/PHYTO-96-0212
Firestone, M., Killham, K., and McColl, J. (1983). Fungal toxicity of mobilized soil aluminum and manganese. Appl. Environ. Microbiol. 46, 758–761.
Foy, C. D. (1984). “Physiological effects of hydrogen, aluminum, and manganese toxicities in acid soil,” in Soil Acidity and Liming, ed. F. Adams (Madison, WI: American Society of Agronomy, Crop Science Society of America, Soil Science Society of America), 57–97.
Fuerst, E. P., Anderson, J. V., Kennedy, A. C., and Gallagher, R. S. (2011). Induction of polyphenol oxidase activity in dormant wild oat (Avena fatua) seeds and caryopses: a defense response to seed decay fungi. Weed Sci. 59, 137–144.
Fuerst, E. P., James, M. S., Pollard, A. T., and Okubara, P. A. (2018). Defense enzyme responses in dormant wild oat and wheat caryopses challenged with a seed decay pathogen. Front. Plant Sci. 8:2259. doi: 10.3389/fpls.2017.02259
Fuerst, E. P., Okubara, P. A., Anderson, J. V., and Morris, C. F. (2014). Polyphenol oxidase as a biochemical seed defense mechanism. Front. Plant Sci. 5:689. doi: 10.3389/fpls.2014.00689
Furuya, H., Takahashi, T., and Matsumoto, T. (1999). Suppression of Fusarium solani f. sp. phaseoli on bean by aluminum in acid soils. Phytopathology 89, 47–52. doi: 10.1094/PHYTO.1999.89.1.47
Gadagi, R., Shin, W., and Sa, T. (2007). “Malic acid mediated aluminum phosphate solubilization by Penicillium oxalicum CBPS-3F-Tsa isolated from Korean paddy rhizosphere soil,” in First International Meeting on Microbial Phosphate Solubilization, eds E. Velázquez and C. Rodríguez-Barrueco (Dordrecht: Springer), 285–290.
Glick, B. R. (2010). Using soil bacteria to facilitate phytoremediation. Biotechnol. Adv. 28, 367–374. doi: 10.1016/j.biotechadv.2010.02.001
Greenshields, D. L., Liu, G., Feng, J., Selvaraj, G., and Wei, Y. (2007). The siderophore biosynthetic gene SID1, but not the ferroxidase gene FET3, is required for full Fusarium graminearum virulence. Mol. Plant Pathol. 8, 411–421. doi: 10.1111/j.1364-3703.2007.00401.x
Guo, L., Han, L., Yang, L., Zeng, H., Fan, D., Zhu, Y., et al. (2014). Genome and transcriptome analysis of the fungal pathogen Fusarium oxysporum f. sp. cubense causing banana vascular wilt disease. PLoS One 9:e95543. doi: 10.1371/journal.pone.0095543
Haas, H., Schoeser, M., Lesuisse, E., Ernst, J. F., Parson, W., Abt, B., et al. (2003). Characterization of the Aspergillus nidulans transporters for the siderophores enterobactin and triacetylfusarinine C. Biochem. J. 371(Pt 2), 505–513. doi: 10.1042/BJ20021685
Hagen, I., Ecker, M., Lagorce, A., Francois, J. M., Sestak, S., Rachel, R., et al. (2004). Sed1p and Srl1p are required to compensate for cell wall instability in Saccharomyces cerevisiae mutants defective in multiple GPI-anchored mannoproteins. Mol. Microbiol. 52, 1413–1425. doi: 10.1111/j.1365-2958.2004.04064.x
Jones, D., Dennis, P., Owen, A., and Van Hees, P. (2003). Organic acid behavior in soils–misconceptions and knowledge gaps. Plant Soil 248, 31–41.
Kanehisa, M., and Goto, S. (2000). KEGG: Kyoto encyclopedia of genes and genomes. Nucleic Acids Res. 28, 27–30.
Klugh-Stewart, K., and Cumming, J. R. (2009). Organic acid exudation by mycorrhizal Andropogon virginicus L. (broomsedge) roots in response to aluminum. Soil Biol. Biochem. 41, 367–373. doi: 10.1016/j.soilbio.2008.11.013
Kobayashi, N., and Ko, W. H. (1985). Nature of suppression of Rhizoctonia solani in Hawaiian soils. Trans. Br. Mycol. Soc. 84, 691–694. doi: 10.1016/S0007-1536(85)80125-X
Leal, S. M. Jr., Roy, S., Vareechon, C., Carrion, S. D., Clark, H., Lopez-Berges, M. S., et al. (2013). Targeting iron acquisition blocks infection with the fungal pathogens Aspergillus fumigatus and Fusarium oxysporum. PLoS Pathog. 9:e1003436. doi: 10.1371/journal.ppat.1003436
Lewis, R. W., Barth, V. P., Coffey, T., McFarland, C., Huggins, D. R., and Sullivan, T. S. (2018). Altered bacterial communities in long-term no-till soils associated with stratification of soluble aluminum and soil pH. Soil Syst. 2:7.
López-Berges, M. S., Capilla, J., Turrà, D., Schafferer, L., Matthijs, S., Jöchl, C., et al. (2012). Hapx-mediated iron homeostasis is essential for rhizosphere competence and virulence of the soilborne pathogen Fusarium oxysporum. Plant Cell 24, 3805–3822. doi: 10.1105/tpc.112.098624
Lysøe, E., Harris, L. J., Walkowiak, S., Subramaniam, R., Divon, H. H., Riiser, E. S., et al. (2014). The genome of the generalist plant pathogen Fusarium avenaceum is enriched with genes involved in redox, signaling and secondary metabolism. PLoS One 9:e112703. doi: 10.1371/journal.pone.0112703
Malonek, S., Rojas, M. C., Hedden, P., Hopkins, P., and Tudzynski, B. (2005). Restoration of gibberellin production in Fusarium proliferatum by functional complementation of enzymatic blocks. Appl. Environ. Microbiol. 71, 6014–6025. doi: 10.1128/aem.71.10.6014-6025.2005
Meyer, J., Shew, H., and Harrison, U. (1994). Inhibition of germination and growth of Thielaviopsis basicola by aluminum. Phytopathology 84, 598–602.
Normant, V., Mourer, T., and Labbé, S. (2018). The major facilitator transporter Str3 is required for low-affinity heme acquisition in Schizosaccharomyces pombe. J. Biol. Chem. 293, 6349–6362. doi: 10.1074/jbc.RA118.002132
Oide, S., Berthiller, F., Wiesenberger, G., Adam, G., and Turgeon, B. G. (2015). Individual and combined roles of malonichrome, ferricrocin, and TAFC siderophores in Fusarium graminearum pathogenic and sexual development. Front. Microbiol. 5:759. doi: 10.3389/fmicb.2014.00759
Oide, S., Krasnoff, S. B., Gibson, D. M., and Turgeon, B. G. (2007). Intracellular siderophores are essential for ascomycete sexual development in heterothallic Cochliobolus heterostrophus and homothallic Gibberella zeae. Eukaryot. Cell 6, 1339–1353. doi: 10.1128/ec.00111-07
Okubara, P. A., Peetz, A. B., and Sharpe, R. M. (2019). Cereal root interactions with soilborne pathogens—from trait to gene and back. Agronomy 9:188.
Ospina-Giraldo, M. D., Mullins, E., and Kang, S. (2003). Loss of function of the Fusarium oxysporum SNF1 gene reduces virulence on cabbage and Arabidopsis. Curr. Genet. 44, 49–57. doi: 10.1007/s00294-003-0419-y
Pelletier, B., Beaudoin, J., Philpott, C. C., and Labbé, S. (2003). Fep1 represses expression of the fission yeast Schizosaccharomyces pombe siderophore-iron transport system. Nucleic Acids Res. 31, 4332–4344.
Pimentel, D., McNair, S., Janecka, J., Wightman, J., Simmonds, C., O’Connell, C., et al. (2001). Economic and environmental threats of alien plant, animal, and microbe invasions. Agric. Ecosyst. Environ. 84, 1–20. doi: 10.1016/S0167-8809(00)00178-X
Pollard, A. T. (2018). Seeds vs fungi: an enzymatic battle in the soil seedbank. Seed Sci. Res. 28, 197–214. doi: 10.1017/S0960258518000181
Ponts, N., Couedelo, L., Pinson-Gadais, L., Verdal-Bonnin, M.-N., Barreau, C., and Richard-Forget, F. (2009). Fusarium response to oxidative stress by H2O2 is trichothecene chemotype-dependent. FEMS Microbiol. Lett. 293, 255–262. doi: 10.1111/j.1574-6968.2009.01521.x
Renshaw, J. C., Robson, G. D., Trinci, A. P. J., Wiebe, M. G., Livens, F. R., Collison, D., et al. (2002). Fungal siderophores: structures, functions and applications. Mycol. Res. 106, 1123–1142. doi: 10.1017/S0953756202006548
Ryan, P., Delhaize, E., and Randall, P. (1995). Malate efflux from root apices and tolerance to aluminium are highly correlated in wheat. Funct. Plant Biol. 22, 531–536. doi: 10.1071/PP9950531
Sazanova, K., Osmolovskaya, N., Schiparev, S., Yakkonen, K., Kuchaeva, L., and Vlasov, D. (2015). Organic acids induce tolerance to zinc-and copper-exposed fungi under various growth conditions. Curr. Microbiol. 70, 520–527. doi: 10.1007/s00284-014-0751-0
Schrettl, M., Bignell, E., Kragl, C., Sabiha, Y., Loss, O., Eisendle, M., et al. (2007). Distinct roles for intra- and extracellular siderophores during Aspergillus fumigatus infection. PLoS Pathog. 3:e128. doi: 10.1371/journal.ppat.0030128
Schroder, J. L., Zhang, H., Girma, K., Raun, W. R., Penn, C. J., and Payton, M. E. (2011). Soil acidification from long-term use of nitrogen fertilizers on winter wheat. Soil Sci. Soc. Am. J. 75, 957–964. doi: 10.2136/sssaj2010.0187
Shi, Y., Wang, H., Yan, Y., Cao, H., Liu, X., Lin, F., et al. (2018). Glycerol-3-phosphate shuttle is involved in development and virulence in the rice blast fungus Pyricularia oryzae. Front. Plant Sci. 9:687. doi: 10.3389/fpls.2018.00687
Sullivan, T. S., Gottel, N. R., Basta, N., Jardine, P. M., and Schadt, C. W. (2012). Firing range soils yield a diverse array of fungal isolates capable of organic acid production and Pb mineral solubilization. Appl. Environ. Microbiol. 78, 6078–6086. doi: 10.1128/AEM.01091-12
Tonukari, N. J., Scott-Craig, J. S., and Waltonb, J. D. (2000). The Cochliobolus carbonum SNF1 gene is required for cell wall–degrading enzyme expression and virulence on maize. Plant Cell 12, 237–247. doi: 10.1105/tpc.12.2.237
Trapnell, C., Pachter, L., and Salzberg, S. L. (2009). TopHat: discovering splice junctions with RNA-Seq. Bioinformatics 25, 1105–1111. doi: 10.1093/bioinformatics/btp120
UniProt Consortium (2018). UniProt: a worldwide hub of protein knowledge. Nucleic Acids Res. 47, D506–D515. doi: 10.1093/nar/gky1049
von Uexküll, H. R., and Mutert, E. (1995). Global extent, development and economic impact of acid soils. Plant Soil 171, 1–15. doi: 10.1007/bf00009558
Walkowiak, S., Rowland, O., Rodrigue, N., and Subramaniam, R. (2016). Whole genome sequencing and comparative genomics of closely related Fusarium head blight fungi: Fusarium graminearum, F. meridionale and F. asiaticum. BMC Genomics 17:1014. doi: 10.1186/s12864-016-3371-1
Keywords: wild oat, Fusarium, weed seed decay, fungal siderophore, soil acidification, sublethal aluminum toxicity, plant pathogens, soil microbiology
Citation: Lewis RW, Okubara PA, Fuerst EP, He R, Gang D and Sullivan TS (2020) Chronic Sublethal Aluminum Exposure and Avena fatua Caryopsis Colonization Influence Gene Expression of Fusarium avenaceum F.a.1. Front. Microbiol. 11:51. doi: 10.3389/fmicb.2020.00051
Received: 07 October 2019; Accepted: 10 January 2020;
Published: 04 February 2020.
Edited by:
Fred Asiegbu, University of Helsinki, FinlandReviewed by:
Thomas Nussbaumer, Helmholtz-Gemeinschaft Deutscher Forschungszentren (HZ), GermanyWilliam Edward Dyer, Montana State University, United States
Copyright © 2020 Lewis, Okubara, Fuerst, He, Gang and Sullivan. This is an open-access article distributed under the terms of the Creative Commons Attribution License (CC BY). The use, distribution or reproduction in other forums is permitted, provided the original author(s) and the copyright owner(s) are credited and that the original publication in this journal is cited, in accordance with accepted academic practice. No use, distribution or reproduction is permitted which does not comply with these terms.
*Correspondence: Ricky W. Lewis, cmlja3kudy5sZXdpc0BnbWFpbC5jb20=; Tarah S. Sullivan, dC5zdWxsaXZhbkB3c3UuZWR1